DOI:
10.1039/D4DT03139C
(Paper)
Dalton Trans., 2025,
54, 1956-1963
Na2B12Si6Se18: a novel B12-cluster-containing quaternary selenoborate framework material†
Received
8th November 2024
, Accepted 3rd December 2024
First published on 9th December 2024
Abstract
We report the successful synthesis of the new quaternary, three-dimensional Na2Si6B12Se18 material using a solid-state synthesis route. This compound is characterized by a unique framework structure that features the rare icosahedral [B12]10+ cation which is connected via Si2Se6 polyhedral units into a 3D framework structure with Na+ ions located inside the channels. The Na2Si6B12Se18 compound crystallizes in the cubic crystal system in the space group Im
, with lattice parameter a = 11.91110(10) Å and unit cell volume of 1689.88(4) Å3. The 3D framework of the [Si6B12Se18]2− anion contains B12 icosahedra with six of their edges bridged by tetrahedral SiSe4 groups. The B12 icosahedral units are connected by the SiSe4 tetrahedral units which bridge between each B12 icosahedron. Ultraviolet–Visible (UV-vis) spectroscopy revealed that Na2Si6B12Se18 exhibits semiconducting behavior with an estimated bandgap of 2.1(1) eV. In situ powder X-ray diffraction (PXRD) studies were conducted to investigate the phase formation of this compound as a function of temperature to obtain information of its thermal stability and structural evolution.
Introduction
The linkage of SiQ4 (Q = S, Se or Te) tetrahedra forms a wide variety of network structures, as observed in numerous thio- and selenosilicate compounds. These structures are not only intriguing from a structural perspective but also hold significant potential for various applications in materials science and technology. The synthesis of compounds that incorporate SiQ4 units as bridges between B12 clusters is particularly fascinating and represents an area of great interest within the field of solid-state inorganic chemistry. Boron is often found in electron deficient compounds, most notably the boron hydrides, where the electron deficiency significantly influences the chemistry and leads to the formation of various intriguing cluster species.1 One notable class of these clusters is the closo-borane anions (B12H122−), which are known for their remarkable chemical stability to both air and moisture.2,3 This stability arises from the large energy gap between the highest occupied molecular orbital (HOMO) and the lowest unoccupied molecular orbital (LUMO), making these compounds less reactive under ambient conditions. In recent years, the synthesis of various thio- and selenoborates has advanced considerably due to modern synthetic techniques. These compounds, characterized by boron–chalcogen bonds, form the chalcoborate class of materials and exhibit diverse structural motifs. Typically, in these structures, the boron atom (B3+) is found in either a trigonal or tetrahedral coordination environment.4–7 Examples of non-oxide chalcoborates where B3+ is present in such coordination environments include the compounds M8[B12(BSe3)6], M4Hg2[B12(BSe3)6], BaB2Se6, SrB2S4, CsBSe3 and others.8–13 These compounds highlight the versatility of boron-chalcogen bonding in forming complex structures. The structural motifs in binary, ternary, and quaternary boron chalcogenides often include units such as B8Q16, (BQ2)n, (BQ3)3−, and (B2Q5)2−(Q = S or Se).4–7,14 In some cases, as observed in compounds like Li9B19S33 and Li4−2xSr2+xB10S19 (where x = 0.27), the structure is defined by corner-sharing BS4 tetrahedral units.15 These examples illustrate the complexity and richness of boron-chalcogen chemistry, which continues to be an area of significant interest in materials science and inorganic chemistry.
Boron chalcogenides are not only expanding the landscape of structural chemistry but are also playing a pivotal role in guiding the discovery of new materials with significant potential for applications; for example, these compounds are at the forefront of research into high-performance IR birefringent materials, advanced nonlinear optical materials, and promising solid state electrolytes.16–20
In addition to the intriguing structures and properties of boron chalcogenides containing SiQ4 tetrahedra and B12 clusters, it is noteworthy that very few compounds have been reported in the quaternary systems involving these components. Through an extensive literature survey, we identified only one quaternary compound, CrSi3(B12)Se12, within the M–Si–B–Q (where M represents alkali, alkaline earth, or transition metals, and Q refers to S, Se, and Te) system.21 This scarcity of reported compounds highlights the novelty and significance of our findings.
