DOI:
10.1039/D4NA00871E
(Paper)
Nanoscale Adv., 2025,
7, 1143-1153
Morphology controlled Cu3BiS3 nanostructures: superior electrocatalytic sensing of organic nitro compounds†
Received
21st October 2024
, Accepted 20th December 2024
First published on 20th December 2024
Abstract
Addressing the pressing need to develop affordable and efficient catalysts is essential. In this study, we successfully synthesized Cu3BiS3 nanostructures with a modified morphology using three different nitrogen bases: DBN, DBU, and DABCO via a hydrothermal technique. These nanostructures were used for the electrochemical detection of organic nitro groups, a previously unexplored application for this material. We conducted a thorough characterization of the Cu3BiS3 nanostructures using various analytical and spectroscopic methods, including PXRD, FESEM, TEM, XPS, UV-vis, and BET, ensuring the reliability of our results. We then investigated their performance in the electrochemical detection of 4-dinitrophenol (4-NP) and 2,4-dinitrophenol (2,4-DNP) using a modified glassy carbon (GC) electrode. The Cu3BiS3 material produced using DABCO exhibited better sensitivity towards 4-NP detection, with a low limit of detection (LOD) of 0.50 μM compared to the ones synthesized using DBN and DBU. Furthermore, the synthesized materials demonstrated the ability to detect their structural analogue, 2,4-DNP. The distinctive hierarchical nanostructures attained in Cu3BiS3 highlight the benefits of developing such catalysts and the impact of nitrogenous bases in defining the morphology of the materials with enhanced catalytic activities.
1 Introduction
With the rapid progress in science and technology and the widespread growth of industries, the demand for fuels and the use of chemicals has increased significantly.1–7 This development has resulted in a surge in CO2 emissions, toxic chemicals, and other greenhouse gases, leading to environmental devastation. Industries release excessive phenolic compounds into the ecosystem, with nitrophenol being one of the most hazardous. Many industries use nitrophenols to produce various chemicals, such as dyes, medicines, and pesticides.8,9 These nitrophenols are carcinogenic, poisonous, inhibitory, and resistant to biological degradation. In particular, 4-nitrophenol (4-NP) and 2,4-dinitrophenol (2,4-DNP) are highly toxic and cause environmental and biological harm.10,11 Even in small amounts, 4-NP affects organs such as the brain and kidneys.12–15 Due to its strong chemical stability and microbial degradation resistance, purifying water bodies contaminated with 4-NP is complicated. Therefore, it is designated as a toxic contaminant worldwide.
The techniques used to detect nitrophenols, such as UV-visible spectroscopy and HPLC, are time-consuming since they require the transfer of samples to the instruments. The literature describes alternative methods like flow injection reflectors, high-performance capillary zone electrophoresis, and enzyme-mediated immune sorbent assays to detect 4-NP. However, their high post-treatment costs and rigorous testing conditions hinder their widespread adoption.16 As a result, electrochemical detection using nanomaterial-based sensors has emerged as a practical approach due to its rapid response, ease of operation, high sensitivity, cost-effectiveness, and eco-friendliness.17 For example, Nurul et al. successfully synthesized silver nanoparticles supported on graphene oxide to detect 4-NP, achieving a limit of detection (LOD) of 1.2 nM.8 Dhanasekaran et al. reported the synthesis of silver nanoparticles doped on Co–Al layered double hydroxides protected by poly(o-phenylenediamine) as electrocatalysts, which exhibited a LOD of 63 nM and 50 nM for detecting 4-NP and 2,4-DNP, respectively, through electrochemical methods.18 Considering the significant LOD of these materials for 4-NP detection, it is imperative to develop improved catalysts for both the detection and degradation of 4-NP and its similar compounds.
Transition metal chalcogenides have garnered significant attention due to their exceptional physical, chemical, and electrochemical properties. The presence of vacant d-orbitals in transition metals enables the formation of chalcogenides with diverse compositions and stoichiometry, including binary, ternary, and quaternary structures.19 These nanostructures exhibit unique characteristics, such as varying bandgaps, excellent electrical conductivity, and smooth charge carrier transfer. Owing to these versatile features, transition metal chalcogenides are used in energy conversion and storage devices, thermoelectric systems, electro-photo catalysis, energy harvesting materials, and hydrogen generation.20–27
Copper-containing semiconductors prepared from abundant and environmentally safe elements have shown great promise as photoelectrocatalysts. Nanostructured materials like Cu2SnS3, Cu2ZnSnS4, Cu2FeSnS4, and Cu2BaSnS4 have been found to be excellent candidates for photoelectrocatalytic water splitting due to their favorable bandgap (1.2–1.5 eV) and high absorptivity coefficients.28 In particular, the wittichenite crystal phase of Cu3BiS3 is considered highly suitable for photovoltaic absorption, boasting a high absorptivity coefficient of ∼104 cm−1 and an appropriate band gap of ∼1.5–1.7 eV.29,30 For instance, Jiajia et al. synthesized a Cu3BiS3 nanorods/TiO2 heterostructure using a simple solution dip-coating technique, which displayed stronger absorption in the visible region compared to pure Cu3BiS3 and pure TiO2.31 When utilized for the photoelectrochemical hydrogen evolution reaction, this material outperformed pure Cu3BiS3 nanorods. While the Cu3BiS3 nanostructure exhibits impressive properties, its capabilities in CO2 and N2 reduction, as well as its potential for detection/sensing applications, have not been extensively explored and warrant further investigation.
We have developed a hydrothermal process to prepare the wittichenite phase of Cu3BiS3 in various morphologies using three different organic nitrogenous bases: DBU, DABCO and DBN. These bases served as ligands or stabilizers to regulate the product's morphology. In this study, for the first time, we present Cu3BiS3 in three different forms – spheres, rods, and worm-like structures for electrochemical 4-NP detection and its analogs (2,4-DNP). These catalysts exhibited remarkable optical and charge transport properties and possess large specific surface areas, as demonstrated by their excellent electrochemical activities.
2 Experimental
2.1 Materials
Cuprous nitrate (Cu(NO3)2·5H2O), Bi(NO3)2·5H2O, thiourea, DBU, DABCO, DBN, ethanol, water, and ethylene glycol were obtained from Sigma-Aldrich. The chemicals were high purity; no other second purification step was required.
