DOI:
10.1039/D4NH00497C
(Review Article)
Nanoscale Horiz., 2025,
10, 460-483
Revolutionizing healthcare: inorganic medicinal nanoarchitectonics for advanced theranostics
Received
2nd October 2024
, Accepted 12th November 2024
First published on 22nd November 2024
Abstract
Over the last two decades, advancements in nanomaterials and nanoscience have paved the path for the emergence of nano-medical convergence science, significantly impacting healthcare. In our review, we highlight how these advancements are applied in various biomedical technologies such as drug delivery systems, bio-imaging for diagnostic and therapeutic purposes. Recently, novel inorganic nanohybrid drugs have been developed, combining multifunctional inorganic nanomaterials with therapeutic agents (known as inorganic medicinal nanoarchitectonics). These innovative drugs are actively utilized in cutting-edge medical treatments, including targeted anti-cancer therapy, photo and radiation therapy, and immunotherapy. This review provides a detailed overview of the current development status of inorganic medicinal nanoarchitectonics and explores potential future directions in their advancements.
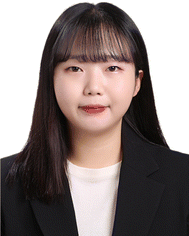
Seungjin Yu
| Seungjin Yu is pursuing a PhD in the Department of Chemistry at Dankook University, where she conducts research at the Intelligent Nanohybrid Materials Laboratory (INML). She is actively engaged in research focused on developing innovative drug delivery systems using two-dimensional layered inorganic materials. Her work explores the potential applications of these materials across various biomedical fields, including targeted therapy and pharmaceutical formulation. Through her research, she aims to improve the effectiveness and precision of drug delivery, ultimately advancing patient care and therapeutic outcomes. |
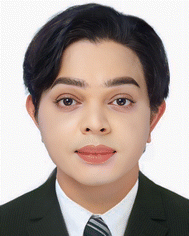
N. Sanoj Rejinold
| Dr Sanoj Rejinold is a leading researcher in nanomaterials, drug delivery systems, and cancer therapy. He currently holds the position of Invited Professor at Dankook University in the Department of Chemistry, South Korea, where he directs research at the Intelligent Nanohybrid Materials Laboratory (INML). With over 60 published papers and an H-index of 33, Dr Rejinold's work focuses on developing nanohybrids, layered double hydroxides, and polymer complexes to treat multi-drug-resistant cancers. His expertise extends to photodynamic and photothermal therapies, targeting metastatic cancers. Dr Rejinold earned his PhD in Nano Chemistry from Amrita Centre for Nanosciences and Molecular Medicine, specializing in biodegradable thermo-responsive polymeric nano-vehicles for cancer therapy. His prestigious Indo-Korea Research Internship enhanced his hands-on experience in tumor xenograft models. His research also explores nanomaterials for viral therapies and innovative drug delivery systems, such as nanogels and microneedles. |
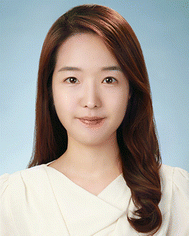
Goeun Choi
| Dr. Goeun Choi is an Assistant Professor in the Department of Chemistry at Dankook University, specializing in inorganic materials chemistry and nano-bio materials with applications in tumor imaging and therapy. She completed her PhD in inorganic chemistry from Ewha Womans University under the mentorship of Prof. Jin-Ho Choy and gained postdoctoral experience at the Center for Intelligent Nano-Bio Materials at Ewha Womans University and the Future Industries Institute at the University of South Australia. Her accolades include the Research Excellence Award from the Korean Society for Laser Medicine and Surgery. |
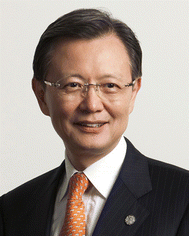
Jin-Ho Choy
| Dr Jin Ho Choy is a highly respected scientist in the field of chemistry and nanomaterials, currently serving as a Chair Professor at Dankook University, South Korea, where he leads the Intelligent Nanohybrid Materials Laboratory (INML). He is internationally recognized for his pioneering work on layered double hydroxides (LDHs) and inorganic–organic nanohybrids, which have extensive applications in drug delivery, cancer therapy, and advanced nanomedicine. His research explores the synthesis, structural characterization, and functionalization of nanomaterials for use in biomedicine, environmental science, and materials engineering. Dr Choy has published extensively in high-impact journals, contributing over 700 papers, several book chapters, and numerous patents in his field. He has received prestigious awards for his groundbreaking research, including recognition from the Korean Chemical Society and the American Chemical Society for his contributions to nanoscience and medicinal chemistry. With a career spanning over four decades, Dr Choy has held various academic and leadership positions, including serving as an editor and reviewer for major scientific journals. His work has attracted significant funding from both government and industry, reflecting the relevance and applicability of his research. He continues to mentor the next generation of scientists and researchers, making significant advancements in nanomedicine and inorganic nanochemistry. |
1. Introduction
Inorganic medicinal nanoarchitectonics represents a revolutionary approach in the field of healthcare, combining the principles of nanotechnology with medicinal chemistry to create advanced theranostic solutions.1–9 This innovative field focuses on the design and synthesis of inorganic nanomaterials that are engineered to interact with biological systems at the molecular level.10–22 By leveraging the unique properties of inorganic materials, such as their stability, biocompatibility, and multifunctionality, researchers have developed a range of applications including targeted drug delivery, enhanced imaging techniques, and effective therapies for various diseases. In particular, inorganic nanohybrids comprising therapeutic agents integrated with inorganic nanomaterials have shown great promise in targeted anti-cancer treatments, photo, radiation, and immunotherapies.23–35 The ability of these nanoarchitectures to simultaneously diagnose and treat diseases, known as theranostics, marks a significant advancement in personalized medicine, offering more precise and effective treatment options with minimal side effects. As the field of inorganic medicinal nanoarchitectonics continues to evolve, it holds the potential to significantly improve patient outcomes and revolutionize the way diseases are diagnosed and treated.
Inorganic medicinal nanoarchitectonics are crucial aspect of medicinal chemistry, converging the selection, synthesis, and development of novel compounds tailored for therapeutic applications in treating various pathological conditions, including cancer, diabetes, and cardiovascular disease.36–42 Advances in inorganic materials chemistry, pharmaceutical, medical science, and nanoscience over the past few decades have led to a variety of changes in the major roles of medicinal chemistry in biomedical technology fields such as drug delivery, bio-imaging, and tissue engineering. In particular, hybrid systems based on medicinal nanoarchitectonics43–45 have been developed into cutting-edge convergence science and technology by being applied to photothermal therapy (PTT), photodynamic therapy (PDT), bio-imaging, and drug delivery, and some of these are successfully entered into pre-clinical and clinical applications as summarized in [Table 1].
Table 1 Latest advancements in inorganic medicinal research field
Hybrid systems |
Characteristics |
Medical applications |
Comments |
Ref. |
Hydrophilic NIC–MgO–HPMC through hybridization of niclosamide (NIC) with MgO followed by coating with hydroxypropyl methylcellulose (HPMC) |
Enhanced intestinal permeability through increasing bioavailability of the hydrophobic drug NIC |
Development of a NIC-based drug delivery system for novel anti-SARS-CoV-2 therapeutics targeting COVID-19 |
The clinical trial findings indicate a fourfold increase in the bioavailability of NIC–MgO–HPMC compared to intact NIC, along with the observation of dose-dependent bioavailability of NIC in phase II clinical trials |
46
|
Hyaluronic acid-hybridizedV2O5 NPs (HA@V2O5 NPs) |
Smart tumour-specific nano theragnostic for postoperative precision theranostics |
Post-operative PTT/chemodynamic therapy (CDT) of residual tumor tissues |
The first example of a combination strategy utilizing receptor mediated targeting (RMT) and GSH-activated in situ transformation of inorganic metal oxides to meet the need of postoperative precision tumor theranostics |
47
|
PEGylated carbon nitride nanosheet@copper-doped polyaniline (CNNS@CuPANI) |
Combined PTT, PDT, CDT |
Osteosarcoma therapy |
Orthotopic spontaneous metastasis osteosarcoma (OS) model as pre-clinical model |
48
|
Nanoceria-loaded magnesium aluminum layered double hydroxide (LDH-CeO2) |
Elevated antioxidation effect of CeO2via neutralizing excessive H+ in rheumatoid arthritis (RA) microenvironment |
Treating RA using a nano-catalytic platform |
Significant elevation of osteoblast viability and osteogenesis by the inhibited expressions of inflammatory cytokines and autoimmune antibody, and of the released Mg ion, resulting in the profound healing of bone tissues in adjuvant-induced RA model mice |
49
|
Ceria nanoclusters |
Polymer-coated ceria nanocluster |
Reactive oxygen species (ROS) scavenging effect |
Can effectively inhibit oxidative stress, inflammation, renal fibrosis, and apoptosis in the CKD (Chronic kidney disease) mice model |
50
|
Manganese doped mesoporous hollow ceria nanoparticles (MnOx–CeO2 NPs) |
Ability to scavenge ROS |
Prevention of hepatic ischemic reperfusion injury |
Multifunctional inorganic nanohybrid system for hepatic Ischemia Reperfusion Injury in mouse model |
51
|
The conjugation of Doxorubicin (Dox)-loaded, aspartic acid-functionalized Au NPs (Au NPs) with TAT peptide |
Developed chemotherapeutic system for efficient delivery of potent anti-cancer drug doxorubicin |
Highly efficient and biocompatible drug delivery systems for cancer therapeutics |
Cytotoxic efficiency of the chemotherapeutic system increased twice as compared to the free Dox in HeLa cell |
52
|
Curcumin (CRC) loaded exfoliated LDH NPs |
∼100 nm size hybrid NPs with improved efficacy and stability |
Sustained curcumin delivery system for lung cancer |
Improved results in anticancer effects in lung cancer xenograft model |
53
|
Pemetrexed (PMX) intercalated into layered double hydroxide (LDH) and coated with Eudragit® S100 (S100), an enteric coating agent |
Enhanced PMX bioavailability and protection against gastric degradation |
Development of orally administrable anticancer drug delivery system |
Significant tumor volume inhibition in A549 tumor mouse model by orally administered PMX-LDH-S100, suppressing tumor volume by 64% and 51% compared to PBS and PMX samples |
54
|
N-IL-12/DOX (doxorubicin)/α-TOS (α-tocopheryl succinate) |
The encapsulation of DOX, IL-12, and α-TOS resulted in a mean size of 275 nm with loading contents of 2.04 × 104, 4.01 × 102, and 7.12 × 102, respectively |
The combination of chemotherapy and local macrophage modulation-immunotherapy as a promising strategy for anti-tumor therapy |
The targeted release and therapeutic impact of the N-IL-12/DOX/α-TOS system, including its enhancement of anti-tumor efficacy, immune response augmentation through tumor-associated macrophage polarization into M1 phenotypes, reduction in lung metastasis, and minimization of side effects |
55
|
PEG-gated large pore-sized mesoporous silica NPs for the Bcl-2-functional-converting peptide (denoted as N9@M-CA∼8P) with celastrol integration |
Controlled release and realizing synergistic effects with celastrol integration at a low dosage as a curative sensitizer |
Combination of chemotherapeutics with polypeptide/protein drugs |
N9@M-CA∼8P nanosystems with celastrol resulted in biosafety and enhanced therapeutic outcomes (90% tumor inhibition) inducing mitochondrion-mediated cell apoptosis in resistant cancer cell lines and in corresponding xenografted mice models |
56
|
ROS-responsive AuNP-based nanosystem in which azide-decorated AuNPs (N3@AuNPs) and diselenide-coated alkyne-decorated AuNPs (Se/Ak@AuNPs) |
In tumor areas with high ROS due to numerous factors, diselenide linkers on AuNPs are cleaved, releasing PEG chains and triggering a click reaction with nearby azide groups |
Potential tool for PTT enhancement in cancer treatment |
Enhancement of photothermal conversion efficiency in large clusters of AuNPs versus isolated AuNPs under 808 nm laser irradiation; significant increase in apoptosis rate in AuNP clusters compared to individual AuNPs in vitro studies |
57
|
DOX/Au-loaded Fe3O4 (DAF) hybrid nanospheres |
Multifunctional nanomedicine accumulation at tumor sites under an external magnet, followed by magnetic resonance imaging-guided synergistic chemo-photothermal therapy |
Mesoporous Fe3O4 nanospheres as a platform for multifunctional biodegradable nanomedicine |
The effectiveness of NIR laser irradiation in cancer treatment through simultaneous drug and heat release, achieving over 90% tumour ablation, followed by the breakdown of DAF hybrid nanospheres into Fe3O4 and Au NPs for elimination from the body |
58
|
In this review, an accessible discussion of research findings across various scientific disciplines, including inorganic chemistry, nanomaterials science, and medicinal chemistry, with a focus on their functional applications in medicine have been elaborated. Additionally, a historical perspective on the evolution of inorganic medicinal chemistry, connecting it to recent advancements in biomedical technology and clinical trials are discussed. Furthermore, we delve into the toxicity and long-term efficacy of new medicinal nanoarchitectonics, highlighting insights from both preclinical and clinical studies. Unlike the earlier days of drug development, which were driven by small research groups, the field of inorganic drug chemistry has evolved significantly with the introduction of combinatorial chemistry, high-throughput screening, and structure-based drug design. The synthesis of drug-like compounds, alongside in vitro screening for toxicity and pharmacokinetics, has become essential in modern inorganic medicinal chemistry. For continued progress, it is crucial to support a range of in vivo experiments at the preclinical stage and to ensure the transfer of knowledge from experienced pharmaceutical chemists to the next generation of researchers. Additionally, recent advances in the biomedical field—spanning tissue engineering, drug delivery, bio-imaging, and theranostics—have been accelerated by research into organic–inorganic hybrid materials. While many inorganic materials are not yet commercialized despite their biocompatibility, biodegradability, and potential for biochemical functionalization, they are expected to soon enter the biopharmaceutical market, gaining increasing attention in recent years [Fig. 1].
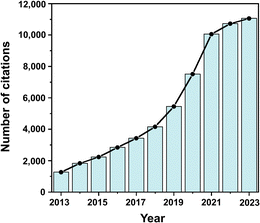 |
| Fig. 1 PubMed indexed publications over 20 years on inorganic nanomedicine. | |
2. Historical foundations of medicinal nanoarchitectonics
“Medicinal nanoarchitectonics” refers to the design and engineering of nanoscale structures (with a size of around 100 nm, but this can vary depending on the application and the type of nanomedicine required) and systems specifically for medical applications. This field combines principles from nanotechnology, chemistry, physics and biochemistry [Fig. 2].59
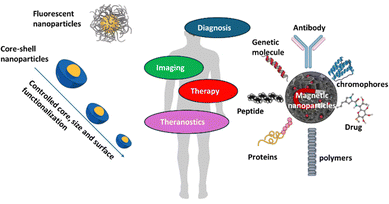 |
| Fig. 2 Use of key properties and structures of nanoparticles (NPs) in medicinal nanoarchitectonics which combines diagnosis, imaging and therapy. | |
The convergence of nanotechnology with its research fields and various applications has recently become the subject of interest from the scientific community. In the late 19th century, Michael Faraday was the first to scientifically demonstrate the optical properties of metallic NPs by synthesizing AuNPs through the reduction of gold chloride.60 The development of medicinal nanoarchitectonics is rooted in early breakthroughs, such as Michael Faraday's work in the 19th century on the optical properties of metallic NPs and Richard Feynman's 1959 lecture entitled “There is plenty of room at the bottom”. Not only did it open up the endless possibilities of the nanoworld, but it also laid the foundations for modern nanomedicine,61 and today's “nanotechnology” was restarted 40 years later with U.S. President Clinton's National Nanotechnology Initiative in 2000.62 Later, with the discovery of fullerene and carbon nanotubes, nanotechnology was actively developed in the 1980s and 1990s.63,64 Especially, the rapid development of nanotechnology and the convergence of medical science have led to the emergence of nanomedicine and medicinal nanoarchitectonics for the treatment of various diseases, including cancer.65–68 Therefore, medicinal nanoarchitectonics research can contribute to the innovative improvement of patients’ health in the fields of biomedical technology and pharmaceuticals using nanotechnology.69–71 Over the past few years, nanomedicines have shown unlimited potential to have far-reaching implications for human health and management, such as prevention of various diseases, early detection, diagnosis, treatment.
Another important medicinal nanoarchitectonics research began with the discovery of superparamagnetic iron oxide nanoparticles (SPIONs), which are now widely applied in the field of magnetic resonance imaging (MRI).72 Various types of iron oxide NPs are used as inorganic materials for MRI, among which magnetite (Fe3O4) is one of the most promising nanomedicines that has already been proven to be biocompatible.
Similarly, Barnett Rosenberg's discovery of the metal drug cisplatin in 196573 led to a higher level of development in the field of inorganic medicinal nanoarchitectonics, and various cisplatin derivatives such as carboplatin, oxaliplatin, heptaplatin have since been reported as new anticancer agents.
3. Inorganic medicinal nanoarchitectonics
Inorganic nanoarchitectonics involves creating nanostructured inorganic materials with precise functions enabled by controlled physico-chemical properties. These materials, which include metals, metal oxides, and other inorganic compounds, are engineered at the nanoscale to exhibit specific properties such as enhanced magnetism, optical characteristics, or catalytic activity making them highly suitable for medical applications. For example, SPIONs are widely used in MRI imaging, while metal-based nanodrugs like cisplatin derivatives are crucial in cancer treatment. Inorganic nanoarchitectonics holds promise for advancing diagnostic, imaging, and therapeutic technologies in medicine.