In this study, we report the synthesis of a new quaternary framework structure, Na2B12Si6Se18, within the A–B–Si–Q (A = alkali or alkaline earth metal) system, marking the first discovery in this series. We successfully synthesized both high-quality single crystals and phase-pure polycrystalline materials of Na2B12Si6Se18. The physical properties of this compound were investigated using various analytical techniques, including single crystal X-ray diffraction, powder X-ray diffraction, thermogravimetric analysis (TGA), differential scanning calorimetry (DSC), and UV-Vis spectroscopy. Moreover, we conducted a detailed in situ X-ray diffraction study of the formation of Na2B12Si6Se18 to gain a deeper understanding of the temperature dependence of its phase formation process from the precursor reactants. This comprehensive analysis not only provides insights into the synthesis conditions required for the formation of Na2B12Si6Se18, but also contributes valuable knowledge to the field of solid-state inorganic chemistry, potentially paving the way for the discovery of other novel quaternary compounds in this system.
Experimental section
Eu2O3 (99.9%, Alfa Aesar), selenium powder (99.999% Fisher scientific), U3O8 (International Bio-Analytical Industries, Inc.,), boron (crystalline 100 mesh, 99.9%, Beantown Chemicals), SiO2 (amorphous powder, 99.9%, Alfa Aesar), NaCl (99.9%, Alfa Aesar) and CaCl2 (99.9%, Alfa Aesar) were utilized. The NaCl–CaCl2 eutectic mixture used for synthesis was stored in a drying oven set to 260 °C to maintain the anhydrous state of the salts.
Synthesis of EuSe
EuSe was synthesized by combining 600 mg of Eu2O3, 300 mg of Se, and 80 mg of B in a sealed, evacuated silica tube. The reactant mixture was subjected to heating at 900 °C for 24 hours, resulting in the formation of EuSe. This material served as a precursor for subsequent reactions.22,23
Single crystal synthesis of Na2B12Si6Se18
During an attempt to synthesize a pentanary compound with the target composition CaEuUSi2Se8, Na2B12Si6Se18 was unexpectedly obtained. The reaction yielded light yellow, transparent crystals along with black and red impurities (see Fig. S1 and S2†). The Scanning electron microscopy (SEM) image of this light yellow crystal and its Energy Dispersive X-ray Spectroscopy (EDS) plot are provided in the ESI file (Fig. S1, S2 and Table. S1†). Additional details about the optimization of the synthesis conditions can be found in the ESI.†
The optimized reaction conditions for crystal growth are: 50 mg of EuSe, 20 mg of boron (B), and 90 mg of selenium (Se), supplemented with 75 mg of a NaCl/CaCl2 flux mixture was sealed in a carbon coated fused silica tube and heated to 800 °C over a period of 12 hours, held at this temperature for 48 hours, and then gradually cooled to 550 °C over a period of 48 hours. Under these conditions, the transparent Na2B12Si6Se18 crystals consistently form.
Synthesis of polycrystalline Na2B12Si6Se18 compound
To obtain a phase-pure Na2B12Si6Se18 sample, a stoichiometric mixture of Na2Se, Si, B, and Se (in the molar ratio of 1
:
6
:
12
:
17) was prepared. This mixture was sealed in a carbon coated fused silica tube and heated to 800 °C over a period of 12 hours, dwelling at 800 °C for 48 hours, and then cooling to 550 °C over another 48 hours before allowing the tube to return to room temperature. This method successfully yielded phase-pure Na2B12Si6Se18. The EDS analyses of the polycrystalline Na2B12Si6Se18 samples are shown in Fig. S3, S4 and Table S2.†
Caution:
Boron selenides are prone to moisture sensitivity, leading to the generation of H
2
Se gas upon contact with water or moisture. Therefore, all reaction procedures must be conducted within fume hoods, with strict adherence to safety protocols.
Single crystal X-ray diffraction (SCXRD)
X-ray intensity data from a block shaped light yellow transparent crystal of Na2B12Si6Se18 were collected at 299(2) K using a Bruker D8 QUEST diffractometer equipped with a PHOTON-II area detector and an Incoatec microfocus source (Mo Kα radiation, λ = 0.71073 Å).24 The detector to crystal distance of 40 mm was fixed, and an exposure time of 10 s per frame was used for the data collection. The raw area detector data frames were reduced and corrected for absorption effects using the SAINT+ and SADABS programs.25 Final unit cell parameters were determined by least-squares refinement over a large number (>5500) of reflections taken from the data set. An initial structural model was obtained with SHELXT.26 Subsequent difference Fourier calculations and full-matrix least-squares refinement against F2 were performed with SHELXL-2018.27
The Na2B12Si6Se18 compound crystallizes in the space group Im
of the cubic crystal system. The asymmetric unit consists of two selenium, one silicon, one boron, and one sodium atomic positions. Se1 and B1 atoms are located on mirror planes (Wyckoff site 24g), Se2 and Si1 atoms are located on mm2.. planes (Wyckoff site 12e and 12d), and the Na present on .–3. symmetry (Wyckoff site 8c). The occupancy of the Na atom was determined to be 0.49 during free refinement of the crystal structure and subsequently fixed at 0.50 for charge balance. All atoms were refined with anisotropic displacement parameters. Crystallographic and refinement data pertinent to the title compound are provided in Table 1.
