DOI:
10.1039/D4NR03712J
(Review Article)
Nanoscale, 2025,
17, 53-64
Advances in Mg3Sb2 thermoelectric materials and devices
Received
10th September 2024
, Accepted 4th November 2024
First published on 5th November 2024
Abstract
Thermoelectric technology offers a green-viable and carbon-neutral solution for energy problems by directly converting waste heat to electricity. For years, Bi2Te3-based compounds have been the main choice materials for commercial thermoelectric devices. However, Bi2Te3 comprises scarce and toxic tellurium (Te) elements, which might limit its large-scale application. Recently, Mg3Sb2 compounds have drawn increasing attention as an alternative to Bi2Te3 thermoelectrics due to their excellent thermoelectric performance. Enabled by effective strategies such as optimizing carrier concentration, introducing point defects, and manipulating carrier scattering mechanisms, Mg3Sb2 compounds have realized an improved thermoelectric performance. In this review, optimizing strategies for both Mg3Sb2-based thermoelectric materials and devices are discussed. Moreover, the flexibility and plasticity of Bi-alloyed Mg3Sb2 mainly stemming from the dense dislocations are outlined. The above strategies summarized here for enhancing Mg3Sb2 thermoelectrics are believed to be applicable to many other thermoelectrics.
Introduction
Thermoelectric technology with the advantages of portable, emission-free, and quiet device design enables conversion from waste heat to electricity, which has been regarded as one of the promising solutions to energy problems. Until now, major high-performance materials used for thermoelectric devices usually contained toxic or high-cost elements (such as Pb, Ge, and Te).1–4 Besides, the realizable thermoelectric conversion efficiencies are still inferior to those of other renewable technologies such as photovoltaic5 and lithium batteries,6 which limits the large-scale application of thermoelectric technology. The key challenges for improving thermoelectric conversion efficiency are the complexity of thermoelectric device fabrication and the enhancement of the thermoelectric performance of the materials, which could be determined by the thermoelectric figure of merit, zT = S2σT/(κe + κL), where σ, S, T, κe, and κL are the electrical conductivity, Seebeck coefficient, absolute temperature, and the electronic and lattice components of thermal conductivity, respectively.7
Many strategies including band structure manipulation,8–10 carrier concentration optimization11,12 and lattice thermal conductivity reduction by the introduction of point defects,13,14 dislocation,15,16 strong anharmonicity,17,18 complex crystal structures,19–21 and weak atomic bonding22,23 have been proven to be effective at improving the thermoelectric performance of materials such as PbTe,4 GeTe,1,24 half-Heusler,25,26 and CoSb3 materials.27 All the above thermoelectric materials mentioned successfully realize zT-improvements at mid- to high-temperature (T > ∼600 K), while there exist limitations on the low-grade waste heat application (T < ∼600 K) for these materials. For a century, the only choice for commercial thermoelectric devices for low-grade waste heat thermoelectric power generation and solid-state cooling is Bi2Te3-based thermoelectrics because of the facile fabrication process of devices and improved thermoelectric performance near room temperature. However, Bi2Te3-based thermoelectrics contain the toxic and scarce element tellurium (Te), which might limit its large-scale application. Additionally, the maximal thermoelectric performance of Bi2Te3 appears at ∼400–500 K, and then the thermoelectric performance degrades when the temperature is above 500 K, which could be understood from the bipolar effect. Therefore, there is an urgent need to explore alternative non-toxic, low-cost, and abundant materials for Bi2Te3.