Among inorganic NPs such as metals and semiconductors, semiconductor nanocrystals are known as quantum dots (QDs), while metal NPs such as gold and silver NPs are used extensively in medicinal nanoarchitectonics research.74–80
As is well known, in the case of metals such as Au and silver and semiconductors such as CdSe, when the particle size is controlled to a few nm or tens of nm in size, they exhibit completely different physical properties from their original bulk properties. For example, Au NPs are no longer bright yellow, the color of jewelry or coins, but exhibit optical properties that can be red, orange, yellow, green, blue, indigo, purple, and even black, depending on the size and absorption of the particles. Semiconductors such as CdSe or CdTe are black in bulk size, but can be yellow, orange, or red when they are smaller to nanoscale.81 This phenomenon arises due to the quantum confinement effect, which become prominent as particle sizes approach the nanoscale. The quantum confinement effect alters energy levels within the material, leading to changes in optical and electronic properties.82 In theranostic applications, this size-dependent quantum effect allows NPs to exhibit enhanced imaging contrast and targeted therapeutic efficiency, making them valuable in biomedical applications requiring precision (Table 2).
Table 2 Summarizing the effects of quantum confinement and its impact on theranostics applications in medicinal nanoarchitectonics
Quantum confinement effects |
Description |
Examples with particle size |
Theranostic applications |
Ref. |
Enhanced optical properties |
Quantum confinement in NPs leads to size-dependent fluorescence, with particles emitting specific wavelengths based on size |
Quantum dots (QD) – size: 2–10 nm (e.g., CdSe QDs, ZnS QDs) |
Enables precise imaging in biological systems, multiplexed imaging for diagnostic clarity, and real-time tracking of drug delivery |
83–85
|
Improved drug delivery |
Increased surface area in quantum-confined structures allows for higher drug loading and controlled release, which is essential in targeted therapies |
Mesoporous silica NPs – size: 50–100 nm; gold nanorods – size: 10–50 nm (dependent on aspect ratio) |
Controlled, pH-triggered drug release, responsive drug delivery systems targeting cancer cells with reduced off-target effects |
86–96
|
Magnetic and photothermal effects |
Quantum confinement enhances magnetic properties and photothermal response in materials like iron oxide, useful for imaging and therapy |
SPIONs – size: 10–20 nm; gold nanoshells – size: 30–100 nm, tuned for near-infrared absorption |
Used in MRI contrast enhancement, hyperthermia for cancer treatment, and dual imaging-therapy applications in cancer theranostics |
97–104
|
Reduced toxicity and enhanced biocompatibility |
Nano-scaling allows for fine-tuning of size and surface properties, which can reduce toxicity and enhance compatibility with biological tissues |
Silica-coated quantum dots – size: 5–15 nm; PEG-coated Au NPs – size: 5–20 nm |
Suitable for in vivo applications, enhancing patient safety, reducing immune response, and providing safer options for imaging and therapy |
105 and 106
|
Increased chemical reactivity |
Smaller size leads to higher surface reactivity, facilitating better interactions with biological targets like cancer cells. |
Silver nanoparticles – size: 10–50 nm; cerium oxide NPs – size: 2–10 nm |
Effective for antibacterial and anticancer applications, scavenging ROS, and increasing therapeutic action and sensitivity in diagnosis |
107 and 108
|
Inorganic NPs at specific sizes leverage these quantum effects to improve their performance in various therapeutic and diagnostic applications. For instance, small-sized Au NPs demonstrate increased absorption in the near-infrared (NIR) region, making them especially effective in PTT for tumor ablation by enabling precise heat generation upon light exposure.109,110 Additionally, QDs (e.g., CdSe, CdTe) possess excellent fluorescence properties due to these quantum effects, which make them suitable for high-resolution imaging applications. This enhanced optical contrast and tunable fluorescence capability allow QDs to be applied effectively in fluorescence-based diagnostic imaging, providing detailed visualization at the cellular and molecular levels.111
Furthermore, careful control of nanoparticle size is essential to maximize these quantum effects and improve therapeutic and diagnostic efficiency. Adjusting the size of inorganic NPs allows researchers to optimize their interaction with biological tissues, thus enhancing their specificity and efficacy. For example, NPs within a specific size range (e.g., 10–100 nm) are optimal for tumor targeting through the enhanced permeability and retention (EPR) effect, which can play a crucial role in improving both imaging and therapeutic outcomes in theranostic applications.112
In addition, many researchers have recently reported the synthesis and physicochemical properties of nano systems that integrating inorganic nanomaterials and biology, such as inorganic–organic hybrid NPs, magnetic nanocrystals and QDs as medicinal nanoarchitectonics. Further, great advances have been made in the synthesis of various NPs using metals, metal oxides, semiconductors, polymers, organic and biomolecules, their chemical stability, their passivating through surface reactions, and methods of functionalization of surfaces.113
Inorganic nanomaterials are not only used in the development of drugs and drug delivery systems, but also in the discovery of biomarkers and molecular diagnostic systems, which is the basis for current medicinal nanoarchitectonics. They can be designed to enable targeted therapy into disease-causing tissues, to regulate the continuous release of therapeutic drugs, and to enable multiple routes of administration. Based on the above advantages, inorganic nanomaterials are being applied to drug delivery and diagnostic technologies, biomarker discovery, and regenerative medicine. Recently, MgO based hybrid NPs that can be used as oral treatment for COVID-19 have been reported,46,114 and are on the approval for emergency use from the Korea Centers for Disease Control and Prevention and the Ministry of Food and Drug Safety as a result of successful Phase 2 clinical trials.
4. Inorganic medicinal nanoarchitectonics for diagnosis
With the advancement of analytical technology, the abilities of inorganic nanomaterials have been also improved, making it possible to use them as nano-imaging agents at the cellular level.115–117 Inorganic nano-imaging preparations are used in a variety of imaging techniques, including fluorescence emission scanning microscopy, resonant Raman spectroscopy, fluorescence Raman microscopy, rayleigh light-scattering microscopy, MRI, computed tomographic (CT) imaging, and X-ray absorption [Fig. 3].
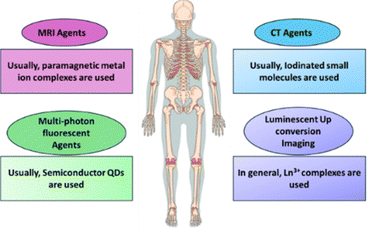 |
| Fig. 3 Various inorganic medicinal nanoarchitectonics for bio-imaging techniques. | |
For example, SPIONs have received a lot of attention as clinical MRI imaging agents for tracking cell pathways.118–120 The basic working principle of SPIONS in MRI is shown in Fig. 4.121 SPION effectively labels green fluorescent protein (GFP) cells, which has no significant impact on cell function and GFP expression. The use of MRI to track GFP, a genetic marker in cells, uptaken by SPIONS, suggests that the technology is effective in monitoring the spatiotemporal movement of cells for understanding cell-based and gene-based therapeutic processes.122 Previously, neural stem cells were labeled with SPIONS and followed by MRI after transplantation in vitro and in vivo phases.123 Recently, SPIONS have been used to label hematopoietic stem cells and progenitor cells, helping to understand their location and transplantation process.124 Cell tracking technology through isotope fluorine-19 (19F), a similar formulation, is known, and it has been reported that it has excellent cellular compatibility as well as a very large degree of cell uptake due to improved selectivity for cells. It has been also demonstrated that fluorine (19F) MRI can serve as a powerful tool for medical imaging by providing unique and highly sensitive cell markers. These markers, created by labeling cells with distinct types of liquid perfluorocarbon (PFC) NPs, enable precise tracking and visualization of cells against any tissue background, offering a significant advancement for cell-based therapies and diagnostic imaging. This approach holds potential in medical applications such as monitoring immune cells in inflammatory diseases, tracking therapeutic cells in regenerative medicine, and enhancing diagnostic accuracy in oncology.125
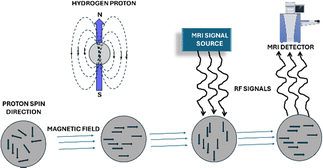 |
| Fig. 4 Schematic diagram of magnetic resonance imaging (MRI) signal processing (This figure has been adapted from ref. 121 with permission from MDPI, copyright 2020). | |
In addition, as a potential tool for the fast and accurate diagnosis of various types of cancer and other lesions, colloidal fluorescence semiconductor QDs have been widely used as agents with high luminescent effect, expanding the application possibilities of fluorescence microscopy.126 Previous studies have demonstrated that QDs with a layered semiconductor monolayer with the potential for resonant Raman spectroscopy from the vibration mode of QDs.127,128 Recent reports indicate that QDs and SPION are currently being used as effective diagnostic and therapeutic agents, and this convergent approach enables the development of personalized nanomedicines with guaranteed safety by diagnosing the dynamics, localization, and engraftment of transplanted stem cells in vivo. However, current technology has limitations in properly imaging and detecting transplanted stem cells, and a new hybrid imaging system has been developed to address this. The system consists of lipid molecules containing dendron (molecules containing two unsaturated bonds (DLU2)) and QDs, and is composed of magnetic NPs.129
As the aforementioned Au NPs have been proven to be excellent imaging agents in the living system, hyper-Raman scattering results have already been reported in biological structures after culturing with Au NPs in cells.130,131 Since then, there have been many studies on the application of Au NPs as other imaging agents to broaden the field of application.132 However, recent research is not just imaging, but is being developed in the direction of convergence of therapeutic and imaging technologies. For example, a nanogel hybridized with Au NPs and chitosan has been synthesized, which has been proposed as a theranostic nanoplatform capable of tumor CT imaging and chemotherapy. The hybrids of Au NPs and chitosan were first cross-linked with tripolyphosphate (TPP) and then modified with poly acrylic acid (PAA). The synthesized hybrid nanogel is an EDC/NHS (1-ethyl-3-(3-dimethylamino)propyl carbodiimide/hydroxysuccinimide) coupling reaction that creates an amide bond between the Au NPs and chitosan/TPP/PAA to functionalize cysteine in Au NPs. As a result of testing this in a squamous cell carcinoma tumor model, it was proved that it is a dual-functional system that is endowed with the function of delivering the anticancer drug doxorubicin (DOX) and CT imaging.133
Immunocytochemistry and flow cytometry, which are existing cell protein detection analysis techniques, require a large number of cells, and have various limitations, such as difficulty in obtaining sufficient cell mass, high analysis costs, or blockage in the nozzle of the flow cytometry due to technical problems. In addition, it was difficult to simultaneously cellular protein imaging and quantification from a single experiment. To address these challenges, an innovative 3D platform has been developed that combines an alginate hydrogel droplet array loaded into biofriendly cells with Au NPs-based metal enhanced fluorescence (MEF).
Simultaneous imaging and quantification of highly sensitive intracellular proteins have been successfully achieved. Unlike traditional 2D immunocytochemistry, the 3D approach significantly enhances the loading of Au NPs into cells, increasing both the quantity and distribution of NPs. This improvement boosts metal-enhanced fluorescence (MEF) signaling, allowing for precise protein quantification. Moreover, this 3D platform enables single-cell protein imaging, providing an advantage over conventional flow cytometry by allowing detailed visualization and analysis at the individual cell level.134
Similarly, plasmonic NPs have been utilized in plasmonic photothermal therapy (PPTT) due to their excellent light absorption properties. Au NPs are well-known to be able to modulate photothermal properties and tissue targeting by synthesis methods, making them suitable therapeutic candidates for PPTT. In previous studies, a PPTT method using gold nanospheres and gold nanorods and silica–gold nanoshells were proposed for visible light lasers.135 Au NPs incorporating temperature-sensitive polymers have been effectively applied in the field of medical technology.136
On the other hand, many newly developed multifunctional inorganic medicinal nanoarchitectonics are being used more actively in the field of theranostic, taking advantage of the advantages of nanometer size and surface chemistry. In vitro, the preferential uptake of 18 nm and 80 nm gold nanoparticles (AuNPs) by SKOV3 ovarian cancer cells, which exhibited four times higher uptake compared to OVCAR5 cells. Quantification of nanoparticle internalization was performed using inductively coupled plasma (ICP) analysis and MARS spectral photon-counting CT imaging (MARS SPCC) imaging. In this study, in vitro, Au NPs (18 nm) and surface-modified Au NPs (18 nm) were selected as contrast agents for the MARS SPCCT, and FTIR spectroscopy confirmed the binding of the Au NPs surface to luteinizing hormone-releasing hormone (LHRH). For the first time, this was an example of the application of surface modified Au NPs with LHRH to target selective ovarian cancer in vitro and in vivo. Inductively coupled plasma-mass spectrometer (ICP-MS) analysis confirmed preferential uptake of LHRH modified AuNPs by organs residing in the abdominal cavity with OC nodules (pancreas: 0.46 ng mg−1, mesentery: 0.89 ng mg−1, ovary: 1.43 ng mg−1, and abdominal wall: 2.12 ng mg−1) whereas the MARS SPCCT analysis suggested scattered accumulation of metal around the abdominal cavity. Therefore, Au NPs superficially modified with LHRH can be targeted for ovarian cancer and have shown potential as contrast agents in small intestine CT-imaging technology.137
5. Inorganic medicinal nanoarchitectonics for bioimaging
Functional inorganic nanomaterials have garnered predominant attention in the field of bioimaging due to their unique optical, magnetic, and structural properties. These nanomaterials can aid several advantages over traditional organic dyes and fluorescent proteins, such as enhanced photostability, tunable optical properties, and compatibility with various imaging modalities. Here are some of the latest developments and applications of functional inorganic nanomaterials in bioimaging:
5.1. Upconversion nanoparticles
QDs exhibit exceptional optoelectronic properties but face challenges due to inherent toxicity in biological environments. To address this issue, recent studies are exploring the use of lanthanide-doped upconversion nanoparticles (UCNPs) as an alternative for luminescent optical labeling, offering a safer and effective solution for bioimaging applications [Fig. 5].138,139
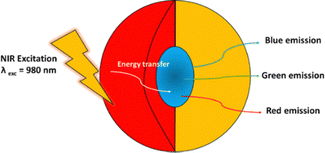 |
| Fig. 5 Lanthanide-doped upconversion nanoparticles (UCNPs) capable of converting near-infrared light into visible and ultraviolet emission. | |
Yttrium oxide (Y2O3) NPs are one of the important heavy rare earth metal oxides.140 The free energy of forming oxides from the element yttrium is the highest known free energy value. Yttrium (Y) is as abundant in nature as copper (Cu), cobalt (Co), and zinc (Zn). Chemically modified Yttrium(III) oxide-based upconverting nanomaterial is a potential imaging platform for in vivo optical-based diagnostic without causing any autofluorescence. In particular, due to their low toxicity, they may be more ideal UCNPs than QDs. Therefore, recently there have been much attention on developing yttrium oxide QDs. These QDs can be synthesized using the wet chemical precipitation method. Thus made monoclinic Yttrium oxide has better photoluminescence properties than that of cubic, and is therefore considered a suitable material for nanophosphors. Yttrium oxide QDs in monoclinic systems can be synthesized at relatively low temperatures (90 °C), and spectroscopy shows strong PL emission in the blue region, so they have potential applications in the display industry. Cytotoxicity tests have revealed that the non-toxic nature, and fluorescence imaging that L6 skeletal cell lines can emit blue fluorescence. This proves that Yttrium oxide QDs can be applied in the field of biomedical technology.141
In other previous research examples, β-NaYF4:Yb,Er UCNPs doped with rare earth elements having strong UC fluorescence were developed as core–shell NPs by coating SiO2 with a thin layer. Afterwards, UCNPs modified with an amino group were covalently bound with CEA8 rabbit antibodies and synthesized as antibody-UCNPs. Antibody-UCNP conjugates are an example of how UCNPs can be used as fluorescence factors for cellular immunolabeling and imaging.142
5.2. QD nanocrystals
QDs are inorganic semiconductor nanocrystals ranging in size from 2 to 8 nm and are rapidly emerging as a new type of fluorescent labeling technology capable of higher brightness, quantum yield, and multicolor fluorescence emission.143 In general, QDs are composed of atoms from group 2 and group 4 elements (CdSe and CdTe) or group 3 and group 5 elements (InP, InAs). Typically, QDs make up the semiconductor core, which is covered with cap molecules that allow it to dissolve better in an outer shell and aqueous solution to improve optical properties. Among the many QDs, the synthetic method of the material coated with a CdSe core and a layer of zinc sulfide (ZnS) is well known. These QDs are highly fluorescent, have high quantum yields, large absorption cross-sectional areas, and high photostability.144 Over the past few decades, various strategies have been developed to improve the dispersion of QDs.145–147
For the application of QDs in the field of imaging, various capping agents have been used to improve dispersibility in aqueous solutions. One of the most widely used methods is ligand exchange, which exchanges hydrophobic surfactant ligands with hydrophilic molecules. Many compounds containing thiol, such as mercaptoacetic acid (a derivative of dihydrolipoic acid) or alkylthiol-terminated DNA, thioalkylated oligo-ethyleneglycols, D,L-cysteine, and poly(ethylene glycol[PEG])-terminated dihydrolipoic acid, have high stability in vitro and in vivo.148–150 Encapsulation strategies have been developed to improve the dispersion of QDs using two or three molecule copolymers that are bipolar, phospholipid micelles, silica shells or bipolar polysaccharides, polymer shells, oligomeric phosphine coatings or phytochelatin-peptides coatings, and histidine-rich proteins.151–153 Initial research focused on glutathione-encapsulated CdTe, zinc selenide (ZnSe), and Zn1−xCdxSe QDs with fluorescence (∼360 and 700 nm) in aqueous solution and quantum yields of up to 50%.154 Another study highlights glutathione-encapsulated CdTe QDs combined with biological probes for cell imaging applications.155 QDs for theranostics have been shown to be readily feasible through covalent bonding with bioactive molecules such as peptides, antibodies, nucleotides, and small molecule ligands.156,157
One of the best inorganic material-based optical contrast agents, the first in vivo study of QDs is a cell lineage tracing experiment in frog embryos.158 And then QDs have been studied for cell signaling,159 cancer markers160 and tumor models in animals.161 Recently, low-toxicity QDs have been developed for cell imaging. For example, ZnSe@ZnS QDs doped with Mn which is less toxic, could be combined with nanohydroxyapatite to be useful in cell imaging.162 In fact, similar studies have been reported before, for example, showing that QDs coated with zinc (Zn2+)-dipicolylamin coordination binding complexes are optically detectable living mouse leg infection model.163 The clinical potential of QDs in in vivo has already been identified as a multimodal imaging platform, and is recently being expanded and developed into NIR QDs.164
QDs are physically or chemically modified into a variety of bioactive molecules, expanding their possibilities in drug delivery, bio-imaging, and tissue engineering applications.165–167 By combining these application technologies to various fields, it was possible to maximize efficacy while minimizing toxicity. Recently, systems have been developed that enable drug delivery regulation and bio-imaging through QDs and nanogels synthesized by various modification methods. To this aim, a creatinine-functionalized carbon dots (QD) into a lecithin-inulin nanogel was made. Then it was coated with polyethylene glycol (PEG) and herceptin were grafted onto the nanogels, enabling HER2-positive breast cancer targeting, demonstrating excellent biocompatibility and in vivo therapeutic efficacy. In the cellular uptake experiment, this nanogel is effectively internalized in SK-BR-3 cells, and the production of Reactive oxygen species (ROS) is significantly improved. In addition, it has been analyzed that the nanogel complex inhibits the expression of the Bcl-2 gene, which promotes the apoptosis signaling pathway while increasing the expression of the P53 and Bax genes. Improved cellular uptake of herceptin selectively inhibited the proliferation of HER-2-positive SK-BR-3 breast cancer cells when compared to triple-negative MDA-MB-231. The intraperitoneal injection of the developed formulation to MCF-7 breast cancer cell-bearing BALB/c mice supported the observation of tumor growth inhibition. Overall, a herceptin-bound and PEG-coated lecithin-insulin nanogel complex has been shown to be a promising theranostics candidate for the treatment and diagnosis of HER-2-positive breast cancer.168
Thus, QDs with unique optoelectronic properties that make them highly effective for imaging in medical applications. Their broad excitation range, narrow emission spectral property, and high photostability enable precise and long-lasting imaging of cells, tissues, and even single molecules, which are especially valuable in diagnostics and tracking therapeutic interventions. Despite concerns over toxicity, advancements like surface modifications and alternative nanoparticles, such as UCNPs, are enhancing their biocompatibility. This makes QDs promising for medical applications like early disease detection, targeted drug delivery, and non-invasive cellular imaging.
