DOI:
10.1039/D4MA01242A
(Review Article)
Mater. Adv., 2025,
6, 3043-3057
Magnetic separation and degradation approaches for effective microplastic removal from aquatic and terrestrial environments
Received
14th December 2024
, Accepted 29th March 2025
First published on 1st April 2025
Abstract
The significant increase in plastic production and consumption presents a serious concern for human health and environmental sustainability. MPs pose risks to human health and ecosystems due to their long-standing existence in the environment. Conventional removal methods like filtration and biological degradation often prove inadequate for effectively addressing MP contamination. This study explores magnetic separation as a promising solution, utilizing advanced magnetic materials, including nanoparticles, to enhance the removal efficiency. By leveraging hydrophobic interactions, chemical modifications, and tailored additives, these materials offer a scalable and eco-friendly approach for mitigating MP pollution with improved separation performance. Bioinspired and biohybrid magnetic materials present further advancements, mimicking biological systems to capture MPs with high efficiency. Additionally, magnetic polymer composites provide promising options due to their stable, multifunctional structures. Furthermore, advanced degradation methods complement these removal techniques by breaking down MPs to less harmful compounds, with processes such as photodegradation, photocatalytic, and electrochemical oxidation enhancing degradation rates. Integrating magnetic separation with degradation processes offers a comprehensive approach, addressing both MP capture and breakdown. This combined strategy provides a promising, cost-effective approach to reduce MP contamination in ecosystems, promoting environmental sustainability.
1. Introduction
Plastic pollution, especially microplastics (MPs) possessing a diameter <5 mm, is a major environmental concern globally. Synthetic plastics such as Bakelite were first developed in the early 20th century, with their widespread use in civilian applications occurring post-World War II.1 Between 1950 and 2015, global plastic waste generation reached approximately 6300 million tons, a figure projected to nearly double to 12 000 million tons by 2050.2 Plastics are basically long chains of carbon bonded with other elements including hydrogen, nitrogen, oxygen, sulphur, and chlorine. Based on the number and interlinking of carbon chains, and bonding with other elements and functional groups, plastics have a wide range of variant polymers such as polyether sulfone (PES), polyvinyl chloride (PVC), polyethylene (PE), polypropylene (PP), polystyrene (thermocole, PS), polyethylene terephthalate (PET), high-density polyethylene (HDPE) etc. Plastics are used in a broad variety of items nowadays as they are inexpensive, easy to produce, inert (resistant to chemicals, heat, and light), have a high strength/weight ratio, and are water resistant.3 The durability of plastics results in their significant resistance to degradation, posing substantial challenges for plastic waste disposal.4
Plastic materials that are being discarded to aquatic and terrestrial ecosystems eventually degrade via phenomena like photodegradation and mechanical fragmentation to a range of smaller plastic debris.5 This domain of plastic particles is categorised as macroplastics (>25 mm), mesoplastics (5–25 mm), microplastics (0.1–5 mm), and nanoplastics (NPs; <100 nm).6 The term “microplastics (MPs)” was first coined by Thompson et al. in 2004.7,8 MPs are defined as plastic particles measuring less than 5 mm that originate from a number of sources like cosmetics, agricultural and industrial waste, as illustrated in Fig. 1. They may be classified as primary and secondary MPs. Primary MPs are intentionally created to be less than 5 mm in size, whereas secondary MPs result from the breakdown of larger plastic debris, industrial processes, and the use of synthetic fibers in textiles.9 MP pollution has emerged as an urgent global environmental crisis.10,11 MPs are rapidly contaminating marine and terrestrial ecosystems, entering the food chain, and posing significant risks to biodiversity and human health. Various studies have shown that pathogenic bacteria such as Pseudomonas and Bacillus, are more abundant on MPs than in surrounding soil.12 MPs facilitate biofilm formation that can enhance pathogen persistence and gene transfer.13 These biofilms may promote antibiotic resistance, posing significant risks to microbial health and ecosystem stability. Ingesting these plastics can have a negative impact on the survival and well-being of aquatic and terrestrial creatures.14 MPs in particular, leached by large plastic surfaces, emit toxic effects in the human body. In addition, such MPs can consequently enter into animal or human bodies through inhalation or consumption and may cause carcinogenicity, DNA damage, and oxidative stress.15 Despite increasing awareness, effective removal and degradation strategies for MPs remain inadequate. This significant environmental challenge requires urgent research in the area of effective MP removal strategies. Current studies predominantly focus on short-term effects, leaving uncertainties about MP degradation pathways and their byproducts over extended periods. The efficiency of conventional techniques including filtration, sedimentation and biological degradation for eliminating MPs has been shown to differ.16 For instance, primary treatments such as screening and sedimentation usually remove 70–80% of MPs, depending on the specific conditions and types of particles involved.17 Filtration methods can capture MPs effectively. However, it requires frequent maintenance, incurs high operational costs, and uses specialized membranes that are prone to fouling. In a recent study, a pilot-scale biofilter achieved MP removal of 79% by particle number and 89% by mass from treated wastewater.18 This technique effectively captured MPs larger than 100 μm. The initial concentration of 917 items per m3 (24.8 μg m−3) dropped to 197 items per m3 (2.8 μg m−3) post-filtration. Although, during the study, smaller MPs below 100 μm were difficult to retain, leading to their presence in the final effluent. Additionally, daily fluctuations in wastewater composition affected removal efficiency and the potential for filter clogging raised concerns about long-term performance, necessitating further optimization.
 |
| Fig. 1 Sources of MP pollution and its effect on ecosystems. | |
The selection of sieve mesh size or filter pore size directly influences the detection limits for the removal of MP particles. Small pore or mesh sizes were susceptible to rapid clogging due to the accumulation of organic and mineral debris.19 The filtered samples needed further purification to remove organic contaminants from MP particles and free MPs that were trapped in the filter pores. This purification can be done using enzymatic processes. In this context, a sequential treatment approach was employed in a study to achieve effective segregation.20 Samples undergo overnight incubation with sodium dodecyl sulphate (SDS) at high temperature, followed by enzymatic digestion with lipase, protease, and amylase. Afterwards, oxidative treatment with hydrogen peroxide was applied, followed by chitinase and cellulase treatments to degrade chitin- and cellulose-based residues. Washing and sieving steps ensured thorough purification. Another method of segregation of other pollutants and soil debris from MP particles is density separation.21 This method is a widely used technique for isolating MPs from environmental samples based on differences in density. It involves suspending the sample in a high-density solution, allowing heavier particles to settle while lighter MPs float and can be recovered. MPs generally exhibit lower density and can be separated based on variation in density when mixed in concentrated salt solution. Recent research has demonstrated an optimized density separation for MP purification using non-ionic surfactants, achieving up to 97 ± 2% PET separation from mixed samples. For instance, Tween 20 (TW20) improved MP purity from 69–85 to 76–96% by altering hydrophobic interactions. However, separation efficiency was lower for MPs with similar densities, and higher surfactant concentrations led to micelle formation, reducing effectiveness. While practical applications in environmental samples confirmed their potential, concerns still remain regarding scalability and the impact of surfactants on water chemistry.
Zinc chloride (ZnCl2) is widely used for separation processes.22 In a research study, a simple overflow density separation method was developed using ZnCl2 (1.7 g cm−3).23 The system achieved >95% recovery of dense MPs from sediment. The method effectively isolated polyamide, rubber, PVC, PET, and polyester, with an average recovery of 96 ± 0.6%. However, potential MP loss during decanting and the need for procedural refinements to enhance efficiency and minimize contamination were identified as key challenges. Around 59.09% of studies on atmospheric microplastics have utilized ZnCl2 for density-based separation.21,24,25 Despite its effectiveness, density separation is limited by the high cost of salt solutions, potential contamination from reagents, and inefficiency in separating MPs with densities similar to natural sediments.
Conventional methods for MP degradation from aquatic and terrestrial environments primarily rely on physical, chemical, and biological techniques. The common MP degradation approach involves biological treatment followed by AOPs or physiochemical processes such as pyrolysis.26 Microorganisms broke down plastic polymers to smaller pieces or benign byproducts via biological breakdown of MPs. Plastics like PET were broken down by enzymes like PETase and bacteria like Ideonella sakaiensis.27 Nevertheless, effectiveness varied according to the kind of polymer, ambient factors, and microbial activity, and breakdown rates were generally observed to be slow.
Conventional MP removal techniques face challenges in scalability, efficiency, and environmental impact. Filtration and sedimentation processes experience constraints with nanoscale MPs, whereas chemical methods generate secondary pollution. On the other hand, biological degradation is a slow and inconsistent process. Additionally, these conventional methods do not address the persistence of smaller MPs and NPs that can bypass removal and remain in the ecosystem.28 Conclusively, these strategies are not fully capable of addressing the complicated and broad nature of MP contamination, most likely due to characteristics such as MP size, material variety, and environmental dispersion.29,30 High energy demands and operational costs further limit the feasibility of such processes indicating an urgent need for innovative, sustainable, and cost-effective solutions. To overcome these drawbacks, researchers have explored advanced techniques that combine separation and degradation processes. Given these challenges, innovative approaches such as magnetic separation, photocatalysis, and hybrid degradation strategies have been explored to enhance MP removal efficiency. These methods address the limitations of conventional approaches by offering improved scalability, real-time monitoring, and integration with degradation processes. Furthermore, the use of magnetic materials and photocatalysts enables faster and more efficient MP breakdown, reducing environmental persistence.