Recently, Mg-based thermoelectrics including MgAgSb,28,29 Mg2Si,29 and Mg3Sb230–32 compounds have drawn increasing attention due to their outstanding thermoelectric performance. In MgAgSb-based compounds, a higher phase purity and small grain size enable a zT close to 1 near room temperature and a zT of ∼1.4 at 475 K, demonstrating its potential to replace Bi2Te3 thermoelectrics.28 In addition to MgAgSb thermoelectrics, Mg2(Si,Sn) with a zT ∼ 1.3 at 700 K is a promising alternative choice for Bi2Te3 as well.33 In order to prove the high-performance of these Mg-based materials, various thermoelectric devices have been fabricated. A thermoelectric conversion efficiency of ∼7.5%29 is demonstrated in a Mg2(Si,Sn) device at 600 K, indicating its possible application in low-grade waste heat. Among the above Mg-based thermoelectrics, Mg3Sb2-based compounds showing similar laminar structural features with local weak bonding to those of Bi2Te3 realize intrinsically low lattice thermal conductivity.34 They once became star thermoelectric materials due to their ultra-low prices35 (Fig. 1a) and extraordinary thermoelectric performances,30,31 which motivated us to focus on Mg3Sb2 thermoelectrics in this review.
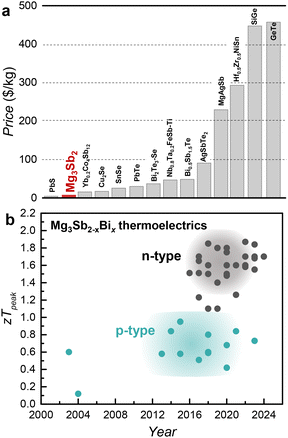 |
| Fig. 1 (a) Cost ($ per kg) of raw materials for thermoelectrics.40 Copyright 2020, Wiley. (b) Peak figure of merit (zTpeak) for Mg3Sb2−xBix thermoelectrics.36,37,39,41,45,47–74 | |
The thermoelectric performance of Mg3Sb2 and Mg3Bi2 compounds was first reported in 2003.36 In the following years, Mg3Sb2-based compounds were studied as p-type thermoelectric materials. Until 2016, n-type Mg3Sb2−xBix with a significant zT ∼ 1.5 at 700 K has been reported,37 which led to Mg3Sb2−xBix once becoming the most efficient and popular among Zintl compounds. The higher zT realized in n-type Mg3Sb2 compared to that of p-type Mg3Sb2 mainly stems from the superior power factor induced by the larger conduction band degeneracy (Nc = 6) in n-type Mg3Sb2 as compared to valence band degeneracy (Nv = 1).38 With further optimization by effective strategies such as optimization of carrier concentration,39–41 manipulation of the carrier scattering mechanism,42,43 strengthened phonon scattering,44 an improved peak zT ∼ 1.8 at high temperature45 (Fig. 1b) and a peak zT of ∼1.02 near room temperature46 in n-type Mg3Sb2−xBix compounds were realized. Such a high zT near room temperature mainly stems from the manipulation of the carrier scattering mechanism for enhancing the mobility of the Mg3Sb2−xBix compounds, the reduction of the lattice thermal conductivity through tuning the Bi/Sb ratio, and the optimization of the carrier concentration by doping heterovalent atoms, making Mg3Sb2−xBix a promising alternative to n-type Bi2Te3.
In this review, representative strategies for improving Mg3Sb2 thermoelectric materials and devices are summarized. At a material level, electronic strategies such as carrier concentration optimization, carrier scattering mechanism manipulation, and thermal strategies by the introduction of a phonon scattering source are outlined. At a device level, the methods adopted for selecting contact layers for devices and prospects for cooling and electricity generation are discussed. In addition, Mg3Sb2−xBix-based plastic semiconductors show a possibility for flexible device application. This review is expected to inspire new insights into advancing Mg3Sb2 thermoelectrics, which may be useful for improving other thermoelectric materials.