2.2 Synthesis of Cu3BiS3
Step-i: in a typical synthesis, a solution was created by mixing ethanol (32 ml), water (2 ml), and ethylene glycol (22 ml) in a round-bottom flask. The precursors of Cu(NO3)2·5H2O (200 mg, 1.07 mmol), Bi(NO3)2·5H2O (110 mg, 0.35 mmol), and DBU (50 μL, 0.35 mmol) were simultaneously added to the solution. The resulting mixture was then transferred to a hydrothermal bomb and stirred for 24 hours. Subsequently, the reaction temperature was set at 170 °C for 2 hours and then cooled to room temperature. The resulting mixture was washed with distilled water multiple times to obtain CuBi precursor. It was dried in a vacuum oven to remove any moisture.
Step-ii: the dried material (CuBi precursor) was dispersed again in 55 ml of distilled water and stirred for 30 minutes, followed by the addition of thiourea (81 mg, 1.07 mmol). The mixture was left for 6 hours at 160 °C in a hydrothermal bomb. After the allotted time, the reaction mixture was allowed to cool to room temperature. The resulting black materials were washed with ethanol and water several times and then dried in an oven at 60 °C for 24 hours.
The similar reactions were performed in the presence of DBN (42 μL, 0.35 mmol) and DABCO (59 mg, 0.35 mmol) separately. The final materials obtained were labeled as Cu3BiS3-DBU, Cu3BiS3-DBN, and Cu3BiS3-DABCO.
2.3 Characterization and instrumentations
The crystal structure predictions of Cu3BiS3 were determined using powder X-ray diffraction (PXRD) with a Bruker D8 X-ray diffractometer equipped with a Cu-Kα radiation source (λ = 0.15406 nm). Surface analyses were carried out using field-emission scanning electron microscopy (FESEM) with an Ultra 55 Carl Zeiss instrument operating at 10 kV, as well as transmission electron microscopy (TEM) with an FEI Technai G2 20 STEM with a 200 kV acceleration voltage. Optical properties, such as absorption spectrum and band gap, were examined using a JASCO-V770 UV/Vis spectrometer. Surface area and pore size analysis were conducted based on adsorption–desorption isotherms using Quantachrome instruments. The elemental composition and oxidation states were also analyzed via X-ray photoelectron spectroscopy (XPS) using a Thermal Scientific Escalab 250Xi spectrometer with Al-Kα radiation.
2.4 Electrochemical measurements
The prepared materials were evaluated for the electrochemical reduction of 4-nitrophenol (4-NP) and 2,4-dinitrophenol (2,4-DNP) using CHI 6612E (Shanghai Chenhua Instrument Co., China) electrochemical workstation in a three-electrode set up. The platinum electrode served as a counter electrode, silver/silver chloride (3.5 M KCl) as the reference electrode, and the electrocatalyst-modified glassy carbon electrode (GCE) as the working electrode. The catalyst was prepared by taking 2 mg of either Cu3BiS3-DBU, Cu3BiS3-DBN, or Cu3BiS3-DABCO in a mixture of water and isopropanol followed by addition of 30 μl of Nafion binder. The prepared slurry of catalyst was coated on the surface of pre-cleaned GCE. The 0.1 M phosphate buffer solution (PBS) was used as supporting electrolyte at different pH. Varying amounts of 4-NP and 2,4-DNP were added into the buffer solution while performing sensing activity.
3. Results and discussion
3.1 Synthesis and characterization of Cu3BiS3 nanostructures
A hydrothermal method was employed to produce Cu3BiS3 nanomaterials with diverse morphologies using three different organic bases (DBN, DBU, and DABCO). The crystal structure and phase purity of the prepared Cu3BiS3 were analyzed using Powder X-ray diffraction (PXRD) (Fig. 1), revealing that all peaks in the three PXRDs can be attributed to the orthorhombic phase of Cu3BiS3 (JCPDS 00-043-1479, a = 7.696 Å; b = 10.38 Å, c = 6.711 Å). The diffraction peaks at 2θ value of 15.74°, 17.09°, 19.53°, 23.15°, 24.70°, 26.74°, 28.10°, 29.00°, 30.31°, 31.31°, 33.88°, 34.87°, 36.07°, 41.51°, 43.04°, 44.17°, 45.34°, 46.38°, 48.01°, 49.91°, 51.83°, 52.77°, 54.55° and 57.61° belong to (110), (020), (111), (200), (210), (201), (211), (031/102), (112), (131/102), (122), (230), (301), (321), (113), (241), (312), (331), (410), (133/340), (251), (060), (430) and (323/342) diffraction planes. The most intense sharp peak at 31.2° corroborates the (131) crystal facet of the material, consistent with JCPDS card data 43-1479.32 No diffraction peaks corresponding to other phases of Cu3BiS3 were detected, underscoring the phase purity of Cu3BiS3.
