DOI:
10.1039/D4MH01343C
(Communication)
Mater. Horiz., 2025,
12, 293-302
Fine-tuning the molecular conformation and packing structures of coumarin-based luminogens to achieve distinct piezochromic properties upon mechanical grinding and under hydrostatic pressures†
Received
27th September 2024
, Accepted 21st October 2024
First published on 29th October 2024
Abstract
Organic luminogens (OLs) exhibiting piezochromic (PC) properties have drawn much attention owing to their great application potentials. Both mechanical grinding (MG) and hydrostatic pressures (HP) can induce PC behaviors of OLs, and it is highly desirable to combine the two strategies to study the PC properties of OLs for comprehensively exploring their application scopes and deeply understanding the intrinsic PC mechanisms. In this work, four coumarin derivatives with different substituents at 3- or 4-positions are designed and synthesized to investigate their PC properties by MG and under HP. By MG, two materials show PL shifts, and the PL of the other two molecules barely change. In contrast, under HP, these molecules all exhibit PL shifts, but with different pressure coefficients. In addition, they show different reversibility of PL change after releasing HP. The different molecular conformation and packing structure changing manners of the materials, indicated by single-crystal and powder X-ray diffraction patterns, and in situ PL lifetime analysis, are anticipated to induce distinct PC behaviors upon disparate force stimulus. Our study indicates that fine-tuning the functionalization position of coumarin derivatives is a powerful strategy to engineer their molecular conformation and packing structures, thus developing versatile pressure-responsive OLs.
New concepts
This work reports a new molecular designing strategy in coumarin-based luminogens to achieve distinct piezochromic properties using different pressure inducing methods. Whereas most previous studies regarding coumarin based piezochromic luminogens focused on 3-position functionalization, in this work new coumarin derivatives are designed to attach various substituents at 3- and especially 4-positions, which realizes systematic fine-tuning in their molecular conformations and packing structures. These materials have diverse piezochromic properties, i.e., either show different degrees of permanent photoluminescence changes upon mechanical grinding, or exhibit instant photoluminescence shifts under hydrostatic pressures with disparate reversibility after pressure release. The distinct piezochromic behaviours may originate from different molecular and packing structure changing manners under pressure, indicated by multiple characterization means. Our study reveals that functionalization of the coumarin molecular backbone at 3- and 4-positions is an effective strategy to adjust their piezochromic properties. Furthermore, in this work, only simply modified phenyl groups are introduced as substituents to the coumarin backbone; considering the abundant options of potential functionalization groups, the position engineering of coumarins is anticipated to have many possibilities in developing versatile piezochromic organic luminogens. Therefore, this concept can provide favorable guidance for future rational design and development of piezochromic materials.
|
Introduction
Organic luminogens (OLs) that exhibit colour or luminescence changes upon external force stimulation, known as organic piezochromic (PC) materials, have attracted much research interest due to their potential applications in pressure sensors, data storage, optoelectronic devices, etc.1–3 Mechanical grinding (MG), applying anisotropic force to the materials, is a straightforward strategy to realize the PC effect, which can be separated into a normal pressure and deviatoric stress.4 In addition, friction accompanied by grinding can generate heat, thus, resulting in a thermal effect. Therefore, in many cases, the PC behaviours induced by MG can be a synergetic consequence of the coexisting pressure effect, deviatoric stress, and thermal effect, making it difficult to fully understand the PC mechanism and rational design of novel materials.5,6 On the other hand, introducing hydrostatic pressures (HP) is another efficient strategy to determine the PC response of materials, which can be realized through diamond anvil cells (DAC).7,8 In this way the applied HP is in situ and isotropic, effectively avoiding the deviatoric stress and thermal effect. Combining the different force and pressure applying modes to investigate the PC properties of OLs will be beneficial for comprehensively studying their application potentials under various application scopes, and deeply understanding the intrinsic origin of their pressure-responsive behaviours. Nevertheless, the relative studies have been only marginally explored due to the strict operating conditions of DAC and lack of suitable molecular model systems.9,10
To date, many series of OLs with PC properties have been developed, including cyanostyrene and cyanostilbene derivatives,11–14 tetraphenylethylene functionalized materials,15–17 triphenylamine-based donor–acceptor fluorophors,18–20etc., most of which are studied under MG. Recently, the PC properties of a few anthracene derivatives were investigated under HP, and by means of this strategy their PC mechanisms can be well elucidated.21,22 Coumarin and its derivatives have been extensively studied as dyes for colouring textile fiber, photosensitizers, fluorescent probes in bioimaging, etc., because of their stable fluorescence emission and structural flexibility.23–25 Lately, several coumarin derivatives with functionalization at the 3-position have been reported to show obvious PC properties, suggesting their promising application potential as pressure-responsive materials.26,27 However, in these studies only the MG strategy is studied, and in particular, the position effect of functionalization to the PC properties has not been illustrated.
In this work, four coumarin derivatives, 3-CPOMe, 3-CPCN, 4-CPOMe, and 4-CPCN, with 7-(diethylamino)coumarin as the molecular backbone were designed and synthesized, to which different substituents at different positions (3- or 4-positions) were attached, and their PC properties upon MG and under HP were explored. The photophysical properties of these compounds in dilute solutions and the aggregated state were studied using UV-Vis absorption spectra and photoluminescence (PL) spectra, and the aggregation effect to their PL emissions was discussed. The four molecules showed different molecular conformation and packing structures, revealed by single-crystal X-ray diffraction (SC-XRD) analysis, which significantly affected their pressure-responsive properties. By MG, 3-CPOMe showed a slight blue-shift, while 4-CPCN had a large red-shift in PL. On the other hand, under HP, these molecules all exhibited considerable PL red-shifts, but with different pressure coefficients of PL wavelength. Furthermore, they presented different degrees of reversibility of PL change after releasing HP. The distinct PC behaviours were speculated to originate from different molecular and packing structure changing manners, indicated by the powder X-ray diffraction (PXRD) patterns of the aggregates before and after grinding, and the in situ PL lifetime analysis under different HP. Our study not only suggests that coumarin derivatives can be versatilely applied as PC materials, but also implies that fine-tuning the functionalization position of the coumarin molecular backbone can be a powerful strategy to engineer their molecular conformation and packing structures, thus, develop various pressure-responsive OLs depending on the application scope and pressure-inducing manner, i.e., by MG or under HP.