Materials properties
According to the Zintl compound perspective, Mg3Sb2 is categorized as a CaAl2Si2-type compound, and its crystal structure is shown in Fig. 2a. It is recognized that Mg atoms occupy two different sites in the lattice. One (Mg1 atom) is located at the octahedral site surrounded by six equivalent Sb atoms and the other (Mg2 atom) is at the tetrahedral site surrounded by four asymmetric Sb atoms. The [Mg2Sb2]2− polyanion framework is covalently bonded in the ab-plane, while Mg2 sits at the interlayers of polyanions and is ionically bonded to the polyanions. Such different bond strength between inter- and intra-layers allows an isotropic atomic structure only along the crystallographic ab plane but leaves a strong difference along the c direction. The bond strength between the inter- and intra-layers helps in understanding the anisotropic of Hall mobility, lattice thermal conductivity, and overall thermoelectric performance along ab-plane and c-axis direction (Fig. 2b).75,76
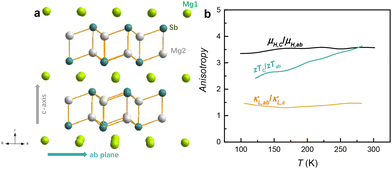 |
| Fig. 2 (a) Crystal structure of Mg3Sb2. (b) Anisotropy factors of Hall mobility, lattice thermal conductivity, and zT for single-crystalline Mg3Sb2.76 | |
Vacancies at Mg and Sb sites are pronounced in Mg3Sb2 compounds, which could be tuned by its stoichiometry. Low-energy electron acceptor defect VMg2− (MgMg → VMg2− + 2h+) in Mg-deficiency case produces holes h+, resulting in a reduction of the energy at the Fermi level (EF) close to equilibrium EF and vacancy concentration30 (Fig. 3a). In a Mg-deficiency case, even if introducing n-type dopants (such as Te), the intrinsic acceptor defects pin the EF, thus making material to be always of p-type.30 Fortunately, excess Mg still allows a single phase thermodynamically according to the binary phase diagram (Fig. 3b). n-type Mg3Sb2 is realizable in the Mg-excess case, which could be explained by the enhanced Mg vacancy formation (Fig. 3a).
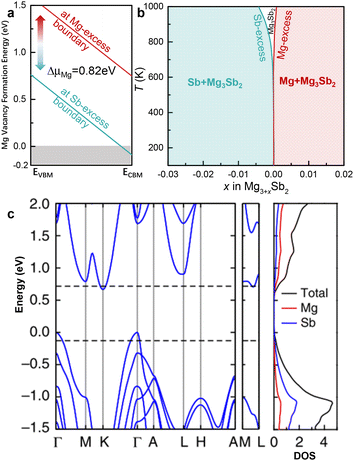 |
| Fig. 3 (a) Mg-vacancy formation energy as a function of Fermi level (EF).30 (b) Calculated Mg–Sb phase diagram30 for Mg3+xSb2. (c) Calculated band structure for Mg3Sb2.55 (a) and (b) Adapted with permission, Copyright 2017, Cell Press.30 (c) Reproduced under the terms of the CC-BY Creative Commons Attribution 4.0 International License.55 | |
Mg defects play pivotal roles in the thermoelectric properties of Mg3Sb2.77 Until now, different methods have been adopted to manipulate Mg vacancies for improving n-type Mg3Sb2 thermoelectrics. Mn-doping is found to increase the formation energies of vacancy at the Mg1 site, helping to stabilize the Mg atom via suppressing its evaporation during the sintering process, thus reducing the concentration of Mg vacancies.78 Moreover, Bi-deficiency leads to the coalescence of the Mg vacancies, which reduces the scattering of charge carriers and strengthens the phonon scattering.79
The calculated band structure reveals that Mg3Sb2 is an indirect semiconductor, with a band gap of ∼0.8 eV (Fig. 3c). The band gap of Mg3Sb2 reported by other calculation methods ranges from 0.4 to 0.7 eV.32,53 It is observed that the valence band maximum (VBM) is located at Γ point, the center of the first Brillouin zone, which is attained from the p-orbitals of Sb. The valence band at the Γ point only offers 1 band degeneracy (Nv) in the Mg3Sb2 compound. In contrast, the conduction bands (CBM) involve a higher degeneracy band (Nc = 6) along the L–M direction in the Brillouin zone. In a higher carrier concentration case, a possible conduction band (Nc = 2) located at K point can participate in electronic transport, resulting in a total Nv = 