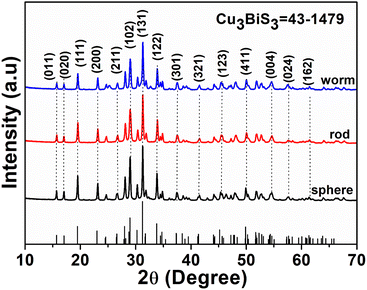 |
| Fig. 1 The PXRD patterns of Cu3BiS3 (JCPDS 43-1479) obtained using DBU (spheres), DBN (worms) and DABCO (rods). | |
The XPS analyses have verified the existence of specific elements and their chemical states in the produced materials. Fig. 2 displays the survey spectrum of Cu3BiS3 hierarchical structures, confirming the presence of Cu, Bi, and S elements. Furthermore, in Fig. 2b, the notable intense peaks at 936.4 eV and 956.7 eV binding energies closely resemble the Cu 2p3/2 and Cu 2p1/2 peaks of Cu3BiS3. Additionally, Fig. 2c illustrates the binding energies of the bismuth 4f doublet at 162.1 eV and 168.5 eV, corresponding to the Bi 4f7/2 and Bi 4f5/2. The spin-orbit splitting value of Cu 2p and Bi 4f is calculated to be 19.7 eV (Cu+) and 5.3 eV (Bi3+). The S 2p peak is observed at the binding energy of 163.1 eV, consistent with existing literature reports. Consequently, the XPS spectra of Cu3BiS3 nanostructures validate the presence of Cu+, Bi3+, and S2− chemical states within the obtained Cu3BiS3.28
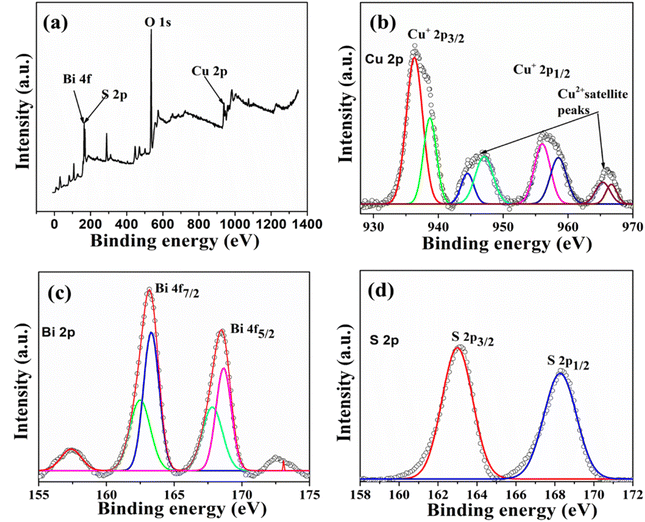 |
| Fig. 2 The XPS spectra of Cu3BiS3 nanostructures (a) survey spectrum showing the presence of all the required elements. (b) XPS spectra of Cu 2p (c) XPS spectrum of Bi 4f and (d) XPS spectrum of S 2p. | |
3.2 Morphology and surface area of Cu3BiS3 nanostructures
The examination of Cu3BiS3 nanomaterials using FESEM revealed variations in the surface morphology of three samples produced in reactions in the presence of three different bases (Fig. 3). Cu3BiS3-DBU samples displayed a spherical morphology, Cu3BiS3-DABCO showed a rod-like surface, and Cu3BiS3-DBN exhibited a worm-like morphology (Fig. 3a–c). The morphological results clearly indicate that the organic basis played a well definite role in architecting the surface of nanocrystals, which have great impact on the catalytic activities of achieved structures. The high magnification FESEM images representing individual sphere, rod, and worms are shown in Fig. S1.† The average particle size of Cu3BiS3 nanostructures is calculated as shown in Fig. S2a–c.† Additionally, TEM analysis confirmed the spherical morphology of Cu3BiS3-DBU with an average diameter of 101.89 nm, the rod-like topography of Cu3BiS3-DABCO with an average length of 13.84 nm and an average width of 21.19 nm, and the worm-like morphology of Cu3BiS3-DBN with an overall size of 97.85 nm (Fig. 3d–f). The EDAS (Fig. S3†) additionally reveals that all the elements were equally distributed within the Cu3BiS3 nanostructures. Furthermore, the high-resolution TEM micrographs of the synthesized materials exhibited close d-spacing with their corresponding PXRD. The distances of 0.28 nm between lattice fringes were consistent with the (131) crystal planes for the sphere-shaped material. Similarly, interplanar spacings of 0.28 nm corresponded to (131) plane for the rod-shaped material, while the spacing of 0.28 nm aligned with the (131) crystal facet of the worm-shaped material. The corresponding SAED patterns of synthesized Cu3BiS3 nanomaterials are shown in Fig. S1d–f.† The bright spots in SAED reveals the high crystalline nature of Cu3BiS3 nanomaterials which is in good agreement with the PXRD values.
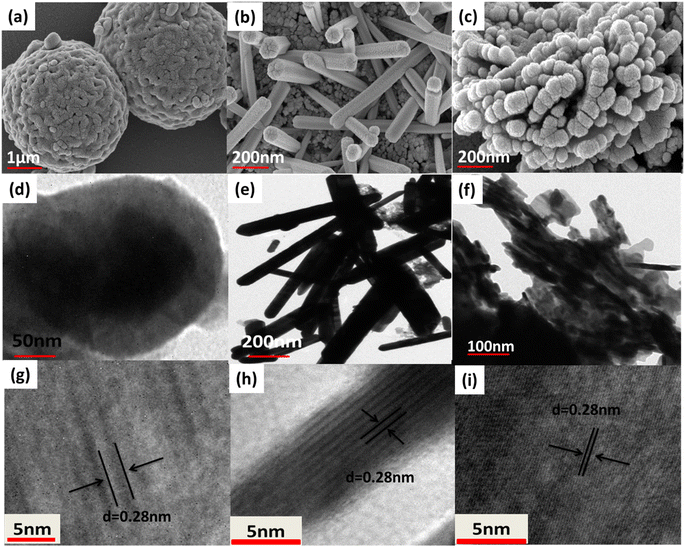 |
| Fig. 3 FESEM images: (a) spheres of Cu3BiS3-DBU, (b) rods of Cu3BiS3-DABCO, (c) worm-like morphology of Cu3BiS3-DBN. TEM (d) spheres, (e) rods, (f) worms of Cu3BiS3 and their respective HRTEM images (g–i) with d-spacing. | |
Besides surface morphology, the exceptional catalytic activities of nanostructured materials can also largely be attributed to their specific surface area. In this study, we analyzed the nitrogen adsorption–desorption BET isotherms of Cu3BiS3 materials (refer to Fig. 4). The specific surface areas of the three Cu3BiS3 nanostructures exhibiting spherical, rod-like, and worm-like morphologies were 19.570 m2 g−1, 22.723 m2 g−1, and 33.676 m2 g−1, respectively. Additionally, the pore size distribution of Cu3BiS3 nanostructures (refer to Fig. S4†) fell within the 10–15 nm range, indicating a mesoporous structure.
