DOI:
10.1039/D4JA00345D
(Technical Note)
J. Anal. At. Spectrom., 2025,
40, 104-109
A new calcite reference material for in situ oxygen isotope analysis using secondary ion mass spectrometry: development and application constraints†
Received
20th September 2024
, Accepted 2nd December 2024
First published on 3rd December 2024
Abstract
The oxygen isotopic microanalysis of calcite is essential for obtaining high spatial resolution data linked to microstructures, a challenge for conventional techniques. This analysis, however, relies heavily on matrix-matched reference materials, of which only a few calcite standards are available. In this study, an inorganically precipitated calcite vein sample (WS-1) was evaluated through 225 SIMS oxygen isotope analyses and was found to have a homogeneous isotopic composition, with an external reproducibility ≤0.21‰ (1σ), suggesting its potential as a SIMS reference material. The precise δ18OVPDB value, determined via traditional gas-source IRMS, was −16.52 ± 0.13‰ (1SD). Matrix effects were assessed using various carbonates, including abiotic aragonite (VS001/1-A), three abiotic calcites (NBS18, Cal-1, WS-1), and a high-Mg calcite (gorgonian coral). The results revealed negligible matrix effects between abiotic aragonite and calcites but significant differences between calcites and high-Mg calcite, likely due to Mg content or differences in biogenic crystal morphology and trace organic composition. This study demonstrates the utility of in situ oxygen isotopic microanalysis for calcite but emphasizes the need for caution when analyzing high-Mg calcitic skeletons.
1 Introduction
Calcite (CaCO3), a widely distributed carbonate mineral, occurs in various geological settings, including igneous, metamorphic, sedimentary, and hydrothermal rocks, as well as in biological skeletons.1–3 It plays a pivotal role in geochemical studies. The oxygen isotopic composition of early calcite cement can be used to trace the origin of parent fluids, delineate complex diagenetic phases, and indicate sedimentary discontinuity events.4
Furthermore, detailed variations in oxygen isotopes within zoned calcite minerals provide insights into burial temperatures, fluid infiltration processes, and the evolutionary history of diagenetic rocks.5–7 The oxygen isotopic composition of calcitic biological skeletons, such as speleothems, corals, foraminifera, and bivalves, also serves as a proxy for reconstructing paleoclimatology and the chemical composition of seawater or groundwater.3,8–12 Particularly, isotopic variations observed at microscales using ultra-high resolution analytical techniques provide valuable archives for extreme weather events and paleoclimate changes.13–15
Conventional bulk analysis of carbonate oxygen isotopes typically involves micro-drilling sample powders, reacting them with phosphoric acid to release CO2, followed by cryo-drying, transfer, and subsequent analysis.16–18 Although this method is highly precise and accurate, it is time-consuming, labor-intensive, and lacks the spatial resolution needed to interpret fine-scale geological processes, such as high-resolution weather reconstructions,13,14 and correlations with zoning textures. Therefore, the development of in situ oxygen isotopic analysis for calcite is crucial.
In situ oxygen isotopic analysis methods, such as secondary ion mass spectrometry (SIMS) and NanoSIMS, bypass the need for complex sample preparation while offering ultra-high spatial resolution.19–26 However, a significant challenge in advancing these methods is the limited availability of suitable reference materials to correct for matrix effects.27–30 Synthetic calcite reference materials with isotopic homogeneity must be produced through intricate procedures and possess specific physical properties, such as mechanical strength, density, and hardness, which can differ from those of natural calcite samples.31 Natural calcite reference materials offer better matrix matching, but they are currently scarce. Only a few widely distributed natural calcite standards are available, such as UWC-1 and UWC-3 (ref. 5 and 32) along with more recent additions like GBW04481 and NJUCal-1.33,34 The limited availability of these standards impedes broader application and emphasizes the need for developing new reference materials. Moreover, matrix effects have also been documented in SIMS oxygen isotopic analysis of carbonates, even among the same crystal types, due to compositional variations, such as Fe–Mg in dolomites,30,35,36 and Ca content in aragonites.37 However, the extent of matrix effects in calcite, particularly in high-Mg and low-Mg calcites—common natural variants—remains underexplored. This knowledge gap is critical for evaluating the applicability of existing standards.