Table 1 Crystallographic data for Na2B12Si6Se18
Crystal data |
|
Chemical formula |
Na2B12Si6Se18 |
Formula weight |
1765.38 |
Crystal system, space group |
Cubic, Im![[3 with combining macron]](https://www.rsc.org/images/entities/char_0033_0304.gif) |
Temperature (K) |
299(2) |
a (Å) |
11.91110(10) |
V (Å3) |
1689.88(4) |
Z
|
2 |
Radiation type |
Mo Kα |
μ (mm−1) |
19.66 |
Crystal size (mm) |
0.31 × 0.06 × 0.03 |
T
min, Tmax |
0.353, 0.494 |
No. of measured, independent and observed [I > 2σ(I)] reflections |
6350, 531, 477 |
R
int
|
0.028 |
R[F2 > 2σ(F2)], wR(F2), S |
0.020, 0.046, 1.08 |
Δρmax, Δρmin (e Å−3) |
1.53, −0.48 |
Powder X-ray diffraction (PXRD)
Powder X-ray diffraction (PXRD) data were obtained by analyzing finely ground powder samples of Na2B12Si6Se18 prepared from stoichiometric amounts of reactants in a solid state reaction. PXRD data were collected on a Bruker D2 PHASER diffractometer using Cu Kα radiation (λ = 1.5418 Å) over the 2θ range 5–65° with a step size of 0.02°. The observed reflections of the PXRD patterns were indexed based on the simulated PXRD patterns obtained from the single crystal data. Fig. 1.
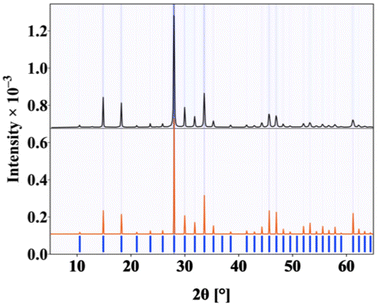 |
| Fig. 1 The PXRD patterns of polycrystalline Na2B12Si6Se18 compound (simulated pattern (red), experimental pattern (black) and Bragg position (blue)). | |
Energy-dispersive X-ray spectroscopy (EDS)
EDS data were collected on single crystals by mounting them directly onto an SEM stub using conducting carbon tape. Quantitative elemental analysis was performed utilizing a Tescan Vega-3 SEM instrument fitted with a Thermo EDS attachment. The SEM was operated in a low-vacuum mode with a 15–20 kV accelerating voltage and a 40 second accumulation time. The summarized SEM results can be found in the ESI.†
UV-visible spectroscopy
UV-visible reflectance spectra were recorded using a PerkinElmer Lambda 35 UV-Vis spectrophotometer. The spectrophotometer was operated in the diffuse reflectance mode and was equipped with an integrating sphere. Reflectance data were converted internally to absorbance via the Kubelka–Munk function. Spectra were recorded over the 200 nm (6.2 eV)–900 nm (1.3 eV) range at room temperature and ambient condition on powder samples.28
Thermogravimetric analysis (TGA) and differential scanning calorimetry (DSC)
TGA/DSC data were collected on polycrystalline powder samples by utilizing a Texas Instruments SDT Q600 Simultaneous TGA/DSC. An alumina pan served as the sample holder. The samples were heated from room temperature to 500 °C at a rate of 5 °C min−1 under nitrogen gas with a controlled flow rate of 100 mL min−1. Subsequently, the resulting powders were further analyzed by PXRD for phase identification.