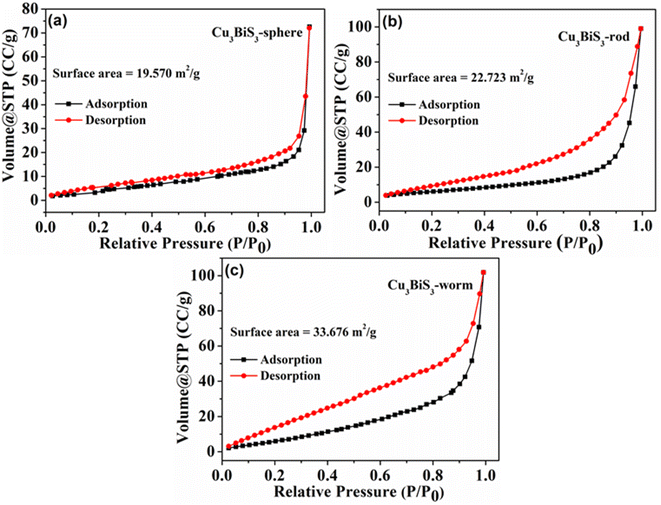 |
| Fig. 4 The nitrogen adsorption–desorption isotherms of Cu3BiS3 nanostructures (a) spheres (b) rods (c) worms. | |
The solid-state UV-visible diffuse reflectance spectra at room temperature examined the optical properties of these materials in the range of 200 to 1200 nm. Analysis of the spectra (Fig. S5a†) revealed three broad peaks in the absorption spectrum of the synthesized Cu3BiS3 materials, spanning 400 to 800 nm. The optical band gap of Cu3BiS3 materials (Cu3BiS3-DBU, Cu3BiS3-DBN, and Cu3BiS3-DABCO) (Fig. S5b†) was determined from the Tauc plot are found to be in the range of 0.45 to 0.90 eV. These measured band gap values are relatively low compared to those previously reported in the literature, suggesting that particle size may have a significant effect.
3.3. Electrochemical detection of 4-NP and 2,4-DNP
The catalyst's electrochemical behavior was explored in a solution containing 100 μM 4-NP in 0.1 M PBS. The resulting cyclic voltammograms (CV) of Cu3BiS3 at different pH levels (5–9) are shown in Fig. 5. As expected, the CV results of the plain GCE exhibited a low reduction current. The obtained CV curves of Cu3BiS3-DBU and Cu3BiS3-DABCO materials with sphere and rod morphology showed poorer current responses (Fig. 5a and b) compared to Cu3BiS3-DBN with a worm-like morphology (Fig. 5c). The higher reduction current of Cu3BiS3-DBN is primarily attributed to its larger surface area, presence of a greater number of active sites, and hierarchical morphological structure. The activity of Cu3BiS3 towards 4-NP was primarily identified by adding 4-NP to 0.1 M PBS solution at pH 6. It was observed that (Fig. S6a–c†) the current was increasing with the addition of 4-NP, which clearly indicated the sensing activity of Cu3BiS3 materials.
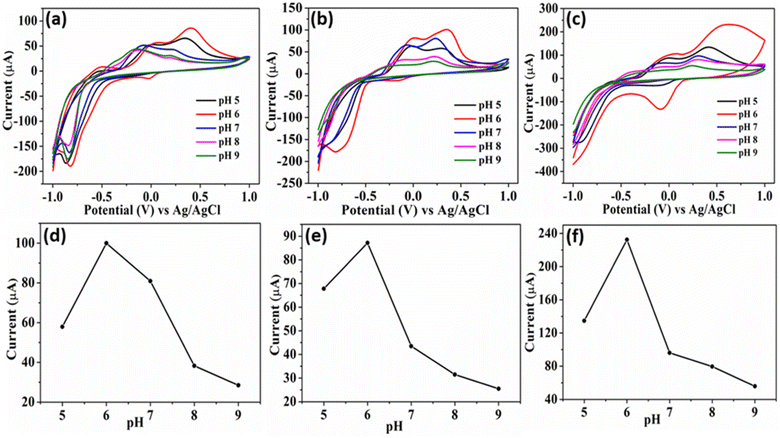 |
| Fig. 5 CV of Cu3BiS3 for 100 μM 4-NP at different 0.1 M PBS pH (5–9) (a) Cu3BiS3-sphere, (b) Cu3BiS3-rod, and (c) Cu3BiS3-worm. (d–f) The corresponding plots of current vs. different pH. | |
The pH of the medium significantly influenced the electrochemical detection of 4-NP due to the involvement of phenolic hydroxyl groups in proton transfer reactions, leading to the formation of quinones.33,34 The current plots obtained at different pH levels (5 to 9) for all three materials (Fig. 5d–f) showed the maximum reduction current achieved in the case of Cu3BiS3-DBN (worm-like). The pH studies revealed that as the pH increased from 5 to 9, the cathodic peak potential of 4-NP consistently shifted to the negative side. The peak current (Ipc) of 4-NP was identified to be higher at pH 6.0. Therefore, for further evaluation of the materials' sensing/detection performance, pH 6.0 was considered the optimal pH in this study.
We conducted a study to assess the impact of 4-NP concentrations on the reduction current at 0.1 M PBS pH 6. We plotted the effect of increasing 4-NP concentrations from 10 μM to 100 μM on the CV (Fig. 6a–c). Our findings indicated that the higher concentration of 4-NP led to a more pronounced intensity of the reduction peak in worms (Fig. 6c) than in sphere and rod morphologies (Fig. 6a and b). Moreover, when we correlated the plots of reduction peak current with the concentration of 4-NP, we observed linear fits, as depicted in Fig. 6d–f. This observation suggests that the increase in concentration had a linear effect on the detection of 4-NP by the synthesized materials, with the maximum limit observed for Cu3BiS3-DBN worms.