8. Higher band degeneracy enables more carriers to contribute to electronic transport, thus improving the electrical conductivity without sacrificing the Seebeck coefficient. Higher Nc of conduction bands than that of valence bands helps to explain the superior thermoelectric performance of n-type Mg3Sb2 compounds than that of the p-type Mg3Sb2 (Fig. 1b). In order to tune the band edge of CBM for improving n-type Mg3Sb2 thermoelectrics, some ionic cations such as Yb and Ca have been considered to dissolve at the octahedral Mg sites for reducing the bonding covalency of CBM in a theoretical study.80
Materials optimization strategy
Optimizing the Hall carrier concentration is utilized on both p-type and n-type Mg3Sb2 thermoelectrics. Pristine Mg3Sb2 shows a p-type characteristic conduction due to the unavoidable vacancies at Mg sites. However, the low Hall carrier concentration results in poor electrical performance. In order to enhance the Hall carrier concentration, different element species are doped such as Pb48 and Bi47 at anion sites and Ag,52 Li,63–65 and Na50 at cation sites. Co-doping Na/Zn is found to synergistically optimize the carrier concentration along with manipulation of the band structure.81 According to the calculated band structure of Mg3Sb2 (Fig. 3c), the electrical performance of p-type Mg3Sb2 is strongly dependent on Γ bands, which are dominated by the p orbitals (including px,y, and pz orbitals) of Sb anions. Zn-doping leads to substitution at the Mg2 site for aligning px,y, and pz orbitals, resulting in a high band degeneracy.81 Moreover, Li/Cd65 and Na/Cd72 co-doping is used to synergistically optimize p-type thermoelectrics as well.
During the synthesis process of Mg3Sb2 samples, loss of Mg at high temperatures due to its high vapor pressure and reactivity leads to Mg3Sb2 generally showing p-type conduction characteristics. The addition of an optimized amount of excess Mg turns material into n-type conduction, while it is insufficient to optimize the Hall carrier concentration of Mg3Sb2. Various dopants reported on increasing the Hall carrier concentration of Mg3Sb2−xBix thermoelectrics with Mg excess, such as chalcogenide (S, Se, and Te)53,59 and rare-earth elements39,41,66,67,82,83 (Gd, La, Tm, Ho, Nd, Ce, and Pr). Additionally, Y shows a high doping efficiency on Mg3Sb2−xBix, enabling an increased Hall carrier concentration ∼1 × 1020 cm−3 (Fig. 4); thus, an improved zT ∼ 1.8 has been realized.57
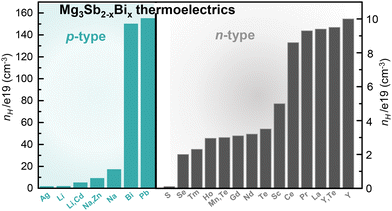 |
| Fig. 4 Maximal Hall carrier concentration for n-type39,41,57–59,66,67,73 and p-type47,48,50,52,63,65,81 Mg3Sb2−xBix thermoelectrics doped with various elements at room temperature. | |
Bi-alloying is widely used in advancing n-type Mg3Sb2 thermoelectrics, of which the main purpose is to suppress the lattice thermal conductivity and inertial effective mass (
). Existing publications have shown that Mg3Sb1.5Bi0.5 based materials have excellent thermoelectric performance at room temperature.55,78 Zhang et al. found that Mg3Sb1.5Bi0.5 with Te-doping is promising for low- and intermediate-temperature thermoelectric applications.55 Shu et al. reported that Mg3Sb1.5Bi0.5 shows two times higher mechanical toughness than n-type Bi2Te3.78 Later, Mg3SbBi with a high average zT below 600 K was recognized as a strong candidate to replace the conventional n-type Bi2Te3-based thermoelectrics.62
The schematic band structure, calculated band gap, weighted mobility (μw), lattice thermal conductivity (more precisely κ − LσT) and zT of Mg3Sb2−xBix (0 ≤ x ≤ 2) are shown in Fig. 5a–d. It is found that μw increases and (κ − LσT) reduces with x increasing from 0 to 1 for the Mg3Sb2−xBix compounds. The increase of μw could be understood by the reduced inertial effective mass (