Results
Synthesis of the target molecules
Scheme 1 shows the synthetic route of the target molecules. The 3-position functionalized 7-(diethylamino)coumarin derivatives with para-methoxylphenyl (3-CPOMe) or para-cyanophenyl (3-CPCN) substituents were synthesized through a one-pot reaction from 4-(diethylamino)salicylaldehyde (1) and para-modified benzylcyanides (2) following a literature reported method.28,29 To synthesize the 4-position functionalized 7-(diethylamino)coumarin derivatives, compound 5 was obtained from the reaction between 3-(diethylamino)phenol (3) and bis-2,4,6-trichlorophenol malonate (4) in the first place, then it was converted into triflate (6).30 The final target molecules were synthesized by the Suzuki coupling reaction between 6 and para-methoxylphenylboronic acid (for 4-CPOMe), or para-cyanophenylboronic acid (for 4-CPCN), respectively. The detailed synthetic procedures and characterization of the target compounds can be found in the ESI.†
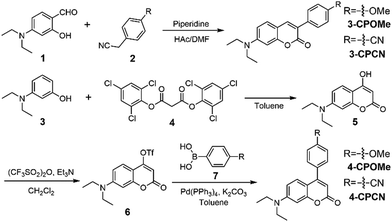 |
| Scheme 1 Synthetic route of the coumarin derivatives. | |
Photophysical properties of the target molecules in dilute solution and the aggregated state
Fig. 1 shows the UV-vis absorption and PL spectra of the four coumarin derivatives in solution and the aggregated state. In THF solution, the four molecules exhibit one main absorption band in ∼350–450 nm in the UV-vis absorption spectra, with the maxima (λabs) at 393 nm for 3-CPOMe, 377 nm for 4-CPOMe, 413 nm for 3-CPCN, and 387 nm for 4-CPCN, respectively. In the PL spectra, one emission band can be observed for the four molecules with the maxima (λPL) at 467 nm for 3-CPOMe, 459 nm for 4-CPOMe, 482 nm for 3-CPCN, and 547 nm for 4-CPCN, respectively. The solvatochromic effects of the four molecules are investigated in different organic solvents. As shown in Fig. S1 (ESI†), the λPL gradually red-shift with the increase of solvent polarity, indicating that the emission peaks possibly originate from the intramolecular charge transfer (ICT) process. The 4-position functionalized molecules generally have blue-shifted absorption and PL spectra, even though the PL spectra of 4-CPCN is red-shifted. On the other hand, the λabs and λPL are red-shifted in the cyanophenyl functionalized molecules compared with the methoxylphenyl modified molecules. These results elucidate that the cyanophenyl functionalization can slightly enhance the ICT process of the molecules in the excited state, which is more significant if the substituents are at the 3-position.
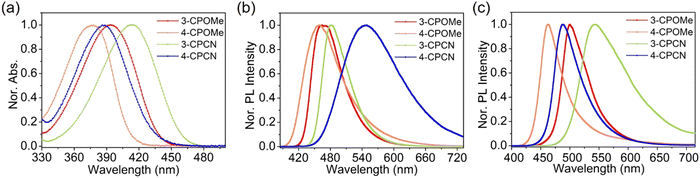 |
| Fig. 1 (a) Normalized UV-Vis absorption spectra of the four molecules in diluted THF solution, and normalized PL spectra of the four molecules in diluted THF solution ((b) 375 nm excitation) and the aggregated state ((c) 365 nm excitation). | |
In the aggregated state, the λPL of 3-CPOMe is 499 nm, which is ∼32 nm red shifted. In contrast, the PL spectra of 4-CPOMe is quite similar with that in solution, whose λPL is at 463 nm. The PL of 3-CPCN is red-shifted as well (λPL = 542 nm), showing a similar trend to 3-CPOMe, but regarding 4-CPCN it is blue-shifted by 59 nm (λPL = 488 nm). These results indicate that aggregation has a similar effect on the emission properties of 3-position substituted molecules 3-CPOMe and 3-CPCN, possibly resulting in J-aggregates, but should be different to the 4-position functionalized counterparts, which might form H-aggregates.31,32
The quantum yields (Φ) of 3-CPOMe and 3-CPCN are 0.81 and 0.84 in THF, respectively. Upon aggregation, the Φ of the two materials both decrease (from 0.81 to 0.34 for 3-CPOMe, and from 0.84 to 0.13 for 3-CPCN). In contrast, the Φ of the 4-position substituted molecules are relatively lower in diluted solution, but enhanced in the aggregated state (from 0.17 to 0.20 for 4-CPOMe, and from 0.18 to 0.40 for 4-CPCN, respectively). The different changing trend of Φ from solution to the aggregated state further supports the fact that functionalization at 3- or 4-positons of the coumarin moiety has distinct aggregation effects to the photophysical properties of the materials.
The aggregation-induced emission (AIE) properties of the four materials have been studied in their THF/H2O mixed solutions. As shown in Fig. S2 (ESI†), as the H2O fractions increase from 0 to ∼70%, the strong PL emissions of 3-position substituted molecules can be basically maintained. A further increase in H2O fractions induces the decrease in the PL intensities, consistent with their decreased Φ from diluted solutions to the aggregated states. On the other hand, the PL intensities of 4-CPOMe are enhanced firstly by addition of 10% H2O, then gradually decrease. The PL intensity in 99% H2O fraction solution is close to that in the initial pure THF solution, which can be understood by their similar Φ values. With the addition of H2O, the PL intensities of 4-CPCN always decrease, reaching the lowest value as the H2O fraction is 90%. The increase in PL intensity can be observed as the H2O fraction is further increased to 99%, exhibiting an AIE feature. This is in accordance with its increased Φ from solution to the aggregated state (Table 1).
Table 1 Summarized UV-vis absorption and PL peaks, quantum yields of the four molecules in solution and the aggregated state
Molecules |
λ
abs
(nm) |
λ
PL
(nm) |
λ
PL
(nm) |
ϕ
|
ϕ
|
The photophysical properties in dilute solution.