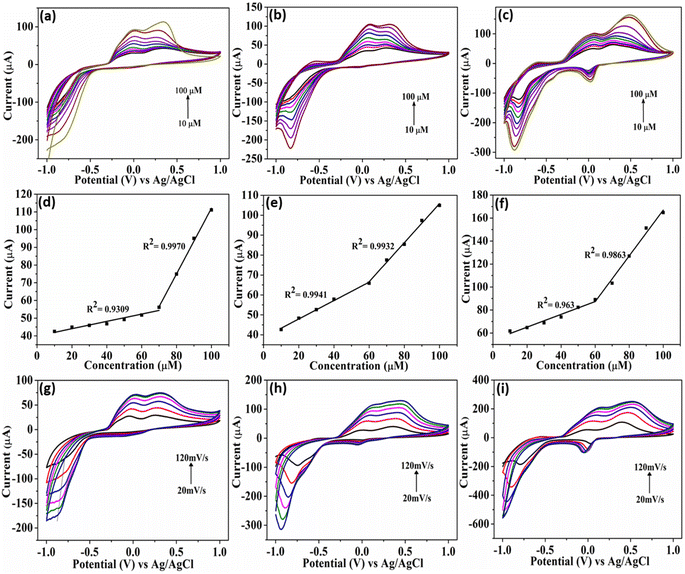 |
| Fig. 6 CV of Cu3BiS3 at different concentrations of 4-NP (10–100 μm). (a) Cu3BiS3-DBU spheres, (b) Cu3BiS3-DABCO rods, and (c) Cu3BiS3-DBN worms. (d and e) The corresponding linear fit plots of current vs. concentration, and (g–i) CV at different scan rates of 20–120 mV s−1 of the three morphologies respectively. | |
Furthermore, in order to explore the changing aspects of the electrode reaction, we studied the CV curves of Cu3BiS3 at different scan rates while maintaining the 4-NP concentration constant. The graphs in Fig. 6(g–i) illustrate that as the scan rates increased (20–120 mV s−1) at a constant 100 μM concentration of 4-NP (pH-6) within a potential window of −1.0 to 1.0 V, there was an increase in the reduction peak current. This enhancement was most noticeable in the Cu3BiS3-DBN worms compared to the other two morphologies, Cu3BiS3-DBU spheres and Cu3BiS3-DABCO rods. This enhanced activity of Cu3BiS3-DBN worms was further supported by the graphs obtained by plotting the square root of scan rates against peak currents (Fig. S6d–f†). The linear trend line indicates a sharp increase in current, possibly due to the diffusion-controlled process.
For further exploration of the 4-NP sensing, the limit of detection (LOD), limit of quantification (LOQ) and sensitivity of the three morphologically different Cu3BiS3 materials were studied. The LOD were calculated by the equation as:35
where
σ is the standard deviation of the blank sample and
S is the slope of the calibration curve. By using this equation, the LOD was determined to be 0.19, 0.13, 0.10 μM for Cu
3BiS
3-S, Cu
3BiS
3-R, and Cu
3BiS
3-W respectively. In
Table 1 we have reported the comparison of 4-NP electrochemical detection by the as synthesized Cu
3BiS
3 nanomaterials with different morphologies. From the results, it is observed that our approach of synthesizing materials has achieved a lower value of LOD, which may be credited to the high specific surface area of the synthesized materials and the resilient contact between the improved electrode surface and analytes. Therefore, it is clear that the Cu
3BiS
3-DBN modified electrode requires the low potential for detection of 4-NP at micromolar levels.
Table 1 The LOD and sensitivity of Cu3BiS3 nanomaterials in detection of 4-NP and 2,4-DNP
Material |
Experiment |
LOD (μM) |
LOQ (μM) |
Sensitivity (μA μM−1 cm−2) |
Cu3BiS3-sphere |
4-NP |
0.193 |
0.586 |
7.87 |
Cu3BiS3-rod |
4-NP |
0.135 |
0.410 |
13.87 |
Cu
3
BiS
3
-worm
|
4-NP
|
0.103
|
0.314
|
26.23
|
Cu3BiS3-sphere |
2,4-DNP |
0.158 |
0.480 |
0.087 |
Cu3BiS3-rod |
2,4-DNP |
0.110 |
0.333 |
0.082 |
Cu
3
BiS
3
-worm
|
2,4-DNP
|
0.104
|
0.240
|
0.766
|
Electrochemical impedance spectroscopy (EIS) is a crucial tool for evaluating electrochemical behaviour. The electrical hysteresis characteristics of Cu3BiS3 and bare glassy carbon electrode (GCE) were investigated using EIS in a 5 mM K3[Fe(CN)6] solution. The loaded electrode created an arc with a lesser radius than the unloaded electrode in EIS (Fig. 7h). This result indicated that the Cu3BiS3-DBN nanomaterial effectively reduces the charge transfer resistance of the GCE surface, leading to improved electron conduction efficiency compared to the unloaded electrode. The presence of Cu3BiS3-DBN with a worm-like morphology significantly alters the electrical properties of the GCE surface, making it a promising EIS sensor for detecting cells, bacteria, and biomarkers. Conversely, Cu3BiS3 with spherical and rod-like morphologies do not exhibit similar effectiveness. The electroactive surface area was examined using CV in 0.1 M KCl solution containing 5 mM K3[Fe(CN)6], as shown in Fig. 7g. The enhanced peak current observed in the Cu3BiS3-DBN with a worm-like morphology indicates an increased active surface area, which is attributed to the improvement in the redox reaction of Fe(CN)63−/4−. These results suggest that Cu3BiS3-DBN exhibits superior electron transfer capability compared to Cu3BiS3-DABCO and Cu3BiS3-DBU.
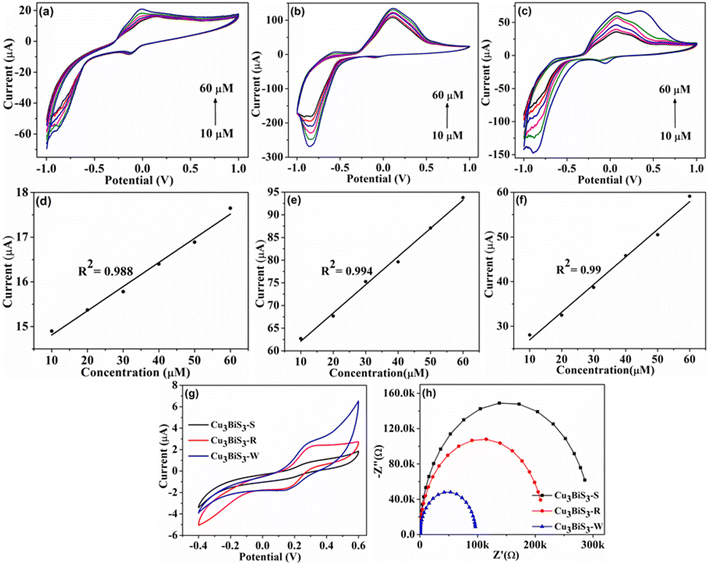 |
| Fig. 7 (a–c) CV at different concentrations for 2,4-DNP, (d–f) linear plots of current vs. concentrations with three different morphologies. (g and h) CV and EIS plots of catalysts in 5 mM K3[Fe(CN)6] with 0.1 M KCl solution. | |
An analogous electrochemical phenomenon was observed in detecting 2,4-DNP in the presence of Cu3BiS3 nanocatalysts. Various studies were conducted to analyze the impact of different parameters, such as pH, scan rates, and 2,4-DNP concentration on the sensing capabilities of Cu3BiS3 catalysts. As depicted in Fig. 7a–c with varying concentrations, the reduction current increases with the rise in 2,4-DNP concentration, which is further evidenced by linear plots (Fig. 7d–f) illustrating the relationship between current and concentrations. Similarly, the influence of pH and scan rates was also investigated with the other parameters (Fig. S7 and S8†). The values of LOD, LOQ and sensitivity of the synthesized electrocatalysts are presented in Table 1. The findings reveal that the worm-shaped nanostructures exhibit the most effective sensing capability among the three different morphologies for detecting 2,4-DNP.