5.3. Gold nanoparticles (GNPs)
Au NPs are valuable tools in medical imaging due to their strong surface plasmon resonance, which enables enhanced contrast and sensitivity in various imaging modalities.169–173 Their ability to be easily modified with biomolecules allows for precise targeting of specific cells or tissues, making them highly effective in detecting cancer, monitoring infections, and visualizing inflammation. Au NPs are also biocompatible, making them safer for in vivo applications. Additionally, their optical properties can be tuned for use in photoacoustic imaging, fluorescence enhancement, and even photothermal therapy, providing versatile benefits for both diagnostic and therapeutic purposes in healthcare.
Recent studies have explored the use of GNPs for multimodal imaging, combining techniques such as photoacoustic imaging, CT, and surface-enhanced Raman scattering (SERS) for improved sensitivity and resolution. Recently tryptophan and riboflavin (ATR) functionalized GNPs have been made as a theragnostic system of which, the ATR, the biomolecular pair exhibits significant resonance energy transfer as evidenced through the in vitro studies on Human liver cancer (Huh) cells. The photo bleaching can be modulated by the energy transfer in ATR, thereby allowing the localization of ATR in Huh by fluorescence imaging.174 In another attempt, Hyaluronic acid-functionalized Au NPs were used as a cancer diagnostic probe for targeted bioimaging applications.175 Similarly 15 nm spherical nanoparticles based on nano gold functionalized ruthenium(III)–doxorubicin complex showed efficient theranostics property as well.176
5.4. Iron oxide nanoparticles (IONPs)
Iron oxide nanoparticles are widely used as contrast agents for magnetic resonance imaging (MRI) due to their excellent magnetic properties. Recent developments have focused on surface engineering to enhance their stability, biocompatibility, and targeting capabilities for applications in cancer diagnosis, drug delivery, and stem cell tracking as tabulated in Table 3. These developments highlight the versatile and rapidly advancing field of IONPs for imaging applications, showcasing their potential in enhancing diagnostic precision and therapeutic efficacy.
Table 3 Recent developments on iron oxide for imaging applications
Development |
Description |
Applications |
Key findings |
Ref. |
Multifunctional iron oxide NPs |
Development of NPs with both imaging and therapeutic functions using silica and gelatin hybridization (41 nm size) |
Cancer theranostics |
Demonstrated effective tumor imaging and drug delivery in in vitro |
177
|
Enhanced MRI contrast agents |
Synthesis of ultra-small iron oxide NPs with improved magnetic properties. |
MRI imaging |
Showed higher contrast efficiency and better biocompatibility than traditional agents |
178
|
Iron oxide-gold core–shell nanoparticles |
Creation of NPs combining iron oxide and gold for dual imaging |
MRI and CT imaging |
Provided enhanced contrast in both MRI and CT scans, allowing for more precise diagnosis with BBB permeability |
179
|
Targeted iron oxide NPs |
Surface functionalization with targeting ligands for specific cell types. HER2 aptamer-conjugated iron oxide NPs with PDMAEMA-b-PMPC coating |
Target breast cancer cell identification |
Increased accumulation in tumor tissues, improving imaging accuracy |
180
|
Biodegradable iron oxide NPs |
Development of iron oxide nanoparticles that degrade into non-toxic byproducts. |
Long-term imaging studies |
Showed safe degradation in biological systems, reducing long-term toxicity concerns |
181
|
Hybrid iron oxide NPs |
Integration with other materials like silica, Au and polymers or liposomes for multimodal imaging |
Combined MRI, PET, and optical imaging |
Enabled comprehensive imaging from a single probe, improving diagnostic capabilities |
182
|
Iron oxide NPs for photoacoustic imaging |
Utilization of iron oxide nanoparticles for photoacoustic imaging. |
Vascular and tumor imaging |
Provided high-resolution images of blood vessels and tumors, enhancing non-invasive diagnostics |
183
|
Iron oxide NPs with enhanced permeability and retention (EPR) effect for combined ferroptosis therapy and imaging |
Mesoporous SPIONs (MSPIONs) with an average diameter of 70 nm and pore size of 3.9 nm. Sorafenib (SFN) and/or brequinar (BQR) were loaded into the mesopores of MSPION |
Multimodal theragnosis |
In the tumor microenvironment (TME), the released Fe2+/3+ can produce ˙OH through the Fenton reaction. In the TME, the released SFN can block the cystine/glutamate reverse transporter, reduce intracellular glutathione (GSH) and GSH peroxidase 4 levels, and thus increase ROS and lipid peroxide levels. In the TME, the released BQR can further boost intracellular oxidative stress by inhibiting dihydroorotate dehydrogenase |
184
|
Iron oxide-graphene oxide composites |
Development of composites combining iron oxide with graphene oxide |
MRI and thermal imaging |
Enhanced imaging capabilities and potential for hyperthermia treatment |
185
|
5.5. Silica nanoparticles
Silica nanoparticles offer a versatile platform for bioimaging due to their tunable size, shape, and surface chemistry. Recent advances have focused on functionalizing silica NPs with fluorescent dyes, targeting ligands, and stimuli-responsive moieties for applications such as cellular imaging, biosensing, and theranostics.186–198 Recently, Mn-SMSN@DOX-IR-780 has been developed through the in situ doping of manganese into Mn-SMSN. This innovative nanoplatform combines chemodynamic therapy (CDT) with tumor imaging. The integration is achieved by loading doxorubicin hydrochloride and IR-780 iodide onto the Mn-SMSN structure. Mn-SMSN@DOX-IR-780 enhances the production of cytotoxic ROS, effectively killing tumor cells. The dual functionality of CDT and imaging provides valuable insights for prostate cancer treatment, offering a promising approach for both therapeutic and diagnostic applications.199
New functional nanoarchitectonics based on silica NPs have been reported lately. For example, room-temperature phosphorescence (RTP) presents significant potential in medical imaging due to its persistent afterglow, which remains visible even after the excitation light source is turned off. This quality allows for prolonged imaging times and enhanced signal-to-noise ratios, making it ideal for high-sensitivity, time-gated bioimaging applications. However, traditional RTP materials—whether metal-based or organic—often suffer from poor photostability and toxicity, limiting their use in long-term biological settings. In response, metal- and fluorophore-free silica nanoparticles (SNPs) are developed with stable, long-lived RTP for high-contrast bioimaging. These biphenyl-doped SNPs (bSNPs) are created through polycondensation of silicon precursors and silyl biphenyls, followed by thermal decomposition to form optically active defects within the silicate network. The resulting defect-engineered, calcined bSNPs (C-bSNPs) display long-lived phosphorescence and can be easily functionalized with tumor-targeting peptides. This innovation allows for precise, autofluorescence-free imaging in vivo, enhancing cancer diagnosis and overcoming the limitations of continuous-wave imaging in medical applications.200
5.6. Lanthanide-doped nanomaterials
Lanthanide-doped NPs exhibit unique luminescent properties suitable for bioimaging applications. Recent research has focused on engineering lanthanide-doped nanomaterials with improved stability, brightness, and multiplexing capabilities for high-resolution imaging and sensing in biological system.201,202
Core@multishell NPs are constructed by doping with various luminescent ions. These NPs exhibit visible and NIR-II luminescence when excited by a NIR laser. This feature enables multiple layers of anti-counterfeiting protection. Additionally, latent fingerprints can be developed with background-free fluorescence using these NPs, enhancing their practical applications in security and forensic analysis as reported lately by Sun et al.203
6. Inorganic medicinal nanoarchitectonics for therapeutic applications
Newly proposed inorganic nanomedicines are emerging as promising tools for the development of novel anticancer drugs and vaccines, leveraging both fundamental medical research and the convergence of technologies from diverse fields.56,204 These innovations offer enhanced diagnosis, treatment, and prevention methods across various medical disciplines, including tissue engineering.
6.1. Photoresponsive medicinal nanoarchitectonics
In recent years, with the development of nanomaterials science and photonic technology, PTT and PDT have received a lot of attention.47,48 These two phototherapy methods use light, combined with either external or internal absorbers, to elevate local temperatures or to generate cytotoxic ROS, respectively. With their unique mechanisms, PDT and PTT complement traditional cancer treatments, showing potential to overcome chemotherapy resistance and disrupt compensatory signaling pathways at the cellular level. PTT employs light, often in the near-infrared spectrum, to elevate tissue temperature, resulting in localized photocoagulation. PTT utilizes higher light intensities to attain temperatures either below coagulative levels (43–55 °C) or within coagulative ranges (55–100 °C), leading to swift cell demise through protein denaturation and damage to cell membranes. Mild heating regimens (41–43 °C) fall under hyperthermia, which lacks tumoricidal effects unless prolonged (>1 h) [Fig. 6].205,206 PDT relies on three key elements: light, a light-activatable compound called a photosensitizer (PS), and oxygen. These components undergo a photodynamic reaction when exposed to light, generating cytotoxic ROS. Upon light absorption, a PS molecule transitions from its ground state to an excited singlet state, which then progresses to a more stable excited triplet state. This triplet state can return to the ground state through either type I or type II photodynamic reactions. In type I, the activated PS transfers an electron to a substrate, forming various ROS such as O2˙− and HO˙2. In type II, the energy is transferred directly to ground-state oxygen, resulting in highly reactive singlet oxygen, 1O2. These active entities initiate numerous subsequent biological processes, including direct cell toxicity, inflammation, and vascular responses. Notably, ROS have a very limited diffusion range within cells and tissues (about 50 nm), necessitating the proximity of photosensitizers to the target structure.205–207
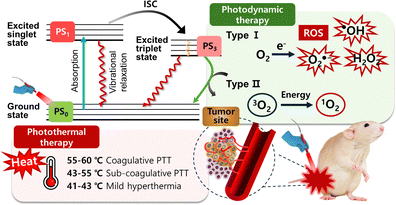 |
| Fig. 6 Mechanism of photothermal therapy and two ROS generation mechanisms (type I and II) for photodynamic therapy. | |
Despite some technical similarities, PDT and PTT differ significantly in clinical implications. PDT achieves greater selectivity by combining targeted light exposure with photosensitizer accumulation and, as a nonthermal method, preserves surrounding collagen structures and nerves. Due to the limited diffusion range of ROS, PDT can target invasive and non-resectable tumor components effectively, although photosensitizer accumulation in the eyes and skin can sometimes cause photosensitivity. In contrast, PTT's selectivity depends on localized light delivery, which creates a temperature gradient and increases the risk of unintended thermal damage. Furthermore, PDT can utilize various light sources, including LEDs and low-power lasers, while PTT requires more expensive, high-energy lasers with advanced cooling and temperature monitoring.
Given the unique demands of PDT and PTT, inorganic nanomaterials offer distinct advantages in enhancing both therapies. Their tunable optical properties and stability make them ideal candidates for use as photosensitizers in PDT and as efficient heat transducers in PTT. For instance, copper (Cu)-based nanomaterials, Au NPs, and QDs exhibit strong absorption in the near-infrared region, enabling precise light activation with minimal off-target effects.109,205,208
For example, a PEGylated carbon nitride nanosheet@copper-doped polyaniline (CNNS@CuPANI) with nano-heterostructure and ROS generation performance from copper was synthesized. Here, nano-heterostructures promote light-induced electron–hole pair separation and doping of Cu2+ has the function of significantly increasing the electron transfer-rate. In addition, the antenna effect of CuPANI enhances the sensitivity of near-infrared light to heterogeneous structures, enabling PDT. Interestingly, the release of doped Cu2+ ions induced a Fenton-like response by reducing glutathione in the tumor microenvironment. PEGylated CNNS@CuPANI, which generates a large amount of ROS, has been used in the treatment of osteosarcoma models with rapid metastasis. In particular, in vivo experiments using orthotopic animal cancer models have shown improvement in treatment effects as well as low lung metastasis rates. In summary, the newly proposed PEGylated CNNS@CuPANI is effective in eliminating osteosarcoma and preventing metastasis, indicating that it is a novel therapeutic strategy for cancer.48
6.2. ROS modulating medicinal nanoarchitectonics
Another example is rheumatoid arthritis (RA), which is a a chronic autoimmune disease featuring an abnormal immune microenvironment and mainly due to the excessive accumulation of ROS [Fig. 7(a)]. Resultantly, inhibiting the inflammatory microenvironment by ROS scavenging is a proper RA treatment. Ceria oxide NPs (denoted as CeO2 NPs) have been widely investigated for their multi-enzyme-like activities mimicking such as superoxide dismutase (SOD) and catalase (CAT), etc. However, excessive hydrogen ions (H+) in RA environments, caused by activated osteoclasts, inhibit the conversion of Ce4+ to Ce3+, thereby diminishing the antioxidative effect of CeO2 NPs. To enhance the ROS scavenging activity of CeO2 NPs, they suggest nanoceria-loaded magnesium aluminium layered double hydroxide (LDH-CeO2), is proposed for enhanced immune modulation based on acid neutralization and metal ion inherent bioactivity. More precisely, the gentle alkalinity of LDH prompts a notable shift in macrophage polarization towards the M2 phenotype, spurred by the heightened antioxidative properties of CeO2, which in turn neutralize excessive H+ levels in the microenvironment of RA. Consequently, this process efficiently attracts regulatory T cells (Tregs) while suppressing the activity of T helper 17 cells (Th17) and plasma cells. Additionally, the release of Mg ions from LDH stimulates osteogenic activity, thus facilitating the healing of damaged bone. The promising therapeutic results observed in mice with adjuvant-induced RA illustrate the strong potential of this treatment approach. It introduces a new and effective therapeutic method for RA, leveraging the immune-modulating and bone-repairing effects of inorganic nanocatalytic materials. However, it is thought that long-term toxicity studies that can occur when accumulated in living tissues are needed in the future for treating RA clinically.49
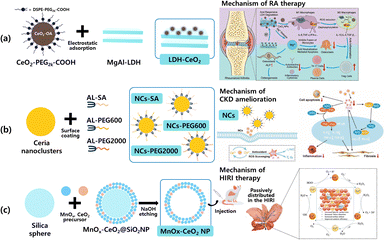 |
| Fig. 7 Illustration of preparation and strategy in ROS scavenging materials against (a) LDH-CeO2 for rheumatoid arthritis (RA), (b) NCs-SA, NCs-PEG600, NCs-PEG2000 for chronic kidney disease (CKD), (c) MnOx–CeO2 NP for ischemia-reperfusion injury (HIRI). (This figure has been adapted from ref. 49–51 with permission from Wiley, copyright 2023; Wiley copyright 2023 and re-drawn from ref. 51 Dove press (CC-BY-NC) copyright 2022). | |
Excessive ROS production plays an important role in the progression of chronic kidney disease (CKD). At present, organic molecules with the ability to scavenge ROS in the treatment of CKD do not meet the requirements for low doses and high efficiency. However, inorganic ceria NPs, which are active and regenerative in scavenging ROS, have been proposed as potential therapies for the treatment of CKD. For this purpose, small size ceria nanoclusters of ≈1.2 nm have been reported.50 The synthesized nanoclusters are modified by three hydrophilic ligands with different molecular weights, including succinic acid (SA), polyethylene glycol diacid 600 (PEG600), and polyethylene glycol diacid 2000 (PEG2000) [Fig. 7(b)].