In situ powder X-ray diffraction (PXRD)
In situ PXRD measurements were conducted using a Rigaku SmartLab equipped with a 9 kW rotating Mo Kα anode utilizing cross-beam optics (convergent radiation), an Anton Paar HTK1200N high-temperature oven chamber, and a capillary rotation attachment. A mixture of Na2Se, Si, B, and Se (in the molar ratio of 1
:
6
:
12
:
17), the same as used to prepare phase-pure powders of Na2B12Si6Se18 as described above, was loaded into quartz capillary (OD = 1 mm, ID = 0.9 mm) that was previously sealed at one end using a MAPP/oxygen mixture torch. Once partially filled with the reaction mixture, the capillary was sealed using clay. The capillary was placed into the capillary rotation attachment which was then loaded into the HTK1200N. Once the capillary was set to rotate and the X-ray beam was aligned with the precise height of capillary, the capillary was heated to 800 °C at 5 °C min−1 with 30 min PXRD measurements from 4.5–35° 2θ taken at 50 °C increments until 600 °C and then at 20 °C increments up to 800 °C. Then, the capillary was held at 800 °C and 10 sequential PXRD measurements were taken, again 30 min in length from 4.5–35° 2θ. Finally, the capillary was cooled to 50 °C at 5 °C min−1 with 30 min PXRD measurements from 4.5–35° 2θ taken at 50 °C increments.
Results and discussion
Structure of the framework
The X-ray structure determination revealed that the compound consists of a complex three-dimensional framework with tunnels containing Na-ions (Fig. 2a and b). This framework is constructed from interconnected SiSe4 tetrahedra and B12 icosahedra, with an overall composition of Na2B12Si6Se18. Chalcogenides containing B12 icosahedra, aside from halogenides or hydroxides, are relatively rare.22,29,30 The observed B12 icosahedron is connected to twelve Se atoms, a structural motif that has been identified in only a few other recently reported compounds, such as CrSi3(B12)Se12, A8[B12(BSe3)6], and Sn4−δB12Se12[Qx] (where Q = Se, Te).9,21,31 The structure of Na2B12Si6Se18 shows similarities to other quaternary compounds, such as Sn4−δB12Se12[Qx] and CrSi3(B12)Se12, both of which also exhibit three-dimensional frameworks that incorporate B12 icosahedral clusters. In the Sn4−δB12Se12[Qx] composition, the 3D framework is built from (B12Se12)14− anionic closo-borane clusters that are connected along the c-axis by octahedral Sn2+ centers. These octahedral units form infinite parallel [SnB12Se12]12− columns that accommodate chains of neutral chalcogen atoms within the tunnels. Similarly, the CrSi3(B12)Se12 compound forms a 3D structure comprising SiSe4 tetrahedra, CrSe6 octahedra, and B12 icosahedra. In this structure, the tunnels formed by the 3D framework are filled with disordered Se atoms. The structural similarities between Na2B12Si6Se18 and these quaternary chalcogenides highlight a common motif of B12 icosahedral clusters linked by various metal centers, forming three-dimensional networks that can accommodate guest ions or atoms within the tunnels. This feature could potentially influence the physical properties, such as ion conductivity or electronic behavior, making these compounds of particular interest for further study.
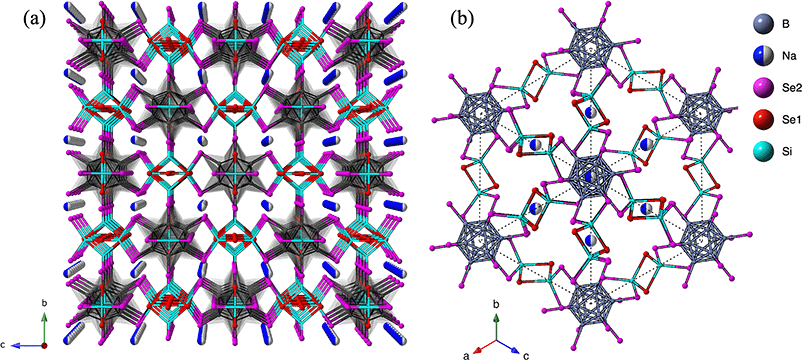 |
| Fig. 2 The unit cell representation of Na2B12Si6Se18 (a) along a-axis, and (b) along [111] direction. | |
In the quaternary composition Na2B12Si6Se18, the three-dimensional (3D) anionic framework, denoted as [B12Si6Se18]2−, is formed by the connection of [B12Se12]14− icosahedra with Si2Se6 tetrahedral units. The [B12Se12]14− cluster is a central feature of this framework, where the Se1 atoms act as bridging elements between the B12 icosahedral cluster and the SiSe4 tetrahedra. The structure is further stabilized as the B12Se12 units share six of their edges with adjacent SiSe4 tetrahedra, creating a robust and interconnected network. Within the SiSe4 tetrahedra, two distinct types of selenium atoms, Se1 and Se2, play crucial roles in the framework's architecture. Se1 serves as a bridge, connecting the SiSe4 tetrahedra with the B12 cluster via edge-sharing (Fig. 3a). Meanwhile, Se2 is responsible for connecting two SiSe4 tetrahedral units (Fig. 3b), also through edge-sharing. This arrangement of Se atoms is essential for the formation of the 3D structure, as it facilitates the strong bonding between the different clusters and tetrahedra resulting in the creation of tunnels within the framework. The sodium ions (Na+) occupy these tunnels, residing within the spaces formed by the anionic framework. The charge balance of the compound is maintained by the oxidation states of the constituent elements: the B12Se12 cluster carries a charge of 14−, silicon (Si) is in the +4-oxidation state, selenium (Se) in the 2− state, and sodium (Na) in the +1 state. This charge balance ensures the overall stability of the compound, with the Na+ ions playing a crucial role in charge compensation within the 3D network.