Given the scarcity of calcite standards and the need for a systematic evaluation of SIMS oxygen isotopic analysis, this study focused on developing a new natural inorganic calcite reference material with homogeneous oxygen isotopic composition at the micrometer scale. Additionally, matrix effects were characterized using carbonate materials, including inorganic aragonite (VS001/1-A),37 inorganic calcites (NBS18, Cal-1 and WS-1), and high-Mg calcite (deep-sea gorgonian coral).
2 Materials and methods
2.1 Sample preparation
The analyzed samples included three inorganic calcite specimens: WS-1, previously characterized for its sampling setting, morphology, and crystal features,38 Cal-1 (δ18OVPBD = −14.03 ± 0.16‰),37 and the US Geological Survey standard, NBS18 (δ18OVPBD = −23.06 ± 0.26‰).39 The latter two calcites exhibit relatively inhomogeneous in situ oxygen isotopic compositions.37 Additionally, a deep-sea gorgonian coral composed of high-Mg calcite (8 mol% Mg, exceeding the 4 mol% boundary between low-Mg and high-Mg calcites40) was included in the study. This coral exhibits a relative homogeneous oxygen isotopic composition across its transverse skeleton (excluding the small axial region), with an average δ18OVPBD value of +2.3‰, and a reproducibility of 0.46‰ based on 153 SIMS measurements.41 An in-house developed aragonite standard, VS001/1-A (δ18OVPBD = −12.41 ± 0.03‰), served as a reference material for in situ oxygen isotopic analysis.37 All specimens were cleaned ultrasonically using deionized water, followed by drying at 50 °C. They were then mounted in epoxy resin and polished with diamond paste. Additionally, a portion of the cleaned and dried WS-1 fragments was ground into powder using a mortar and pestle for subsequent solution-based analyses.
2.2 Oxygen isotope analysis by SIMS
In situ oxygen isotopic analyses were conducted using a CAMECA IMS1280-HR ion microprobe at the Guangzhou Institute of Geochemistry, Chinese Academy of Sciences. The sample chamber vacuum was optimized by storing of the sample mount overnight in a high-vacuum chamber, followed by additional pumping down to approximately 1.9 × 10−9 mbar for 1 to 2 hours in the analysis chamber. A primary Cs+ ion beam with a current of 1–2.5 nA, a diameter of ∼15 μm and an impact energy of 20 keV was used to sputter secondary ions from the sample surface. Charge compensation during the measurement sessions was achieved using a normal-incidence electron-flood gun. Each analysis included a 30 seconds pre-sputtering phase to remove the gold coating. A field aperture of 5000 × 5000 μm and a contrast aperture of 400 μm were employed. The 18O and 16O ions were collected simultaneously in two off-axis Faraday cups (H′2 and L′2) with a mass resolution (full width at half maximum) of 2400. Typical secondary ion intensities for 16O ranged from 1.6 × 109 to 2.6 × 109 counts per second.
To assess oxygen isotopic homogeneity, random sampling was performed on 26 different fragments of WS-1 calcite, leading to 225 spot analyses across five measurement sessions. The natural aragonite standard, VS001/1-A, served as a reference for monitoring instrumental conditions and was used to correct the fractionation resulting from variations in instrumental conditions across different sessions. It was analyzed after every 6–8 analyses of WS-1, NBS18, Cal-1, and the deep-sea gorgonian coral.
Oxygen isotopic compositions are reported in per mil notation relative to Vienna Pee Dee Belemnite (VPDB; 18O/16OVPBD = 0.0020672). Instrumental mass fractionation28 corrections were calculated as follows:
| δ18OSIMS = ((18O/16OSIMS)/(18O/16OVPDB) − 1) × 1000 | (1) |
| IMF = {(1 + δ18OSIMS/1000)/(1 + δ18OIRMS/1000) − 1} × 1000 | (2) |
| δ18Ocorrected = {(1 + δ18OSIMS /1000)/(1 + IMF/1000) − 1} × 1000 | (3) |
where
18O/
16O
SIMS and
δ18O
SIMS represent the raw ratio and isotopic value measured by SIMS, while
δ18O
IRMS and
δ18O
corrected denote values derived from conventional technique and the final corrected values, respectively.