The surface-modified nanoclusters have a high Ce3+/Ce4+ ratio within the crystal lattice, exhibiting excellent ROS scavenging activity. This shows that when compared to ceria NPs (≈ 45 nm), which have a relatively large size, the nanoclusters showed superior blood concentration-time curves, low organ accumulation, and rapid metabolism. Especially, when nanoclusters with PEG2000 surfaces were administered to mice with CKD, they were able to effectively inhibit oxidative stress, inflammation, renal fibrosis, and apoptosis in their kidney. Nanoclusters with such excellent therapeutic efficacy were able to improve the progression of CKD. Therefore, it was suggested that nanoclusters with the ability to scavenge ROS could become inorganic nanomedicines that can be used for clinical CKD treatment in the future.50 Moreover, hepatic ischemia-reperfusion injury (HIRI) is also primarily caused by excessive ROS accumulation as CKD. It is another example of a liver function disorder that can occur after liver resection or transplantation. To overcome HIRI, ceria NPs (MnOx–CeO2 NPs) with manganese-doped mesopores were proposed which are known to act as reversible reductive antioxidants, have great potential as a therapeutic agent for treating HIRI [Fig. 7(c)].51
Similarly, an ultra-small copper-based multienzyme-like nanoparticles (CMP NPs) with catalase-like and superoxide dismutase-like activities, which effectively scavenge ROS and protect against HIRI was reported. The CMP NPs demonstrated superior therapeutic effects compared to the FDA-approved antioxidant drug N-acetylcysteamine acid (NAC) by suppressing inflammation, preventing hepatocyte apoptosis, and maintaining endothelial function through ROS scavenging.209
6.3. Targeted drug delivery systems
Antibody–drug conjugates are a therapeutic field that is being studied to meet the needs of modern “precision medicine” development. In this study, they developed FMSN-DOX (doxorubicin)-H2-AE01 that is enzyme-sensitive by coating the surface with epidermal growth factor receptor (EGFR) antibody (AE01) and human serum albumin (HSA) on flabellate mesoporous silica NPs (FMSN) containing the drug. HSA and antibodies on the surface of the particles are designed to enhance the biocompatibility of the particles, prevent early drug release, and enable selective biodegradation by matrix metalloproteinase-2 (MMP-2) overexpressed in some tumor tissues. Safety and efficacy of this antibody–drug conjugates were confirmed through cytotoxicity test, about 85–90% of cells were killed by synthesized NPs. In addition, NPs containing antibody-drugs showed different drug release results against cancer cells caused by different MMP-2 and pH. This enzyme-sensitive FMSN-DOX-H2-AE01 hybrid drug shows potential to be developed as a promising therapeutic agent for the treatment of cancer.210
6.4. Anticancer nanotherapeutic agents
Additionally, to overcome limitations in anti-cancer drug delivery with inorganic material, a highly stable gold nanoparticle system using aspartic acid as a capping agent (GNPs) has been reported. Furthermore, the gold nanoparticle system was used for the efficient delivery of the potent anti-cancer drug DOX.52 To increase cellular internalization, GNPs were loaded with the cationic cell penetrating peptide TAT via covalent or non-covalent interactions, forming GNPs-TAT complexes. Another inorganic material, LDH, has been combined with curcumin (CRC) to create a sustained delivery system targeting lung cancer, showing promising improvements in anticancer effects within a lung cancer xenograft model.53 Building on this advancement, the well-known anti-cancer drug Pemetrexed (PMX), which faces challenges such as low bioavailability when administered orally due to its lack of chemical stability in gastrointestinal conditions, has seen a novel solution. To address this major issue, pemetrexed was first intercalated into LDH to form a PMX-LDH nanohybrid. Then, Eudragit® S100 (S100), an enteric coating agent, was functionalized to form a 2D van der Waals nanohybrid drug PMX-LDH-S100, which was aimed to protect the drug from degradation under gastric conditions. Orally administered PMX-LDH-S100 significantly inhibited tumor volume in an A549 tumor mouse model, suppressing tumor volume by 64% and 51% compared to PBS and PMX samples, respectively. Although these studies enhance delivery of anticancer drug, they may face challenges related to side effects from the chemotherapeutic agent in question. The use of chemotherapy invariably brings the risk of unintended and potentially harmful off-target impacts. The diminished immune response of tumors following chemotherapy may lead to tumor spread and advancement. Thus, in order to address the challenge of chemotherapy-induced immune deficiency, a nanosystem comprising hollow mesoporous silica nanoparticles (HMSNs) interconnected by thioketal (TK) bonds, and enveloped with carboxymethyl chitin (CMCH) through electrostatic attraction, along with surface-modified glucose-regulated protein 78 binding peptide, was formulated for the encapsulation of DOX, interleukin-12 (IL-12), and α-tocopheryl succinate (α-TOS).55 HMSNs were utilized to address the limitations associated with the chemotherapeutic drug, DOX, including poor solubility, lack of specificity, and noticeable side effects, as well as to leverage their advantages of biocompatibility, high specific surface area, and facile functionalization. N-IL-12/DOX/α-TOS was constructed for loading IL-12, DOX and α-TOS to enhance chemotherapy, and simultaneously promoted TAM polarization into tumoricidal M1 phenotypes to trigger immune response N-IL-12/DOX/α-TOS enabled local release of IL-12, DOX and α-TOS through pH and H2O2 triggering in the tumor microenvironment (TME). Furthermore, the joint treatment involving N-IL-12/DOX/α-TOS significantly enhanced the effectiveness of anti-tumor therapy, boosted immune responses by fostering the transformation of tumor-associated macrophages (TAMs) into tumoricidal M1 phenotypes, and reduced lung metastasis while minimizing side effects. N-IL-12/DOX/α-TOS emerged as a promising approach for merging chemotherapy with localized macrophage modulation-based immunotherapy in anti-tumor treatment.55 Similarly, the combination of chemotherapy and polypeptide/protein pharmacology has been proposed as an effective treatment option to overcome cancer multidrug resistance (MDR). However, due to the low biostability and low cell permeability of biomacromolecules, it is not easy to control the drug delivery location and release time at the target site in vivo, and it was found that the expected synergistic effect was not achieved with a simple drug combination. In order to achieve the payload of Bcl-2 function-transforming peptide (denoted as N9@M-CA∼8P) drug, multiarm PEG-coated mesoporous NPs were synthesized, and release regulation and synergistic effects were induced through fusion with celastrol which is a low-dose therapeutic sensitizer to overcome the above limitations [Fig. 8]. These results demonstrate that N9 peptide is released in large pores of the M-CA∼8P nanosystem, depending on the pH in the biomimicry environment and in cancer cells and tumor sites. Fusion of N9@M-CA∼8P nanosystems with celastrol induced mitochondria-mediated apoptosis in in vitro and in vivo mouse models in which cancer cells were transplanted, resulting in biosafety and improved therapeutic effects (90% tumor suppression rate). This study demonstrates the potential of an effective and safe resistant cancer treatment that combines stimuli-responsive biomolecular nanosystems with low-dose natural compounds.56
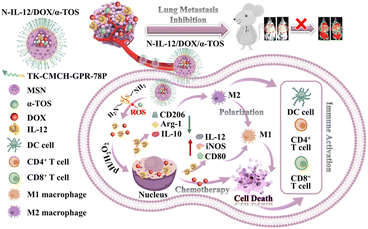 |
| Fig. 8 Schematic diagram of anti-tumor therapy and inhibition of metastasis by N-IL-12/DOX/α-TOS through enhanced chemotherapy and TAM polarization. (This figure has been adapted from ref. 55 with permission from Elsevier, copyright 2024). | |
6.5. Multifunctional therapeutic materials
Various types of metal and semiconductor nanocrystals are actively used in phototherapy. Phototherapy is divided into two types: PTT and PDT. PTT is a method of using heat emitted from a PTT agent in the response of absorption of near-infrared wavelengths for treatment, and PDT is a method of killing pathogens related to specific malignant tumors through a PDT agent that produces ROS with therapeutic effects.
New inorganic nanohybrid NPs have been developed as PTT agents for effective PTT of tumors. This is a ROS reactive nanosystem based on Au NPs, a model system in which the surface of Au NPs is modified with azide, coated with diselenide so that the nanocluster can exhibit a selective clicking into nanoclusters when exposed to ROS, and Au NPs modified with alkyne (Se/Ak@AuNPs) were also comparatively analyzed. Se/Ak@AuNP is designed and synthesized so that the alkyne component and the diselenide linker are contained in a long chain of polyethylene glycol (PEG), so that the alkyne component cannot access the azide component of the N3@AuNPs due to steric hinderance. When the concentration of ROS increased due to rapid metabolic rate, cellular response signaling, mitochondrial dysfunction, and activity of oncogene, the binding of diselenides in the tumor site was degraded, the long PEG chain bound to the Au NPs was loosened, and the surrounding azide induced a selective click reaction by the alkyne component. Clicked Au NPs form larger cluster NPs, and 808 nm laser irradiation has shown that these Au NPs-clusters have significantly improved photothermal conversion efficiency when compared to Au NPs. In vitro experiments, Au NPs-clusters showed higher apoptosis than Au NPs. Therefore, Au NPs-clusters clicked with ROS reactivity have been proven to be excellent PTT agent with excellent cancer treatment effects.57 Another study utilized inorganic photothermal therapeutic Au NPs and organic chemotherapeutic doxorubicin hydrochloride successfully loaded onto Fe3O4 for cancer imaging and therapy. A multifunctional nanomedicine, combining Au and Fe3O4, demonstrated effectiveness in treating tumors through both photothermal therapy and chemotherapy when exposed to near-infrared light. Additionally, this nanomedicine exhibited improved biodegradability in the acidic tumor microenvironment, enhanced T2-weighted MRI contrast, and magnetic targeting capabilities.58 Most of the various types of medical NPs that have already been developed are organic NPs, such as polymers including liposomes, and although inorganic NPs are very limited, they have enabled innovative applications in the health and medical fields211,212 Some of them have already been commercialized in the market, and there is still a great need in the medical market.
Table 4 FDA-approved inorganic nanomedicinal technology-based products
Product name |
Material composition |
Nanoparticles (NPs) advantage |
Indications |
FDA approval year |
INFeD®(Sanofi Avertis) |
Iron dextran (low MW) |
Allows increased dosage |
Iron deficiency in chronic kidney disease (CKD) |
1957 |
DexIron®/Dexferrum®(Sanofi Avertis) |
Iron dextran (low MW) |
Allows increased dosage |
Iron deficiency in chronic kidney disease (CKD) |
1957 |
Feridex®/Endorem®(AMAG pharmaceuticals) |
SPION coated with dextran |
Superparamagnetic properties |
Imaging agent |
1996 (2008)* |
Ferrlecit®(Sanofi Avertis) |
Sodium ferric gluconate |
Allows increased dosage |
Iron deficiency in chronic kidney disease (CKD) |
1999 |
Venofer®(Luitpold pharmaceuticals) |
Iron sucrose |
Allows increased dosage |
Iron deficiency in chronic kidney disease (CKD) |
2000 |
GastroMARK™; umirem®(AMAG pharmaceuticals) |
SPION coated with silicone |
Superparamagnetic properties |
Imaging agent |
2001 (2009)* |
OsSatura®(IsoTis Orthobiologics) |
Hydroxyapatite |
Mimic the structure of bone, facilitating cell attachment and proliferation |
Bone substitute |
2003 |
Vitoss®(Stryker) |
Calcium phosphate |
Mimic the structure of bone, facilitating cell attachment and proliferation |
Bone substitute |
2003 |
Ostim®(Heraseus Kulzer) |
Hydroxyapatite |
Mimic the structure of bone, facilitating cell attachment and proliferation |
Bone substitute |
2004 |
NanOss®(Rti surgical) |
Hydroxyapatite |
Mimic the structure of bone, facilitating cell attachment and proliferation |
Bone substitute |
2005 |
EquivaBone®(Zimmer Biomet) |
Hydroxyapatite |
Mimic the structure of bone |
Bone substitute |
2009 |
Feraheme™/ferumoxytol (AMAG pharmaceuticals) |
Ferumoxytol SPION with polyglucose sorbitol carboxymethylether |
Long-term sustained release with magnetite suspension, reduced dosage |
Deficiency anemia iron in chronic kidney disease (CKD) |
2009 |
Inorganic and metallic NPs Nanotherm®(MagForce) |
Iron oxide |
Cell uptake and superparamagnetism |
Glioblastoma |
2010 |
Hensify (NBTXR3) |
Hafnium oxide nanoparticles used for enhancing radiotherapy in the treatment of soft tissue sarcoma. |
Enhanced radio therapy via targeted approach |
Sarcoma |
2020 (fast track designation) |
Gadopiclenol (Elucirem) |
Paramagnetic macrocyclic non-ionic complex of gadolinium |
Contrast agents used in combination with magnetic resonance imaging (MRI) to detect and visualize lesions with abnormal vascularity in the central nervous system and body |
Contrast agent |
(2022) |
Flyrcado (flurpiridaz F 18) |
Flurpiridaz F 18 is a radioactive compound based on the fluorine-18 isotope. |
PET Tracer flyrcado to improve heart diagnosis |
PET agent |
2024 |
7. Examples of existing functional inorganic nanomaterials approved by the FDA
Most of the various types of medical NPs that have already been developed are organic NPs, such as polymers including liposomes, and although inorganic NPs are very limited, they have enabled innovative applications in the health and medical fields211,212 Some of them have already been commercialized in the market, and there is still a great need in the medical market. Table 4 shows FDA-approved products that use only inorganic nanocarriers or are hybrids with polymers such as dextran for bone implants and regenerative nanomedicine.
8. Metabolization of inorganic medicinal nano architectonics
Metabolization and metabolism are closely related terms, often used in the context of how substances are processed in biological systems, but they have distinct meanings: While metabolism refers to all the biochemical reactions that occur within a living organism to sustain life. It includes two main processes such as catabolism (Breaking down molecules to release energy) and anabolism (Building complex molecules from simpler ones for growth and repair). o-Metabolic processes cover everything from energy production to waste elimination and are essential for cellular function, growth, and response to the environment.233–240
In the case of metabolization, which specifically refers to the process by which a substance (often a drug or foreign compound) is broken down and converted by the body's metabolic systems. It is commonly used in pharmacology to describe how a drug or nanoparticle is transformed in the body, including how it is absorbed, distributed, modified (often by the liver), and eventually excreted.241–248
The metabolization of a substance can impact its bioavailability, effectiveness, and safety profile, especially in drug development and nanomedicine. In essence, metabolization is a part of metabolism, typically describing how the body handles external compounds (xenobiotics), whereas metabolism is a broader term that encompasses all chemical reactions in living organisms.
The metabolization of inorganic nanostructures has become an important area of study, especially as these nanomaterials are increasingly used in biomedical applications. Researchers are investigating how inorganic NPs are processed, distributed, and cleared by the body, with a focus on understanding the interactions between NPs and biological systems to improve their safety and efficacy.
8.1. Biodegradation and biotransformation
Certain inorganic nanostructures, such as silica and IONPs, can partially biodegrade in physiological environments.249–251 This degradation can be influenced by factors like particle size, surface modifications, and the body's pH. For example, IONPs can undergo oxidation and reduction reactions in tissues, eventually breaking down into non-toxic iron ions that integrate with the body's iron stores. Silica NPs can dissolve into silicic acid, which is excreted through urine. The rate and extent of degradation are highly dependent on the NP's coating and surface functionalization, which can be tuned for controlled degradation rates.
Functionalizing the surface of NPs with biocompatible polymers, such as polyethylene glycol (PEG), helps to improve circulation time by preventing rapid uptake by the immune system.252 PEGylation, for instance, reduces protein binding on nanoparticle surfaces, minimizing recognition by macrophages and extending nanoparticle retention time. This extended circulation allows for enhanced therapeutic effect but requires careful balance, as excessive PEGylation can hinder cellular uptake for targeted therapies.
8.2. Biodistribution and retention
Inorganic NPs often accumulate in organs like the liver, spleen, and lungs due to their high vascularization and the filtering action of macrophages in these organs. Recent studies have shown that by altering particle size and surface charge, researchers can control nanoparticle distribution. Small particles (<5 nm) can be rapidly cleared via renal excretion, whereas larger particles are typically taken up by the liver and spleen. Tailoring these properties enables selective targeting and reduces potential toxicity from prolonged tissue retention.253,254
8.3. Bio-persistence
Some inorganic NPs, such as gold or titanium dioxide, are considered bio-persistent, meaning they do not readily degrade in the body.255,256 This persistence can raise concerns about long-term toxicity, especially if NPs accumulate in vital organs. Strategies to mitigate this include using biodegradable coatings or designing nanocomposites with mixed materials that allow for gradual degradation and excretion. Recent trends also involve exploring biocompatible coatings that improve cellular uptake and degradation pathways.
8.4. Enzyme-mediated breakdown
In the case of enzyme-responsive nanostructures, where NPs are engineered to degrade in response to specific enzymes present in target tissues or disease environments.257–261 This approach is particularly relevant for tumor-targeting applications, as enzymes like matrix metalloproteinases (MMPs) are overexpressed in many tumors. Incorporating enzyme-cleavable linkers in nanoparticle coatings enables selective breakdown and release of therapeutic agents directly in the disease site.
These advances in the metabolization of inorganic architectonics aim to optimize therapeutic efficacy, reduce long-term toxicity, and enable more precise control over nanoparticle behavior in the body, making these materials safer and more effective for clinical use.
Some of recent strategies in the metabolization of inorganic nanostructures are as follows:
(1) Silica NPs: these NPs are often used in drug delivery and imaging applications due to their porous structure and biocompatibility. Silica NPs can degrade into silicic acid in the body, which is excreted through urine. Studies have shown that modifying silica with biodegradable coatings, like PEG, can slow their degradation and extend circulation time. PEG-coated Silica NPs are retained in the blood longer, improving their bioavailability and reducing rapid clearance by the liver and spleen.262–268
(2) IONPs: used widely in MRI imaging and hyperthermia treatment, IONPs can degrade into iron ions after administration. The body can incorporate these ions into natural iron metabolic pathways, such as ferritin storage in the liver, minimizing long-term toxicity.269 IONPs coated with dextran or silica have demonstrated reduced aggregation and enhanced bioavailability, showing promise for both diagnostic and therapeutic applications.270
(3) Au NPs: known for their stability and photothermal properties, Au NPs are used in imaging and cancer therapy. However, gold is bio-persistent, meaning it doesn’t naturally degrade. Researchers have been exploring biodegradable shells (e.g., liposomes or polymers) around Au NPs that release the particles upon reaching target tissues.271 Small Au NPs (<5 nm) are more likely to be cleared renally,272 while larger particles tend to accumulate in the liver and spleen.