).44 In addition, large mass/strain fluctuations of Bi/Sb alloying defects and the strong reduction in sound velocity44 lead to a lattice thermal conductivity reduction. These result in a zT-improvement with x increasing from 0 to 1 for the Mg3Sb2−xBix compounds. When x is above 1, a reduction of zT appears mainly due to the bipolar effect induced by Bi alloying. Mg3Bi2 is approximately a degenerate semiconductor with slightly overlapped conduction and valence bands (Fig. 5a). This could explain why Bi-alloying leads to a reduction of the band gap (Fig. 5b), resulting in a reduction of μw and zT and an increase of lattice thermal conductivity with the increase of x from 1 to 2 due to the bipolar effect (Fig. 5c–e). A maximal μw and minimal (κ − LσT) enable a maximal zT ∼ 0.8 that appears at x = 1, which is competitive with those of Bi2Te3 thermoelectrics.44
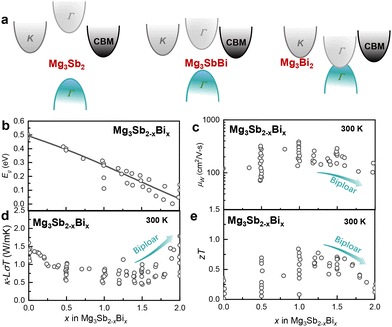 |
| Fig. 5 (a) The schematic band structure and (b) calculated band gap44 for Mg3Sb2−xBix alloys. (c) Weighted mobility (μw),32,44 (d) lattice thermal conductivity (more precisely κ − LσT)32,44 and (e) zT 32,44 of Mg3Sb2−xBix (0 ≤ x ≤ 2) alloys at 300 K. | |
In addition to Bi-alloying and doping various elements for tuning the carrier concentration, manipulation of the carrier scattering mechanism is crucial for enhancing the thermoelectric performance of Mg3Sb2−xBix alloys, especially near room temperature. The mobility of n-type Mg3Sb2-based materials is firstly attributed to ionization scattering,84 which sacrifices its mobility and limits its enhancement of thermoelectric performance as compared to the cases of pure acoustic phonon scattering. Nevertheless, Te-doped Mg3Sb2 single crystals without ionized impurity scattering prove that the thermally activated mobility previously observed in polycrystalline materials was caused by grain boundary resistance.85
As shown in Fig. 6a, the Hall mobility increases with the grain size enlarging. In order to enhance the mobility, many efforts are focused on enlarging the grain size. Changing the sintering process is one way to obtain coarse-grain Mg3Sb2−xBix alloys. Shi et al. used melting and hot-deforming techniques to increase the grain size.57 Jia et al. reported that increasing the sintering temperature enables an increase in grain size.43 Optimizing the sample synthesis method is another way to enlarge grain size. Chen et al. grew coarse-grained Mg3Bi2−xSbx crystals with an average grain size of ∼800 μm, leading to a high Hall carrier mobility of ∼210 cm2 V−1 s−1.42 Note that changing both the temperature and time of synthesizing Mg3Sb2-based compounds has the effect of increasing grain sizes. Enhancing the grain size with other heat treatment techniques at various times and temperatures is also expected. Besides, other dopants such as Nb40 and Co43 have the effect of increasing the Hall mobility by grain boundary engineering strategies. Nb-doping is recognized to wet the grain boundary and then speed up the grain growth, resulting in a reduced grain boundary resistivity40 (Fig. 6b). As shown in Fig. 6c, Co-doping enables an increased Hall mobility without enlarging the grain size, which explains that Co-doping affects the electron scattering mechanism by intentionally introducing the point defects.43
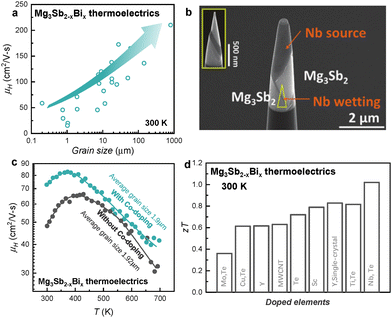 |