In this context, magnetic separation techniques present a viable approach to address MP contamination. By incorporating ferromagnetic materials or magnetic nanoparticles (MNPs), these approaches allow the selective removal of MPs from contaminated environment. This method offers several advantages over conventional techniques, such as improved efficiency, lower operational costs, and the potential for real-time monitoring of removal processes. Additionally, the incorporation of chemical additives has been shown to significantly enhance the magnetic properties of MPs. Chemical additives such as Mg/Zn-modified biochar,31 magnetic carbon nanotubes,32 adhesive magnetic microrobots,33 and nano-Fe3O4 particles34 can considerably improve the magnetic characteristics of MPs. These modifications facilitate MP accumulation on material surfaces and eventually its removal from water samples, providing feasible methods for decreasing plastic contamination in aquatic ecosystems. Conclusively, the magnetic separation technique is an effective way to extract MPs from the environment providing a quick and precise separation. However, the persisting nature of MPs emphasizes the necessity for effective degradation methods. MPs can be degraded either through abiotic factors, such as light and chemical processes, or through biological mechanisms involving microorganisms.35 Degradation of MPs through abiotic processes involves use of chemical and physical agents.36 These physical and chemical factors include UV radiation, oxidation, thermal impacts, hydrolysis, and wave action, contributing to the breakdown of larger polymeric fragments to smaller and simpler fragments.37–39 On the other hand, biological factors involve microbial and enzymatic actions to degrade suspended MPs and NPs. Combination of removal and degradation strategies can be beneficial for developing thorough solutions to address MP pollution and its lasting effects.
In light of the above discussion, this review article presents a comprehensive discussion on the integration of magnetic separation with various degradation strategies including biodegradation, photocatalytic degradation, and electrochemical oxidation processes. It highlights their combined potential to address MP contamination. The existing literature often focuses solely on conventional methods or individual degradation techniques,40–43 and rarely on their synergistic combination. This review goes beyond conventional discussions by integrating novel hybrid systems, such as bioinspired magnetic microrobots and polymer-magnetic composites as emerging solutions for MP removal. These approaches hold significant potential for large-scale environmental remediation, making this study relevant to the fields of environmental science and engineering. This review uniquely bridges this gap by discussing hybrid technologies that enhance removal efficiency while minimizing environmental impact.
This article also focuses on advancements in MNP modifications, including the use of superparamagnetic iron oxide nanoparticles (SPIONPs) influencing MP removal efficacy. By bridging the gap between MP separation and degradation, this review introduced an innovative framework for holistic MP remediation, setting it apart from previously published works.
2. Magnetic materials for MP removal
Magnetic separation is an emerging technique used for the removal of MPs from aquatic as well as terrestrial environment. This method utilizes the distinctive characteristics of magnetic materials and the hydrophobic nature of plastics to facilitate its separation.44 This approach involves introducing magnetic materials such as ferromagnetic nanoparticles or magnetically sensitive additives in polluted environmental samples. These magnetic particles adhere to MPs facilitating its removal either directly or via agglomeration due to its hydrophobic qualities.45,46 The process is schematically illustrated in Fig. 2. MPs exhibit hydrophobicity, enabling strong interactions with hydrophobically modified MNPs. These MNPs enhance adsorption through hydrophobic-hydrophobic interactions, facilitating MP aggregation on their surface.47 Electrostatic interactions also play a role, where oppositely charged MPs and magnetic materials enhance adhesion.48 Once MPs are magnetized through particle attachment or direct coating, an external magnetic field facilitates its separation from water. This mechanism improves MP removal efficiency while allowing material recovery for potential reuse. For instance, hydrophobic Fe nanoparticles have been developed to magnetize plastics using direct binding to the hydrophobic surfaces of MPs enabling its magnetic recovery.44 Another study investigated MP removal using MNPs under varying water conditions.49 The results showed 83.1–92.9% removal within 1 hour, influenced by particle aggregation. Electrostatic interactions played a key role, with acidic and saline conditions enhancing overall removal efficiency. These findings support MNP-based strategies for nano-scale MP remediation in water. Table 1 provides an overview of the MP removal efficiencies achieved by different magnetic materials and methodologies. Small MPs (<20 μm) showed high recoveries, averaging around 92% under the tested conditions, while average recoveries were reported at 93% for large MPs, with specific rates of 96% for PP, 92% for PVC, and 105% for polyurethane (PU). Similarly, another study investigated magnetic seeded filtration for the removal of MPs from dilute suspensions.50 Agglomeration was facilitated by electrostatic interactions between magnetic and non-magnetic particles. The study found that ionic strength and pH significantly impacted the efficiency of the process, achieving up to 95% separation efficiency. Once the magnetization of MP completed, an external magnetic field was provided to the system. It allowed the MP-magnetic particle complexes to get isolated and extracted from the medium.
 |
| Fig. 2 Removal of MPs by (A) direct magnetising MPs under external magnetic field and (B) agglomeration of MPs showing separation under an external magnetic field or by sedimentation. | |
Table 1 An overview on MP removal efficiency achieved by different magnetic materials
Magnetic material |
MP type |
MP size (μm) |
Removal efficiency (%) |
Separation method used |
Ref. |
‘*’ and ‘—’ represent mm and data not available, respectively. |
Hydrophobic Fe nanoparticles |
PE and PS |
<20 |
92 |
Hydrophobization of Fe nanoparticles facilitates sorption to MPs |
44
|
Magnetite (Fe3O4) particles |
PVC & PMMA |
<1* |
95 |
Magnetic-seeded filtration (hetero-agglomeration) |
50
|
Nano-Fe3O4 |
PE, PP, PS and PET |
200–900 |
>80 |
Surface absorption |
34
|
Iron oxide nanoflowers |
PE bead |
375 |
— |
Surface absorption through ultrasonic treatment |
51
|
Magnetic magnesium hydroxide |
PE |
≤270 |
∼92 |
Coagulation-flocculation treatment |
52
|
Hydrophobic magnetite |
PET, PP, LDPE, PVC, and PS |
— |
80–100 |
Selective hetero-agglomeration between magnetic seed particles and (nonmagnetic) target particles |
53
|
Magnetic sepiolite |
PE |
∼48 |
98.40 |
Coprecipitation |
54
|
Fe3O4 magnetic medium carrier |
PVC |
48–374 |
∼100 |
Surface adsorption |
55
|
Magnetic maifanite |
PS |
48 |
98.46 |
Surface adsorption |
56
|
Magnetic carbon nanotubes |
PE, PET, PA |
48 |
100 |
Magnetic carbon nanotubes adsorption |
32
|
Magnetic zeolitic imidazolate framework nanocomposite |
PS |
1.1 |
≥98 |
Surface adsorption |
57
|
Surface modified iron nanoparticles |
PE, PET, and PTFE |
63–15 |
≥87 |
High-gradient magnetic separation |
58
|
Magnetic corncob biochar |
PA |
<75 |
∼97 |
Adsorption by hydrophobic interaction, and electrostatic interaction |
59
|
Fe3O4 superhydrophobic magnetic adsorbents modified by different saturated fatty acids |
PS |
— |
92.89 |
Hydrophobic interaction |
60
|
In a recent study, flower-shaped iron oxide nanoflowers were used to bind with PE MPs using ultrasonic treatment followed by separation using a permanent magnet.51 Under optimal conditions, this approach successfully removed up to 1000 mg of MPs per g of nanoflowers. Additionally, another approach utilized Fe3O4 magnetic medium carriers that effectively enveloped MP particles leading to the formation of strong magnetic aggregates.55 These aggregates were then successfully captured with the help of a high-gradient magnetic filter. This approach attained 100% removal efficiency over time, showcasing the remarkable efficacy of magnetic separation methods in addressing MP contamination in water ecosystems. Conversely, magnetically modified biochar has emerged as a highly efficient and sustainable material for the removal of NP pollutants. A study demonstrated the removal of PVC-NPs using magnetic biochar, achieving 94% removal with magnetic corncob biochar and 92% with magnetic walnut shell biochar.61 The observed adsorption was influenced by surface iron oxide content and ionic composition. Monovalent ions enhanced removal whereas divalent ions reduced efficiency. Additionally, the magnetization of PVC-NPs by iron oxides facilitated their aggregation, enabling easy separation via an external magnetic field. These findings highlight the potential of magnetic biochar for wastewater treatment applications, offering a cost-effective and scalable solution for NP remediation.