In addition, the electrochemical properties of Cu3BiS3 nanostructures were confirmed through chronoamperometry response for 4-nitrophenol (5–35 μm) and 2,4-dinitrophenol (10–50 μm) in a 20 ml solution at pH 6 PBS, under a potential of 0.30 V (Fig. 8a–f). The research indicated that the catalysts operational conditions were directly impacted by the regular addition of the substrates at 50 seconds intervals. Each incremental addition (5 μm for 4-nitrophenol and 10 μm for 2,4-dinitrophenol) displayed a consistent linear trend. The electrode system exhibited rapid responses to each 4-nitrophenol and 2,4-dinitrophenol addition and reached current saturation within 5 seconds. The linear correlation between current and concentration maintained a consistent pattern, demonstrating a proportional relationship between the response current and the concentrations of 4-nitrophenol and 2,4-dinitrophenol (Fig. S9†). This study underscores the sensitivity and precision of Cu3BiS3 morphological structures in detecting 4-nitrophenol and 2,4-dinitrophenol.
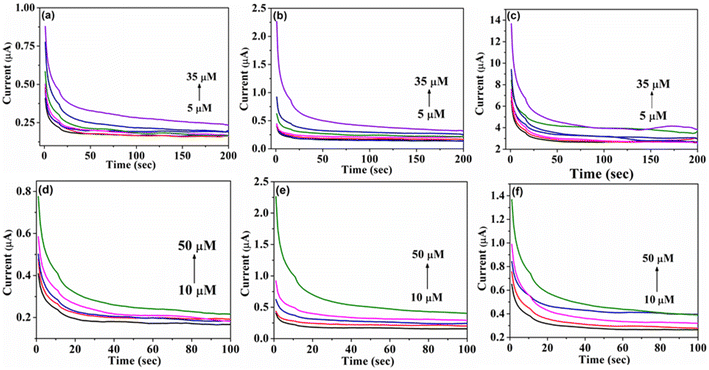 |
| Fig. 8 Chronoamperometry studies of (a–c) 4-NP, and (d–f) 2,4-DNP for Cu3BiS3 spheres, rods, and worms respectively. | |
3.4. Interference study of 4-NP and 2,4-DNP with Cu3BiS3
The interference studies of 4-NP and 2,4-DNP were conducted in the presence of other potential interferents that could hinder the accurate detection of 4-NP and 2,4-DNP (refer to Fig. S10†). A range of interferents, including nitrobenzene, resorcinol, catechol, quinone, aniline, KCl, and acetate, were selected to demonstrate the selectivity of the modified electrode. It was observed that the Cu3BiS3 catalysts exhibited minimal change in current response in the presence of interferents when compared to 4-NP and 2,4-DNP, even at higher concentrations. Among the three morphologies, the worm shape displayed exceptional anti-interference ability.
When Cu3BiS3 nanomaterial is used as an electrocatalyst to detect 4-NP, its mesoporous nanostructure can smoothen the adsorption of H2O and 4-NP. Henceforward, 4-NP molecules can be electrochemically reduced to 4-hydroxylaminophenol (4-OH-AP) through the four-electron process (Step i), where the reduction peak (R1) is observed. Successively, the electrochemical oxidation–reduction interaction occurs between 4-OH-AP and 4-nitrosophenol via the two-electron process (Step ii), additionally ensuing in the predominance of the oxidation peak (O) and the other reduction peak (R2) (Fig. 9).36 Likewise, the electrochemical detection of 2,4-DNP on the surface of Cu3BiS3 take place through the concurrent exchange of hydroxyl radical by removing two nitro groups (+0.031 and +0.32 V). The quinone is additionally reduced (−0.16, −0.58, and −0.80 V); hence, all the nitro groups are concentrated in amine groups.18,37
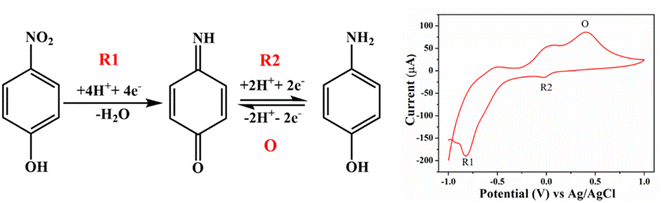 |
| Fig. 9 Plausible detection mechanism of 4-NP and 2,4-DNP for three Cu3BiS3 catalysts. | |
4 Conclusion
Utilising hydrothermal approach, Cu3BiS3 nanostructure with three distinctive morphologies were created and used for the electrochemical detection of 4-NP and 2,4-DNP. The nanostructures showed outstanding performance in precisely detecting trace levels of 4-NP with a LOD of 0.103 μM for worms, as well as in the detection of 2,4-DNP with LOD of 0.104 μM for worms. Due to the hierarchical morphology, small particle size, associated with numerous exposed active sites, and fast charge transfer the Cu3BiS3 nanostructure showed exceptional detectivity of nitro compounds. Here, the specific morphology comprising nanorods, nanospheres, and nanoworms is the key feature of Cu3BiS3 to achieve outstanding electrocatalytic performance. The Cu3BiS3 material exhibited exceptional long-term stability, selectivity, and reproducibility of catalysts. Our findings showed that morphology is also influencing catalytic activity in addition to the surface area. The use of nitrogenous base (DBN, DBU, and DABCO) tuned morphology will provide insight into building novel multifunctional, with hierarchical distinctive morphologies nanomaterials for sensing and other catalytic activities.
Data availability
The data supporting this article have been included as part of the ESI.†
Author contributions
Manzoor Ahmad Pandit, Dasari Sai Hemanth Kumar, Mohan Varkolu – data curation, formal analysis, investigation, methodology, writing – original draft Krishnamurthi Muralidharan – conceptualization, supervision, funding acquisition, writing – review & editing.