| Fig. 6 (a) Grain size dependent Hall mobility at 300 K for Mg3Sb2−xBix thermoelectircs.32,42,43,45,86–88 (b) Scanning electron microscope (SEM) image of a needle-shaped atom probe tomography (APT) specimen for Mg3Sb2-Nb samples.40 (c) Temperature-dependent Hall mobility for Mg3Sb2−xBix-Te compounds with and without Co-doping.43 (d) zT for Mg3Sb2−xBix thermoelectrics at 300 K.45,46,57,62,86,88–91 (b) Reproduced under the terms of the CC-BY Creative Commons Attribution 4.0 International License.40 (c) Reproduced under the terms of the CC-BY Creative Commons Attribution 4.0 International License.43 | |
Combining the strategies of nH optimization, Bi-alloying, and manipulation of the scattering mechanism, the zT at both room temperature (Fig. 6d) and high temperatures (Fig. 1b) of Mg3Sb2−xBix thermoelectrics has been significantly improved. Since 2019, the thermoelectric performance of Mg3Sb2−xBix thermoelectrics near room temperature has been a focus of research. Up to now, Mg3Sb2-based thermoelectrics have been recognized as one of the most promising alternative choices to Bi2Te3-based thermoelectrics due to its non-toxic elements and exceptional near-room temperature thermoelectric performance. Shi et al. realized a zT ∼ 0.8 at 300 K and ∼1.3 at 500 K by Sc-doping.89 Y-doped single-crystal Mg3Sb2−xBix achieved a zT ∼ 0.8 near room temperature mainly due to high weighted mobility.90 Besides, co-doping of elements such as Mo/Te,45 Nb/Te,46 Cu/Te,91 and Ti/Te86 allowed synergetic effects on enhancing thermoelectric performance.
In addition to the high thermoelectric performance of materials for enhanced conversion efficiency and refrigeration capacity, the good thermal stability of Mg3Sb2−xBix materials is also important to ensure the long-term performance of devices. Mg3Sb2-based thermoelectrics are sensitive to water vapor and oxygen, and they tend to decompose spontaneously to Bi/Sb and Mg(OH)2 in humid ambient air.96 Such decomposition leads to Mg deficiency in the matrix, thus a significant deterioration of thermoelectric performance for both materials and devices. In order to extend the service life of Mg3Sb2-based thermoelectrics, different effective strategies have been adopted. By eliminating moisture in the air, the thermoelectric properties of Mg3Sb2−xBix compounds remained nearly unchanged for two months.96 For Mg3Sb2−xBix thermoelectrics, boron nitride95 or polydimethylsiloxane97 was coated onto the surfaces to avoid Mg loss in humid ambient air and enhance the reliability of the devices. In addition to isolating Mg3Sb2−xBix and humid ambient air, inhibiting the formation of Mg vacancies by doping elements such as Er,71 Co,71 Mg2B,93 Mn,94 and CrMnFeCoCu92 has been found to effectively improve thermal stability (Fig. 7), paving a path for further long-term device application.
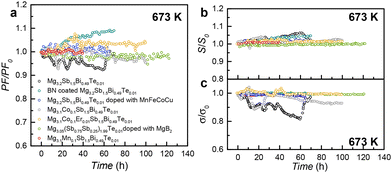 |
| Fig. 7 Long-term electronic property measurements including PF/PF0 (a), S/S0 (b), and σ/σ0 for Mg3Sb2−xBix-1%Te thermoelectrics alloyed with MnFeCoCu,92 Co,71 Er,71 MgB2,93 Mn,94 or coated with BN95 at 673 K. | |
Mg3Sb2−xBix based thermoelectric devices
Various thermoelectric devices are fabricated to demonstrate the high-zT of Mg3Sb2−xBix compounds. Selecting a candidate contact layer for Mg3Sb2−xBix thermoelectric devices is the first and crucial step because of its possibility of impacting the thermal stability, interface resistivity, and thermal resistance of the devices. Up to now, strategies such as the high-throughput method98 (Fig. 8a) and the calculation of the phase diagram method99 (Fig. 8b) were used to select contact layers for Mg3Sb2−xBix thermoelectric devices, in which the coefficient of thermal expansion, shear strengths, and contact resistivity are taken into consideration. Both metal elements and alloys as contact layers for Mg3Sb2−xBix thermoelectric devices are summarized in Fig. 8c.