2.3 Bulk oxygen isotope analysis by isotope ratio mass spectrometry (IRMS)
Bulk oxygen isotope compositions of WS-1 were determined using dual-inlet isotope-ratio mass spectrometry (IRMS; 253 Plus, ThermoFisher Scientific™, USA) equipped with a conventional phosphoric acid digestion system. The analytical procedures followed those described by Guo et al. (2019).42 For each measurement session, a powdered carbonate sample (1 mg) was digested with 102% H3PO4 at 90 °C to extract CO2. Bulk isotopic compositions were normalized to an IAEA standard (NBS18) and reported on the VPDB scale.
3 Results and discussion
3.1 Homogeneity of potential oxygen isotope reference material
The homogeneity of oxygen isotopes in WS-1 calcite was assessed through 225 measurements conducted across five sessions using SIMS. To ensure robustness, more than 26 randomly selected fragments were analyzed. Fig. 1a presents the oxygen isotope values measured by SIMS corrected for IMF, yielding 1σ values of 0.13‰, 0.21‰, 0.21‰, 0.18‰ and 0.16% for each session, respectively. The distribution of these values, shown in Fig. 1b, conforms to a normal distribution, affirming the uniformity of oxygen isotopes in WS-1 calcite.
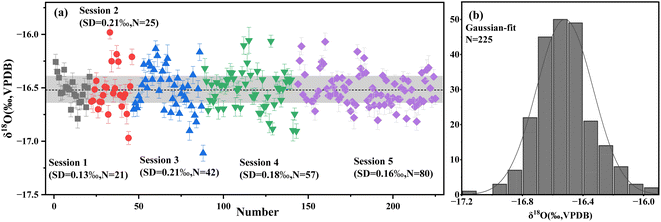 |
| Fig. 1 SIMS results for oxygen isotopes in WS-1 calcite (a), with corresponding frequency histogram and probability density curve (b). The dark dashed line represents the oxygen isotope value determined by IRMS. The histograms display the frequency distribution of the oxygen isotope values, while the overlaid curves show the probability density, indicating a Gaussian distribution of the data sets. | |
The standard deviation (1σ) values from the SIMS results, representing spot-to-spot reproducibility, ranged from 0.13‰ to 0.21‰ across the sessions. These values are consistent with those reported for other well-established calcite reference materials,5,33,34,37 demonstrating the acceptable external precision achieved in this research. This level of reproducibility confirms that WS-1 calcite is a promising candidate for use as a reference material in SIMS isotopic microanalysis.
Further evaluation of the isotopic homogeneity was conducted using the H-index, a key indicator for microscale analysis.43,44 The H-index represents the ratio of measurement precision to the total combined uncertainty, which accounts for both instrumental uncertainties and sample heterogeneity.43,44 In this study, the H-index values obtained for WS-1 calcite ranged from 1 to 3 (Fig. 2), indicating satisfactory isotopic homogeneity. This conclusion is further supported by the normal distribution observed in the δ18O values (Fig. 1b), confirming the reliability of WS-1 calcite as a suitable reference material.
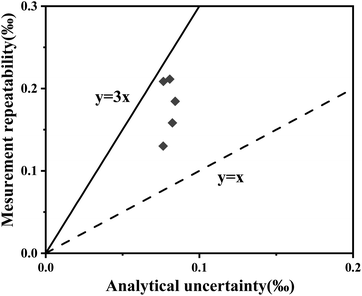 |
| Fig. 2 Assessment of the homogeneity of WS-1 calcite. The homogeneity index values of 1 (dashed line) and 3 (solid line) are indicated. | |
3.2 Oxygen isotope composition of calcite reference material WS-1
The oxygen isotopic composition of WS-1 calcite, analyzed using both traditional mass spectrometry and in situ microanalysis, is summarized in Table 1, with detailed data provided in ESI Table S1.† WS-1 fragments were analyzed using the IRMS technique over three sessions, yielding an average oxygen isotope composition of −16.44 ± 0.25‰ (1SD) with detailed data provided in ESI Table S2.† The mean oxygen isotopic compositions of WS-1, as determined by SIMS and corrected using the VS001/1-A standard, are as follows: −16.61 ± 0.13‰ (1SD, N = 21), −16.36 ± 0.21‰ (1SD, N = 25), −16.20 ± 0.21‰ (1SD, N = 42), and −16.30 ± 0.18‰ (1SD, N = 57), −16.62 ± 0.16‰ (1SD, N = 80) for the five measurement sessions. These SIMS results are consistent with the IRMS value of −16.52 ± 0.13‰ (1SD), falling within the expected analytical uncertainties. Based on these findings, the IRMS value of −16.52 ± 0.13‰ (1SD) is recommended as the reference oxygen isotopic value for WS-1 calcite.