Conflicts of interest
The authors declare no conflicts of interest.
Acknowledgements
Authors thank Institute of Eminence grant (no. UoH-IoE-RC3-21-043) of University of Hyderabad for project (KM) and fellowship (DSHK). The instrument support grant from the Department of Science and Technology, New Delhi, India (through FIST program) is gratefully acknowledged. MAP thanks to ACHREM, University of Hyderabad for fellowship.
References
- B. Srinivas, P. Manzoor Ahmad and K. Muralidharan, Importance of Clean Surfaces on the Catalyst: SnS2 Nanorings for Environmental Remediation, ACS Omega, 2019, 4, 14970–14980 CrossRef CAS PubMed
.
- P. Manzoor Ahmad, B. Srinivas and K. Muralidharan, A simplistic approach for the synthesis of CuS-CdS heterostructure: A novel photo catalyst for oxidative dye degradation, J. Environ. Chem. Eng., 2020, 8, 103542 CrossRef
.
- P. Manzoor Ahmad, D. S. H. Kumar, B. Srinivas, M. Ramadoss and K. Muralidharan, Chalcopyrite with Magnetic and Dielectric Properties: An Introductory Catalyst for 4-Nitrophenol Reduction, J. Phys. Chem. C, 2020, 124, 18010–18019 CrossRef
.
- A. Joseph, B. Srinivas, P. M. Ahmad, S. Khatun, A. K. Rengan and K. Muralidharan, Impact of bandgap tuning on ZnS for degradation of environmental pollutants and disinfection. Environmental Science and Pollution Research, Environ. Sci. Pollut. Res., 2022, 29, 56863–56875 CrossRef CAS PubMed
.
- G. A. Rather, A. Nanda and P. M. Ahmad, Green and Facile Synthesis of Zinc Oxide Nanoparticles Using Bergenia cilliata aqueous extract and evaluation of its photocatalytic activity, J. Inorg. Chem. Comm., 2021, 134, 109020 CrossRef
.
- P. M. Ahmad, D. S. H. Kumar, M. Ramadoss, Y. Chen and K. Muralidharan, Template free-synthesis of cobalt–iron chalcogenides [Co0.8Fe0.2L2, L = S, Se] and their robust bifunctional electrocatalysis for the water splitting reaction and Cr(VI) reduction, RSC Adv., 2022, 12, 7762 RSC
.
- M. Ramadoss, Y. Chen, S. Zhe, M. Karpuraranjith, D. Yang, P. M. Ahmad and K. Muralidharan, Iron-Modulated 3D CoNiP Vertical Nanoarrays: An Exploratory Binder-Free Electrocatalyst for Efficient Overall Water Splitting, J. Phys. Chem. C, 2021, 125, 20972–20979 CrossRef CAS
.
- N. I. Ikhsan, P. Rameshkumar and N. H. Huang, Controlled synthesis of reduced graphene oxide supported silver nanoparticles for selective and sensitive electrochemical detection of 4-nitrophenol, Electrochim. Acta, 2016, 192, 392–399 CrossRef CAS
.
- K.-Y. Hwa, K.-Y. Ganguly, A. Santhan and T. S. K. Sharma, Synthesis of Water-Soluble Cadmium Selenide/Zinc Sulfide Quantum Dots on Functionalized Multiwalled Carbon Nanotubes for Efficient Covalent Synergism in Determining Environmental Hazardous Phenolic Compounds, ACS Sustainable Chem. Eng., 2022, 10, 1298–1315 CrossRef CAS
.
- P. Wang, J. Xiao, A. Liao, P. Li, M. Guo and Y. Xia, Electrochemical determination of 4-nitrophenol using uniform nanoparticle film electrode of glass carbon fabricated facilely by square wave potential pulses, Electrochim. Acta, 2015, 176, 448 CrossRef CAS
.
- C. Rajkumar, P. Veerakumar, S.-M. Chen, B. Thirumalraj and K.-C. Lin, Ultrathin Sulfur-Doped Graphitic Carbon Nitride Nanosheets as Metal-Free Catalyst for Electrochemical Sensing and Catalytic Removal of 4-Nitrophenol, ACS Sustain. Chem. Eng., 2018, 6, 6021–16031 Search PubMed
.
- Y. Yang, K. Jiang, J. Guo, J. Li, X. Peng, B. Hong, X. Wang and H. Ge, Facile fabrication of Au/Fe3O4 nanocomposites as excellent nanocatalyst for ultrafast recyclable reduction of 4-nitropheol, Chem. Eng. J., 2019, 381, 122596 CrossRef
.
- B. Wang, X. Lv, D. Feng, L. Xie, J. Zhang, M. Li, Y. Xie, J. Li and H. Zhou, Highly stable Zr(IV)-based metal-organic frameworks for the detection and removal of antibiotics and organic explosives in water, J. Am. Chem. Soc., 2016, 138, 6204–6216 CrossRef CAS PubMed
.
- J. Zhang, T. Yao, H. Zhang, X. Zhang and J. Wu, Preparation of raspberry-like gamma-Fe2O3/crackled nitrogen-doped carbon capsules and their application as supports to improve catalytic activity, Nanoscale, 2016, 8, 18693–18702 RSC
.
- S. Vinoth, P. Sampathkumar, K. Giribabu and A. Pandikumar, Ultrasonically assisted synthesis of barium stannate incorporated graphitic carbon nitride nanocomposite and its analytical performance in electrochemical sensing of 4-nitrophenol, Ultrason. Sonochem., 2020, 62, 104855 CrossRef CAS PubMed
.
- Q. Ding, Z. Kang, L. Cao, M. Lin, H. Lin and D.-P. Yang, Conversion of waste eggshell into difunctional Au/CaCO3 nanocomposite for 4-Nitrophenol electrochemical detection and catalytic reduction, Appl. Surf. Sci., 2020, 510, 145526 CrossRef CAS
.
- L. Wang, T. Meng, G. Yu, S. Wu, J. Sun, H. Jia, H. Wang, X. Yang and Y. Zhang, A label free electrochemical biosensor for ultra-sensitively detecting telomerase activity based on the enhanced catalytic currents of acetaminophen catalyzed by Au nanorods, Biosens. Bioelectron., 2019, 124–125, 53–58 CrossRef CAS PubMed
.