 |
| Fig. 3 The (a) polyhedral view of [B12Se6]2− icosahedron cluster extended along [111] direction, (b) bridging of SiSe4 tetrahedra between two [B12Se6]2−cluster, and (c and d) a representation of NaSe6 octahedra and their connectivity with its neighboring NaSe6 units. | |
The sodium (Na) atoms in Na2B12Si6Se18 are coordinated with six neighboring selenium (Se) atoms, forming a NaSe6 octahedral geometry (Fig. 3c). When these NaSe6 polyhedra are extended throughout the three-dimensional structure, it becomes evident that the NaSe6 units are interconnected via vertex-sharing (Fig. 3d). The Na–Se bond distance observed within the NaSe6 octahedra is approximately 3.1813(5) Å, which is consistent with bond lengths reported for other compounds, such as Na4Si2Se6−oP48, NaScSe2 and NaCuTSe3.32–34 The Si–Se bond distances vary slightly, ranging from 2.2777(9) Å to 2.2808(8) Å. These values are in close agreement with those observed in other selenium-containing silicate compounds, including Ba6Si2−xGexSe12, Li2HgMSe4, Na2Hg3M2Se8, and Ba3SiSe5.35–37 This consistency in Si–Se bond lengths across various compounds highlights the structural similarity and predictability of silicon–selenium bonding within tetrahedral frameworks. The B–Se bond length is approximately 1.980(3) Å, while the B–B distances range between 1.779(7) Å and 1.781(4) Å. These bond lengths are comparable to those found in related compounds, such as CrSi3(B12)Se12, A8[B12(BSe3)6], and Sn4−δB12Se12[Qx] (where Q = Se, Te), which also contain B12 icosahedral clusters.9,21,31
UV-visible diffuse reflectance spectroscopy
The optical properties of the quaternary Na2B12Si6Se18 polycrystalline compound was examined at room temperature through optical absorption studies. The absorption plot with respect to wavelength (nm) is shown in Fig. S7.† The Kubelka–Monk equation was employed to analyze the optical absorption data, as shown in Fig. 4. The band gap observed for the compound is ∼2.1(1) eV, consistent with semiconducting behavior.
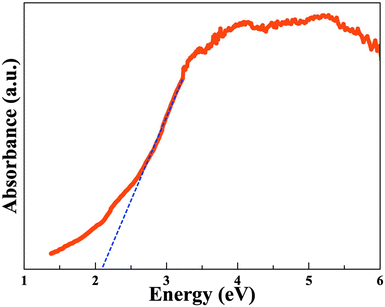 |
| Fig. 4 The optical absorption plot of the polycrystalline Na2B12Si6Se18 compound. | |
In situ powder X-ray diffraction (PXRD)
To better understand the formation of the Na2B12Si6Se18 phase and determine whether it forms during heating or cooling, we carried out a series of controlled thermal reactions. Initially, we heated a reaction mixture containing Na2Se, Si, B, and Se (in a molar ratio of 1
:
6
:
12
:
17) to a temperature of 800 °C over a period of 4 hours. The sample was then held at this temperature for 36 hours, followed by quenching the red-hot reaction tube in ice-chilled water. To further investigate the effect of dwelling time and temperature on the phase formation, we repeated the thermal quenching experiment but reduced the dwelling time from 36 hours to 12 hours and lowered the reaction temperature from 800 °C to 600 °C. After these modified conditions, the samples were ground, and their powder X-ray diffraction (PXRD) patterns were collected for phase analysis. Our analysis revealed that the Na2B12Si6Se18 phase forms when the sample is heated to 800 °C, regardless of whether the dwell time is shorter or longer. However, no formation of the Na2B12Si6Se18 phase was observed when the sample was heated to temperatures below 800 °C (see Fig. S5†).