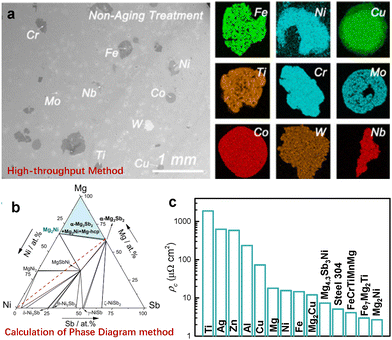 |
| Fig. 8 (a) High-throughput method98 and (b) calculation of the phase diagram method99 for screening the Mg3Sb2−xBix contact layer. (c) Contact resistivity of several contact materials for Mg3Sb2−xBix thermoelectrics.99–102 (a) Adapted with permission, Copyright 2022, Elsevier.98 (b) Reproduced under the terms of the CC-BY Creative Commons Attribution 4.0 International License.99 | |
Several types of metal powders were mixed and then co-sintered, which could be defined as a high-throughput method for quickly investigating interfacial reaction and diffusion between pure metals and Mg3Sb2−xBix alloys (Fig. 8a). These metals start from a chemical reaction with Mg3Sb2−xBix and then diffuse into the matrix material. Rapid diffusion causes deterioration of the electrical and thermal properties of devices; thus they are not good choices for contact layers. The interfacial reaction energy and activation energy barrier calculated from first principles can help us to better understand the atomic migration, and select candidate contact layers.103 Experimentally, Ni and Fe demonstrate relatively lower contact interface resistivity, which is the reason for being the primary choices as contact layers in many previous publications.44,100 The general design strategy by considering the coefficient of thermal expansion, shear strengths, and contact resistivity was then adopted to select contact layers such as the Fe7Mg2Cr,98 Fe7Mg2Ti,98 and FeCrTiMnMg compounds.101 Fe7Mg2Cr and Fe7Mg2Ti as contact layers displayed a low contact resistivity (<10 μΩ cm2) after aging treatment at 723 K for 15 days.98 An FeCrTiMnMg interface exhibits low contact resistivity of ∼4.0 μΩ cm2 and high shear strength of 36.7 MPa.101
Another typical method of exploring candidate contact layers is from phase diagrams. Mg2Cu is thermodynamically stable in the phase region of Mg-Mg2Cu-Mg3Bi1.5Sb0.5, making it a more reliable choice of contact layer than Fe.104 Mg2Cu with a low melting point (853 K) could be facilely hot-pressed on Mg3Sb2−xBix without sacrificing thermoelectric properties.104 Later, Yin et al. explored the calculation of the phase diagram method to describe the thermodynamic properties of materials quantitatively and then screened Mg2Ni as a thermodynamically stable contact layer for Mg3Sb2−xBix-based thermoelectric devices.99
Mg3Sb2−xBix compounds achieve excellent thermoelectric performance at both room and high temperatures, which enables the possibility of application on both power generation and cooling. As illustrated in Fig. 9a, single leg with high thermoelectric conversion efficiency is realizable, demonstrating that the high zT attained from the materials is reliable. In addition, Mg3Sb2−xBix based thermoelectric modules are successfully fabricated and a maximal thermoelectric conversion efficiency of ∼14.5% is demonstrated in Mg3Sb2−xBix/TAGS modules.111 It is noteworthy that the conversion efficiency of Mg3Sb2−xBix based thermoelectrics is competitive with that of commercial Bi2Te3 near room temperature. At higher temperatures, Mg3Sb2−xBix based thermoelectrics overpower Bi2Te3 thermoelectrics in terms of conversion efficiency. Additionally, the long-term stability of Mg3Sb2−xBix thermoelectric devices may be achieved by selecting reliable contact layers and improving the thermal stability of materials. Doping with MgB2
93 leads to nearly unchanged output power in Mg3Sb2−xBix based thermoelectric single leg over time. By adopting a suitable diffusion barrier layer such as FeCrTiMnMg,101 the Mg3Sb2−xBix thermoelectric device also showed high reliability.