2.1. Economic feasibility of magnetic separation for MP removal
Magnetic separation is an efficient method for MP removal, but its large-scale application remains costly. The production of MNPs like Fe3O4 and other magnetic materials requires energy-intensive methods like coprecipitation and sol–gel synthesis, increasing operational expenses.62 The cost of magnetic material synthesis depends on purity and surface modifications that impact both efficiency and scalability.63 A study reported that magnetic separation consumed ∼0.036 kW h m−3, reducing long-term operational expenses.63 The research also showed that the removal efficiency exceeded 90%, making it suitable for large-scale MP remediation. Additionally, material recovery rates of 95–98% led to lower recurring expenses and enhanced cost-effectiveness. A study estimated the treatment cost of low-gradient magnetic separation (LGMS) at $0.13 per cubic meter, with magnetic materials accounting for 33% of total expenses.62 The cost of surface-functionalized Fe3O4 nanoparticles (FNPs) is around $119 per kg, with minimal expected reductions. High-gradient magnetic separation (HGMS) is more costly, with operational expenses of $85.85 per hour ($0.52 per cubic meter), primarily due to high energy consumption. Over 52% of HGMS costs stem from power use, pumping, and flushing systems. Therefore, alternative synthesis methods have been explored to lower costs. Biochar-supported magnetic composites and waste-derived iron oxides serve as affordable substitutes for high-purity engineered nanomaterials.64 Despite these advantages, significant challenges remain in large-scale production, including maintaining cost-effectiveness, enhancing material recovery efficiency, and ensuring the long-term stability of these alternative materials. Further research is required to address these limitations and improve the practical applicability of magnetic materials for the removal of MP pollutants.
2.2. Magnetic nanoparticles
In recent years, the potential of MNPs has been explored as a novel solution to address the growing MP pollution crisis. MNPs exhibit unique properties such as high surface area, superparamagnetism, and chemical tunability, making them effective for adsorption of MPs and NPs followed by their removal from contaminated sources. MNPs demonstrate these remarkable characteristics and related phenomenon owing to its compact dimensions and the surface efficacy of these particles.65 MNPs are comprised of regions known as magnetic domains that exhibit a magnetic moment in a distinct direction. As an external magnetic field is introduced, the domains coincide with the field and the particles get magnetized and cluster around it.66 MNPs derive their properties from materials such as iron, nickel, cobalt, and their oxides, as well as compounds with multiple metals like copper, zinc, manganese, barium, and strontium.65,66 In particular, SPIONs, including Fe3O4 and metal-substituted ferrites, have gained significant attention over the past decade.67 This interest is driven by their biocompatibility, chemical stability, cost-effectiveness, and distinct magnetic properties, making them highly valuable in various applications. A study explored the use of ultra-thin magnetic Fe3O4 nano discs (NDs) for the adsorption and separation of MPs and NPs from water.68 The NDs showed an impressive adsorption capacity of 188.4 mg g−1 for particles ranging from micro- to nano-scale, driven by electrostatic and magnetic forces. Notably, the NDs retained over 90% removal efficiency even after five continuous cycles. Recovery of NDs involved magnetic separation followed by calcination at 500 °C under a nitrogen atmosphere for 2 hours, effectively decomposing adsorbed MPs. The regenerated NDs maintained high adsorption capacity, demonstrating strong stability and reusability.
Recent research showed that the removal efficiency of MPs using MNPs can be greatly influenced by extrinsic factors such as dissolved organic matter, pH and the salinity of the medium.69 It has been observed that FNPs facilitated the MP aggregation resulting in a removal rate of 83 to 92% within 1 hour. Furthermore, electrostatic interactions also played a key role in the higher removal rate observed under acidic conditions (pH < 6.7) due to interaction between positively and negatively charged MPs. The increased salinity [4.47–66.8% (0% NaCl) to 55.1–74.8% (1% NaCl)] enhanced aggregation by compressing the electrical double layer. Another study investigated the removal of polystyrene (PS) MPs from water using CuFe2O4, achieving a high removal efficiency of 98.02% at 0.2 g L−1.70 Among environmental factors, dissolved organic matter like fulvic acid (FA) had the most significant impact. At an FA concentration of 5 mg L−1, the removal efficiency dropped to 45.75% while at 20 mg L−1, it further decreased to only 7.77%. This decline was due to formation of the complex functional groups in FA that occupied the active sites on the CuFe2O4 surface, thus preventing effective interaction with PS MPs and hence the removal efficiency was reduced. However, after use, CuFe2O4 was magnetically separated, dried, and regenerated via pyrolysis at 500 °C for 4 hours. This thermal treatment effectively removed adsorbed MPs, restoring its adsorption capacity. Even after four reuse cycles, CuFe2O4 retained 83.88% of its initial removal efficiency, demonstrating its strong recyclability and stability in MP remediation.
2.3. Bioinspired magnetic materials
Various novel strategies including chemical recycling, enzymatic degradation, pyrolysis, magnetic separation, and solvent-based extraction for the separation and recycling of plastic materials have been explored to eliminate the problem of plastic pollution.71,72 Among them, bioinspired magnetic separation methods have emerged as one of the viable options.73 By employing biological principles, these techniques enhance the efficacy and efficiency of the separation of plastic contaminants. Bioinspired magnetic separation applies concepts of biomimicking magnetic actuation to improve the removal of contaminants like MPs and NPs from water environments.74 Interestingly, bioinspired magnetic materials are designed to mimic the properties and functionalities of biological systems, utilizing the prepared magnetic composites to achieve desirable performance characteristics of MP removal. These materials are engineered to replicate the movement and behaviour of living organisms, allowing for controlled motion when subjected to external magnetic fields, as shown in Fig. 3.71 Advancing the notion of bioinspired magnetic separation, a novel strategy for mitigating MP pollution in aquatic environments involves using reconfigurable liquid metal microrobots termed as “LiquidBots”.75 These microrobots were coated in gallium oxide, which enhanced their magnetic control and electrostatic interactions. This design mimicking zoetic mobility enabled efficient removal of MP particles from water. The investigation indicated that the microrobots were capable of achieving 74.3 and 82.1% removal of MPs and NPs, respectively, in a span of 2 hours. The study also demonstrated their regenerative ability through sonication, reinforcing their role as a sustainable approach to overcome plastic waste. LiquidBots were recovered through magnetic retrieval followed by sonication in HCl resulting in further removal of MPs and dissolved Ga2O3. The resultant material e-coalesced to GaIn-Fe and was subjected to sonication in PVP HCl to restore functionality. This process allowed multiple regeneration cycles with minimal material loss. Further advancing the application of bioinspired methods, a magnetic microsubmarine based on a sunflower pollen grain was developed for the recyclable removal of large microparticles (100 μm in diameter).73 It achieved over 70% removal efficiency through non-contact shoveling. This approach utilized microsubmarine movement to generate fluid flow. Microsubmarines were magnetically retrieved, washed with ethanol to remove adsorbed pollutants, and dried for reuse. This regeneration process maintained their structure, magnetic actuation, and adsorption efficiency over multiple cycles without significant performance loss. This flow facilitated the capture and transport of MPs and pollutants without direct contact. This approach seems promising as it does not require additional chemicals or invasive methods, thereby making it a sustainable solution for water remediation.
 |
| Fig. 3 Schematic diagram illustrating the controlled zoetic locomotion achieved by microrobots under the influence of an external magnetic field. | |
2.4. Biohybrid magnetic materials
Biohybrid magnetic materials are composite systems that combine biological components such as cells or microorganisms with magnetic materials to create functional entities in order to achieve specific functionalities such as biodegradability, magnetic manipulation, and motility.76 The biological components of biohybrids such as functionalized bacteria, can enhance biodegradability and facilitate the breakdown of MPs to less harmful substances over time. The magnetic properties enable precise locomotion and wireless manipulation of the biohybrid materials when subjected to an external magnetic field, leading to effective removal of contaminants. A recent study addressed the biohybrid microrobots, referred to as magnetic algae robots (MARs) created by decorating the surface of the microalgae Chlorella vulgaris with positively charged FNPs.77 By integrating MNPs with algae, the MARs could achieve precise locomotion and manipulation under an external magnetic field, enhancing their ability to target and collect plastic pollutants. These MARs demonstrated impressive removal efficiencies of 92% for NPs and 70% for MPs when operated under an external magnetic field. This innovative approach aimed to address the growing environmental issue of MPs and NPs in a cost-effective and eco-friendly manner. Other innovative algae-based hybrid bio-microrobots have been engineered to address MP contamination in aquatic systems.78 By functionalizing algae with biphenolic acids, researchers enhanced the capability of microrobots to effectively recognize and adsorb MPs. It achieved removal efficiencies between 83.1 and 87.7% thereby indicating its potential as a stable solution for mitigating MP pollution in aquatic environments.
A research study introduced a bacterial consortium immobilized in magnetically active floating biochar gel beads that effectively removed high molecular weight polycyclic aromatic hydrocarbons from marine environment.79 This system achieved removal efficiencies of 89.8% for pyrene (PYR), 66.9% for benzo(a)pyrene (BAP), and 78.2% for indeno(1,2,3-cd)pyrene (INP). The magnetic characteristics of the biochar beads facilitated its easy retrieval using an external magnetic field. It also prevented any secondary pollution risks post-biodegradation in marine ecosystems. The versatility and effectiveness of microbial consortia immobilized in magnetic materials demonstrated high efficiency in removing high-molecular-weight polycyclic aromatic hydrocarbons. This method showed a potential strategy for the removal of persistent MPs using similar bio-magnetic approaches.