To gain a more detailed understanding of the phase formation process at different temperatures, we performed an in situ PXRD synthesis by loading the reaction mixture into a quartz capillary and heating the sample to 800 °C inside the hot stage of the X-ray diffractometer. This approach allowed us to monitor the phase evolution in real-time, providing insights into the specific conditions under which the Na2B12Si6Se18 phase forms Fig. 5.
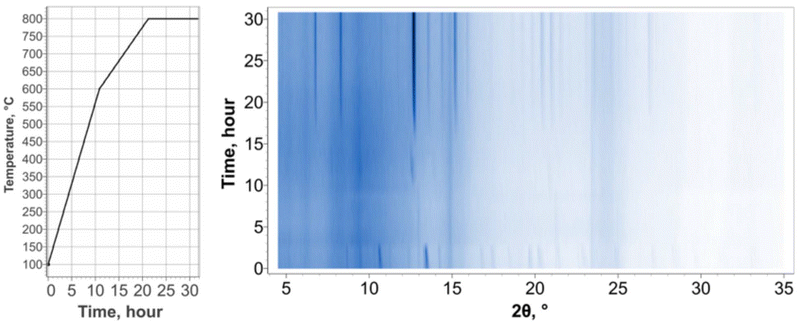 |
| Fig. 5 The waterfall plot of the in situ PXRD patterns collected upon heating and annealing the reaction mixture. The waterfall plot is shown on the right as time vs. 2θ with the graph of temperature vs. time on the left. | |
The pattern for the initial reaction mixture contains peaks for Se8, Si, and Na2Se (the B used was amorphous), as expected. The first significant change in the patterns occurred between 200 and 250 °C: the peaks for Se and Na2Se vanish leaving only the peaks for Si. This is most likely due to the Se8 melting (M.P. = 221 °C) and dissolving the Na2Se concurrently. The next major change occurs between 600 and 650 °C: the Si peaks disappear, and new peaks appear which do not correspond to the starting reagents or the final product. It is unclear what phase(s) these peaks belong to as the patterns could not be successfully matched to any known phases. Due to the simultaneous disappearance of the Si peaks, it is likely that this change is due to the reaction between the Si and the molten Se8/Na2Se mixture to form one or more selenosilicates. This may be enabled by either the solvation of the Si or the direct reaction requiring elevated temperatures. Finally, the last significant change in the pattern occurs between 700 and 750 °C: the peaks corresponding to the unknown phase(s) formed between 600 and 650 °C vanish and the peaks for the target phase, Na2Si6B12Se18, appear simultaneously. Due to the unknown identity of the peaks directly preceding those for Na2Si6B12Se18, it is unclear what phases are reacting to form the final product. However, it is clear that there is a minimum temperature (∼750 °C) above which this reaction must be heated in order for the desired product to form.
Upon cooling, Fig. 6, only one significant change is observed: peaks for Na4Si4Se10 appear at approximately 550 °C. This is a previously reported phase38 consisting of Si4Se10 super-tetrahedra separated by Na cations. The formation of this phase suggests that the B in the reaction mixture is completely consumed in the formation of the target phase, Na2Si6B12Se18, while the Na, Si, and Se are not.
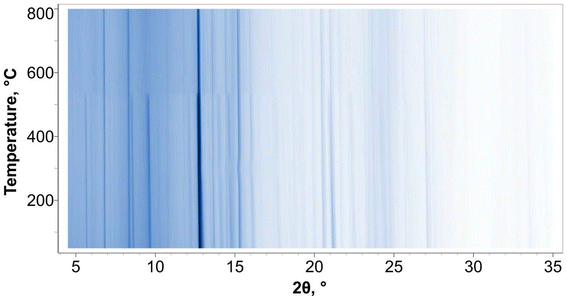 |
| Fig. 6 The waterfall plot of the in situ PXRD patterns collected upon cooling the reaction mixture. The waterfall plot is shown as temperature vs. 2θ. | |
TGA studies
The thermal stability of Na2B12Si6Se18 polycrystalline powder samples was assessed using thermogravimetric analysis (TGA) (Fig. S6†). The TGA measurements were conducted under nitrogen flow, with the temperature increased at a rate of 5 °C min−1 to 500 °C. The results showed no significant weight loss, indicating the material's thermal stability under these conditions. To further confirm this, post-TGA powder X-ray diffraction (PXRD) analyses were performed on the samples. The PXRD patterns of the samples collected after heating revealed no structural degradation, confirming that the Na2B12Si6Se18 phases remained stable up to at least 500 °C, with no detectable phase transitions or decomposition occurring within this temperature range. As the material is synthesized above 800 °C, this is not unexpected.