The photophysical properties in the aggregated state.
|
3-CPOMe
|
393 |
467 |
499 |
0.81 |
0.34 |
4-CPOMe
|
377 |
459 |
463 |
0.17 |
0.20 |
3-CPCN
|
413 |
482 |
542 |
0.84 |
0.13 |
4-CPCN
|
387 |
547 |
488 |
0.18 |
0.40 |
Theoretical calculations of frontier molecular orbitals
The frontier molecular orbitals and energy levels of the four molecules in the gas phase are studied by theoretical calculations based on density functional theory (DFT) at the B3LYP/6-31G** level. As shown in Fig. 2, the HOMO coefficients of the four molecules are basically distributed on the 7-(diethylamino)coumarin moiety, which have certain distribution on the methoxylphenyl group of 3-CPOMe and cyanophenyl group of 3-CPCN as well. On the other hand, the LUMO coefficients are heavily located at the inner ester part of the 7-(diethylamino)coumarin moiety, and they are partially distributed on the cyanophenyl groups of 3-CPCN and 4-CPCN, which can be easily understood by its electron withdrawing nature. The relatively separate distribution of the HOMO and LUMO coefficients of the four molecules indicates the typical ICT characteristic of the HOMO–LUMO transition, which mainly occurs at the 7-(diethylamino)coumarin moiety, in correspondence to their photophysical properties.
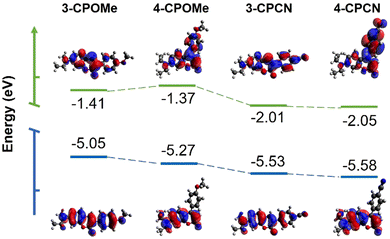 |
| Fig. 2 Frontier molecular orbitals and energy levels of the four molecules. | |
Table 2 summarizes the theoretical and experimental frontier molecular energy levels and bandgaps of the four molecules. Basically, based on theoretical calculation, 4-position substituted molecules have lower HOMO energy levels and larger bandgaps than the 3-position functionalized ones, suggesting the relatively interrupted conjugation of the whole molecular backbones, explaining their relatively blue-shifted UV-vis absorption and PL spectra. Introduction of a cyano group instead of the methoxyl group could lower both the HOMOs and LUMOs of the molecules, yielding a relatively narrower bandgap. Therefore, cyanophenyl group substituted molecules have relatively red-shifted UV-vis absorption and PL spectra compared with the methoxylphenyl group modified molecules in diluted solution. The experimental HOMO levels of the molecules were determined by cyclic voltammetry (CV, Fig. S3, ESI†), and the LUMO levels were calculated based on the HOMOs and the optical bandgaps, which show similar trends with the theoretical results.
Table 2 Summarized frontier molecular energy levels and bandgaps of the four molecules. The upper are the theoretical values, and the bottom are the experimental values derived from CV measurements and optical bandgaps
Molecules |
HOMO (eV) |
LUMO (eV) |
Bandgap (eV) |
3-CPOMe
|
−5.05 |
−1.41 |
3.64 |
−5.08 |
−2.26 |
2.82 |
4-CPOMe
|
−5.27 |
−1.37 |
3.90 |
−5.00 |
−1.96 |
3.04 |
3-CPCN
|
−5.53 |
−2.01 |
3.52 |
−5.30 |
−2.60 |
2.70 |
4-CPCN
|
−5.58 |
−2.05 |
3.53 |
−5.42 |
−2.57 |
2.85 |
Piezochromic properties induced by mechanical grinding
The PC properties of the materials were firstly evaluated by grinding the powder in agate mortars. As shown in Fig. 3, after grinding, the λPL of 3-CPOMe exhibits a slight blue-shift (4 nm) from 499 nm to 495 nm. However, those of 4-CPOMe and 3-CPCN have no obvious changes. In sharp contrast, the PL spectra of 4-CPCN red-shift apparently by up to 43 nm (λPL shifts from 488 nm to 531 nm) upon grinding, which exhibits the most significant PC properties among the four molecules. After being treated with DCM vapour, the PL spectra of the ground powder of the four molecules are able to basically recover back to their original states, indicating that the PC behaviours are not derived from permanent chemical structure changes of molecules during grinding. The inserted photos show the PL changes of the powder samples before (or after vapour treatment) and after grinding under 365 nm light excitation, in which obvious fluorescence change from light-green to yellow-green of 4-CPCN can be straightforwardly observed. Table 3 summarizes the shifts of PL peaks before and after grinding, and after vapour treatment.
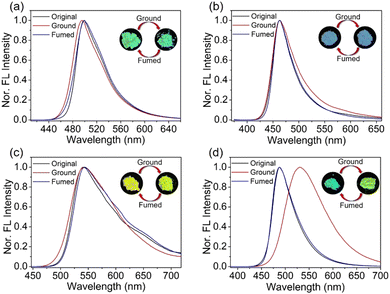 |
| Fig. 3 Piezochromic properties of 3-CPOMe (a), 4-CPOMe (b), 3-CPCN (c), and 4-CPCN (d) before grinding, upon grinding and after vapour treatment. | |
Table 3 Summarized PL shifts of the four materials before and after grinding, and after vapour treatment
Molecules |
λ
PL (original, nm) |
λ
PL (ground, nm) |
λ
PL (recover, nm) |
3-CPOMe
|
499 |
495 |
499 |
4-CPOMe
|
463 |
463 |
463 |
3-CPCN
|
542 |
542 |
542 |
4-CPCN
|
488 |
531 |
488 |
Piezochromic properties induced by hydrostatic pressures
The PC properties of the materials under HP were studied by choosing the microcrystals as the samples (Fig. 4). It should be noted that in the HP PL measurements, the excitation light is a 365 nm LED light, thus, the PL spectra could be slightly different with those measured in the photophysical and MG PL experiments.