3. Magnetic polymer composites
A hybrid magnetic system integrates both magnetic and polymeric characteristics by typically employing materials such as iron oxide, cobalt, and nickel alloys within a polymeric matrix.80 These composites may be synthesized by various methods such as blending, in-situ polymerization,81 or coprecipitation,82 ensuring stable dispersion of magnetic particles within the polymer structure. The formed structure allows for targeted magnetic actuation, enabling easy capture and removal of MPs from water as well as providing potential for degradation of MP pollutants. This dual functionality makes magnetic polymer composites highly promising for advanced wastewater treatment. The production of magnetic polymer particles can be categorized in three main approaches.80 To improve stability and effectiveness, MNPs are produced initially and subsequently coated with a polymer shell using the magnetic core–polymer shell technique. Another approach is the polymer core–magnetic shell technique that initially forms polymer microspheres. This is followed by the deposition of magnetic material on the surface to efficiently integrate the magnetic properties within the polymer matrix. Finally, in the embedded magnetic particle method, the magnetic particles are embedded directly within the polymer matrix. This method involves the synthesis of MNPs and multiple polymer microspheres that may be combined through physical interaction to form stable composites. These production methods are important for creating efficient magnetic adsorbents that are key in tackling MP pollution, particularly in water bodies. Based on these production techniques, magnetic adsorbents utilize these composite materials to effectively capture and retain pollutants like MPs/NPs using magnetic forces.
Magnetic adsorbents are different from conventional adsorbents that strictly rely on surface interactions. Magnetic adsorbents contain magnetic particles that attach to MPs and NPs, forming stable complexes, and provide efficient removal of MPs from water under the influence of an external magnetic field.83 MPs/NPs may adhere to a magnetic carrier medium in magnetic separation, providing them with the magnetic characteristics required for efficient extraction. A study investigated magnetic sepiolite as a carrier to improve PE removal from water.56 The co-precipitation method yielded a manipulable material within a magnetic field. The highest removal efficiency of 98.4% was achieved after 600 seconds, with turbidity reduced to 2.6 NTU. The agglomeration of PE with magnetic sepiolite occurred swiftly, with initial removal observable within 6 seconds and near-total removal within 120 seconds under a magnetic field. Another research study focused on the synthesis of SPIONPs as a magnetic carrier medium that can attract oppositely charged MPs, forming aggregates that were easily collected by magnets.84 The experimental setup systematically investigated the magnetic removal of MPs/NPs across sizes ranging from 100 nm to 100 μm. The removal efficiency for MPs ranging from 1–5 μm was observed to be 5.38 g of MPs per g of SPIONPs under the influence of an external magnetic field. While MPs can be efficiently removed using magnetic separation, NPs pose a relatively high challenge due to their small size and high stability in water. To address this challenge, magnetic ZIF-8 nanocomposites (Nano-Fe@ZIF-8) for the removal of PET-NPs and depolymerization were developed.85 They have been shown to achieve rapid magnetic aggregation and glycolytic breakdown to bis(2-hydroxyethyl) terephthalate within 30 minutes. This method enabled accurate quantification at ppm levels. However, challenges remain in regenerating the material and optimizing the reaction conditions for large-scale applications.
4. MP degradation
MP degradation is a process involving the breaking down of MPs to smaller fragments or monomers, or their alteration through environmental factors, biological organisms, or chemical reactions. This transformation happens due to interactions with physical, biological, or chemical agents in the environment. Larger plastic waste can be break down to smaller fragments by physical processes such as mechanical abrasion,86 UV-induced photodegradation,87 and thermal degradation.31,86 Although the particle size may decrease as a result of these approaches, the plastic traces may not be entirely mineralized or eliminated. MP degradation can be addressed through a range of methodologies, including biological, chemical, and physical interventions. Biological degradation involves breaking down of these MP materials to smaller oligomers and monomers with the aid of microorganisms.88 However, this process may extend over several months due to the inherently slow rates of degradation. Other methods such as AOPs have also emerged as effective chemical methods, generating reactive radicals that oxidize and decompose MPs, with techniques like photocatalysis and Fenton-like reactions being extensively studied. Although, degradation of MPs involves physical, biological, and chemical processes, its effectiveness depends on the extent of polymer breakdown. As illustrated in Fig. 4, MP removal via magnetic separation follows multiple degradation pathways. Complete degradation through biodegradation or AOPs mineralizes MPs to CO2 and H2O, eliminating environmental risks. However, partial degradation may result in the formation of NPs and toxic intermediates that persist in the ecosystem. Additionally, incomplete removal may lead to secondary pollution, where unrecovered MPs re-enter water bodies. An alternative solution is recycling and reuse, where collected MPs are re-processed to new materials. Understanding these post-separation pathways is crucial for designing sustainable MP remediation strategies.
 |
| Fig. 4 Fate of MPs post magnetic separation. Possible outcomes include complete degradation, partial breakdown to NPs, secondary pollution, or recycling and reuse. | |
4.1. Biodegradation of MPs
The biodegradation process involves the use of microorganisms, such as bacteria and fungi, to degrade MPs and NPs. Anthropogenic MP pollution significantly influencing the microbial ecosystem may cause stressful conditions for microorganisms. Due to the substantial amount of MPs in the natural environment, microorganisms have evolved to endure the harsh circumstances caused by these pollutants. Significant exposure of these contaminants affects the production of degrading enzymes in microorganisms, as these organisms adapt to the stressful conditions created by MPs in their environment.89 Microorganisms utilize enzymes such as cutinase and hydrolases to break down MPs to simpler compounds that can be used as carbon sources.90 The first purified enzyme known to break down PE was manganese peroxidase, as evident by a decrease in its average molecular weight and tensile strength.91 MPs can be depolymerized either directly by microbes or microbial enzymes through the process of hydrolysis. The microbial-assisted enzymatic degradation of MPs occurs through the activity of extracellular or intracellular depolymerases. In extracellular degradation, microorganisms release enzymes in the environment to break down MPs to smaller molecules that can be absorbed. Enzymes like PETase catalyze the hydrolysis of ester bonds in PET.92 They bind to PET surfaces and breaks ester linkages through nucleophilic attack. This cleavage generates intermediate oligomers, which undergo further hydrolysis to monomers as illustrated in Fig. 5. The final products, terephthalic acid and ethylene glycol, can be utilized in biochemical recycling processes. On the other hand, microbes utilize their own carbon reservoirs for hydrolysis in the case of intracellular depolymerization.93
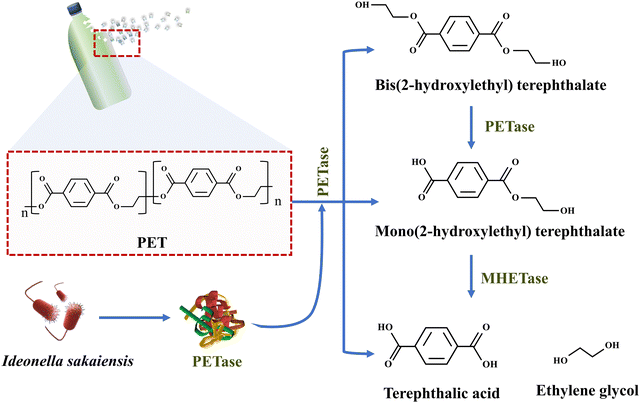 |
| Fig. 5 Enzymatic hydrolysis of PET by PETase and MHETase produced by microbes like Ideonella sakaiensis, leading to its breakdown to terephthalic acid and ethylene glycol. | |
A study found that microbial enzymes specifically laccases and laccase-like multicopper oxidases (LMCOs) from the fungal strain Aspergillus flavus PEDX3, play a crucial role in the biodegradation of PE MPs.94 These enzymes increase the hydrophilicity of the PE surface, facilitating microbial attachment and subsequent degradation. The laccases and LMCOs were identified as key enzymes capable of catalyzing the oxidation of various substrates, potentially aiding in the breakdown of PE MP particles. Enzymatic degradation of plastic is often considered a slow process greatly dependent on the monomeric arrangement of the polymer.95 A recently conducted research study demonstrated excellent PET degradation efficiency of hydrolase enzyme found in Thermobifida fusca (TfH).96 TfH achieved around 50% weight loss in over three weeks for commercial PET. The results indicated that the degradation rate depends on the crystallinity of PET and the incubation temperature. PET with low crystallinity allowed more efficient degradation by the enzyme. The high activity of TfH was attributed to its thermophilic origin, which supports the effective performance at elevated temperatures. The integration of magnetic separation and degradation presents a promising avenue for large-scale MP remediation. Multifunctional magnetic composites allowing adsorption and catalytical degradation of MPs offer a sustainable solution. A recent study demonstrated the combined effect of superparamagnetic separation and nanozyme-assisted degradation for MP removal.97 Bare Fe3O4 nanoaggregates efficiently captured diverse MPs via hydrogen bonding, achieving rapid magnetic separation. The peroxidase-like nanozyme activity of Fe3O4 catalyzed oxidative MP degradation with nearly 100% efficiency at elevated temperatures. After degradation, Fe3O4 retained its magnetic and catalytic properties, allowing easy recovery and reuse. Another research study on mussel-inspired adhesive magnetic microrobots demonstrated the dual capability of MP capture and enzymatic degradation.98 The polydopamine-coated Fe3O4 microrobots exhibited strong adhesion to MPs, enabling efficient retrieval under a magnetic field. The immobilization of lipase on their surface facilitated enzymatic degradation, breaking down MPs post-separation. To test reusability, the microrobots were recovered after separation and subjected to an ultrasound bath to remove residual MPs. However, after multiple cycles, the polydopamine layer detached resulting in reduction of adhesion efficiency. The Fe3O4 core remained intact and could be recoated with polydopamine for reuse, ensuring the sustainability of the process. This dual-function approach offers a sustainable and cost-effective strategy for MP remediation. Future research should focus more on optimizing such kinds of hybrid systems for enhanced efficiency and environmental safety.