Table 1 Mean δ18O values of carbonates determined by SIMS and IRMS
Session |
Sample |
Mean SIMS δ18O value (‰) |
Number |
Mean SIMS δ18O value corrected by VS001/1-A (‰) |
Mean IRMS δ18O value (‰) |
IMF (‰) |
SD (‰) |
1 |
NBS18 |
−27.69 |
11 |
−23.11 |
−23.06 (±0.26)39 |
−0.05 |
0.36 |
VS001/1-A |
−16.99 |
13 |
−12.41 |
−12.41 (±0.03)37 |
0.00 |
0.28 |
WS-1 |
−21.21 |
21 |
−16.61 |
−16.52 (±0.13) |
−0.09 |
0.13 |
2 |
NBS18 |
−24.62 |
4 |
−23.40 |
|
−0.34 |
0.30 |
VS001/1-A |
−13.64 |
4 |
−12.41 |
|
0.00 |
0.29 |
WS-1 |
−17.59 |
25 |
−16.36 |
|
0.16 |
0.21 |
3 |
NBS18 |
−24.92 |
5 |
−23.00 |
|
0.06 |
0.17 |
VS001/1-A |
−14.34 |
12 |
−12.41 |
|
0.00 |
0.29 |
WS-1 |
−18.12 |
42 |
−16.20 |
|
0.32 |
0.21 |
4 |
NBS18 |
−24.89 |
7 |
−23.37 |
|
−0.31 |
0.18 |
VS001/1-A |
−13.93 |
13 |
−12.41 |
|
0.00 |
0.22 |
WS-1 |
−17.81 |
57 |
−16.30 |
|
0.22 |
0.18 |
Cal-1 |
−14.05 |
11 |
−14.05 |
−14.03 (±0.16)45 |
−0.02 |
0.36 |
High-Mg calcite coral |
−3.23 |
29 |
−1.72 |
2.30 (±0.05)41 |
−4.02 |
0.28 |
5 |
VS001/1-A |
−10.85 |
14 |
−12.41 |
|
0.00 |
0.26 |
WS-1 |
−15.07 |
80 |
−16.62 |
|
−0.01 |
0.16 |
3.3 Potential matrix effects
Matrix effects are a critical consideration in in situ analyses, as they can influence the accuracy of SIMS measurements. Previous studies have demonstrated the impact of matrix composition on SIMS oxygen isotopic analysis. For example, Śliwiński et al. (2016) and Xu et al. (2022) showed that variations in the Fe/Mg or Fe/(Fe + Mg) ratios in dolomite can significantly affect the IMF.30,35 Rollion-Bard and Marin-Carbonne (2011) found that MgO content alters SIMS IMF values across a range of carbonates, including calcite, dolomite, ankerite, magnesite, siderite, and rhodochrosite.36 He et al. (2021) also noted that variations in Ca composition can lead to matrix effects in SIMS oxygen isotopic analysis of aragonite.37 Husson et al. (2020) and Śliwiński et al. (2018) utilized the carbonate ternary diagram [Ca–Mg-(Fe + Mn)] to illustrate the non-linear relationship between element composition and IMF.46,47 Despite these insights, matrix effects between different carbonates—specifically aragonite versus calcite, and low-Mg versus high-Mg calcites—have not been thoroughly explored. This gap is particularly relevant given the widespread occurrence of these carbonates in marine skeletons and stalagmites, which are key to paleoenvironmental reconstructions.