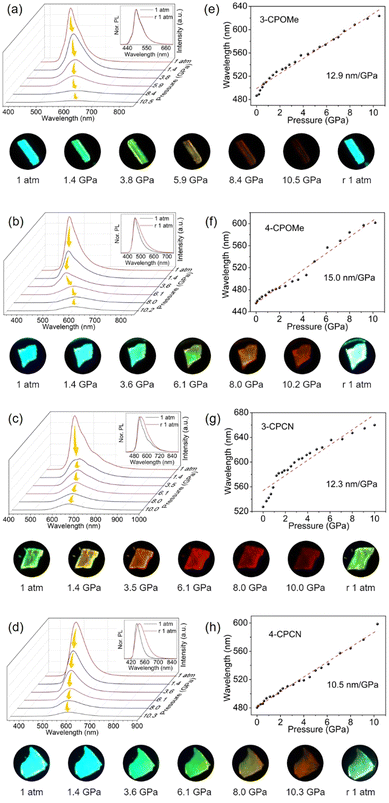 |
| Fig. 4 (a)–(d) The PL spectra of the four molecules upon different hydrostatic pressures, and below are the corresponding photos; (e)–(h) the linear fitting of the pressure-dependent λPL, and inset is the obtained pressure coefficients of emission wavelengths. | |
Generally, as the pressure increases, the λPL of the 4 materials all red shift, and the PL intensities decrease gradually, indicating typical PC behaviours. The pressure coefficients of emission wavelengths are 12.9 nm GPa−1 for 3-CPOMe, 15.0 nm GPa−1 for 4-CPOMe, 12.3 nm GPa−1 for 3-CPCN, and 10.5 nm GPa−1 for 4-CPCN, respectively. Interestingly, the 3-position modified molecules show similar pressure coefficients, while those of 4-position substituted molecules are largely different.
After release of pressure to the ambient conditions, the emission spectra of 3-CPOMe almost fully recovers back to the original state, similar to the grinding experiments. Those of 4-CPOMe and 3-CPCN become broader, and the λPL are slightly red shifted (4 nm for 4-CPOMe, from 455 nm to 459 nm, 14 nm for 3-CPCN, from 527 nm to 541 nm), which are different with the MG experiment. The most obvious red shift of 25 nm (from 481 nm to 506 nm) occurs for 4-CPCN, although it is much smaller than the shifts in the grinding experiment. The large permanent red shift of λPL for 4-CPCN suggests that there can be an irreversible structural change of the molecular organization in the aggregates, while that for 4-CPOMe and 3-CPCN, and especially for 3-CPOMe, can be minor. Table 4 summarizes the λPL and the full width at half maximum (FWHM) of the corresponding PL peaks under different HP.
Table 4 Summarized λPL of the PL spectra under different HP (numbers in the brackets are the FWHM of the corresponding PL peaks)
Molecules |
1 atm (nm) |
∼1.4 GPa (nm) |
∼3.6 GPa (nm) |
∼6.0 GPa (nm) |
∼8.0 GPa (nm) |
∼10.0 GPa (nm) |
R1 atm (nm) |
3-CPOMe
|
487 (56) |
522 (69) |
556 (87) |
574 (99) |
608 (122) |
624 (46) |
487 (56) |
4-CPOMe
|
455 (55) |
479 (70) |
498 (86) |
557 (110) |
584 (116) |
602 (115) |
459 (73) |
3-CPCN
|
527 (78) |
583 (126) |
611 (117) |
636 (113) |
647 (115) |
660 (119) |
541 (102) |
4-CPCN
|
481 (72) |
498 (73) |
518 (76) |
542 (92) |
564 (101) |
599 (116) |
506 (121) |
Discussion
Single crystal structure analysis
The single crystals (CCDC 2368567, 2368568, 2368569, 2368805†) suitable for SC-XRD analysis of the four molecules were obtained by slowly evaporating their DCM/EtOH mixed solution. As shown in Fig. 5, consistent with the DFT calculation results, the 3-position substituted molecules 3-CPOMe and 3-CPCN show a linear shaped molecular configuration with a slight twist between the coumarin moiety and the phenyl unit, with torsion angles of 27.7° for 3-CPOMe, and 7.5° for 3-CPCN, respectively. In the aggregates the molecules show “head-to-tail” dimer like stacking, with only small offsets along both the long molecular axis and short molecular axis. The π–π distance is 3.47 Å for 3-CPOMe, but 3.33 Å for 3-CPCN within the dimers, suggesting the relatively strong intermolecular interactions. Between neighbouring dimers, 3-CPOMe and 3-CPCN exhibit a “column-like” packing structure, in which the neighbouring dimer molecules of 3-CPOMe are inclined with each other, but those of 3-CPCN are in parallel. In 3-CPOMe, intermolecular short contacts exist both between the inclined dimers and the neighbouring columns, while those are only observed between neighbouring columns in 3-CPCN.
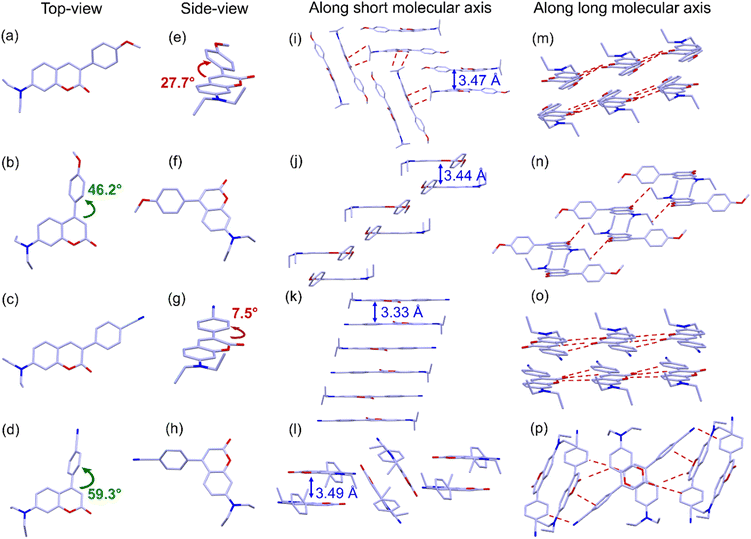 |
| Fig. 5 Molecular conformation of the molecules in the top-view (a)–(d) and side-view (e)–(h); packing structures of the aggregates along the short molecular axis (i)–(l) and long molecular axis (m)–(p) of the four molecules; the intermolecular short contacts are marked by the dashed lines. | |
As the substituents are moved to the 4-position, 4-CPOMe and 4-CPCN exhibit a more twisted molecular conformation, whose torsion angles increase to 46.2° for 4-CPOMe, and 59.3° for 4-CPCN, respectively. As a result, even though 4-CPOMe and 4-CPCN show dimer like stacking between nearest neighbouring molecules, the stacking mainly exists between the 7-(diethylamino)coumarin moieties, and the methoxylphenyl unit or cyanophenyl unit are largely offset. The π–π distances are 3.44 Å for 4-CPOMe and 3.49 Å for 4-CPCN, calculated based on the distance between the 7-(diethylamino)coumarin moieties, suggesting the moderately strong intermolecular interactions. The neighbouring dimers are paralleled with each other for 4-CPOMe, but inclined for 4-CPCN. Even though the neighbouring dimers do not organize exactly in the same way, they both form “layer-by-layer” lamellar packing structures. Between neighbouring dimers 4-CPCN has more intermolecular short contacts than 4-CPOMe. Briefly, fine-tuning the substituent structure and position selectively produces four types of molecular arrangement modes: inclined dimers with column-like packing for 3-CPOMe, paralleled dimers with column-like packing for 3-CPCN, paralleled dimers with lamellar packing for 4-CPOMe, and inclined dimers with lamellar packing for 4-CPCN, respectively.