4.2. AOPs
AOPs are considered as effective methods for degrading MPs through various mechanisms that involve generation of highly reactive hydroxyl radicals (˙OHs) and superoxide anions (O2˙−), that interact with the polymer structures of MPs. AOPs are essential for mitigating MP pollution, utilizing three distinct degradation methodologies i.e., direct photodegradation, photocatalytic oxidation, and electrochemical oxidation.99 Direct photodegradation employs solar energy to catalyze photochemical reactions that break down MPs to simpler molecules.100 On the other hand, photocatalytic oxidation utilizes semiconductors like titanium dioxide (TiO2) to generate reactive radicals upon light exposure resulting in expediting plastic decomposition.101 Next, electrochemical oxidation utilizes an electrical current to yield reactive oxidants that break plastic polymers effectively.100 Collectively, these strategies demonstrate the various applications of AOPs, each designed to utilize specific energy modalities for improved MP degradation. Recent research work presented an innovative degradation method for MPs utilizing AOPs with functionalized carbon nano springs.102 Manganese was encapsulated in nitrogen-doped carbon nanotubes serving as a catalyst. The system achieved a 50 wt% removal rate of MPs and showed that non-toxic degradation intermediates served as nutrients for algae. This study suggested a promising and eco-friendly alternative to conventional metal-based degradation methods.
4.2.1. Direct photodegradation.
The phenomenon of direct photodegradation has emerged as an effective method for tackling the plastic pollutants related to ecological challenge. Direct photodegradation is a primary pathway for the breakdown of atmospheric organic matters. It plays an essential role in the decomposition of hydrocarbons and polymers in the environment.103 Recent research has reported that UV radiation can change the physicochemical properties of plastics including PE, PS, PET, and PVC.104 These changes can lead to their decomposition as UV photolysis causes cracks to form on the plastic surface, subsequently making the surface rougher. With the passage of time, the plastic breaks into smaller fragments, with sizes ranging from nm to μm. However, direct photodegradation is a slow process and takes a long period of time to degrade a small amount of plastic. Also, the process of direct photolysis is shown to have insufficient potential to breakdown the micro and nano range of plastic contaminants.105,106
4.2.2. Photocatalytic oxidation.
Photocatalytic oxidation is a process where a photocatalyst is activated by solar energy to generate reactive oxygen species (ROSs). These ROSs such as ˙OH & O2˙− promote the degradation of organic pollutants, including MPs and NPs.107 These radicals induce oxidative stress by attacking polymer bonds in MPs. The radicals such as ˙OH & O2˙− initiate electron transfer and hydrogen abstraction, weakening molecular stability. This triggers chain scission by breaking long polymer chains to smaller fragments.108 Continuous oxidation converts these fragments to oxygenated intermediates, such as carbonyl and carboxyl groups. Further oxidative degradation mineralizes these intermediates, ultimately forming CO2 and H2O. The degradation of plastics in the presence of photocatalysts relies on a series of key processes, as illustrated in Fig. 6. Initially, the photocatalyst absorbs solar energy that generates electron–hole pairs. These charges then migrate from inside the photocatalyst material to its surface resulting in the creation of active sites. At the surface, the charges interact with the reactants that initiate redox reactions leading to the breakdown of the plastic molecules. This process effectively utilizes solar energy and the unique surface properties of the photocatalyst to accelerate plastic degradation in a sustainable manner.109
 |
| Fig. 6 An overview illustrating the mineralization of MPs under the influence of photocatalytic activity. | |
In a study, zinc oxide nanorods (ZnO-NRs) were used as a photocatalyst for the degradation of PP MPs using photocatalytic oxidation.110 The ZnO-NRs setup achieved a 65% reduction in particle volume after an incubation of 456 hours under visible light exposure. The study demonstrated effective photodegradation through chain scission mechanisms and produced non-toxic by-products such as acrolein (propenal), ethynyloxy/acetyl radicals, butyraldehyde, hydroxypropyl, acetone, and pentyl groups. The chain scission mechanism initiated with the formation of free radicals involving cleavage of polymer chains, leading to the generation of reactive species. Once free radicals are formed, they react with O2 and other molecules and generate hydroperoxide radicals (˙OOHs). These radicals further reacted to produce alkoxy and OH˙ that continued the degradation process. The process ends through cross-linking reactions, where different radicals combined and effectively stopped the chain scission. This resulted in a variety of degradation products, including aldehydes, ketones, and alcohols. Another study on PVC–TiO2 composite films demonstrated that TiO2 as a photocatalyst significantly enhanced photocatalytic degradation for polymer-based materials and offered insights applicable to MP degradation.111 Under ambient air, the TiO2-embedded PVC films showed a two-thirds reduction in molecular weight and 27% weight loss after 300 hours of irradiation. This process generated CO2, H2O, and visible cavities, supporting the potential of TiO2 in accelerating MP degradation through ROS formation. Similarly, a new quaternary layered double hydroxide composite photomaterial CuMgAlTi-R400 effectively demonstrated MP degradation under visible light irradiation.112 The research also illustrated that after 300 hours, the size of the PS particles decreased by 54.2%, and PE decreased by 33.7%. The degradation process involved the formation of hydroxyl and carbonyl intermediates, leading to chain breaking of the polymer molecules and the production of ketones, aldehydes, and esters, along with the release of volatile intermediates. The results ultimately showed that MPs were mineralized to water and CO2. The findings indicated that CuMgAlTi-R400 proved to be an effective photocatalyst for degrading MPs under visible light. A summary of some recent studies on MP degradation efficiencies achieved through various photocatalytic materials and conditions is provided in Table 2. The photocatalytic oxidation method for MP degradation shows potential but is limited to small-scale applications. The preparation and reproducibility of catalysts present significant challenges and cost concerns. Therefore, further investigation is needed to determine the applicability of photocatalytic oxidative degradation technology.
Table 2 Recent studies on MP degradation via photocatalytic oxidation reported in the literature
Photocatalyst |
MP type |
Treatment duration (h) |
Degradation efficiency (%) |
Ref. |
‘—’ represents data not available. |
ZnO NRs |
PP |
456 |
∼65 |
110
|
CuMgAlTi |
PS |
300 |
54.2 |
112
|
N-TiO2 |
HDPE |
50 |
4.65 |
113
|
TiO2 |
PS |
12 |
98.40 |
114
|
BiOI/TiO2 |
PVC |
336 |
30.8 |
115
|
Ag/TiO2/RGO |
PE |
4 |
76 |
116
|
Boron-doped goethite |
PE |
300 |
12 |
117
|
FePc-TiO2 |
PS |
— |
35 |
118
|
BiOCl-X |
PE |
24 |
5.38 |
119
|
TiO2 |
PP |
50 |
50.5 ± 0.5% |
120
|
4.2.3. Electrochemical oxidation.
Electrochemical oxidation is an advanced treatment technology that utilizes electrochemical processes to generate reactive species for breaking down of complex plastic polymers to less harmful substances or value-added chemicals. Electrochemical advanced oxidation processes (EAOPs) offer advantages over photocatalytic oxidation.121 EAOP technology is simpler to operate, produces less secondary pollution, and performs reliably. EAOP has proven effective in degrading various toxic and resistant organic pollutants making it a promising approach for managing MP contaminants.121 An electro-Fenton-like (EF-like) technology has been developed using a TiO2/graphite cathode that offered a sustainable method for degrading PVC-MPs.122 The method achieved a 75% dichlorination efficiency and 56% weight removal of PVC MPs in a period of 6 hours of treatment at elevated temperature (100 °C). The efficiency of MP degradation via electrochemical oxidation was notably influenced by the selection of anode material, that regulates the generation of reactive species. The efficacy may further be enhanced by the anode modifications. The impact of anode modification on MP degradation efficacy was demonstrated using a CeO2–PbO2 anode for electrochemical oxidation.123 This significantly improved the degradation of PVC MPs. A weight reduction of 38.67% was achieved within a 6-hour treatment period. The addition of CeO2 notably increased the electrocatalytic efficiency compared to using pure PbO2 alone.