- T. Dhanasekaran, R. Manigandan, A. Padmanaban, R. Suresh, K. Giribabu and V. Narayanan, Fabrication of Ag@Co-Al Layered Double Hydroxides Reinforced poly(o-phenylenediamine) Nanohybrid for Efcient Electrochemical Detection of 4-Nitrophenol, 2,4-Dinitrophenol and Uric acid at Nano Molar Level, Sci. Rep., 2019, 9, 13250 CrossRef CAS PubMed
.
- D. Chauhan, Pooja, V. Nirbhaya, C. Srivastava, R. Chandra and S. Kumar, Nanostructured transition metal chalcogenide embedded on reduced graphene oxide based highly efficient biosensor for cardiovascular disease detection, Microchem. J., 2020, 155, 104697 CrossRef CAS
.
- S. Zhao, K. Wang, X. Zou, L. Gan, H. Du, C. Xu, F. Kang, W. Duan and J. Li, Group VB transition metal dichalcogenides for oxygen reduction reaction and strain-enhanced activity governed by p-orbital electrons of chalcogen, Nano Res., 2019, 12, 925–930 CrossRef CAS
.
- E. Singh, K. Kim, G. Yeom and H. Nalwa, Two-dimensional transition metal dichalcogenide based counter electrodes for dye-sensitized solar cells, RSC Adv., 2017, 7, 28234–28249 RSC
.
- J. Cherusseri, N. Choudhary, K. Kumar, Y. Jung and J. Thomas, Recent trends in transition metal dichalcogenide based supercapacitor electrodes, Nanoscale Horiz., 2019, 4, 840–858 RSC
.
- J. Feng, X. Sun, C. Wu, L. Peng, C. Lin, S. Hu and J. Yang, Metallic few-layered VS2 ultrathin Nanosheets: high two-dimensional conductivity for in-plane supercapacitors, J. Am. Chem. Soc., 2011, 133, 17832–17838 CrossRef CAS PubMed
.
- B. Poudel, Q. Hao, Y. Ma, Y. Lan, A. Minnich, B. Yu, X. Yan, M. Dresselhaus, G. Chen and Z. Ren, High-Thermoelectric performance of nanostructured bismuth antimony telluride bulk alloys, Science, 2008, 320, 634–638 CrossRef CAS PubMed
.
- Q. Li, N. Zhang, Y. Yang, G. Wang and D. Ng, High efficiency photocatalysis for pollutant degradation with MoS2/C3N4 heterostructures, Langmuir, 2014, 30, 4–11 Search PubMed
.
- G. Lee, S. Anandan, S. Masten and J. Wu, Photocatalytic hydrogen evolution from water splitting using Cu doped ZnS microspheres under visible light irradiation, Renewable Energy, 2016, 89, 18–26 CrossRef CAS
.
- Y. Zhang, Q. Zhou, J. Zhu, Q. Yan, S. Dou and W. Sun, Nanostructured metal chalcogenides for energy storage and electrocatalysis, Adv. Funct. Mater., 2017, 27, 1–34 Search PubMed
.
- J. Li, Y. Zhao, X. Han and D. Xiao, A facile strategy for fabricating particle-on-flower Au-Cu3BiS3 nanostructures for enhanced photoelectrocatalytic activity in water splitting, New J. Chem., 2021, 45, 1231–1239 RSC
.
- N. Gerein and J. Haber, One–step synthesis and optical and electrical properties of thin film Cu3BiS3 for use as a solar absorber in photovoltaic devices, Chem. Mater., 2006, 18, 6297–6302 CrossRef CAS
.
- F. Mesa, G. Gordillo, T. Dittrich, K. Ellmer, R. Baier and S. Sadewasser, Transient surface photovoltage of P-type Cu3BiS3, Appl. Phys. Lett., 2010, 96, 082113 CrossRef
.
- J. Li, X. Han, M. Wang, Y. Zhao and C. Dong, Fabrication and enhanced hydrogen evolution reaction performance of a Cu3BiS3 nanorods/TiO2 heterojunction film, New J. Chem., 2018, 42, 4114–4120 RSC
.
- T. Manimozhi, J. Archana and K. Ramamurthi, Shape Controlled synthesis of Cu3BiS3 Nano-and microstructures by PEG assisted solvothermal method and functional properties, Ceram. Int., 2018, 44, 15385–15392 CrossRef CAS
.
- P. Wang, J. Xiao, A. Liao, P. Li, M. Guo, Y. Xia, Z. Li, X. Jiang and W. Huang, Electrochemical determination of 4-nitrophenol using uniform nanoparticle film electrode of glass carbon fabricated facilely by square wave potential pulses, Electrochim. Acta, 2015, 176, 448–455 CrossRef CAS
.
- P. Balasubramanian, T. Balamurugan, S.-M. Chen and T.-W. Chen, Simplistic synthesis of ultrafine CoMnO3 nanosheets: An excellent electrocatalyst for highly sensitive detection of toxic 4-nitrophenol in environmental water samples, J. Hazard. Mater., 2019, 361, 123–133 CrossRef CAS PubMed
.
- S. Ramki, R. Sukanya, S.-M. Chen, M. Sakthivel and J. Wang, Simple hydrothermal synthesis of defective CeMoSe2 dendrites as an effective electrocatalyst for the electrochemical sensing of 4-nitrophenol in water samples, New J. Chem., 2019, 43, 17200 RSC
.
- M. Wang, Y. Liu, L. He, Z. Zhang, Q. Jia, Y. Song and S. Fang, Bimetallic metal–organic framework derived FeOx/TiO2 embedded in mesoporous carbon nanocomposite for the sensitive electrochemical detection of 4-nitrophenol, Sens. Actuators, B, 2019, 281, 1063–1072 CrossRef CAS
.
- S. Md Abdus, C. S. Pallab, M. R. Mohammed, A. Jahir, M. A. Abdullah and Al-M. Mohammad, Fabrication of a 2,4-dinitrophenol sensor based on Fe3O4@Ag@Ni nanomaterials and studies on their antibacterial properties, New J. Chem., 2018, 42, 872–881 RSC
.
|
This journal is © The Royal Society of Chemistry 2025 |
Click here to see how this site uses Cookies. View our privacy policy here.