Since the nearest neighbours of the four materials in the aggregated state all form dimer like organization, their mutual positions are expected to have the largest contributions to their optical properties in aggregates.33 In particular, because the ICT process mainly occurs within the 7-(diethylamino)coumarin moieties, how they are organized within the dimer plays an significant role in determining the aggregation effect to their PL emission. Based on the SC-XRD data, the displacement angles along the long molecular axis of the neighbouring coumarin moieties within the dimer are 44.7° for 3-CPOMe and 41.2° for 3-CPCN, while those are 70.9° for 4-CPOMe and 71.9° for 4-CPCN, respectively. On the basis of the molecular exciton dimer model,34 the relatively smaller displacement angles (smaller than 54.7°) suggest the formation of J-aggregate for 3-CPOMe and 3-CPCN, in accordance with the large red-shifts of the PL emission peaks from solution to the aggregated state. On the other hand, larger displacement angles indicate an H-aggregation effect of the 4-position modified molecules, consistent with their minor red-shift or blue-shift of PL spectra upon aggregation.
Mechanical force induced crystallinity change
The pressure effect and thermal effect derived from MG are often correlated with the crystallinity change and phase transition of materials. To further understand how the MG influences the PL spectra change, the crystalline powder of the four materials before and after grinding, and after vapour fuming with organic solvent were investigated with PXRD (Fig. 6). First of all, all the PXRD patterns of the crystalline powder before grinding are compared with those extracted from the SC-XRD data, which matches well with the simulated patterns. This indicates that the materials have a similar packing structure in crystalline powder as in the single crystals. In addition, the diffraction peaks are sharp and intense, suggesting the good crystallinity.
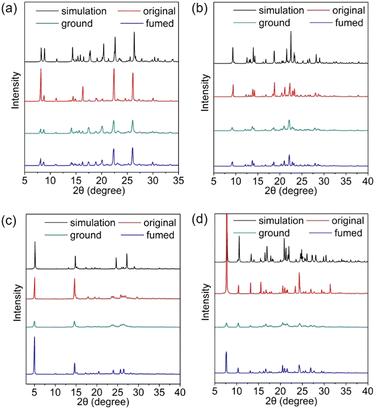 |
| Fig. 6 XRD patterns of 3-CPOMe (a), 4-CPOMe (b), 3-CPCN (c), and 4-CPCN (d) derived from simulation of the SC-XRD (black line), before grinding (red line), after grinding (green line), and after vapour treatment (blue line). | |
After grinding, there are no shifts of the existing diffraction peaks, and no appearance of new diffraction peaks for the four materials, suggesting no crystal-to-crystal phase transition of the powder upon grinding. However, the diffraction intensities of all the powders become weak, implying the decrease in their crystallinity. To 3-CPOMe, the most obvious decrease in peak intensities occurs along the 011 and 022 planes, which are between the inclined dimer columns. Similarly, the 001 and 100 planes in 3-CPCN are the heaviest destroyed crystalline planes, which are between dimer columns as well. In 4-position modified molecules, the most intense and easiest vanished diffraction peaks can be indexed as the 001 and 002 planes for 4-CPOMe, and the 100 plane for 4-CPCN, respectively, which are between the dimer layers. These results indicate that grinding significantly breaks the organizations between neighbouring dimer columns or layers, however, these types of arrangements normally play only minor roles in determining the optical properties of aggregates.12,33 This might explain the basically unchanged PL spectra of 4-CPOMe and 3-CPCN after grinding.
Comparing the PC results induced by MG and HP, under HP, 3-CPOMe does not show an obvious PL change, and the PL shift of 4-CPCN is much less significant than the MG results. It is worth noting that the pressure induced by MG should be much weaker than in the HP experiments. Thus, the PC behaviours of the two materials are probably not only originated from the normal pressure. On the other hand, the PL of 3-CPOMe and 4-CPCN shows no obvious variation on a hotplate with temperature up to 100 °C, suggesting that the thermal effect generated during grinding should not play an important role in the PC behaviour by MG. From another perspective, based on the SC-XRD data, the neighbouring dimers of 3-CPOMe and 4-CPCN both show an incline with each other, indicating that the molecules might be relatively more “loosely packed” in the aggregated state. This kind of “loosely packed” structures are possibly easier to change upon deviatoric stress, thus resulting in the more significant changes in the PL spectra upon grinding.35,36
PL lifetime analysis
The mutual organizational changes of neighbouring molecules in the aggregates play crucial rules in determining their pressure-responsive properties, which can be reflected by PL lifetime analysis to a certain extent, and it is particularly helpful to understand the PC behaviours under HP, as the applied pressures can be quantitatively controlled. Therefore, the PL lifetimes of the four compounds under different HP were studied in situ. As shown in Fig. 7, without any external pressure, the τ of 3-CPOMe is 5.73 ns, and as the HP increases, the τ gradually increase as well, reaching a maximum value of 12.72 ns at 4.0 GPa. The prolonged PL lifetimes, combined with the red shifts of the emission peaks and the weakened PL intensities, imply a more pronounced formation of excimers. The evolution of excimer formation is usually expected to be accompanied with enhanced electronic coupling between neighbouring molecules, thus 3-CPOMe molecules are anticipated to gather together more tightly in this process.37–39 A further increase in external HP induces the decrease of PL lifetime, and at 10 GPa τ (4.70 ns) is even lower than the initial value without applied pressure. At the same time, the emission peaks continue red-shifting. This suggests the more intensively squeezed situation of neighbouring molecules, which is also supported by the receded PL intensities, as the too close neighbouring molecules can induce severe non-radiative decay of the excited states. Thus, increasing the HP to 3-CPOMe aggregates is estimated to keep squeezing the molecules together. The aggregates of 3-CPCN show a similar changing trend of the PL lifetimes with 3-CPOMe at different HP, indicating that the applied pressures probably push the molecules closer as well.