(4) QDs: quantum dots, like cadmium-based QDs, are used for their bright fluorescence in imaging. However, cadmium can be toxic, so researchers have developed alternative materials such as carbon-based QDs or silicon QDs, which are more biocompatible and biodegradable. For example, graphene QDs273 can break down into non-toxic byproducts and are naturally cleared from the body faster than traditional cadmium-based QDs, making them safer for in vivo imaging applications.
(5) MgO: MgO NPs are being researched for antimicrobial and antiviral applications. MgO can be metabolized to release magnesium ions, which are biocompatible and essential for cellular function.274 When used in nano-drug formulations, MgO provides a controlled release of therapeutic ions and can be fully metabolized by the body, leaving minimal residues.
These examples illustrate how inorganic nanostructures, like Au NPs, silica NPs, and QDs, can be precisely engineered to enhance their metabolization in the body, ultimately improving their safety and effectiveness for biomedical applications. Through strategic modifications, such as coating NPs with biocompatible materials or functionalizing them with targeting ligands, researchers can reduce potential toxicity, ensure efficient clearance, and extend circulation time. For instance, surface modifications with PEG or other stabilizing agents help NPs evade the immune system and circulate longer, allowing for targeted delivery to diseased cells while minimizing off-target effects. Moreover, tailoring the particle size, shape, and surface charge of these nanostructures can further improve their biodistribution, making them highly effective for applications such as targeted drug delivery, imaging, and diagnostics. GNPs, for example, are being developed with tumor-targeting ligands to accumulate selectively in cancerous tissues, enhancing imaging and therapeutic outcomes.275–280 Similarly, QDs modified with nontoxic coatings allow for safe, high-resolution imaging in real time, proving invaluable for tracking cellular processes.281–289 The advancement of these metabolizable nanostructures reflects the potential of “inorganic medicinal nanoarchitectonics,” a field that combines material science and biomedical engineering to create multifunctional platforms that address the complexities of disease detection and treatment, ultimately paving the way for safer, more effective, and patient-specific medical solutions.
9. Issues and prospects of inorganic medicinal nano architectonics in convergence research
Since biocompatibility is the most concerned parameter in biomaterials, it is an utmost priority to secure the safety through toxicity studies of medicinal nano architectonics that will be newly developed based on inorganic nanomedicine convergence science, and long-term and short-term toxicity tests are required by Ministry of Food and Drug Safety approved institutions and must be verified before clinical trials. When new functional inorganic nanomedicines are synthesized through a new hybrid system, they can have toxicity in the body that is completely different from the characteristics of each component, so it is essential to carefully verify the designed inorganic hybrid material and have a clear understanding of whether it is a reasonable system.
In addition to toxicity issues, there is also a growing interest in new carbon derivatives based on inorganic nanomedicines, which can be used in PTT and PDT. New hybrid technologies with other organic and inorganic NPs could be of great effect in other fields of research, such as bioelectronics and artificial intelligence.
While polymeric NPs offer high biocompatibility, biodegradability, and flexible surface modification, making them ideal for sustained therapeutic release, the inorganic NPs provide superior structural stability, durability, and optical properties, beneficial for PTT and PDT.109,115,290,291 Anchoring polymers on inorganic NPs enhances colloidal stability and prevents issues like dissolution or aggregation, improving biocompatibility and reducing cytotoxicity as mentioned earlier. Polymers also offer precise control over surface chemistry and interparticle interactions, facilitating stable assembly. Although polymeric NPs offer advantages in biocompatibility and controlled drug release, their stability can be limited under certain conditions. Creating hybrid systems that integrate polymeric and inorganic materials provides a robust solution, combining the stability and functional versatility of both components. This approach enhances the nanoparticles’ adaptability for theranostics applications, enabling both targeted therapy and precise diagnostic imaging in a single platform.290
Another important application of inorganic nanomedicine is boron neutron capture therapy (BNCT), which is one of the areas of cancer treatment, and the development of new boron clusters and boron derivatives is a very big issue for the BNCT community. Our lab has been focusing on the development of these BNCT inorganic nanomedicines for the past ∼10 years. For successful cancer treatment with BNCT, sufficient amounts of 10B must be selectively uptaken in the cancer tissue. For this purpose, the inorganic carrier layered double hydroxide (LDH) is used as a carrier for boron delivery to increase the efficiency of uptake boron in cancer tissues.292 These high amount of 10B-containing drugs (mercaptoundecahydro- closo-dodecaborate, BSH) can be intercalated into the two-dimensional layer of LDH, a 2D carrier, to form BSH-LDH nanohybrids, and the applicability of BNCT has been confirmed by the results that the 10B proportion of blood in the tumor in the BSH-LDH-treated group was 4.4 times higher after 2 hours compared to the conventional BSH only treated group in a glioblastoma U87 cells implanted model. Strategies using LDH and other clay-based drug delivery systems are evaluated to be a promising therapeutic platform for cancer treatment through BNCT. However, it is necessary to conduct sufficient in vivo toxicity and experiments to prove the therapeutic effect in various animal models, and having patents based on this is the way to ensure international competitiveness.
Although QDs have shown excellent bio applicability, their toxicity in vivo, especially at high concentrations, is one of the challenges that must be addressed. Of course, various surface protection technologies have been developed to prevent leakage of core ions and improve the stability of QDs, but there is still a long way to go to achieve maximum efficiency. Therefore, in order to utilize QDs as nanomedicines with proven safety in vivo, it is necessary to develop groundbreaking new methods.
Another area of research worth noting is carbon materials, most of which are focused on the energy sector.213,293–298 However, although there are several cases where carbon nanostructured materials have been applied in the field of diagnosis and treatment, there seems to be a limit to their use in other medical fields. The major studies on carbon composites in the field of medical technology are summarized in Table 5. The well-designed nano systems shown could be used in the field of diagnostic treatment, highlighting their potential as multifunctional materials in a variety of medical applications.
Table 5 Various types of carbon nanostructures, their derivatives, and their applications in Theranostics applications
|
Graphene-based hybrids |
Fullerenes and modified forms |
Carbon nanotubes and hybrids |
Carbon quantum dots |
Derivatives and members |
Mono-, bi-, multilayer graphene, graphene oxide (GO), reduced GO |
C60, C70 and others endohedral metallofullerenes |
Single-walled carbon nanotubes (SWCNTs) multi-walled carbon nanotubes (MWCNTs) |
Graphene quantum dots; Carbon quantum dots |
Modification |
Oxidation to -COOH: PEG, folic acid, chitosan |
Oxidation: Poly-hydroxylation and carboxylation; PEGylation |
Oxidation to COOH: -PEG, folic acid Cycloaddition surface coating by sorption (non-covalent): BSA, proteins glycolipids, surfactants, Fe2O3, DNA, PEGylated phospholipids polysaccharides surface amination; |
Covalent coatings with amine-containing agents to oxygen containing groups; chelation of lanthanum ions (e.g., Eu3+), sol–gel technology, doping with elements such as N, S, P, nanohybrids with inorganic particles. (e.g. iron oxide, zinc oxide, silica, and titania), Conjugated with pembrolizumab), a human monoclonal antibody (mAb) targeting the immune checkpoint programmed death receptor (PD-1) and its programmed death ligand-1 (PD-L1) |
Oxidation to -OH: ether-carboxyl cycloaddition Surface coatings by adsorption (non-covalent): Ni/Au aptamers therapeutic agents polymers (PEI) Fe3O4, BSA, proteins |
Specific covalent functionalization: glycosylation Cationic/anionic groups encapsulation and chelation of lanthanum ions (e.g., Gd3+) Cyclodextrin sorption complex |
Use of Theranostics |
Drug/gene delivery, imaging, and photodynamic therapy, tissue engineering, antibacterial activity, biosensing |
Drug/gene delivery, magnetic, photoacoustic imaging, photothermal/photodynamic therapy, enzyme inhibition, antioxidants |
Photo-thermo-acoustic therapy, magnetic resonance imaging, drug/gene peptide delivery, biosensing |
Drug/gene delivery, imaging, photodynamic therapy, biosensing; immunotherapy for treating a wide range of cancers. |
Reference |
213–218
|
219–222
|
223–228
|
218, 223 and 229–232
|
In addition, to expand the clinical application of new carbon-hybrid materials, long-term toxicity and stability analyses must be conducted to prove the safety and durability of the materials.
Despite the diversity of various inorganic nanomedicine in the field of diagnostic treatment, future research needs to focus on the long-term in vivo safety and biocompatibility of inorganic NPs. In addition, new functional inorganic hybrid NPs require advanced research and development into multifunctional materials that can provide maximum therapeutic and diagnostic effects to patients, as seen in the example of combining in vivo imaging with photo immunochemotherapy. After the establishment of these various functional platform technologies, it is essential to have a full understanding of improved physicochemical properties and in vivo metabolism prior to clinical application.
If the safety and stability of inorganic NPs are ensured, attention should be given to the stable colloidal dispersibility of NPs when developing them as an injectable formulation, according to the final research and development goals. Additionally, if they are intended to be developed as oral drugs, understanding the absorption mechanisms and metabolic processes through the intestinal membrane is essential.
Compared to existing research, there are not many inorganic nanomedicines that have been commercialized for diagnosis and treatment in the pharmaceutical market. In this sense, the market for inorganic nanomedicines can be said to be a blue ocean. Therefore, if more efforts are made in research and development in terms of commercialization of inorganic nanomedicines, it is sure that greater opportunities will be given.
If a new generation of inorganic materials chemists devises innovative strategies, it could foster substantial advancements toward a future where current limitations in material design are overcome. Such creativity may lead to the development of more stable, functional, and sustainable materials that address pressing challenges in energy, medicine, and environmental protection. By exploring new molecular architectures, enhancing materials' durability, and optimizing their compatibility for a range of applications, these chemists could transform fields like renewable energy storage, biodegradable electronics, and safe nanotechnology, offering a promising outlook for science and society.
10. Perspectives
Convergent inorganic medicinal nanoarchitectonics, where complex, multifunctional inorganic nanostructures are engineered for therapeutic and diagnostic applications holds immense potential for advancing nanomedicine. By combining principles from chemistry, materials science, and nanomedicine, this approach facilitates the development of nano-architectures that can address some of medicine's most persistent challenges.
10.1. Multifunctionality
Convergent inorganic nanohybrids offer the advantage of integrating multiple functionalities into a single nanoparticle system. These functionalities may include therapeutic agents, imaging probes, targeting ligands, and stimuli-responsive elements, allowing for simultaneous diagnosis and treatment of diseases.
10.2. Improved therapeutic efficacy
By combining therapeutic agents with imaging components, inorganic nanohybrids enable real-time monitoring of drug delivery and therapeutic response. This capability facilitates personalized medicine approaches by tailoring treatments based on individual patient responses.
10.3. Enhanced imaging modalities
Inorganic nanohybrids can incorporate various imaging modalities such as MRI, CT, fluorescence imaging, and photoacoustic imaging. This versatility enables multimodal imaging, providing complementary information for accurate disease diagnosis and treatment monitoring.
10.4. Targeted drug delivery
Functionalization of inorganic nanohybrids with targeting ligands allows for site-specific delivery of therapeutic agents, reducing off-target effects and improving treatment efficacy. Targeting ligands can be designed to recognize specific biomarkers overexpressed in diseased tissues, enabling precision medicine approaches.
10.5. Theranostic platforms for personalized medicine
Convergent inorganic nanohybrids have the potential to serve as theranostic platforms for personalized medicine, where diagnostics and therapeutics are tailored to individual patient profiles. By integrating imaging and therapeutic capabilities into a single nanoparticle system, clinicians can monitor disease progression, optimize treatment regimens, and minimize adverse effects.
11. Challenges and opportunities
Inorganic medicinal nanoarchitectonics present promising opportunities, though they come with notable challenges and future directions for research. Challenges primarily include issues of toxicity, biocompatibility, and the complexity of achieving precise control over nanoparticle design to ensure predictable behavior in biological environments. Regulatory hurdles also pose difficulties, as the unique properties of these nanostructures often necessitate new testing and approval frameworks. Despite these challenges, there are significant opportunities: inorganic nanoarchitectures can be tailored for high stability, multifunctionality, and targeted delivery, which is ideal for applications like theranostics, where diagnosis and therapy are integrated into a single platform.
Future research is moving towards designing hybrid systems that combine inorganic and organic materials, enhancing both efficacy and biocompatibility. Additionally, personalized nanomedicine offers an exciting frontier, with researchers exploring how these materials can be customized for specific patient needs, diseases, or even individualized therapeutic responses. Emphasis on green synthesis methods and biodegradable nanomaterials also points toward sustainable and safer medical applications. As interdisciplinary collaboration advances, inorganic medicinal nanoarchitectonics have the potential to revolutionize fields such as oncology, regenerative medicine, and infectious disease treatments.
Author contributions
JHC and NSR Conceptualized the review, contributed to the overall structure and design of the manuscript, and provided expertise on the application of nanoscale materials for theranostics. NSR, SY, and GC Led the writing and coordination of the manuscript, with a particular focus on nanohybrid systems for cancer therapy and drug delivery. Contributed significantly to the literature review and analysis of recent advancements in nanomedicine. All authors have conducted in-depth research on bio-hybrid materials and their biomedical applications. Contributed to the review's section on bio-imaging and theranostics platforms and provided critical revisions throughout the manuscript. JHC Supervised the project and provided critical insights on inorganic nanohybrids and their therapeutic applications. All authors have contributed to the conceptual framework of the manuscript and ensured the review's scientific accuracy and coherence.
Data availability
All the data associated with this manuscript are available within the manuscript.
Conflicts of interest
There are no conflicts to declare.
Acknowledgements
This study was supported by the National Research Foundation of Korea (NRF) grant funded by the Korean government (MSIT) (No. 2022R1F1A1076459), the Ministry of Education (No. RS-2023-00245466 and RS-2023-00242339), and the Korea Basic Science Institute (National research Facilities and Equipment Centre) (No. RS-2024-00402984).
Notes and references
- J. Song, W. Lyu, K. Kawakami and K. Ariga, Nanoscale, 2024, 16, 13230–13246 RSC.
- N. Agamendran, M. Uddin, M. S. Yesupatham, M. Shanmugam, A. Augustin, T. Kundu, R. Kandasamy, K. Sasaki and K. Sekar, Langmuir, 2024, 40, 3320–3342 CAS.
- S. K. Kim, J. U. Lee, M. J. Jeon, S. K. Kim, S. H. Hwang, M. E. Hong and S. J. Sim, RSC Adv., 2023, 13, 27225–27232 RSC.
- X. Shen, J. Song, K. Kawakami and K. Ariga, Materials, 2022, 15, 5404 CrossRef CAS.
- X. Shen, J. Song, C. Sevencan, D. T. Leong and K. Ariga, Sci. Technol. Adv. Mater., 2022, 23, 199–224 CrossRef PubMed.
- Y. M. Wang, Y. Xu, X. Zhang, Y. Cui, Q. Liang, C. Liu, X. Wang, S. Wu and R. Yang, Nanomaterials, 2022, 12, 287 CrossRef CAS.
- K. Ariga, X. Jia, J. Song, J. P. Hill, D. T. Leong, Y. Jia and J. Li, Angew. Chem., Int. Ed., 2020, 59, 15424–15446 CrossRef CAS PubMed.
- M. L. Cortez, A. Lorenzo, W. A. Marmisolle, C. von Bilderling, E. Maza, L. Pietrasanta, F. Battaglini, M. Ceolin and O. Azzaroni, Soft Matter, 2018, 14, 1939–1952 RSC.
- K. Ariga, Anal. Sci., 2016, 32, 1141–1149 CrossRef.
- S. N. Song, X. L. Zhao, X. C. Yang, Y. Ding, F. D. Ren, X. Y. Pang, B. Li, J. Y. Hu, Y. Z. Chen and W. W. Gao, ACS Appl. Mater. Interfaces, 2024, 16, 16011–16028 CrossRef CAS.
- S. Zhang, A. Tamura and N. Yui, RSC Adv., 2024, 14, 3798–3806 RSC.
- W. Tian, C. Wang, R. Chu, H. Ge, X. Sun and M. Li, Biomater. Res., 2023, 27, 100 CrossRef CAS.
- M. A. Gonzalez-Reyna, G. A. Molina, K. Juarez-Moreno, A. Rodriguez-Torres, R. Esparza and M. Estevez, Biomater. Adv., 2023, 153, 213561 CrossRef CAS PubMed.
- S. Mohanan, X. Guan, M. Liang, A. Karakoti and A. Vinu, Small, 2023, e2301113, DOI:10.1002/smll.202301113.
- M. Komiyama, Beilstein J. Nanotechnol., 2023, 14, 218–232 CrossRef CAS.
- P. Jayachandran, S. Ilango, V. Suseela, R. Nirmaladevi, M. R. Shaik, M. Khan, M. Khan and B. Shaik, Biomedicines, 2023, 11 Search PubMed.
- A. Wu, Y. Guo, X. Li, Q. Li, G. Chen, H. Zang and J. Li, J. Colloid Interface Sci., 2023, 630, 161–169 CrossRef CAS.
- Y. Zhang, E. Ranaei Pirmardan, A. Barakat, M. Naseri and A. Hafezi-Moghadam, ACS Appl. Mater. Interfaces, 2022, 14, 42976–42987 CrossRef CAS.