It has been found that the introduction of certain surfactants in the electrochemical system may be helpful to overcome certain challenges such as limited oxidant activations, poor interaction with MP and risk of micelle formations.124 Given the challenges in achieving effective oxidant activation and MP interaction, the strategic addition of surfactants such as SDS emerged as a key innovation. By enhancing reactive species generation, SDS addressed critical barriers in MP degradation efficiency. This increase was particularly notable at higher current densities resulting in more effective oxidation of PS-MPs. SDS improved the interaction between electro-generated oxidative species and the insoluble PS-MPs. SDS in the electrochemical system promoted persulfate ion generation, making adequate SDS concentration essential for high persulfate levels during electrolysis. EAOPs offer an eco-friendly alternative for MP degradation, suggesting further research in optimizing anode modifications, environmental impact assessments, and scalability to address the global challenge of MP pollution more sustainably.
5. Conclusions
MP pollution presents a persistent environmental challenge, necessitating innovative and scalable removal strategies. This review highlights the integration of magnetic separation and advanced degradation techniques as a promising approach to mitigate MP contamination in aquatic and terrestrial ecosystems. Magnetic materials including nanoparticles, bioinspired systems and polymer composites, enable efficient MP capture by leveraging hydrophobic and electrostatic interactions. These materials facilitate rapid and selective separation while minimizing operational costs. Complementing magnetic removal, degradation techniques such as enzymatic hydrolysis, photocatalytic oxidation, and electrochemical processes enhance MP breakdown to non-toxic byproducts. Synergistic strategies combining separation and degradation improve overall remediation efficiency, offering sustainable alternatives to conventional methods. Further research in optimizing material properties, enhancing degradation kinetics, and ensuring environmental safety remains essential for large-scale applications. The continuous development of hybrid technologies integrating magnetic separation with advanced degradation will pave the way for effective and eco-friendly MP remediation solutions.
6. Challenges and future perspectives
MP removal through magnetic separation and advanced degradation methods presents several challenges that must be addressed for large-scale application. Material stability and reusability remain key concerns. MNPs and composites tend to aggregate over time, reducing their efficiency in capturing MPs. Surface fouling by organic matter and dissolved contaminants in natural environments further limits their long-term effectiveness. Designing anti-fouling and self-regenerating magnetic materials is crucial for sustained performance.
Another major challenge is the selectivity and efficiency of degradation techniques. AOPs such as photocatalysis and electrochemical oxidation, generate reactive species for MP breakdown. However, controlling reaction pathways to avoid toxic byproducts is a persistent issue. The integration of catalyst-functionalized magnetic materials could enhance degradation selectivity while enabling simultaneous MP capture and breakdown. Additionally, biohybrid systems utilizing engineered microorganisms or enzymes require improved retention strategies to prevent microbial loss and optimize degradation rates.
Future research should focus on developing hybrid materials that combine separation and degradation within a single system. Smart nanomaterials with responsive surface modifications could improve interaction with MPs under variable environmental conditions. The exploration of bioinspired microrobots for targeted MP capture and degradation represents an emerging frontier. Additionally, the integration of AI-driven monitoring and control systems with MP remediation technologies could enable adaptive, real-time adjustments for enhanced efficiency. Scaling up these innovations requires a multidisciplinary approach, bridging nanotechnology, environmental engineering, and biotechnology. Collaborative efforts should prioritize the development of cost-effective, eco-friendly, and energy-efficient systems to ensure practical deployment. Addressing these challenges will accelerate the transition from laboratory-scale studies to real-world MP remediation solutions, ultimately contributing to global environmental sustainability.
Data availability
No new data were generated or analyzed as part of this review.
Conflicts of interest
There are no conflicts of interest to declare.
Acknowledgements
Dr Priyanka Gupta is thankful to the Department of Science and Technology, Government of India, for awarding her an INSPIRE faculty fellowship and research grant (IFA22-ENG 345) in support of her research endeavors. She also expresses her gratitude to the Department of Chemical Engineering at the Harcourt Butler Technical University Kanpur for hosting her and providing laboratory space.
References
- R. Geyer, J. R. Jambeck and K. L. Law, Sci. Adv., 2017, 3, e1700782 CrossRef PubMed.
- Y. Zhang, H. Jiang, K. Bian, H. Wang and C. Wang, J. Environ. Chem. Eng., 2021, 9, 105463 CrossRef CAS.
- S. Sharma and S. Chatterjee, Environ. Sci. Pollut. Res., 2017, 24, 21530–21547 CrossRef PubMed.
- A. Sivan, Curr. Opin. Biotechnol, 2011, 22, 422–426 CrossRef CAS PubMed.
- S. Ziajahromi, D. Drapper, A. Hornbuckle, L. Rintoul and F. D. Leusch, Sci. Total Environ, 2020, 713, 136356 CrossRef CAS PubMed.
- Y. Zhang, Y. Luo, X. Guo, T. Xia, T. Wang, H. Jia and L. Zhu, Water Res., 2020, 178, 115861 CrossRef CAS PubMed.
- Y. Cao, C. Sathish, X. Guan, S. Wang, T. Palanisami, A. Vinu and J. Yi, J. Hazard. Mater., 2024, 461, 132537 CrossRef CAS PubMed.
- R. C. Thompson, Y. Olsen, R. P. Mitchell, A. Davis, S. J. Rowland, A. John, D. McGonigle and A. E. Russell, Science, 2004, 304, 838 CrossRef CAS PubMed.
- J. L. Conkle, C. D. Báez Del Valle and J. W. Turner, Environ. Manage., 2018, 61, 1–8 CrossRef.
- A. Zeb, W. Liu, N. Ali, R. Shi, Q. Wang, J. Wang, J. Li, C. Yin, J. Liu and M. Yu, J. Hazard. Mater., 2024, 461, 132636 CrossRef CAS PubMed.
- K. Li, L. Du, C. Qin, N. Bolan, H. Wang and H. Wang, Carbon Res., 2024, 3, 9 CrossRef CAS.
- N. Wu, Y. Zhang, Z. Zhao, J. He, W. Li, J. Li, W. A. Xu, Y. Ma and Z. Niu, Sci. Total Environ., 2020, 708, 134876 CrossRef CAS PubMed.
- M. Sun, M. Ye, W. Jiao, Y. Feng, P. Yu, M. Liu, J. Jiao, X. He, K. Liu and Y. Zhao, J. Hazard. Mater., 2018, 345, 131–139 CrossRef CAS.
- M. Smith, D. C. Love, C. M. Rochman and R. A. Neff, Curr. Environ. Health Rep., 2018, 5, 375–386 CrossRef CAS PubMed.
- S. Nikhar, P. Kumar and M. Chakraborty, Next Nanotechnol., 2024, 5, 100060 CrossRef.
- L. Mai, L. J. Bao, L. Shi, C. S. Wong, E. Y. Zeng and P. Research, Environ. Sci. Pollut. Res., 2018, 25, 11319–11332 CrossRef CAS.
- M. Carnevale Miino, S. Galafassi, R. Zullo, V. Torretta and E. C. Rada, Sci. Total Environ., 2024, 930, 172675 CrossRef CAS PubMed.
- F. Liu, N. B. Nord, K. Bester and J. Vollertsen, Water, 2020, 12, 1085 CrossRef CAS.
- T. A. Lastovina and A. P. Budnyk, J. Water Process Eng., 2021, 43, 102209 CrossRef.
- T. Mani, A. Hauk, U. Walter and P. Burkhardt-Holm, Sci. Rep., 2015, 5, 17988 CrossRef CAS.
- T. W. Crutchett and K. R. Bornt, MethodsX, 2024, 12, 102638 CrossRef PubMed.
- J. H. Seo, Y. Shin, I. Song, J. Lim, Y. S. Ok and S. Weon, TrAC, Trends Anal. Chem., 2024, 178, 117859 CrossRef CAS.
- T. W. Crutchett and K. R. Bornt, MethodsX, 2024, 12, 102638 CrossRef PubMed.
- S. Allen, D. Allen, V. R. Phoenix, G. Le Roux, P. Durántez Jiménez, A. Simonneau, S. Binet and D. Galop, Nat. Geosci., 2019, 12, 339–344 CrossRef CAS.
- L. Cutroneo, A. Reboa, I. Geneselli and M. Capello, Mar. Pollut. Bull., 2021, 166, 112216 CrossRef CAS PubMed.
- I. A. Ricardo, E. A. Alberto, A. H. Silva Júnior, D. L. P. Macuvele, N. Padoin, C. Soares, H. Gracher Riella, M. C. V. M. Starling and A. G. Trovó, Chem. Eng. J., 2021, 424, 130282 CrossRef CAS.