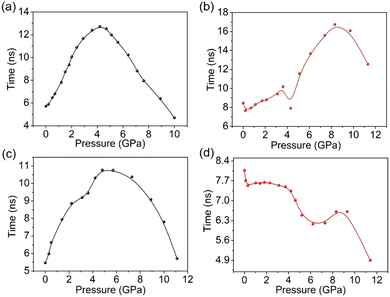 |
| Fig. 7 PL lifetimes of the four molecules under different hydrostatic pressures: (a) 3-CPOMe, (b) 4-CPOMe, (c) 3-CPCN, and (d) 4-CPCN. | |
The PL lifetimes of 4-CPOMe firstly show a slight decrease from 8.47 ns to 7.62 ns as the pressure increases from 1 atm to 0.2 GPa, then only minor changes can be observed even when the pressures reach up to 4.3 GPa. The mild changes in the lifetimes indicate that in the pressure range of 1 atm–4.3 GPa the mutual arrangements of neighbouring molecules should not have significant variations. On the other hand, since the methoxylphenyl unit is connected with the coumarin moiety by a single bond and they are twisted with each other, possibly in this pressure range the planarity of molecular conformation occurs preferably, which can also induce the red-shift of PL emission.40,41 When the pressure further increases, there is an abrupt increase in PL lifetimes, which gets to the maximum value of 16.75 ns at 8.3 GPa, then it gradually decreases and is as low as 12.56 ns at 11.3 GPa. The apparent difference in lifetime changing trend suggests that in the higher pressure range of 4.3–11.3 GPa the molecules in the aggregates should alter in a different way compared to in the low pressure range. Combining the facts that under these pressures the PL emission peaks red shift, and the intensities are weakened a lot, it is reasonable to speculate that molecules are squeezed together to form excimers under this situation. When pressure increases from 1 atm to 0.3 GPa, the lifetimes of 4-CPCN aggregates initially decrease slightly, then basically remain stable at ∼7.5 ns. Thus, in this pressure range the molecules possibly undergo planarity of molecular conformation as well. A further increase in the pressure makes the PL lifetimes drop abruptly, decreasing to 4.90 ns at 11.4 GPa. The obvious difference in the PL change compared to that of 4-CPCN implies that the neighbouring molecules should change in a different manner, which could involve significant permanent structural changes. As a result, after release of the hydrostatic pressures, a red-shift of PL emission by 25 nm can be observed.
It is noteworthy that only 3-CPOMe exhibits almost fully reversible PC behaviours by applying and releasing HP, and certain irreversible PL changes occur for the other three materials. Since under high HP the aggregates should all undergo certain contraction, therefore, slipping of neighbouring molecules, both between dimers and within dimers possibly takes place. The relatively planar molecular configuration and special packing structure with an incline along the long molecular axis of 3-CPOMe might be favorable for hindering the slipping of molecules, conducive to the reversibility of the PC properties. On the other hand, 4-CPCN with a twisted molecular conformation and inclined dimer arrangements exhibit the most significant irreversible PL shifts, suggesting that this type of structural combination is not very sustainable in the presence of external pressures.
Conclusions
In conclusion, four coumarin derivatives with different substituents (electron-donating methoxylphenyl group (3-CPOMe and 4-CPOMe), or electron-withdrawing cyanophenyl group (3-CPCN and 4-CPCN)) at 3- or 4-positions are designed and synthesized. The position of the substituents significantly affects the molecular shape and conformation, in which the 3-position substituted molecules are linear-shaped with a relatively planar conformation, but the 4-position substituted molecules are “L-shaped” with a twisted molecular conformation, supported by the theoretical calculation and SC-XRD analysis. The four materials all form dimer-like organization within their nearest neighbouring molecules. Furthermore, fine-tuning the substituent structure and position selectively arranges the dimers into different manners in the aggregates, which are inclined and column-like packing in 3-CPOMe, paralleled and lamellar packing in 4-CPOMe, paralleled and column-like packing in 3-CPCN, and inclined and lamellar packing in 4-CPCN, respectively. In consequence, they have different PC properties by MG or under HP. Upon grinding, 3-CPOMe and 4-CPCN show PL changes to a different degree, and in this process deviatoric stress plays an important role. Under HP, the twisted molecules (4-CPOMe and 4-CPCN) may mainly undergo conformation planarization in the low pressure range, then are squeezed closer like the planar ones in a high pressure range, except for 4-CPCN, which probably has significant permanent packing structure changes. In particular, the inclined dimer organization in 3-CPOMe favors its recovery of the aggregated structure after HP release, producing the fully reversible PC properties under HP. Our study suggests that coumarin derivatives can be effectively applied as PC materials through both MG and under HP. In addition, functionalization of the coumarin molecular backbone at 3- or 4-positions can selectively engineer the molecular conformation and packing structure in the aggregates, resulting in distinct PC properties depending on different pressure-inducing manners. It is worth noting that in this work the substituents introduced to the coumarin backbone are just simply modified phenyl groups, considering the abundant options of potential functionalization groups, the position engineering of coumarin is supposed to have many possibilities in developing various PC OLs. Therefore, this work could provide favorable guidance for the future rational design and development of PC materials.
Data availability
The data about synthetic details and characterization of the target compounds have been included as part of the ESI.† Crystallographic data for the target compounds have been deposited at the CCDC under 2368567, 2368568, 2368569, and 2368805.†
Conflicts of interest
There are no conflicts to declare.