To address this issue, we evaluated the IMF values for aragonite and several calcites, including three low-Mg calcites (WS-1, NBS18, Cal-1) and a high-Mg calcite (gorgonian coral). As shown in Fig. 3, the IMF values for aragonite and low-Mg calcites were consistently negligible within analytical uncertainty. This contrasts with Linzmeier et al. (2016), who reported a SIMS instrumental bias of approximately 0.6‰ between UWArg-7 aragonite and UWC-3 calcite.25 This discrepancy may stem from differences in chemical composition, particularly Ca content, between UWArg-7 (whose elemental composition has not yet been reported) and our aragonite standard (VS001/1-A). Variations in Ca content can lead to significant matrix effects in SIMS oxygen isotopic analysis of aragonites.48 The similar crystalline structures,49 mineral densities,50 and chemical compositions – such as the Mg–Ca content-of VS001/1-A and low-Mg calcites (see Table S3†) likely contribute to their negligible IMF differences. Thus, the newly developed WS-1 reference material could serve as an effective standard for both inorganic aragonite (with a composition similar to VS001/1-A) and low-Mg calcite systems.
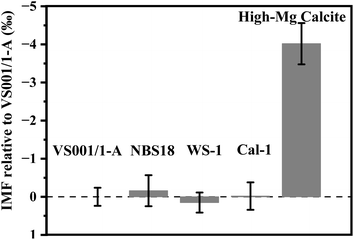 |
| Fig. 3 Instrumental mass fractionation relative to VS001/1-A for carbonate minerals. Error bars indicate the external precision of multiple analyses. | |
In contrast, the high-Mg calcite (MgO content of 2.97 wt%, see Table S3†) exhibited a significant IMF difference compared to low-Mg calcite (MgO content <0.65 wt%, see Table S3†), with an observed offset of approximately −4‰. Although both are classified as calcite, the differing Mg content substantially influences the IMF. Previous studies suggest that carbonate MgO content can vary IMF by about −0.3‰ per wt% MgO,36 which does not fully explain the −4‰ offset observed between WS-1 and high-Mg calcite. This discrepancy may be attributed to factors beyond Mg content, such as variations in other elements (e.g., Ca), crystal unit size, arrangement, or the presence of minor organic matter and water.48,51–53 The correlation between IMF and Ca content in our samples deviates significantly from trends observed in various aragonite (shown in Fig. 4). Nonetheless, the influence of Ca, particularly the minimum Ca in high-Mg calcite, cannot be entirely excluded. Furthermore, unlike inorganic calcite, high-Mg calcite derived from gorgonian coral consists of smaller crystal units with nanometer size and more regular arrangement, along with organic matter and water, as confirmed by our prior research.54 The presence of organic matter may distort crystal structure in high-Mg calcite compared to the normal calcite,55 potentially enhancing ion sampling efficiency (secondary ion yield, 16O intensity/primary ion beam, Table S1†). Helser et al. (2018) and Wycech et al. (2018) observed a discrepancy between SIMS and micromilling/IRMS δ18O values of biogenic carbonates, with SIMS producing lower values attributed to organic matter and water content.56,57 These factors may contribute to the larger IMF differences observed between high-Mg calcite (from gorgonian coral) and other forms.51–53 Therefore, caution is advised when performing SIMS oxygen isotopic analyses of calcite, particularly for high-Mg biogenic calcites. When using WS-1 as the reference material, it is advisable that calcite samples contain <1 wt% MgO to minimize analytical offsets. This recommendation is based on the documented ∼0.3‰ shift in SIMS oxygen isotopic analyses caused by 1 wt% MgO in CaCO3.36 These considerations are essential for accurate correction and interpretation of SIMS-derived oxygen isotopic data in carbonate materials.