Conclusion
The successful synthesis of the quaternary 3D chalcogenide compound Na2Si6B12Se18via solid-state methods has revealed a distinctive framework featuring the rare icosahedral [B12]10+ cation. The assembly of this cation into a 3D tunnel structure filled with Na+ ions, and its crystallization in the cubic Im
space group, highlight its complex and novel architecture. The structural connectivity between B12 icosahedra and SiSe4 tetrahedra underscores the intricacy of the framework, while the compound's semiconducting properties, with a bandgap of 2.1(1) eV, point to potential applications in electronic materials. The in situ PXRD studies further emphasize the phase formation process at different temperatures. The thermal stability of the material was confirmed by TGA/DSC measurements.
Data availability
The CCDC 2362801† entries encompass the supplementary crystallographic data associated with this paper. Raw data for other measurements are available upon request.
Conflicts of interest
The authors declare no competing financial interest.
Acknowledgements
The authors gratefully acknowledge the support from the U.S. Department of Energy, Office of Basic Energy Sciences, Division of Materials Sciences and Engineering, under award DE-SC0018739. Synthesis, structural characterization, optical, TGA, and in situ PXRD studies were conducted at the University of South Carolina.
References
- R. B. King, Chem. Rev., 2001, 101, 1119–1152 CrossRef CAS PubMed.
- T. J. Udovic, M. Matsuo, A. Unemoto, N. Verdal, V. Stavila, A. V. Skripov, J. J. Rush, H. Takamura and S. Orimo, Chem. Commun., 2014, 50, 3750–3752 RSC.
- M. P. Pitt, M. Paskevicius, D. H. Brown, D. A. Sheppard and C. E. Buckley, J. Am. Chem. Soc., 2013, 135, 6930–6941 CrossRef CAS PubMed.
- B. Krebs and H.-U. Hürter, Angew. Chem., Int. Ed., 1980, 19, 481–482 CrossRef.
- B. Krebs, Angew. Chem., Int. Ed., 1983, 22, 113–134 CrossRef.
- B. Krebs and W. Hamann, J. Common Met., 1988, 137, 143–154 CrossRef CAS.
- C. Jansen, J. Küper and B. Krebs, Z. Anorg. Allg. Chem., 1995, 621, 1322–1329 CrossRef CAS.
- A. Lindemann, J. Kuchinke and B. Krebs, Z. Anorg. Allg. Chem., 1999, 625, 1165–1171 CrossRef CAS.
- A. Hammerschmidt, M. Döch, S. Pütz and B. Krebs, Z. Anorg. Allg. Chem., 2004, 630, 2299–2303 CrossRef CAS.
- A. Hammerschmidt, A. Lindemann, M. Döch and B. Krebs, Z. Anorg. Allg. Chem., 2003, 629, 1249–1255 CrossRef CAS.
- J. Kuchinke, A. Hammerschmidt and B. Krebs, Solid State Sci., 2003, 5, 189–196 CrossRef CAS.
- A. Hammerschmidt, A. Lindemann, M. Döch, C. Köster and B. Krebs, Z. Anorg. Allg. Chem., 2002, 628, 1561–1567 CrossRef CAS.
- A. Lindemann, J. Küper, W. Hamann, J. Kuchinke, C. Köster and B. Krebs, J. Solid State Chem., 2001, 157, 206–212 CrossRef CAS.
- C. Püttmann, H. Diercks and B. Krebs, Phosphorus, Sulfur Silicon Relat. Elem., 1992, 65, 1–4 Search PubMed.
- R. Bertermann, W. Müller-Warmuth, C. Jansen, F. Hiltmann and B. Krebs, Solid State Ionics, 1999, 117, 245–255 CrossRef CAS.
- L. S. Breton, G. Morrison, M. R. Lacroix, P. S. Halasyamani and H.-C. zur Loye, Chem. Commun., 2022, 58, 7992–7995 RSC.
- Y.-Y. Li, B.-X. Li, G. Zhang, L.-J. Zhou, H. Lin, J.-N. Shen, C.-Y. Zhang, L. Chen and L.-M. Wu, Inorg. Chem., 2015, 54, 4761–4767 CrossRef CAS PubMed.