- C. E. Duru, I. A. Duru and C. E. Enyoh, Bull. Natl. Res. Cent., 2021, 45, 104 CrossRef.
- A. U. R. Bacha, I. Nabi and L. Zhang, ACS EST Eng., 2021, 1, 1481–1501 CrossRef CAS.
- Y. Zhang, A. Diehl, A. Lewandowski, K. Gopalakrishnan and T. Baker, Sci. Total Environ, 2020, 720, 137383 CrossRef CAS PubMed.
- S. L. Cherniak, H. Almuhtaram, M. J. McKie, L. Hermabessiere, C. Yuan, C. M. Rochman and R. C. Andrews, Chemosphere, 2022, 288, 132587 CrossRef CAS PubMed.
- J. Wang, C. Sun, Q. X. Huang, Y. Chi and J. Yan, J. Hazard. Mater., 2021, 419, 126486 CrossRef CAS PubMed.
- Y. Tang, S. Zhang, Y. Su, D. Wu, Y. Zhao and B. Xie, Chem. Eng. J., 2021, 406, 126804 CrossRef CAS.
- H. Zhou, C. Mayorga-Martinez and M. Pumera, Chem. Rev., 2021, 121(8), 4999–5041 CrossRef CAS PubMed.
- X. Shi, X. Zhang, W. Gao, Y. Zhang and D. He, Sci. Total Environ., 2022, 802, 149838 CrossRef CAS PubMed.
- S. S. Ali, T. Elsamahy, R. Al-Tohamy and J. Sun, Environ. Sci. Ecotechnology, 2024, 21, 100427 CrossRef CAS.
- J. Reineccius, M. Schönke and J. Waniek, Environ. Sci. Technol., 2022, 57, 963–975 CrossRef PubMed.
- K. Hu, P. Zhou, Y. Yang, T. Hall, G. Nie, Y. Yao, X. Duan and S. Wang, ACS EST Eng, 2021, 2, 110–120 CrossRef.
- A. L. Andrady, P. W. Barnes, J. F. Bornman, T. Gouin, S. Madronich, C. White, R. G. Zepp and M. A. Jansen, Sci. Total Environ., 2022, 851, 158022 CrossRef CAS.
- A. Sarno, K. Olafsen, S. Kubowicz, F. Karimov, S. T. Sait, L. Sørensen and A. M. Booth, Environ. Sci. Technol. Lett., 2020, 8, 250–255 CrossRef.
- C. Shi, H. Wu, W. Wang, J. Zhao, F. Niu and J. Geng, J. Cleaner Prod., 2024, 434, 140111 CrossRef CAS.
- H. Jeon, J. Yoon and J. Han, Sep. Purif. Technol., 2025, 354, 129113 CrossRef CAS.
- H. Zhao, F. Song, H. Zhou and P. Ji, Environ. Pollut., 2024, 361, 124854 CrossRef CAS PubMed.
- S. Ye, M. Cheng, G. Zeng, X. Tan, H. Wu, J. Liang, M. Shen, B. Song, J. Liu, H. Yang and Y. Zhang, Water Res., 2020, 179, 115876 CrossRef CAS.
- J. Grbic, B. Nguyen, E. Guo, J. B. You, D. Sinton and C. M. Rochman, Environ. Sci. Technol. Lett., 2019, 6, 68–72 CrossRef CAS.
- F. Rhein, H. Nirschl and R. Kaegi, Water Res. X, 2022, 17, 100155 CrossRef CAS PubMed.
- Y. Cao, C. Sathish, X. Guan, S. Wang, T. Palanisami, A. Vinu and J. Yi, J. Hazard. Mater., 2024, 461, 132537 CrossRef CAS PubMed.
- S. Vohl, M. Kristl and J. Stergar, Nanomaterials, 2024, 14, 1179 CrossRef CAS PubMed.
- G. Ji, Y. Xing and T. You, J. Environ. Chem. Eng., 2024, 113377 CrossRef CAS.
- R. Yan, S. Lin, W. Jiang, X. Yu, L. Zhang, W. Zhao and Q. Sui, Sci. Total Environ, 2023, 898, 165431 CrossRef CAS PubMed.
- F. Rhein, F. Scholl and H. Nirschl, Chem. Eng. Sci., 2019, 207, 1278–1287 CrossRef CAS.
- A. Gallo Cordova, B. Corrales Pérez, P. Cabrero, C. Force, S. Veintemillas Verdaguer, J. G. Ovejero and M. Del Puerto Morales, Chem. Eng. J., 2024, 490, 151725 CrossRef CAS.
- Y. Zhang, J. Zhao, Z. Liu, S. Tian, J. Lu, R. Mu and H. Yuan, J. Water Process Eng., 2021, 43, 102250 CrossRef.
- F. Rhein, H. Nirschl and R. Kaegi, Water Res. X, 2022, 17, 100155 CrossRef CAS PubMed.
- C. Shi, S. Zhang, J. Zhao, J. Ma, H. Wu, H. Sun, S. Cheng and P. Technology, Sep. Purif. Technol., 2022, 288, 120564 CrossRef CAS.
- S. Zhang, C. Shi, Y. Nie, B. Xing, X. Wen and S. Cheng, J. Water Process Eng., 2023, 51, 103495 CrossRef.
- C. Shi, H. Wu, W. Wang, J. Zhao, F. Niu and J. Geng, J. Cleaner Prod., 2024, 434, 140111 CrossRef CAS.
- F. Pasanen, R. O. Fuller and F. Maya, Chem. Eng. J., 2023, 455, 140405 CrossRef CAS.
- S. J. Ramage, E. Pagaling, R. K. Haghi, L. A. Dawson, K. Yates, R. Prabhu, S. Hillier and S. Devalla, Sci. Total Environ., 2022, 831, 154912 CrossRef CAS PubMed.
- J. Li, X. Chen, S. Yu and M. Cui, Sci. Total Environ., 2023, 875, 162647 CrossRef CAS PubMed.
- H. P. Wang, X. H. Huang, J. N. Chen, M. Dong, C. Z. Nie and L. Qin, Chem. Eng. J., 2023, 476, 146562 CrossRef CAS.
- J. Li and X. Chen, J. Environ. Chem. Eng., 2024, 12, 113067 CrossRef CAS.
- P. Y. Toh, S. P. Yeap, L. P. Kong, B. W. Ng, D. Chan, A. L. Ahmad and J. K. Lim, Chem. Eng. J., 2012, 211–212, 22–30 CrossRef CAS.
- C. He, K. Fang, H. Gong, J. Liu, X. Song, R. Liang, Q. He, Q. Yuan and K. Wang, Water Res., 2022, 217, 118449 CrossRef CAS PubMed.
- N. Mpongwana and S. Rathilal, Water, 2022, 14, 1550 CrossRef CAS.
- S. Vohl, M. Kristl and J. Stergar, Nanomaterials, 2024, 14, 1179 CrossRef CAS PubMed.
- P. Farinha, J. M. P. Coelho, C. P. Reis and M. M. Gaspar, Nanomaterials, 2021, 11, 3432 CrossRef CAS PubMed.
- J. Kudr, Y. Haddad, L. Richtera, Z. Heger, M. Cernak, V. Adam and O. Zitka, Nanomaterials, 2017, 7, 243 CrossRef PubMed.
- Y. Cao, C. Sathish, Z. Li, M. I. Ahmed, V. Perumalsamy, C. Cao, C. Yu, B. Wijerathne, A. Fleming and L. Qiao, Chem. Eng. J., 2024, 497, 154610 CrossRef CAS.
- R. Yan, S. Lin, W. Jiang, X. Yu, L. Zhang, W. Zhao and Q. Sui, Sci. Total Environ., 2023, 898, 165431 CrossRef CAS PubMed.
- X. Zhang, Y. Zhai, Z. Wang, X. Liu, X. Liu, Y. Zhou, G. Liu, M. Xu and P. Technology, Sep. Purif. Technol., 2023, 322, 124245 CrossRef CAS.
- J. Choi, H. Kim, Y. R. Ahn, M. Kim, S. Yu, N. Kim, S. Y. Lim, J. A. Park, S. Ha and K. S. Lim, RSC Adv., 2024, 14, 9943–9966 RSC.
- B. J. Ni, Z. R. Zhu, W. H. Li, X. Yan, W. Wei, Q. Xu, Z. Xia, X. Dai and J. Sun, Environ. Sci. Technol. Lett., 2020, 7, 961–967 CrossRef CAS.
- M. Sun, W. Chen, X. Fan, C. Tian, L. Sun and H. Xie, Appl. Mater. Today, 2020, 20, 100682 CrossRef.
- A. V. Chesnitskiy, A. E. Gayduk, V. A. Seleznev and V. Y. Prinz, Materials, 2022, 15, 7781 CrossRef CAS PubMed.
- X. Wu, X. Peng, L. Ren, J. Guan and M. Pumera, Adv. Funct. Mater., 2024, 2410167 CrossRef CAS.
- K. Bente, A. Codutti, F. Bachmann and D. Faivre, Small, 2018, 14, 1704374 CrossRef PubMed.