- E. Kirbas Cilingir, M. Sankaran, J. M. Garber, F. A. Vallejo, M. Bartoli, A. Tagliaferro, S. Vanni, R. M. Graham and R. M. Leblanc, Nanoscale, 2022, 14, 9686–9701 RSC.
- D. B. Momekova, V. E. Gugleva and P. D. Petrov, ACS Omega, 2021, 6, 33265–33273 CrossRef CAS.
- R. Moradi, N. P. Khalili, N. L. W. Septiani, C. H. Liu, E. Doustkhah, Y. Yamauchi and S. V. Rotkin, Small, 2022, 18, e2104847 CrossRef PubMed.
- S. Sharma, M. K. Masud, Y. V. Kaneti, P. Rewatkar, A. Koradia, M. S. A. Hossain, Y. Yamauchi, A. Popat and C. Salomon, Small, 2021, 17, e2102220 CrossRef.
- S. Xie, Y. Li, Y. Suo, Z. Wang, B. Zhang, J. Li, J. Huang, Y. Wang, C. Ma, D. Lin, T. Ma and Y. Shao, Biol. Trace Elem. Res., 2024 DOI:10.1007/s12011-024-04341-y.
- N. C. Madlala, N. Khanyile and A. Masenya, Nanomaterials, 2024, 14 Search PubMed.
- D. Yang, X. Dong, L. Jiang, F. Liu, S. Ma, X. Shi, Y. Du, C. Chen and H. Deng, Small, 2024, 20, e2402334 CrossRef.
- J. Song, R. Zheng, R. Yang, S. Yu, J. Xiao and J. Liu, Anal. Bioanal. Chem., 2024, 416, 3271–3280 CrossRef CAS PubMed.
- B. Fan, Y. Fang, B. Xue, Q. Wu, Z. Luo, X. Zhang, L. Calvez and L. Wu, ACS Appl. Mater. Interfaces, 2024, 16, 7182–7188 CrossRef CAS PubMed.
- P. Barge, S. Gugawad, S. N. Devendrappa, S. Hadakar and S. M. Taur, Int. J. Clin. Pediatr. Dent., 2023, 16, 168–175 CrossRef.
- D. A. Ahmed, M. A. El-Apasery and S. M. Ragai, Sci. Rep., 2023, 13, 19889 CrossRef CAS PubMed.
- I. Chemistry, Int. J. Anal. Chem., 2023, 2023, 9872352 Search PubMed.
- M. Nurdin, I. D. W. Sari, M. Mardhatillah, N. Herdianto, D. Wibowo, M. Maulidiyah, M. Mappasomba, A. Ansharullah and C. Bijang, Indian J. Microbiol., 2023, 63, 216–221 CrossRef CAS.
- M. Mofarrah, D. Jafari-Gharabaghlou, M. Farhoudi-Sefidan-Jadid and N. Zarghami, Heliyon, 2023, 9, e16309 CrossRef CAS PubMed.
- H. Y. Woo, Y. Choi, H. Chung, D. W. Lee and T. Paik, Nano Converg., 2023, 10, 17 CrossRef CAS.
- Y. Xu, F. Gu, S. Hu, Y. Wu, C. Wu, Y. Deng, B. Gu, Z. Chen and Y. Yang, Biosens. Bioelectron., 2023, 228, 115173 CrossRef CAS.
- M. Lei, Y. Huang, Y. Zhou, C. O. Mensah, D. Wei and B. Li, J Environ Sci (China), 2023, 129, 30–44 CrossRef CAS.
-
G. L. Patrick, An introduction to medicinal chemistry, Oxford University Press, New York, NY, Seventh edn, 2023 Search PubMed.
- H. V. Tolomeu and C. A. M. Fraga, Molecules, 2023, 28, 838 CrossRef CAS PubMed.
- F. López-Gallego and L. Salassa, Chem. Catal., 2023, 3 Search PubMed.
- D. Lee, J. Shin, H. Son, S. Y. Cheon, Y. Lee, J. Park and H. Koo, Nanoscale Adv., 2023, 5, 1600–1610 RSC.
- F. Teixidor, R. Núñez and C. Viñas, Molecules, 2023, 28, 4449 CrossRef CAS PubMed.
- L. Kumar, S. Verma, P. Utreja and D. Kumar, Recent Pat. Anti-Cancer Drug Discovery, 2023, 18, 343–363 CrossRef CAS PubMed.
- Q. Gao, L. Tan, Z. Wen, D. Fan, J. Hui and P.-P. Wang, Nano Res., 2023, 16, 11107–11124 CrossRef.
- N. B. Fernandes, Y. Nayak, S. Garg and U. Y. Nayak, Coord. Chem. Rev., 2023, 478, 214977 CrossRef CAS.
- K. Li, Z. Yu, I. Dovgaliuk, C. Le Coeur, V. Lütz-Bueno, E. Leroy, B. Brissault, Y. D. R. de Mimerand, M. Lepoitevin and C. Serre, Chem. Commun., 2023, 59, 4923–4926 RSC.
- M. Liu, T. Gao, L. Jiang, S. Li, B. Shi and F. Li, Biomed. Pharmacother., 2023, 159, 114261 CrossRef CAS.
- G. Choi, N. S. Rejinold, H. Piao, Y. B. Ryu, H. J. Kwon, I. C. Lee, J. I. Seo, H. H. Yoo, G. W. Jin and J. H. Choy, Small, 2023, 2305148 CrossRef.
- T. Chen, A. Wang, H. Qiu, X. Liu, Y. Wang, S. Wang, Z. Liu, X. Guo, S. Ji and H. Liang, ACS Sustainable Chem. Eng., 2023, 11, 7419–7433 CrossRef CAS.
- H. Song, Y. Ji, Y. Zhu, J. Xia, C. Hu, Y. Jin, J. Zhang, Z. Hu and J. Dai, Carbon, 2023, 204, 196–210 CrossRef CAS.
- H. Fu, Y. Guo, W. Fang, J. Wang, P. Hu and J. Shi, Adv. Sci., 2024, 11, 2307094 CrossRef CAS.
- Q. Wu, L. Yang, L. Zou, W. Yang, Q. Liu, A. Zhang, J. Cao, G. Shi, J. He and X. Yang, Adv. Healthcare Mater., 2023, 12, 2300632 CrossRef CAS.
- P. Si, J. Lei, C. Yang, P. Zhang, X. Li, S. Zheng, Q. Li and J. Zhang, Int. J. Nanomed., 2023, 2225–2238 CrossRef CAS PubMed.
- K. Bansal, N. Devi, M. Aqdas, M. Kumar, J. N. Agrewala, O. Katare, R. K. Sharma and N. Wangoo, J. Drug Delivery Sci. Technol., 2023, 88, 104959 CrossRef CAS.
- H. Piao, G. Choi and J. H. Choy, Colloids Surf., B, 2023, 228, 113386 CrossRef.
- H. Piao, G. Choi, N. S. Rejinold, J. Yu, S.-J. Choi and J.-H. Choy, J. Ind. Eng. Chem., 2024, 130, 572–579 CrossRef CAS.
- X. Ding, X. Liu, T. Qiu, Y. Zhou, N. Michał, S. Roman, Q. Liu, Y. Liu and N. Peng, Int. J. Biol. Macromol., 2024, 261, 129761 CrossRef CAS PubMed.
- Y. Wu, M. Zhou, R. Lin, L. Yu, X. Zhang and J. Xie, ACS Appl. Mater. Interfaces, 2023, 15, 30427–30442 CrossRef CAS.
- H.-T. D. Bui, Y. Park, Y. M. Jung, S. Y. Chew and H. S. Yoo, J. Mater. Chem. B, 2023, 11, 6961–6974 RSC.
- C. Zhang, M. Wang, J. Zhang, B. Zou and Y. Wang, Colloids Surf., B, 2023, 229, 113467 CrossRef CAS PubMed.
- S. Bisso and J.-C. Leroux, Int. J. Pharm., 2020, 578, 119098 CrossRef CAS.
- B. S. Sekhon and S. R. Kamboj, Nanomedicine, 2010, 6, 516–522 CrossRef CAS.
- P. Richard Feynmann, Eng. Sci., 1960, 23, 22–36 Search PubMed.
- S. Hassan, G. Prakash, A. B. Ozturk, S. Saghazadeh, M. F. Sohail, J. Seo, M. R. Dokmeci, Y. S. Zhang and A. Khademhosseini, Nano Today, 2017, 15, 91–106 CrossRef CAS.
- S. Iijima, Nature, 1991, 354, 56–58 CrossRef CAS.
- H. W. Kroto, A. Allaf and S. Balm, Chem. Rev., 1991, 91, 1213–1235 CrossRef CAS.
- A. S. Thakor and S. S. Gambhir, Ca-Cancer J. Clin., 2013, 63, 395–418 CrossRef.
- H. Chen, W. Zhang, G. Zhu, J. Xie and X. Chen, Nat. Rev. Mater., 2017, 2, 1–18 Search PubMed.
- W. Fan, B. Yung, P. Huang and X. Chen, Chem. Rev., 2017, 117, 13566–13638 CrossRef CAS.
- X. Huang, Y. Liu, B. Yung, Y. Xiong and X. Chen, ACS Nano, 2017, 11, 5238–5292 CrossRef CAS.
- S. N. Bhatia, X. Chen, M. A. Dobrovolskaia and T. Lammers, Nat. Rev. Cancer, 2022, 22, 550–556 CrossRef CAS.
- J. Ren, N. Andrikopoulos, K. Velonia, H. Tang, R. Cai, F. Ding, P. C. Ke and C. Chen, J. Am. Chem. Soc., 2022, 144, 9184–9205 CrossRef CAS.
- S. Mahajan, M. Aalhate, S. K. Guru and P. K. Singh, J. Controlled Release, 2022, 347, 211–236 CrossRef CAS.
- N. F. Attia, E. M. Abd El-Monaem, H. G. El-Aqapa, S. E. Elashery, A. S. Eltaweil, M. El Kady, S. A. Khalifa, H. B. Hawash and H. R. El-Seedi, Appl. Surf. Sci. Adv., 2022, 11, 100284 CrossRef.
- B. A. Chabner, Cancer Res., 2010, 70, 428–429 CrossRef CAS.
- X. Hu, Y. Zhang, T. Ding, J. Liu and H. Zhao, Front. Bioeng. Biotechnol., 2020, 8, 990 CrossRef.
- C. Song, W. Sun, Y. Xiao and X. Shi, Drug Discovery Today, 2019, 24, 835–844 CrossRef CAS PubMed.
- G. Pasparakis, Wiley Interdiscip. Rev.:Nanomed. Nanobiotechnol., 2022, 14, e1817 CAS.
- U. Badıllı, F. Mollarasouli, N. K. Bakirhan, Y. Ozkan and S. A. Ozkan, TrAC, Trends Anal. Chem., 2020, 131, 116013 CrossRef.
- S. Yu, G. Choi and J.-H. Choy, Nanomaterials, 2023, 13, 1102 CrossRef CAS PubMed.
- R. Dubey, D. Dutta, A. Sarkar and P. Chattopadhyay, Nanoscale Adv., 2021, 3, 5722–5744 RSC.
- Y. Chen, C. D'Amario, A. Gee, H. T. Duong, O. Shimoni and S. M. Valenzuela, Acta Biomater., 2020, 102, 384–393 CrossRef CAS.
- M. B. Cortie, Gold Bull., 2004, 37, 12–19 CrossRef CAS.
- P. Juzenas, W. Chen, Y.-P. Sun, M. A. N. Coelho, R. Generalov, N. Generalova and I. L. Christensen, Adv. Drug Delivery Rev., 2008, 60, 1600–1614 CrossRef CAS.
- S. Bandaru, N. George, B. Sharma, M. Palanivel, A. Mukherjee, W. Y. Wu, K. K. Ghosh, W. B. Ball, B. Gulyas, P. Padmanabhan, S. Ghosh and S. Chakrabortty, Biomater. Sci., 2024 10.1039/d4bm00811a.
- A. Savalan, Turk. J. Biol., 2024, 48, 338–347 CrossRef PubMed.
- L. Shi, G. Huanood, S. Miura, M. Kuragano and K. Tokuraku, Int. J. Mol. Sci., 2024, 25 Search PubMed.
- S. Nimrawi, P. Gannett and Y. M. Kwon, Expert Opin. Drug Delivery, 2024, 21, 1349–1362 CrossRef CAS PubMed.
- V. Rahimkhoei, A. H. Alzaidy, M. J. Abed, S. Rashki and M. Salavati-Niasari, Adv. Colloid Interface Sci., 2024, 329, 103204 CrossRef CAS.
- G. Unnikrishnan, A. Joy, M. Megha, E. Kolanthai and M. Senthilkumar, Discover Nano, 2023, 18, 157 CrossRef CAS.
- M. Ischyropoulou, K. Sabljo, L. Schneider, C. M. Niemeyer, J. Napp, C. Feldmann and F. Alves, Adv. Mater., 2023, 35, e2305151 CrossRef.
- F. Yanar, D. Carugo and X. Zhang, Molecules, 2023, 28 Search PubMed.
- T. M. Zimina, N. O. Sitkov, K. G. Gareev, V. Fedorov, D. Grouzdev, V. Koziaeva, H. Gao, S. E. Combs and M. Shevtsov, Biosensors, 2022, 12 Search PubMed.
- R. Ghosh, S. Mondal, D. Mukherjee, A. Adhikari, M. Bhattacharyya and S. K. Pal, Curr. Drug Delivery, 2022, 19, 991–1000 CrossRef CAS PubMed.
- N. Moradifar, A. A. Kiani, A. Veiskaramian and K. Karami, Curr. Cardiol. Rev., 2022, 18, e110621194025 CrossRef CAS PubMed.
- Q. Chen, Y. Chen, W. Zhang, Q. Huang, M. Hu, D. Peng, C. Peng, L. Wang and W. Chen, ChemMedChem, 2020, 15, 1940–1946 CrossRef CAS.
- M. Vallet-Regí, F. Schüth, D. Lozano, M. Colilla and M. Manzano, Chem. Soc. Rev., 2022, 51, 5365–5451 RSC.
- A. Jahangiri-Manesh, M. Mousazadeh, S. Taji, A. Bahmani, A. Zarepour, A. Zarrabi, E. Sharifi and M. Azimzadeh, Pharmaceutics, 2022, 14, 664 CrossRef CAS PubMed.
- L. Zhu, W. Lian, N. Yu, J. Meng, H. Zeng, Y. Wang, X. Wang, M. Wen and Z. Chen, Adv. Healthcare Mater., 2024, 13, e2400127 CrossRef.
- Y. Ma, Y. Zhou, C. Wang, B. Gao, J. Li, M. Zhu, H. Wu, C. Zhang and Y. Qin, Adv. Mater., 2023, 35, e2303741 CrossRef.
- K. Wu, A. Mohsin, W. Q. Zaman, Z. Zhang, W. Guan, M. Chu, Y. Zhuang and M. Guo, J. Nanobiotechnol., 2022, 20, 224 CrossRef CAS PubMed.
- C. F. Cuadrado, A. Diaz-Barrios, K. O. Campana, E. C. Romani, F. Quiroz, S. Nardecchia, A. Debut, K. Vizuete, D. Niebieskikwiat, C. E. Avila, M. A. Salazar, C. Garzon-Romero, A. Blasco-Zuniga, M. R. Rivera and M. P. Romero, Pharmaceutics, 2022, 14 Search PubMed.
- W. Zhu, C. Zhang, Y. Chen and Q. Deng, Polymers, 2021, 13 Search PubMed.
- Y. Chen, P. Han, Y. Wu, Z. Zhang, Y. Yue, W. Li and M. Chu, Small, 2018, 14, e1802799 CrossRef.
- M. Wang, K. Deng, W. Lu, X. Deng, K. Li, Y. Shi, B. Ding, Z. Cheng, B. Xing, G. Han, Z. Hou and J. Lin, Adv. Mater., 2018, 30, e1706747 CrossRef PubMed.
- F. Wo, R. Xu, Y. Shao, Z. Zhang, M. Chu, D. Shi and S. Liu, Theranostics, 2016, 6, 485–500 CrossRef CAS PubMed.
- G. Oberdörster, J. Intern. Med., 2010, 267, 89–105 CrossRef PubMed.
- T. T. Truong, S. Mondal, V. H. M. Doan, S. Tak, J. Choi, H. Oh, T. D. Nguyen, M. Misra, B. Lee and J. Oh, Adv. Colloid Interface Sci., 2024, 103263 CrossRef CAS PubMed.
- L. Z. Flores-López, H. Espinoza-Gómez and R. Somanathan, J. Appl. Toxicol., 2019, 39, 16–26 CrossRef.
- H. Yu, F. Jin, D. Liu, G. Shu, X. Wang, J. Qi, M. Sun, P. Yang, S. Jiang and X. Ying, Theranostics, 2020, 10, 2342 CrossRef CAS PubMed.
- X. Li, Y. Zhang, G. Liu, L. Zhou, Y. Xue and M. Liu, RSC Adv., 2022, 12, 7635–7651 RSC.
- E.-K. Lim, T. Kim, S. Paik, S. Haam, Y.-M. Huh and K. Lee, Chem. Rev., 2015, 115, 327–394 CrossRef CAS PubMed.
- S. Huang and G. Huang, RSC Adv., 2024, 14, 20884–20897 RSC.
- J. Fang, W. Islam and H. Maeda, Adv. Drug Delivery Rev., 2020, 157, 142–160 CrossRef CAS PubMed.
- M. J. N. Amaldoss, J.-L. Yang, P. Koshy, A. Unnikrishnan and C. C. Sorrell, Drug Discovery Today, 2022, 27, 103386 CrossRef CAS PubMed.
- G.-W. Jin, G. Choi, N. S. Rejinold, H. Piao, Y. B. Ryu, H.-J. Kwon, I. C. Lee and J.-H. Choy, bioRxiv, 2022, 07.19, 500639 Search PubMed.