Acknowledgements
C. W. acknowledges the financial support from the National Natural Science Foundation of China (NSFC, 22205003) and the Start-up Foundation for Talents of Anhui University. J. Y. acknowledges the funding support from the NSFC (51673001). Q. L. and L. L. acknowledge the funding support from the Special Construction Project Fund for Shandong Province Taishan Scholars. H. N. acknowledges the funding support from the NSFC (52172172) and School of Chemistry & Chemical Engineering, Anhui University.
References
- Y. Sagara and T. Kato, Mechanically induced luminescence changes in molecular assemblies, Nat. Chem., 2009, 1, 605–610 CrossRef CAS PubMed
.
- Y. Gong, G. Chen, Q. Peng, W. Z. Yuan, Y. Xie, S. Li, Y. Zhang and B. Z. Tang, Achieving Persistent Room Temperature Phosphorescence and Remarkable Mechanochromism from Pure Organic Luminogens, Adv. Mater., 2015, 27, 6195–6201 CrossRef CAS
.
- K. P. Gan, M. Yoshio and T. Kato, Columnar liquid-crystalline assemblies of X-shaped pyrene–oligothiophene conjugates: photoconductivities and mechanochromic functions, J. Mater. Chem. C, 2016, 4, 5073–5080 RSC
.
- A. Li, S. Xu, C. Bi, Y. Geng, H. Cui and W. Xu, Piezochromic mechanism of organic crystals under hydrostatic pressure, Mater. Chem. Front., 2021, 5, 2588–2606 RSC
.
- Z. Chi, X. Zhang, B. Xu, X. Zhou, C. Ma, Y. Zhang, S. Liu and J. Xu, Recent advances in organic mechanofluorochromic materials, Chem. Soc. Rev., 2012, 41, 3878–3896 RSC
.
- K. Chung, M. S. Kwon, B. M. Leung, A. G. Wong-Foy, M. S. Kim, J. Kim, S. Takayama, J. Gierschner, A. J. Matzger and J. Kim, Shear-Triggered Crystallization and Light Emission of a Thermally Stable Organic Supercooled Liquid, ACS Cent. Sci., 2015, 1, 94–102 CrossRef CAS
.
- R. A. Forman, G. J. Piermarini, J. D. Barnett and S. Block, Pressure Measurement Made by the Utilization of Ruby Sharp-Line Luminescence, Science, 1972, 176, 284–285 CrossRef CAS
.
- J. H. Eggert, K. A. Goettel and I. F. Silvera, Ruby at high pressure. I. Optical line shifts to 156 GPa, Phys. Rev. B: Condens. Matter Mater. Phys., 1989, 40, 5724–5732 CrossRef CAS PubMed
.
- Z. Fu, Z. Yang, X. Yang, K. Wang and B. Zou, Multiple responses of 1,6-diphenyl-1,3,5-hexatriene to mechanical stimulation: emission enhancement, piezochromism and negative linear compressibility, Chem. Sci., 2023, 14, 4817–4823 RSC
.
- K. Nagura, S. Saito, H. Yusa, H. Yamawaki, H. Fujihisa, H. Sato, Y. Shimoikeda and S. Yamaguchi, Distinct Responses to Mechanical Grinding and Hydrostatic Pressure in Luminescent Chromism of Tetrathiazolylthiophene, J. Am. Chem. Soc., 2013, 135, 10322–10325 CrossRef CAS PubMed
.
- J. Shi, S.-J. Yoon, L. Viani, S. Y. Park, B. Milián-Medina and J. Gierschner, Twist-Elasticity-Controlled Crystal Emission in Highly Luminescent Polymorphs of Cyano-Substituted Distyrylbenzene (βDCS), Adv. Opt. Mater., 2017, 5, 1700340 CrossRef
.
- J. Gierschner and S. Y. Park, Luminescent distyrylbenzenes: tailoring molecular structure and crystalline morphology, J. Mater. Chem. C, 2013, 1, 5818–5832 RSC
.
- A. Afrin and P. Chinna Ayya Swamy, Symphony of light: AIE and MFC in carbazole-based cyanostilbenes, J. Mater. Chem. C, 2024, 12, 1923–1944 RSC
.
- L. Zhu and Y. Zhao, Cyanostilbene-based intelligent organic optoelectronic materials, J. Mater. Chem. C, 2013, 1, 1059–1065 RSC
.
- Z. Yang, Z. Chi, Z. Mao, Y. Zhang, S. Liu, J. Zhao, M. P. Aldred and Z. Chi, Recent advances in mechano-responsive luminescence of tetraphenylethylene derivatives with aggregation-induced emission properties, Mater. Chem. Front., 2018, 2, 861–890 RSC
.
- Y. Dong, J. W. Y. Lam, A. Qin, Z. Li, J. Sun, H. H. Y. Sung, I. D. Williams and B. Z. Tang, Switching the light emission of (4-biphenylyl)phenyldibenzofulvene by morphological modulation: crystallization-induced emission enhancement, Chem. Commun., 2007, 40–42, 10.1039/B613157C
.
- Y. Hong, J. W. Y. Lam and B. Z. Tang, Aggregation-induced emission, Chem. Soc. Rev., 2011, 40, 5361–5388 RSC
.
- W. Yang, C. Li, M. Zhang, W. Zhou, R. Xue, H. Liu and Y. Li, Aggregation-induced emission and intermolecular charge transfer effect in triphenylamine fluorophores containing diphenylhydrazone structures, Phys. Chem. Chem. Phys., 2016, 18, 28052–28060 RSC
.
- P. Gayathri, M. Pannipara, A. G. Al-Sehemi and S. P. Anthony, Triphenylamine-based stimuli-responsive solid state fluorescent materials, New J. Chem., 2020, 44, 8680–8696 RSC
.
- Z. Huang, F. Tang, F. He, L. Kong, J. Huang, J. Yang and A. Ding, Pyrene and triphenylamine substituted cyanostyrene and cyanostilbene derivatives with dual-state emission for high-contrast mechanofluorochromism and cell imaging, Org. Chem. Front., 2022, 9, 5118–5124 RSC
.