- X. Peng, M. Urso, M. Kolackova, D. Huska and M. Pumera, Adv. Funct. Mater., 2024, 34, 2307477 CrossRef CAS.
- G. Qi, J. Liu, C. Tian and S. Zhang, Chem. Eng. J., 2024, 156216 CrossRef CAS.
- K. Qiao, W. Tian, J. Bai, L. Wang, J. Zhao, T. Song and M. Chu, Mar. Pollut. Bull., 2020, 159, 111489 CrossRef CAS PubMed.
- Q. Lu, K. Choi, J. D. Nam and H. Choi, Polymers, 2021, 13, 512 CrossRef CAS PubMed.
- M. S. Cho, S. T. Lim, I. B. Jang, H. J. Choi and M. S. Jhon, IEEE Trans. Magn., 2004, 40, 3036–3038 CrossRef CAS.
- J. Choi, S. Han, H. Kim, E. H. Sohn, H. J. Choi and Y. Seo, ACS Appl. Nano Mater., 2019, 2, 6939–6947 CrossRef CAS.
- E. A. López Maldonado, N. A. Khan, S. Singh, P. C. Ramamurthy, B. Kabak, J. R. V. Baudrit, M. Q. A. Silvia, R. Varshney, E. Serra-Pérez and J. García, Desalin. Water Treat., 2024, 100198 CrossRef.
- H. Gaß, T. M. Kloos, A. Höfling, L. Müller, L. Rockmann, D. W. Schubert and M. Halik, Small, 2024, 20, 2305467 CrossRef PubMed.
- F. Pasanen, R. O. Fuller and F. Maya, Chem. Eng. J., 2024, 490, 151453 CrossRef CAS.
- Y. K. Song, S. H. Hong, M. Jang, G. M. Han, S. W. Jung and W. Shim, Environ. Sci. Technol., 2017, 51, 4368–4376 CrossRef CAS PubMed.
- J. Duan, Y. Li, J. Gao, R. Cao, E. Shang and W. Zhang, Water Res., 2022, 216, 118320 CrossRef CAS PubMed.
- Z. Cai, M. Li, Z. Zhu, X. Wang, Y. Huang, T. Li, H. Gong and M. Yan, Microorganisms, 2023, 11, 1661 CrossRef CAS PubMed.
- S. Oberbeckmann and M. Labrenz, Ann. Rev. Mar. Sci., 2020, 12, 209–232 CrossRef PubMed.
- A. R. Othman, H. A. Hasan, M. H. Muhamad, N. I. Ismail and S. R. S. Abdullah, Environ. Chem. Lett., 2021, 19, 3057–3073 CrossRef CAS.
- M. Zandieh, E. Griffiths, A. Waldie, S. Li, J. Honek, F. Rezanezhad, P. Van Cappellen and J. Liu, Exploration, 2024, 4, 20230018 CrossRef CAS PubMed.
- S. Q. Chen, Q. S. Huang, Y. Li, J. Wu, S. Chen and Z. F. Yan, J. Hazard. Mater., 2024, 480, 135895 CrossRef CAS PubMed.
- J. Yuan, J. Ma, Y. Sun, T. Zhou, Y. Zhao and F. Yu, Sci. Total Environ., 2020, 715, 136968 CrossRef CAS PubMed.
- J. Zhang, D. Gao, Q. Li, Y. Zhao, L. Li, H. Lin, Q. Bi and Y. Zhao, Sci. Total Environ., 2020, 704, 135931 CrossRef CAS PubMed.
- Z. Cai, M. Li, Z. Zhu, X. Wang, Y. Huang, T. Li, H. Gong and M. Yan, Microorganisms, 2023, 11, 1661 CrossRef CAS PubMed.
- R. J. Müller, H. Schrader, J. Profe, K. Dresler and W. D. Deckwer, Macromol. Rapid Commun., 2005, 26, 1400–1405 CrossRef.
- M. Zandieh and J. Liu, Angew. Chem., 2022, 134, e202212013 CrossRef.
- H. Zhou, C. C. Mayorga Martinez and M. Pumera, Small Methods, 2021, 5, 2100230 CrossRef CAS PubMed.
- P. Xiang, T. Zhang, Q. Wu and Q. Li, Sustainability, 2023, 15, 12698 CrossRef CAS.
- H. Du, Y. Xie and J. Wang, J. Hazard. Mater., 2021, 418, 126377 CrossRef CAS PubMed.
- Y. He, A. U. Rehman, M. Xu, C. A. Not, A. M. Ng and A. B. Djurišić, Heliyon, 2023, 9 Search PubMed.
- J. Kang, L. Zhou, X. Duan, H. Sun, Z. Ao and S. Wang, Matter, 2019, 1, 745–758 CrossRef CAS.
- W. Hu, D. Liu, S. Su, L. Ren, H. Ren, L. Wei, S. Yue, Q. Xie, Z. Zhang, Z. Wang, N. Yang, L. Wu, J. Deng, Y. Qi and P. Fu, Adv. Sustain. Syst., 2021, 5, 2100027 CrossRef CAS.
- T. J. Suhrhoff and B. M. Scholz Böttcher, Mar. Pollut. Bull., 2016, 102, 84–94 CrossRef CAS PubMed.
- L. P. Domínguez Jaimes, E. I. Cedillo González, E. Luévano-Hipólito, J. D. Acuña-Bedoya and J. Hernández-López, J. Hazard. Mater., 2021, 413, 125452 CrossRef PubMed.
- D. Ortiz, M. Munoz, J. Carbajo, Z. De Pedro and J. Casas, J. Environ. Chem. Eng., 2023, 5, 110755 Search PubMed.
- Y. Nosaka and A. Y. Nosaka, Chem. Rev., 2017, 117, 11302–11336 CrossRef CAS PubMed.
- M. Surana, D. S. Pattanayak, V. Yadav, V. Singh and D. Pal, Environ. Res., 2024, 247, 118268 CrossRef CAS PubMed.
- W. Li, W. Zhao, H. Zhu, Z. J. Li and W. Wang, J. Mater. Chem. A, 2023, 11, 2503–2527 RSC.
- A. Uheida, H. G. Mejía, M. Abdel Rehim, W. Hamd and J. Dutta, J. Hazard. Mater., 2021, 406, 124299 CrossRef CAS PubMed.
- S. Cho, W. Choi and P. A. Chemistry, J. Photochem. Photobiol., A, 2001, 143, 221–228 CrossRef CAS.
- S. Jiang, M. Yin, H. Ren, Y. Qin, W. Wang, Q. Wang and X. Li, Polymers, 2023, 15, 2347 CrossRef CAS PubMed.
- B. E. Llorente García, J. M. Hernández López, A. A. Zaldívar Cadena, C. Siligardi and E. I. Cedillo-González, Coatings, 2020, 10, 658 CrossRef.
- I. Nabi, A. U. R. Bacha, K. Li, H. Cheng, T. Wang, Y. Liu, S. Ajmal, Y. Yang, Y. Feng and L. Zhang, iScience, 2020, 23, 101326 CrossRef CAS PubMed.
- C. Yang, K. Deng, T. Peng and L. Zan, Chem. Eng. Technol., 2011, 34, 886–892 CrossRef CAS.
- M. H. Fadli, M. Ibadurrohman and S. Slamet, IOP Conf. Ser.: Mater. Sci. Eng., 2021, 012055 CAS.
- G. Liu, D. Zhu, W. Zhou, S. Liao, J. Cui, K. Wu and D. Hamilton, Appl. Surf. Sci., 2010, 256, 2546–2551 CrossRef CAS.
- W. Fa, L. Zan, C. Gong, J. Zhong and K. Deng, Appl. Catal., B, 2008, 79, 216–223 CrossRef CAS.
- R. Jiang, G. Lu, Z. Yan, J. Liu, D. Wu and Y. Wang, J. Hazard. Mater., 2021, 405, 124247 CrossRef CAS PubMed.
- J. Jeyaraj, V. Baskaralingam, T. Stalin and I. Muthuvel, Environ. Res., 2023, 233, 116366 CrossRef CAS PubMed.
- J. Lu, R. Hou, Y. Wang, L. Zhou and Y. Yuan, Water Res., 2022, 226, 119277 CrossRef CAS PubMed.
- F. Miao, Y. Liu, M. Gao, X. Yu, P. Xiao, M. Wang, S. Wang and X. M. Wang, J. Hazard. Mater., 2020, 399, 123023 CrossRef CAS PubMed.
- Z. Ning, X. Duan, Y. Li, X. Zhao and L. Chang, J. Cleaner Prod., 2023, 432, 139668 CrossRef CAS.
- J. Lu, R. Hou, Y. Wang, L. Zhou and Y. Yuan, Water Res., 2022, 226, 119277 CrossRef CAS PubMed.
Footnote |
† Current address: Department of Chemical Engineering, Harcourt Butler Technical University Kanpur, 208002, India. |
|
This journal is © The Royal Society of Chemistry 2025 |
Click here to see how this site uses Cookies. View our privacy policy here.