- S. Eom, M. H. Kim, R. Yoo, G. Choi, J. H. Kang, Y. J. Lee and J.-H. Choy, J. Mater. Chem. B, 2022, 10, 9389–9399 RSC.
- N. Rajana, A. Mounika, P. S. Chary, V. Bhavana, A. Urati, D. Khatri, S. B. Singh and N. K. Mehra, J. Controlled Release, 2022, 352, 1024–1047 CrossRef CAS.
- M. E. Roseland, Y. K. Dewaraja and K. K. Wong, Curr. Opin. Endocrinol., Diabetes Obes., 2022, 29, 456–465 CrossRef PubMed.
- J. Wang, Y. Dong, P. Ma, Y. Wang, F. Zhang, B. Cai, P. Chen and B. F. Liu, Adv. Mater., 2022, 34, 2201051 CrossRef CAS.
- J. W. Bulte, Am. J. Roentgenol., 2009, 193, 314–325 CrossRef PubMed.
- A. Solanki, J. D. Kim and K.-B. Lee, Nanomed., 2008, 3, 567–578 CrossRef CAS.
- N. R. Nelson, J. D. Port and M. K. Pandey, J. Nanotheranostics, 2020, 1, 105–135 CrossRef.
- Z. Zhang, N. Mascheri, R. Dharmakumar, Z. Fan, T. Paunesku, G. Woloschak and D. Li, Cytotherapy, 2009, 11, 43–51 CrossRef CAS.
- M. M. Garrigós, F. A. Oliveira, M. P. Nucci, J. B. Mamani, O. F. Dias, G. N. Rego, M. S. Junqueira, C. J. Costa, L. R. Silva and A. H. Alves, Pharmaceutics, 2023, 15, 828 CrossRef.
- Jasmin, G. T. de Souza, R. A. Louzada, P. H. Rosado-de-Castro, R. Mendez-Otero and A. C. Campos de Carvalho, Int. J. Nanomed., 2017, 779–793 CrossRef CAS.
- K. C. Partlow, J. Chen, J. A. Brant, A. M. Neubauer, T. E. Meyerrose, M. H. Creer, J. A. Nolta, S. D. Caruthers, G. M. Lanza and S. A. Wicldine, FASEB J., 2007, 21, 1647–1654 CrossRef CAS.
- P. Farias, B. S. Santos, A. Fontes and C. L. Cesar, Microscopy, 2007, 1, 840–847 Search PubMed.
- J. Geurts and V. Wagner, Phys. Status Solidi B, 2005, 242, 2644–2649 CrossRef CAS.
- M. A. Walling, J. A. Novak and J. R. E. Shepard, Int. J. Mol. Sci., 2009, 10, 441–491 CrossRef CAS.
- S. Yamada, H. Yukawa, K. Yamada, Y. Murata, J.-I. Jo, M. Yamamoto, A. Sugawara-Narutaki, Y. Tabata and Y. Baba, Sensors, 2022, 22, 5705 CrossRef CAS PubMed.
- X. Qian, X.-H. Peng, D. O. Ansari, Q. Yin-Goen, G. Z. Chen, D. M. Shin, L. Yang, A. N. Young, M. D. Wang and S. Nie, Nat. Biotechnol., 2008, 26, 83–90 CrossRef CAS.
- J. Kneipp, H. Kneipp and K. Kneipp, Proc. Natl. Acad. Sci. U. S. A., 2006, 103, 17149–17153 CrossRef CAS PubMed.
- C. Shao, Z. Li, C. Zhang, W. Zhang, R. He, J. Xu and Y. Cai, Mater. Today Bio, 2022, 17, 100441 CrossRef CAS PubMed.
- Z. Liu, D. Zhou, X. Yan, L. Xiao, P. Wang, J. Wei and L. Liao, Int. J. Nanomed., 2022, 17, 4757 CrossRef CAS.
- D. Sebben, G. Strohle, P. S. Roy and H. Li, Microchim. Acta, 2023, 190, 144 CrossRef CAS.
- X. Huang, P. K. Jain, I. H. El-Sayed and M. A. El-Sayed, Lasers Med. Sci., 2008, 23, 217–228 CrossRef PubMed.
- Y.-Y. Yuan, X.-Q. Liu, Y.-C. Wang and J. Wang, Langmuir, 2009, 25, 10298–10304 CrossRef CAS PubMed.
- D. Kumar, M. Moghiseh, K. Chitcholtan, I. Mutreja, C. Lowe, A. Kaushik, A. Butler, P. Sykes, N. Anderson and A. Raja, J. Mater. Chem. B, 2023, 11, 1916–1928 RSC.
- M. Yang, H. Gong, D. Yang, L. Feng, S. Gai, F. Zhang, H. Ding, F. He and P. Yang, Chin. Chem. Lett., 2024, 35, 108468 CrossRef CAS.
- Y. Xie, G. Sun, G. A. Mandl, S. L. Maurizio, J. Chen, J. A. Capobianco and L. Sun, Angew. Chem., 2023, 135, e202216269 CrossRef.
- V. Chawla, S. Sharma and Y. Singh, ACS Biomater. Sci. Eng., 2023, 9, 2647–2662 CrossRef CAS PubMed.
- S. G. Nath and E. Anila, Ceram. Int., 2023, 49, 13200–13207 CrossRef CAS.
- Y. Sun, Y. Chen, L. Tian, Y. Yu, X. Kong, J. Zhao and H. Zhang, Nanotechnology, 2007, 18, 275609 CrossRef.
- R. Katwal, Nano-Struct. Nano-Objects, 2023, 35, 101001 CrossRef.
- X. Michalet, F. F. Pinaud, L. A. Bentolila, J. M. Tsay, S. Doose, J. J. Li, G. Sundaresan, A. Wu, S. Gambhir and S. Weiss, Science, 2005, 307, 538–544 CrossRef CAS PubMed.
- R. C. Somers, M. G. Bawendi and D. G. Nocera, Chem. Soc. Rev., 2007, 36, 579–591 RSC.
- A. P. Alivisatos, W. Gu and C. Larabell, Annu. Rev. Biomed. Eng., 2005, 7, 55–76 CrossRef CAS.
- T. Jamieson, R. Bakhshi, D. Petrova, R. Pocock, M. Imani and A. M. Seifalian, Biomaterials, 2007, 28, 4717–4732 CrossRef CAS PubMed.
- G. Iyer, F. Pinaud, J. Tsay and S. Weiss, Small, 2007, 3, 793–798 CrossRef CAS.
- W. Liu, M. Howarth, A. B. Greytak, Y. Zheng, D. G. Nocera, A. Y. Ting and M. G. Bawendi, J. Am. Chem. Soc., 2008, 130, 1274–1284 CrossRef CAS PubMed.
- K. Susumu, H. T. Uyeda, I. L. Medintz, T. Pons, J. B. Delehanty and H. Mattoussi, J. Am. Chem. Soc., 2007, 129, 13987–13996 CrossRef CAS.
- Y. Chen, R. Thakar and P. T. Snee, J. Am. Chem. Soc., 2008, 130, 3744–3745 CrossRef CAS PubMed.
- O. Carion, B. Mahler, T. Pons and B. Dubertret, Nat. Protoc., 2007, 2, 2383–2390 CrossRef CAS PubMed.
- F. Pinaud, D. King, H.-P. Moore and S. Weiss, J. Am. Chem. Soc., 2004, 126, 6115–6123 CrossRef CAS PubMed.
- J. Y. Ying, Y. Zheng and S. T. Selvan, Proc. SPIE, 2008, 6866 DOI:10.1117/12.784053.
- Y. Zheng, S. Gao and J. Y. Ying, Adv. Mater., 2007, 19, 376–380 CrossRef CAS.
- R. E. Bailey, A. M. Smith and S. Nie, Phys. E, 2004, 25, 1–12 CrossRef CAS.
- A. M. Smith, H. Duan, A. M. Mohs and S. Nie, Adv. Drug Delivery Rev., 2008, 60, 1226–1240 CrossRef CAS PubMed.
- B. Dubertret, P. Skourides, D. J. Norris, V. Noireaux, A. H. Brivanlou and A. Libchaber, Science, 2002, 298, 1759–1762 CrossRef CAS PubMed.
- D. S. Lidke, P. Nagy, R. Heintzmann, D. J. Arndt-Jovin, J. N. Post, H. E. Grecco, E. A. Jares-Erijman and T. M. Jovin, Nat. Biotechnol., 2004, 22, 198–203 CrossRef CAS.
- X. Wu, H. Liu, J. Liu, K. N. Haley, J. A. Treadway, J. P. Larson, N. Ge, F. Peale and M. P. Bruchez, Nat. Biotechnol., 2003, 21, 41–46 CrossRef CAS PubMed.
- X. Gao, Y. Cui, R. M. Levenson, L. W. Chung and S. Nie, Nat. Biotechnol., 2004, 22, 969–976 CrossRef CAS PubMed.
- R. Zhou, M. Li, S. Wang, P. Wu, L. Wu and X. Hou, Nanoscale, 2014, 6, 14319–14325 RSC.
- W. MatthewáLeevy, Chem. Commun., 2008, 2331–2333 Search PubMed.
- L. L. Chen, L. Zhao, Z. G. Wang, S. L. Liu and D. W. Pang, Small, 2022, 18, 2104567 CrossRef CAS.
- M. K. Ali, S. Javaid, H. Afzal, I. Zafar, K. Fayyaz, Q. Ain, M. A. Rather, M. J. Hossain, S. Rashid and K. A. Khan, Environ. Res., 2023, 116290 CrossRef CAS.
- H. Du, T. Yin, J. Wang and G. Jie, Anal. Chem., 2023, 95, 7053–7061 CrossRef CAS.
- Y. Xu, P. Niu, L. Zhang, Z. Wen, S. Cheng, M. Lyu and J. Zhu, Nanoscale, 2023, 15, 9691–9699 RSC.
- M. Ghomi, E. N. Zare, H. Alidadi, N. Pourreza, A. Sheini, N. Rabiee, V. Mattoli, X. Chen and P. Makvandi, Adv. Compos. Hybrid Mater., 2023, 6, 51 CrossRef CAS.
- H. Javid, M. A. Oryani, N. Rezagholinejad, A. Hashemzadeh and M. Karimi-Shahri, J. Mater. Chem. B, 2024, 12, 10786–10817 RSC.
- S. Park, V. P. Nguyen, X. Wang and Y. M. Paulus, Int. J. Mol. Sci., 2024, 25 CAS.
- Y. O. Ibrahim, N. Maalej, B. Masood Pirzada, A. Younis Raja, D. H. Anjum, N. Jan, A. Behouch and A. Ul Haq Qurashi, Phys. Med., 2024, 120, 103326 CrossRef PubMed.
- S. Thirumurugan, P. Dash, R. Sakthivel, Y. C. Lin, Y. S. Sun, C. P. Lin, A. N. Wang, X. Liu, U. Dhawan and R. J. Chung, Biomater. Adv., 2024, 158, 213778 CrossRef CAS.
- S. M. Queiroz, T. S. Veriato, L. Raniero and M. L. Castilho, Radiol. Phys. Technol., 2024, 17, 153–164 CrossRef.
- J. D. Pajović, R. J. Dojčilović, S. Kaščáková, M. Réfrégiers, D. K. Božanić and V. Djoković, Colloids Surf., B, 2023, 227, 113340 CrossRef.
- M. Hejazi, S. Arshadi, M. Amini, B. Baradaran, P. Shahbazi-Derakhshi, P. Sameti, J. Soleymani, A. Mokhtarzadeh and S. M. Tavangar, Microchem. J., 2023, 193, 108953 CrossRef CAS.
- C. G. Retnam, S. V. Rose and B. S. Kumari, J. Mol. Liq., 2024, 401, 124684 CrossRef.
- N. Ghane, S. Khalili, S. N. Khorasani, O. Das, S. Ramakrishna and R. E. Neisiany, Sci. Rep., 2024, 14, 11400 CrossRef CAS.
- Z. Li, R. Bai, J. Yi, H. Zhou, J. Xian and C. Chen, Chem. Biomed. Imaging, 2023, 1, 315–339 CrossRef CAS PubMed.
- H. Hu, M. Yuan, J. Chen, T. Fan, N. Nguyen, C. A. Madison, T. Yan, Z. Xiao, Y. Li, S. Eitan, H.-C. Zhou, J. P. Pellois and Y. Wang, Adv. Compos. Hybrid Mater., 2024, 7, 76 CrossRef CAS.
- C. V. Zuben de Valega Negrão, N. N. Cerize, A. D. Silva Justo-Junior, R. B. Liszbinski, G. P. Meneguetti, L. Araujo, S. A. Rocco, K. d Almeida Gonçalves, D. R. Cornejo and P. Leo, Nanomedicine, 2024, 19, 231–254 CrossRef.
- W. Zheng, C. Liu, J. Jin, W. Sun, J. Zhao, M. Zhao, S. Yao, B. Zhu, F. Chen, J. Shang, K. Wang, P. Guo, J. Qin and X. Cheng, PNAS Nexus, 2022, 1, pgac087 CrossRef.
- J. Cardellini, A. Surpi, B. Muzzi, V. Pacciani, C. Innocenti, C. Sangregorio, V. A. Dediu, C. Montis and D. Berti, ACS Appl. Nano Mater., 2024, 7, 3668–3678 CrossRef CAS.
- T. T. V. Phan, S. Mondal, M. Santhamoorthy, T. T. Truong, T. P. Nguyen and J. Oh, Colloids Surf., B, 2024, 238, 113910 CrossRef.
- J. Yang, W. Xiong, L. Huang, Z. Li, Q. Fan, F. Hu, X. Duan, J. Fan, B. Li and J. Feng, J. Nanobiotechnol., 2024, 22, 204 CrossRef CAS PubMed.
- M. Jedrzejczak-Silicka, K. Szymańska, E. Mijowska and R. Rakoczy, Int. J. Mol. Sci., 2024, 25, 930 CrossRef CAS PubMed.
- A. Abrishami, A. R. Bahrami, S. Nekooei, A. Sh Saljooghi and M. M. Matin, Commun. Biol., 2024, 7, 393 CrossRef CAS PubMed.
- C. A. Ferreira, S. Goel, E. B. Ehlerding, Z. T. Rosenkrans, D. Jiang, T. Sun, E. Aluicio-Sarduy, J. W. Engle, D. Ni and W. Cai, Nano Lett., 2021, 21, 4692–4699 CrossRef CAS PubMed.
- C. Mochizuki, J. Nakamura and M. Nakamura, Biomedicines, 2021, 9 Search PubMed.
- J. Montoya Mira, L. Wu, S. Sabuncu, A. Sapre, F. Civitci, S. Ibsen, S. Esener and A. Yildirim, ACS Omega, 2020, 5, 24762–24772 CrossRef CAS.
- H. Winter, M. J. Neufeld, L. Makotamo, C. Sun and A. M. Goforth, Nanomaterials, 2020, 10 Search PubMed.
- J. Vaz-Ramos, R. Cordeiro, M. Castro, C. Geraldes, B. F. O. Costa, H. Faneca and L. Duraes, Mater. Sci. Eng., C, 2020, 115, 111124 CrossRef CAS PubMed.
- H. Jing, S. Sinha, H. S. Sachar and S. Das, Colloids Surf., B, 2019, 177, 433–439 CrossRef CAS PubMed.
- M. Shi, J. Zhang, J. Li, Y. Fan, J. Wang, W. Sun, H. Yang, C. Peng, M. Shen and X. Shi, J. Mater. Chem. B, 2019, 7, 368–372 RSC.
- P. Kumar, P. Tambe, K. M. Paknikar and V. Gajbhiye, J. Controlled Release, 2018, 287, 35–57 CrossRef CAS PubMed.
- W. Zhang, M. Liu, A. Liu and G. Zhai, Curr. Pharm. Des., 2017, 23, 3367–3382 Search PubMed.
- C. Baleizao and J. P. Farinha, Nanomedicine, 2015, 10, 2311–2314 CrossRef CAS.
- J. N. Liu, W. B. Bu and J. L. Shi, Acc. Chem. Res., 2015, 48, 1797–1805 CrossRef CAS PubMed.
- M. H. Chan and H. M. Lin, Biomaterials, 2015, 46, 149–158 CrossRef CAS PubMed.
- G. Qin, W. Xie, X. Luo, G. Zou, Q. Mo and W. Zhong, Microporous Mesoporous Mater., 2024, 370, 113012 CrossRef CAS.
- H. Chang, Y. Park, K. Kim, C. Han, Y. Yoon, W. Yoo, J. Yoo, D. Lee, H. Han and K. Kim, Chem. Eng. J., 2024, 152529 CrossRef CAS.
- G. Ferro-Flores, A. Ancira-Cortez, B. Ocampo-García and L. Meléndez-Alafort, Nanomaterials, 2024, 14, 296 CrossRef CAS PubMed.
- L. Tong, J. Cao, K. Wang, J. Song and J. Mu, Adv. Opt. Mater., 2024, 12, 2301767 CrossRef CAS.
- L. Sun, L. Yang and Q. Cai, J. Alloys Compd., 2024, 999, 175056 CrossRef CAS.
- N. S. Rejinold, G.-W. Jin and J.-H. Choy, Nano Lett., 2024, 24, 14541–14551 CrossRef CAS PubMed.
- M. Overchuk, R. A. Weersink, B. C. Wilson and G. Zheng, ACS Nano, 2023, 17, 7979–8003 CrossRef CAS.
- G. Feng, G.-Q. Zhang and D. Ding, Chem. Soc. Rev., 2020, 49, 8179–8234 RSC.
- Y. Liu, R. Qin, S. A. Zaat, E. Breukink and M. Heger, J. Clin. Transl. Res., 2015, 1, 140 Search PubMed.
- M. He, Z. Cheng, Z. Wang, M. Li, H. Liang, H. Liu, L. Yu, L. Zhao and F. Yu, Adv. Healthcare Mater., 2023, 12, 2300752 CrossRef CAS.