- Y. Dong, B. Xu, J. Zhang, X. Tan, L. Wang, J. Chen, H. Lv, S. Wen, B. Li, L. Ye, B. Zou and W. Tian, Piezochromic Luminescence Based on the Molecular Aggregation of 9,10-Bis((E)-2-(pyrid-2-yl)vinyl)anthracene, Angew. Chem., Int. Ed., 2012, 51, 10782–10785 CrossRef CAS
.
- Y. Dai, H. Liu, T. Geng, R. Duan, X. Li, Y. Liu, W. Liu, B.-G. He, L. Sui, K. Wang, B. Zou, B. Yang and Y. Qi, Piezochromic fluorescence of anthracene derivative crystals with different stacking patterns designed around excimers, J. Mater. Chem. C, 2023, 11, 4892–4898 RSC
.
- R. M. Christie, Fluorescent dyes, Rev. Prog. Color. Relat. Top., 1993, 23, 1–18 CAS
.
- F. Gao, J. Xu and Y.-Y. Yang, Study of Visible Light Photoinduced Polymerization of Methyl Methacrylate Initiated By Biimidazole/Coumarin Dye Systems, J. Photopolym. Sci. Technol., 1999, 12, 339–342 CrossRef
.
- D. Cao, Z. Liu, P. Verwilst, S. Koo, P. Jangjili, J. S. Kim and W. Lin, Coumarin-Based Small-Molecule Fluorescent Chemosensors, Chem. Rev., 2019, 119, 10403–10519 CrossRef CAS PubMed
.
- Y.-X. Peng, H.-Q. Liu, R.-G. Shi, F.-D. Feng, B. Hu and W. Huang, Tetraphenyethylene-Fused Coumarin Compound Showing Highly Switchable Solid-State Luminescence, J. Phys. Chem. C, 2019, 123, 6197–6204 CrossRef CAS
.
- V. F. Traven, D. A. Cheptsov, J. I. Svetlova, I. V. Ivanov, C. Cuerva, C. Lodeiro, F. Duarte, S. F. Dunaev and V. V. Chernyshev, The role of the intermolecular π⋯π interactions in the luminescence behavior of novel coumarin-based pyrazoline materials, Dyes Pigm., 2021, 186, 108942 CrossRef CAS
.
- Y.-Y. Zhang, X.-M. Meng, X.-L. Wang and L.-H. Xu, Studies on the synthesis and spectra characteristics of stilbenylcoumarin organic materials, Dyes Pigm., 2003, 56, 189–194 CrossRef CAS
.
- H. Zeng and C.-J. Li, A Complete Switch of the Directional Selectivity in the Annulation of 2-Hydroxybenzaldehydes with Alkynes, Angew. Chem., Int. Ed., 2014, 53, 13862–13865 CrossRef CAS
.
- L. Schmidt, T. Doroshenko, P. Barbie, A. Grüter, G. Jung and U. Kazmaier, Synthesis of Fluorescent Amino Acids via Palladium-Catalyzed Allylic Alkylations, Synthesis, 2016, 3077–3086 CAS
.
- E. G. McRae and M. Kasha, Enhancement of Phosphorescence Ability upon Aggregation of Dye Molecules, J. Chem. Phys., 1958, 28, 721–722 CrossRef CAS
.
- F. C. Spano, J. Clark, C. Silva and R. H. Friend, Determining exciton coherence from the photoluminescence spectral line shape in poly(3-hexylthiophene) thin films, J. Chem. Phys., 2009, 130, 4904 CrossRef
.
- J. Gierschner, Y.-S. Huang, B. Van Averbeke, J. Cornil, R. H. Friend and D. Beljonne, Excitonic versus electronic couplings in molecular assemblies: The importance of non-nearest neighbor interactions, J. Chem. Phys., 2009, 130, 044105 CrossRef
.
- M. Kasha, H. R. Rawls and M. Ashraf El-Bayoumi, Pure Appl. Chem., 1965, 11, 371–392 CAS
.
- X. Luo, J. Li, C. Li, L. Heng, Y. Q. Dong, Z. Liu, Z. Bo and B. Z. Tang, Reversible Switching of the Emission of Diphenyldibenzofulvenes by Thermal and Mechanical Stimuli, Adv. Mater., 2011, 23, 3261–3265 CrossRef CAS PubMed
.
- S.-J. Yoon, J. W. Chung, J. Gierschner, K. S. Kim, M.-G. Choi, D. Kim and S. Y. Park, Multistimuli Two-Color Luminescence Switching via Different Slip-Stacking of Highly Fluorescent Molecular Sheets, J. Am. Chem. Soc., 2010, 132, 13675–13683 CrossRef CAS
.
- P. F. Jones and M. Nicol, Excimer Fluorescence of Crystalline Anthracene and Naphthalene Produced by High Pressure, J. Chem. Phys., 1965, 43, 3759–3760 CrossRef CAS
.
- Z. A. Dreger, H. Lucas and Y. M. Gupta, High-Pressure Effects on Fluorescence of Anthracene Crystals, J. Phys. Chem. B, 2003, 107, 9268–9274 CrossRef CAS
.
- H. Liu, Y. Dai, Y. Gao, H. Gao, L. Yao, S. Zhang, Z. Xie, K. Wang, B. Zou, B. Yang and Y. Ma, Monodisperse π–π Stacking Anthracene Dimer under Pressure: Unique Fluorescence Behaviors and Experimental Determination of Interplanar Distance at Excimer Equilibrium Geometry, Adv. Opt. Mater., 2018, 6, 1800085 CrossRef
.
- Q. Qi, J. Qian, X. Tan, J. Zhang, L. Wang, B. Xu, B. Zou and W. Tian, Remarkable Turn-On and Color-Tuned Piezochromic Luminescence: Mechanically Switching Intramolecular Charge Transfer in Molecular Crystals, Adv. Funct. Mater., 2015, 25, 4005–4010 CrossRef CAS
.
- Y. Dai, S. Zhang, H. Liu, K. Wang, F. Li, B. Han, B. Yang and B. Zou, Pressure Tuning Dual Fluorescence of 4-(N,N-Dimethylamino)benzonitrile, J. Phys. Chem. C, 2017, 121, 4909–4916 CrossRef CAS
.
|
This journal is © The Royal Society of Chemistry 2025 |
Click here to see how this site uses Cookies. View our privacy policy here.