- S.-S. Han, W.-D. Yao, S.-X. Yu, Y. Sun, A. Gong and S.-P. Guo, Inorg. Chem., 2021, 60, 3375–3383 CrossRef CAS PubMed.
- Y. Huang, Y. Zhang, D. Chu, Z. Yang, G. Li and S. Pan, Chem. Mater., 2023, 35, 4556–4563 CrossRef CAS.
- K. Kaup, K. Bishop, A. Assoud, J. Liu and L. F. Nazar, J. Am. Chem. Soc., 2021, 143, 6952–6961 CrossRef CAS PubMed.
- M. Sugimori, H. Fukuoka, H. Imoto and T. Saito, J. Organomet. Chem., 2000, 611, 547–550 CrossRef CAS.
- S.-P. Guo, G.-C. Guo, M.-S. Wang, J.-P. Zou, H.-Y. Zeng, L.-Z. Cai and J.-S. Huang, Chem. Commun., 2009, 4366–4368 RSC.
- S.-H. Lin, J.-G. Mao, G.-C. Guo and J.-S. Huang, J. Alloys Compd., 1997, 252, L8–L11 CrossRef CAS.
-
APEX3 Version 2019.1-0 and SAINT+ Version 8.40A, Bruker Nano, Inc., Madison, WI, USA, 2019 Search PubMed.
- SADABS-2016/2: L. Krause, R. Herbst-Irmer, G. M. Sheldrick and D. Stalke, J. Appl. Crystallogr., 2015, 48, 3–10 CrossRef CAS PubMed.
-
(a) SHELXT: G. M. Sheldrick, Acta Crystallogr., Sect. A:Found. Adv., 2015, 71, 3–8 CrossRef PubMed;
(b) SHELXL: G. M. Sheldrick, Acta Crystallogr., Sect. C:Struct. Chem., 2015, 71, 3–8 Search PubMed.
- C. B. Hübschle, G. M. Sheldrick and B. Dittrich, ShelXle: A Qt Graphical User Interface for SHELXL, J. Appl. Crystallogr., 2011, 44(6), 1281–1284 CrossRef PubMed.
- P. Kubelka, Z. Tech. Phys., 1931, 12, 593–601 Search PubMed.
- S.-P. Guo, Y. Chi, B.-W. Liu and G.-C. Guo, Dalton Trans., 2016, 45, 10459–10465 RSC.
- S.-S. Han, Q.-T. Xu, W. Liu and S.-P. Guo, Dalton Trans., 2022, 51, 4619–4622 RSC.
- D. G. Chica, I. Spanopoulos, S. Hao, C. Wolverton and M. G. Kanatzidis, Chem. Mater., 2021, 33, 1723–1730 CrossRef CAS.
- F. Kamm, F. Pielnhofer, M. Schlosser and A. Pfitzner, Inorg. Chem., 2023, 62, 11064–11072 CrossRef CAS PubMed.
- G. Panigrahi, S. Yadav, S. Jana, A. Ghosh, M. K. Niranjan and J. Prakash, New J. Chem., 2022, 46, 22076–22087 RSC.
- A. A. Berseneva, V. V. Klepov, K. Pal, K. Seeley, D. Koury, J. Schaeperkoetter, J. T. Wright, S. T. Misture, M. G. Kanatzidis, C. Wolverton, A. V. Gelis and H.-C. zur Loye, J. Am. Chem. Soc., 2022, 144, 13773–13786 CrossRef CAS PubMed.
- L. T. Menezes, A. Assoud and H. Kleinke, Dalton Trans., 2023, 52, 15831–15838 RSC.
- L. Gao, J. Xu, X. Tian, B. Zhang, X. Wu and K. Wu, Chem. Mater., 2022, 34, 5991–5998 CrossRef CAS.
- H. Cheng, A. Tudi, P. Wang, K. Zhang, Z. Yang and S. Pan, Dalton Trans., 2021, 50, 11999–12005 RSC.
- B. Eisenmann, J. Hansa and H. Schafer, Z. Anorg. Allg. Chem., 1985, 40, 450–457 Search PubMed.
Footnote |
† Electronic supplementary information (ESI) available: EDS tabulated data, PXRD patterns, and TGA plot. CCDC 2362801. For ESI and crystallographic data in CIF or other electronic format see DOI: https://doi.org/10.1039/d4dt03139c |
|
This journal is © The Royal Society of Chemistry 2025 |
Click here to see how this site uses Cookies. View our privacy policy here.