- C.-S. Lin, M.-Q. He, M.-Y. An, Q.-H. Zhao, Z.-H. Zhang, K.-Y. Deng, Y. Ai and H.-B. Xin, Small, 2024, 2403313 CrossRef.
- H. Wu, X. Ding, Y. Chen, Y. Cai, Z. Yang and J. Jin, ACS Omega, 2023, 8, 12752–12760 CrossRef CAS.
- K. Bhattacharjee and B. L. Prasad, Chem. Soc. Rev., 2023, 52, 2573–2595 RSC.
- C.-M. Hirschbiegel, X. Zhang, R. Huang, Y. A. Cicek, S. Fedeli and V. M. Rotello, Adv. Drug Delivery Rev., 2023, 195, 114730 CrossRef CAS.
- J.-H. He and N. Y. Abd Elazem, Facta Univ., Ser.:Mech. Eng., 2022, 20, 211–235 Search PubMed.
- D. Bitounis, H. Ali-Boucetta, B. H. Hong, D. H. Min and K. Kostarelos, Adv. Mater., 2013, 25, 2258–2268 CrossRef CAS PubMed.
- A. Bianco, Angew. Chem., Int. Ed., 2013, 52, 4986–4997 CrossRef CAS PubMed.
- Y. Wang, S. Zhang, T. Xu, T. Zhang, Y. Mo, J. Liu, L. Yan and F. Xing, Sens. Actuators, B, 2018, 263, 417–425 CrossRef CAS.
- J. Arkowski, M. Obremska, K. Kędzierski, A. Sławuta and M. Wawrzyńska, Adv. Clin. Exp. Med., 2020, 29, 1497–1504 CrossRef PubMed.
- S. Chung, R. A. Revia and M. Zhang, Adv. Mater., 2021, 33, 1904362 CrossRef CAS PubMed.
- L. Fusco, A. Gazzi, G. Peng, Y. Shin, S. Vranic, D. Bedognetti, F. Vitale, A. Yilmazer, X. Feng and B. Fadeel, Theranostics, 2020, 10, 5435 CrossRef CAS.
- J. Liu, S.-i Ohta, A. Sonoda, M. Yamada, M. Yamamoto, N. Nitta, K. Murata and Y. Tabata, J. Controlled Release, 2007, 117, 104–110 Search PubMed.
- Z. Chen, L. Ma, Y. Liu and C. Chen, Theranostics, 2012, 2, 238 Search PubMed.
- S. K. Sharma, L. Y. Chiang and M. R. Hamblin, Nanomedicine, 2011, 6, 1813–1825 Search PubMed.
-
F. Cataldo and T. Da Ros, Medicinal chemistry and pharmacological potential of fullerenes and carbon nanotubes, Springer Science & Business Media, 2008 Search PubMed.
- A. Alam, C. Wan and T. McNally, Eur. Polym. J., 2017, 87, 422–448 Search PubMed.
- Z. Liu, S. Tabakman, K. Welsher and H. Dai, Nano Res., 2009, 2, 85–120 Search PubMed.
- S. Marchesan, K. Kostarelos, A. Bianco and M. Prato, Mater. Today, 2015, 18, 12–19 Search PubMed.
- L. Golubewa, I. Timoshchenko, O. Romanov, R. Karpicz, T. Kulahava, D. Rutkauskas, M. Shuba, A. Dementjev, Y. Svirko and P. Kuzhir, Sci. Rep., 2020, 10, 22174 CrossRef CAS.
- J. H. Choi, F. T. Nguyen, P. W. Barone, D. A. Heller, A. E. Moll, D. Patel, S. A. Boppart and M. S. Strano, Nano Lett., 2007, 7, 861–867 CrossRef CAS PubMed.
- Y. Wang and A. Hu, J. Mater. Chem. C, 2014, 2, 6921–6939 RSC.
- J. Liu, R. Li and B. Yang, ACS Cent. Sci., 2020, 6, 2179–2195 CrossRef CAS PubMed.
- C. Zhao, X. Song, Y. Liu, Y. Fu, L. Ye, N. Wang, F. Wang, L. Li, M. Mohammadniaei and M. Zhang, J. Nanobiotechnol., 2020, 18, 1–32 Search PubMed.
- R. Ganji Arjenaki, G. Samieepour, S. E. Sadat Ebrahimi, M. Pirali Hamedani, M. Saffari, M. Seyedhamzeh, A. N. Kamali, A. Najdian and M. Shafiee Ardestani, Arabian J. Chem., 2024, 17, 105518 Search PubMed.
- J. Gao, L. Yuan, H. Jiang, G. Li, Y. Zhang, R. Zhou, W. Xian, Y. Zou, Q. Du and X. Zhou, Front. Pharmacol., 2024, 15, 1448308 CrossRef PubMed.
- H. Mu, J. Hu, Z. Lin, L. Wei, Q. Li, X. Wang, P. Geng, R. Zhong, S. Cui, W. Liu, C. Hu, G. Xu and G. Tan, Life Sci., 2024, 358, 123165 CrossRef CAS.
- J. Wang, Y. Liu, C. Xiu, X. Wang, Y. Liu, Y. Hu, J. Yang and Y. Lei, Diabetes, Metab. Syndr. Obes.: Targets Ther., 2024, 17, 3833–3846 CrossRef.
- H. B. Durumutla, A. Prabakaran, F. El Abdellaoui Soussi, O. Akinborewa, H. Latimer, K. McFarland, K. Piczer, C. Werbrich, M. K. Jain, S. M. Haldar and M. Quattrocelli, JCI Insight, 2024, 9, e182599 CrossRef.
- Q. Jiang, Y. Wen, P. Chen, X. Hong, R. Qian, J. Liu, J. Li, F. Huang and L. Han, Biomed. Chromatogr., 2024, e6007, DOI:10.1002/bmc.6007.
- Z. Fan and H. Shuai, Int. Immunopharmacol., 2024, 142, 113172 CrossRef CAS PubMed.
- S. Bi, Z. Xu, Z. Wang, Y. Liu, B. Yu, J. Tian, C. Liu, L. Qiao and Y. Zhang, Phytomedicine, 2024, 134, 155992 CrossRef CAS PubMed.
- P. Li, S. S. Hon, M. S. Tsang, L. L. Kan, A. Y. Lai, B. C. Chan, P. C. Leung and C. K. Wong, Int. J. Mol. Sci., 2024, 25 CAS.
- S. Bouchebti, Y. Gershon, A. Gordin, D. Huchon and E. Levin, Proc. Natl. Acad. Sci. U. S. A., 2024, 121, e2410874121 Search PubMed.
- J. Marin-Saez, R. Lopez-Ruiz, M. A. Faria, I. Ferreira and A. Garrido Frenich, J. Hazard. Mater., 2024, 480, 136192 CrossRef CAS PubMed.
- C. Lei, Z. Ding, M. Tao, Y. Lu, L. Xu, B. Cheng, C. Wang and Z. Wang, J. Agric. Food Chem., 2024, 72, 19710–19720 CrossRef CAS PubMed.
- K. I. Behringer, V. Fritz and M. Hellwig, J. Agric. Food Chem., 2024, 72, 19040–19050 CrossRef CAS PubMed.
- K. I. Behringer, J. Kapeluch, A. Fischer and M. Hellwig, J. Agric. Food Chem., 2024, 72, 5766–5776 CrossRef CAS.
- A. F. Reinertsen, S. Libreros, R. Nshimiyimana, C. N. Serhan and T. V. Hansen, ACS Pharmacol. Transl. Sci., 2023, 6, 1898–1908 CrossRef CAS.
- Y. Larsson, A. Mongelli, V. Kisielius and K. Bester, J. Hazard. Mater., 2024, 463, 132834 CrossRef CAS PubMed.
- Z. Bao, C. Guo, Y. Chen, C. Li, T. Lei, S. Zhou, D. Qi and Z. Xiang, Cell Rep., 2023, 42, 112718 CrossRef CAS PubMed.
- J. K. Sakkos, B. R. Mutlu, L. P. Wackett and A. Aksan, ACS Appl. Mater. Interfaces, 2017, 9, 26848–26858 CAS.
- M. Fisher, G. Bar-Nes, Y. Zeiri and A. Sivan, Biodegradation, 2008, 19, 321–328 CAS.
- A. M. Lauwers and W. Heinen, Arch. Mikrobiol., 1974, 95, 67–78 Search PubMed.
- S. L. Kumar, P. Sureka, A. Sowmitha, J. Sentisenla and M. Swathy, Eur. Polym. J., 2024, 113226 Search PubMed.
- S. Lee, A. Byun, J. Jo, J.-M. Suh, J. Yoo, M. H. Lim, J.-W. Kim, T.-H. Shin and J.-S. Choi, Nanoscale Adv., 2024, 6, 2177–2184 RSC.
- Q. Zhang, R. Yin, G. Guan, H. Liu and G. Song, Wiley Interdiscip. Rev.:Nanomed. Nanobiotechnol., 2024, 16, e1929 CAS.
- K. Y. Abdel-Halim, S. R. Osman, A. M. K. Nassar, A. Khozimy and H. M. El-Danasoury, Environ. Anal. Health. Toxicol., 2022, 37, e2022025–2022020 CrossRef PubMed.
- Y. Liu, H. Wen, X. Wu, M. Wu, L. Liu, J. Wang, G. Huo, J. Lyu, L. Xie and M. Dan, Nanomaterials, 2021, 11 Search PubMed.
- Q. Liang, C. Hou, Y. Tan, N. Wei, S. Sun, S. Zhang and J. Feng, Pest Manage. Sci., 2024, 80, 1314–1324 CrossRef CAS PubMed.
- P. Liu, Y. Huang, C. Zhan, F. Zhang, C. Deng, Y. Jia, T. Wan, S. Wang and B. Li, Mater. Today Bio, 2023, 21, 100722 CrossRef CAS.
- F. Guo, Y. Jiao, Y. Du, S. Luo, W. Hong, Q. Fu, A. Li, G. Wang and G. Yang, Int. J. Biol. Macromol., 2022, 220, 1133–1145 CrossRef CAS.
- J. Li, C. Zhou, J. Zhang, F. Xu, Y. Zheng, S. Wang and D. Zou, Colloids Surf., B, 2021, 205, 111852 CrossRef CAS PubMed.
- G. Liu, G. Lin, X. Lin, H. Zhou, H. Chen, L. Hao and X. Zhou, Environ. Sci. Pollut. Res. Int., 2019, 26, 25107–25116 CrossRef CAS.
- P. Sun, Q. Zhang, W. Nie, X. Zhou, L. Chen, H. Du, S. Yang, Z. You, J. He and C. He, ACS Biomater. Sci. Eng., 2019, 5, 6766–6778 CrossRef CAS PubMed.
- M. Qian, L. Chen, Y. Du, H. Jiang, T. Huo, Y. Yang, W. Guo, Y. Wang and R. Huang, Nano Lett., 2019, 19, 8409–8417 CrossRef CAS.
- P. Yuan, X. Mao, X. Wu, S. S. Liew, L. Li and S. Q. Yao, Angew. Chem., Int. Ed., 2019, 58, 7657–7661 CrossRef CAS.
- M. M. Lu, Y. Ge, J. Qiu, D. Shao, Y. Zhang, J. Bai, X. Zheng, Z. M. Chang, Z. Wang, W. F. Dong and C. B. Tang, Int. J. Nanomed., 2018, 13, 7697–7709 CrossRef CAS PubMed.
- J. G. Croissant and C. J. Brinker, Enzymes, 2018, 43, 181–214 CAS.
- X. Wang, X. Li, A. Ito, Y. Sogo, Y. Watanabe, N. M. Tsuji and T. Ohno, ACS Appl. Mater. Interfaces, 2017, 9, 43538–43544 CrossRef CAS.
- M. Kong, J. Tang, Q. Qiao, T. Wu, Y. Qi, S. Tan, X. Gao and Z. Zhang, Theranostics, 2017, 7, 3276–3292 CrossRef CAS PubMed.
- R. Agarwal, S. Adhikary, S. Bhattacharya, S. Goswami, D. Roy, S. Dutta, A. Ganguly, S. Nanda and P. Rajak, Environ. Sci. Adv., 2024, 3, 635–660 CAS.
- T. H. Chung, S. C. Hsu, S. H. Wu, J. K. Hsiao, C. P. Lin, M. Yao and D. M. Huang, Nanoscale, 2018, 10, 2998–3007 Search PubMed.
- X. Chang, X. Tang, J. Liu, Z. Zhu, W. Mu, W. Tang, Y. Zhang and X. Chen, Adv. Funct. Mater., 2023, 33, 2303596 CrossRef CAS.
- C. Zhou, M. Long, Y. Qin, X. Sun and J. Zheng, Angew. Chem., Int. Ed., 2011, 50, 3168–3172 CrossRef CAS PubMed.
- S. Reagen, Y. Wu, D. Sun, C. Munoz, N. Oncel, C. Combs and J. X. Zhao, Int. J. Mol. Sci., 2022, 23 Search PubMed.
- A. Khalid, R. Norello, A. Abraham, J. P. Tetienne, T. Karle, E. Lui, K. Xia, P. Tran, A. O’Connor, B. Mann and R. De Boer, Nanomaterials, 2019, 9 Search PubMed.
- P. Manivasagan, V. T. Nguyen, S. W. Jun, G. Hoang, S. Mondal, H. Kim, V. H. M. Doan, J. Kim, C. S. Kim and J. Oh, J. Controlled Release, 2019, 311–312, 26–42 CrossRef CAS PubMed.
- Y. Zhao, W. Liu, Y. Tian, Z. Yang, X. Wang, Y. Zhang, Y. Tang, S. Zhao, C. Wang, Y. Liu, J. Sun, Z. Teng, S. Wang and G. Lu, ACS Appl. Mater. Interfaces, 2018, 10, 16992–17003 CrossRef CAS.
- S. Balakrishnan, S. Mukherjee, S. Das, F. A. Bhat, P. Raja Singh, C. R. Patra and J. Arunakaran, Cell Biochem. Funct., 2017, 35, 217–231 CrossRef CAS PubMed.
- S. Balakrishnan, F. A. Bhat, P. Raja Singh, S. Mukherjee, P. Elumalai, S. Das, C. R. Patra and J. Arunakaran, Cell Prolif, 2016, 49, 678–697 CrossRef CAS PubMed.
- C. H. Chen, Y. J. Wu and J. J. Chen, Front. Biosci., 2016, 21, 1211–1221 CrossRef CAS.
- J. Liu, Y. Liang, T. Liu, D. Li and X. Yang, Nanoscale Res. Lett., 2015, 10, 218 CrossRef PubMed.
- D. Chen, F. Chen, J. Lu, L. Wang, F. Yao and H. Xu, J. Mater. Sci.: Mater. Med., 2024, 35, 6 CrossRef CAS PubMed.
- H. Ehzari and M. Safari, Front. Chem., 2022, 10, 881960 CrossRef CAS PubMed.
- Q. Ou, S. M. Tawfik, X. Zhang and Y. I. Lee, Analyst, 2020, 145, 4305–4313 RSC.
- D. Guo, P. Xu, D. Chen, L. Wang, Y. Zhu, Y. Zuo and B. Chen, Int. J. Nanomed., 2020, 15, 521–536 CrossRef CAS PubMed.
- S. M. Tawfik, M. Sharipov, S. Kakhkhorov, M. R. Elmasry and Y. I. Lee, Adv. Sci., 2019, 6, 1801467 CrossRef PubMed.
- M. Yu, K. Zhao, X. Zhu, S. Tang, Z. Nie, Y. Huang, P. Zhao and S. Yao, Biosens. Bioelectron., 2017, 95, 41–47 Search PubMed.
- D. A. Ananth, A. Rameshkumar, R. Jeyadevi, S. Jagadeeswari, N. Nagarajan, R. Renganathan and T. Sivasudha, Spectrochim. Acta, Part A, 2015, 138, 684–692 Search PubMed.
- P. Singh, A. A. Prabhune, S. B. Ogale and D. Guin, J. Mater. Chem. B, 2013, 1, 6538–6543 RSC.
- K. Yang, Z. Li, Y. Cao, X. Yu and J. Mei, Int. J. Mol. Sci., 2009, 10, 4418–4427 Search PubMed.
- C. Yi, Y. Yang, B. Liu, J. He and Z. Nie, Chem. Soc. Rev., 2020, 49, 465–508 Search PubMed.
- K. C. Lee, S. Eom, J. H. Ahn, J. Y. Kim, J.-H. Choy and Y. J. Lee, Nucl. Med. Biol., 2023, 126, 108620 Search PubMed.
- G. Choi, I. R. Jeon, H. Piao and J. H. Choy, Adv. Funct. Mater., 2018, 28, 1704470 Search PubMed.
- G. Wang, J.-h Ahn, J. Yao, M. Lindsay, H. Liu and S. Dou, J. Power Sources, 2003, 119, 16–23 Search PubMed.
- K. Shi, M. Ren and I. Zhitomirsky, ACS Sustainable Chem. Eng., 2014, 2, 1289–1298 CrossRef CAS.
- A. Muhulet, F. Miculescu, S. I. Voicu, F. Schütt, V. K. Thakur and Y. K. Mishra, Mater. Today Energy, 2018, 9, 154–186 CrossRef.
- C. Liu and H.-M. Cheng, J. Phys. D: Appl. Phys., 2005, 38, R231 Search PubMed.
- E. Frackowiak and F. Beguin, Carbon, 2002, 40, 1775–1787 CAS.
- J. Jyoti, T. K. Gupta, B. P. Singh, M. Sandhu and S. K. Tripathi, J. Energy Storage, 2022, 50, 104235 CrossRef.
Footnote |
† These authors contributed equally. |
|
This journal is © The Royal Society of Chemistry 2025 |
Click here to see how this site uses Cookies. View our privacy policy here.