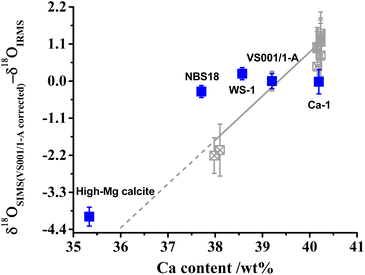 |
| Fig. 4 Relationship between Ca content and matrix bias. The matrix bias (δ18OSIMS (VS001/1-A corrected)–δ18OIRMS) indicates that all samples were corrected by VS001/1-A. Error bars represent external precision of multiple measurements (1σ). Gray data points are sourced from He et al. (2021).48 | |
4 Conclusion
The newly introduced calcite reference material, WS-1, has demonstrated excellent performance for in situ oxygen isotope analysis via SIMS, with spot-to-spot reproducibility ranging from 0.13‰ to 0.21‰ and consistent isotopic homogeneity. The average δ18O value obtained from SIMS measurements of WS-1 is −16.44 ± 0.25‰ (1SD, N = 225), closely matching the IRMS-derived value of −16.52 ± 0.13‰ (1SD). This confirms the accuracy of the recommended oxygen isotopic value for WS-1. Additionally, the assessment of matrix effects indicates that WS-1 is suitable for use with both inorganic aragonite and low-Mg calcite systems. However, it is not applicable for high-Mg calcite organic skeletons, such as those found in deep-sea gorgonian corals, where significant matrix effects were observed.
Data availability
Data for this article are available at ESI.†
Conflicts of interest
The authors declare no conflicts of interest.
Acknowledgements
We thank Dr Xiaoping Xia for providing us with the sample. This work was supported by the Strategic Priority Research Program of the Chinese Academy of Sciences (XDB0840000) and the Natural Sciences Foundation of China (42241104 and 42273012). This is contribution No. IS-3583 from GIGCAS.
References
- R. A. Exley, Earth Planet. Sci. Lett., 1983, 65, 303–310 CrossRef CAS.
-
A. Freiwald and J. Roberts, Cold-Water Corals and Ecosystems, Springer Science & Business Media, 2005 Search PubMed.
- L. F. Robinson, J. F. Adkins, N. Frank, A. C. Gagnon, N. G. Prouty, E. Brendan Roark and T. v. de Flierdt, Deep Sea Res., Part II, 2014, 99, 184–198 CrossRef CAS.
- B. Vincent, L. Emmanuel, P. Houel and J.-P. Loreau, Sediment. Geol., 2007, 197, 267–289 CrossRef CAS.
- C. M. Graham, J. W. Valley, J. M. Eiler and H. Wada, Contrib. Mineral. Petrol., 1998, 132, 371–389 CrossRef CAS.
- J. R. Bowman, J. W. Valley and N. T. Kita, Contrib. Mineral. Petrol., 2009, 157, 77–93 CrossRef CAS.
- A. C. Denny, I. J. Orland and J. W. Valley, Chem. Geol., 2020, 531, 119327 CrossRef CAS.
- M. Bar-Matthews, A. Ayalon, M. Gilmour, A. Matthews and C. J. Hawkesworth, Geochim. Cosmochim. Acta, 2003, 67, 3181–3199 CrossRef CAS.
- R. van Geldern, M. M. Joachimski, J. Day, U. Jansen, F. Alvarez, E. A. Yolkin and X. P. Ma, Palaeogeogr., Palaeoclimatol., Palaeoecol., 2006, 240, 47–67 CrossRef.
- J. B. Kimball, R. B. Dunbar and T. P. Guilderson, Chem. Geol., 2014, 381, 223–233 CrossRef CAS.
- S. Chaabane, M. López Correa, P. Montagna, N. Kallel, M. Taviani, C. Linares and P. Ziveri, Mar. Chem., 2016, 186, 11–23 CrossRef CAS.
- K. M. Edgar, H. Pälike and P. A. Wilson, Paleoceanography, 2013, 28, 468–480 CrossRef.
- H. Yan, Solid Earth Sci., 2020, 5, 249–253 Search PubMed.
- H. Yan, C. Liu, Z. An, W. Yang, Y. Yang, P. Huang, S. Qiu, P. Zhou, N. Zhao, H. Fei, X. Ma, G. Shi, J. Dodson, J. Hao, K. Yu, G. Wei, Y. Yang, Z. Jin and W. Zhou, Proc. Natl. Acad. Sci. U. S. A., 2020, 117, 7038–7043 CrossRef CAS PubMed.
- O. M. Medd, L. M. Otter, I. S. Williams, R. A. Stern, M. W. Förster, S. M. Eggins, L. Rodriguez-Sanz, N. J. Abram, M. He, M. J. Ellwood, J. A. Hargreaves, S. J. Fallon and B. M. Knowles, Geochem., Geophys., Geosyst., 2024, 25(11), e2024GC011577 CrossRef CAS.
- S. Assonov, M. Groening, A. Fajgelj, J.-F. Hélie and C. Hillaire-Marcel, Rapid Commun. Mass Spectrom., 2020, 34, e8867 CrossRef CAS PubMed.
- T. Ishimura, U. Tsunogai and F. Nakagawa, Rapid Commun. Mass Spectrom., 2008, 22, 1925–1932 CrossRef CAS PubMed.
- K. Nishida and T. Ishimura, Rapid Commun. Mass Spectrom., 2017, 31, 1875–1880 CrossRef CAS.
- X. Ling, Q. Li, L. Feng, D. Zhang, Y. Liu, G. Tang, J. Li, S. Wu, L. Huang, T. Li, Y. Liu, R. Werner and X. Li, Crystals, 2021, 11(11), 1322 CrossRef CAS.
- Y. Liu, Q. Li, G. Tang and X. Li, Crystals, 2022, 12(3), 383 CrossRef CAS.
- W. Yang, S. Hu, J. Zhang, J. Hao and Y. Lin, Sci. China: Earth Sci., 2015, 58, 1758–1767 CrossRef CAS.
- J. Zou, W. Deng, X. Chen, X. Liu, Y. Guo, G. Cai, X. Xia, Q. Yang, Y. Zhang, T. Zeng and G. Wei, Solid Earth Sci., 2021, 6, 129–141 Search PubMed.
- W. Zhang, X. Xia, Y. Zhang, T. Peng and Q. Yang, J. Anal. At. Spectrom., 2018, 33, 1559–1563 RSC.
- Q. Yang, X. Xia, W. Zhang, Y. Zhang, B. Xiong, Y. Xu, Q. Wang and G. Wei, Solid Earth Sci., 2018, 3, 81–86 Search PubMed.
- B. J. Linzmeier, R. Kozdon, S. E. Peters and J. W. Valley, PLoS One, 2016, 11, e0153890 CrossRef PubMed.
- T. E. Helser, C. R. Kastelle, J. L. McKay, I. J. Orland, R. Kozdon and J. W. Valley, Rapid Commun. Mass Spectrom., 2018, 32, 1781–1790 CrossRef CAS.
- J. M. Eiler, C. Graham and J. W. Valley, Chem. Geol., 1997, 138, 221–244 CrossRef CAS.
- M. E. Hartley, T. Thordarson, C. Taylor, J. G. Fitton and Eimf, Chem. Geol., 2012, 334, 312–323 CrossRef CAS.
- N. Shimizu and S. R. Hart, J. Appl. Phys., 1982, 53, 1303–1311 CrossRef CAS.
- J.-Y. Xu, Q.-L. Li, G.-Q. Tang, K. Lu, Y. Liu, L.-J. Feng and J. C. Melgarejo, Anal. Chem., 2022, 94, 7944–7951 CrossRef CAS PubMed.
- Y. Lin, L. Feng, J. Hao, Y. Liu, S. Hu, J. Zhang and W. Yang, J. Anal. At. Spectrom., 2014, 29, 1686 RSC.
- J. D. Blum, C. A. Gazis, A. D. Jacobson and C. P. Chamberlain, Geology, 1998, 26, 411–414 CrossRef CAS.
- R. Li, X.-L. Wang, H. Chen, H. Zheng, Y. Guan, J. Gu and G. Jin, Geostand. Geoanal. Res., 2021, 45, 747–754 CrossRef CAS.
- G.-Q. Tang, X.-H. Li, Q.-L. Li, Y. Liu and X.-X. Ling, Surf. Interface Anal., 2020, 52, 190–196 CrossRef CAS.
- M. G. Śliwiński, K. Kitajima, R. Kozdon, M. J. Spicuzza, J. H. Fournelle, A. Denny and J. W. Valley, Geostand. Geoanal. Res., 2016, 40, 157–172 CrossRef.
- C. Rollion-Bard and J. Marin-Carbonne, J. Anal. At. Spectrom., 2011, 26, 1285–1289 RSC.
- M. He, T. Chen, X. Liu, Y.-N. Yang, X. Xia, Q. Yang, P. He, J. Di, Y. Zhang and G. Wei, J. Anal. At. Spectrom., 2021, 36, 1389–1398 RSC.
- X. N. Yin, M. H. He, L. Zhang, G. H. Zhu, W. F. Deng and G. J. Wei, J. Anal. At. Spectrom., 2024, 39, 1277–1283 RSC.
- A. P. Gajurel, C. France-Lanord, P. Huyghe, C. Guilmette and D. Gurung, Chem. Geol., 2006, 233, 156–183 CrossRef CAS.
- X. Long, Y. Ma and L. Qi, J. Struct. Biol., 2014, 185, 1–14 CrossRef CAS.
- M. He, X. Yu, W. Deng, X. Chen, X. Peng, K. Ta, H. Xu, Z. Cui, Q. Yang, Y. Yang, Y. Zhang and G. Wei, J. Geophys. Res.: Biogeosci., 2024, 129(10), e2024JG008032 CrossRef.
- Y. Guo, W. Deng and G. Wei, Geochim. Cosmochim. Acta, 2019, 248, 210–230 CrossRef CAS.
- V. G. Batanova, J. M. Thompson, L. V. Danyushevsky, M. V. Portnyagin, D. Garbe-Schönberg, E. Hauri, J.-I. Kimura, Q. Chang, R. Senda, K. Goemann, C. Chauvel, S. Campillo, D. A. Ionov and A. V. Sobolev, Geostand. Geoanal. Res., 2019, 43, 453–473 CrossRef CAS.
- D. Harries, Geochemistry, 2014, 74, 375–384 CrossRef.
- X. Liu, W. Deng and G. Wei, Rapid Commun. Mass Spectrom., 2019, 33, 411–418 CrossRef CAS.
- J. M. Husson, B. J. Linzmeier, K. Kitajima, A. Ishida, A. C. Maloof, B. Schoene, S. E. Peters and J. W. Valley, Earth Planet. Sci. Lett., 2020, 538, 116211 CrossRef CAS.
- M. G. Śliwiński, K. Kitajima, M. J. Spicuzza, I. J. Orland, A. Ishida, J. H. Fournelle and J. W. Valley, Geostand. Geoanal. Res., 2018, 42, 49–76 Search PubMed.
- M. He, T. Chen, X. Liu, Y.-N. Yang, X. Xia, Q. Yang, P. He, J. Di, Y. Zhang and G. Wei, J. Anal. At. Spectrom., 2021, 36, 1389–1398 RSC.
- G. Falini, S. Albeck, S. Weiner and L. Addadi, Science, 1996, 271, 67–69 CrossRef.
- L. Fernández-Díaz, A. Fernández-González and M. Prieto, Geochim. Cosmochim. Acta, 2010, 74, 6064–6076 CrossRef.
- D. Vielzeuf, J. Garrabou, A. Baronnet, O. Grauby and C. Marschal, Am. Mineral., 2008, 93, 1799–1815 CrossRef CAS.
- S. U. Noé and W. C. Dullo, Coral Reefs, 2006, 25, 303–320 CrossRef.
- C. Rollion-Bard, J.-P. Cuif and D. Blamart, Minerals, 2017, 7, 154 CrossRef.
- M. He, X. Yu, W. Deng, X. Chen, X. Peng, K. Ta, H. Xu, Z. Cui, Q. Yang, Y. Yang, Y. Zhang and G. Wei, J. Geophys. Res.: Biogeosci., 2024, 129, e2024JG008032 Search PubMed.
- U. Brand, K. Azmy, M. A. Bitner, A. Logan, M. Zuschin, R. Came and E. Ruggiero, Chem. Geol., 2013, 359, 23–31 Search PubMed.
- T. E. Helser, C. R. Kastelle, J. L. McKay, I. J. Orland, R. Kozdon and J. W. Valley, Rapid Commun. Mass Spectrom., 2018, 32, 1781–1790 CAS.
- J. B. Wycech, D. C. Kelly, R. Kozdon, I. J. Orland, H. J. Spero and J. W. Valley, Chem. Geol., 2018, 483, 119–130 CrossRef CAS.
|
This journal is © The Royal Society of Chemistry 2025 |
Click here to see how this site uses Cookies. View our privacy policy here.