Fig. 9b shows the cooling temperature difference achieved in Mg3Sb2−xBix based thermoelectric devices. Mao et al. investigated its cooling performance for the first time by constructing a uni-couple consisting of n-type Mg3.2Sb0.5Bi1.498Te0.002 and p-type Bi0.5Sb1.5Te3, of which a maximal cooling temperature of ∼90 K was realized.113 Later, MgAgSb thermoelectrics were chosen as p-type legs subsequently for assembling Te-free modules.114 Noting that much effort has been devoted to advancing conversion efficiency, there is still room for improving the cooling capability of Mg3Sb2−xBix based thermoelectrics.
Plasticity and flexibility
Many plastic and flexible semiconductors such as InSe,117 Ag2S,118 GaSe,119 and SnSe2
120 have been uncovered and have drawn much attention due to their possible applications in wearable health monitor equipment. Among these semiconductors, only Ag2(S, Se, and Te) and SnSe2 exhibit the possibility of thermoelectric application near room temperature. Recently, the plasticity and flexibility of Mg3Sb2−xBix based thermoelectrics were studied.82,92 It is found that wires of Mg3Sb2−xBix compounds obtained during the lathing are thin and twisty.82 Besides, compressive stress–strain measurements of Mg3Sb2−xBix show that its strain could reach ∼30% at 573 K, demonstrating promising plasticity.92 Zhao et al. revealed that dense gliding of dislocations is the main mechanism for the plasticity of the Mg3Bi2 thermoelectrics.121 Edge dislocations with Burgers vector b = c [0001] corresponding to the slip plane (1210) and edge dislocations with Burgers vector b = c/2 [0001] corresponding to the slip plane (0110) were observed, suggesting a slip of the atomic plane along the [0001] direction during plastic deformation. This led to a large tensile strain of ∼100% along the (0001) plane.121 Both in-plane and out-of-plane flexible thermoelectric devices with Cu as electrodes were fabricated and the output power is shown in Fig. 10.116 The interface between Cu and materials limits the high output power density of the devices; therefore, it is important to explore suitable electrodes for improving the flexible Mg3Sb2−xBix thermoelectric devices.
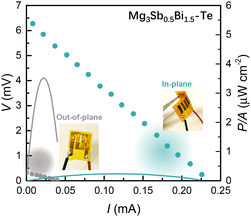 |
| Fig. 10 Current-dependent voltage (V) and output power density (P/A) for in-plane and out-of-plane Mg3Sb0.5Bi1.498Te0.002 thermoelectric devices.116 Reproduced under the terms of the CC-BY Creative Commons Attribution 4.0 International License.116 | |
Summary and outlook
Improvements in Mg3Sb2−xBix materials using strategies such as manipulation of carrier concentration, carrier scattering mechanism, and suppression of lattice thermal conductivity synergistically enable a high thermoelectric conversion efficiency and large cooling capacity. Here, we summarize the various strategies for enhancing the thermoelectric performance of Mg3Sb2−xBix materials and outline thermoelectric conversion efficiency and thermoelectric cooling capability in Mg3Sb2−xBix based devices. With competitive thermoelectric conversion efficiency and cooling temperature to those of commercial Bi2Te3 devices, Mg3Sb2−xBix based devices are becoming a popular option for refrigeration and power generation near room temperature. The stability of both materials and devices is crucial for the long-term service of Mg3Sb2−xBix thermoelectrics. The thermal stability of n-type Mg3Sb2−xBix materials is closely related to the Mg vacancies; an increase in Mg-vacancy formation energy enhances thermal stability. Such Mg-vacancy formation energy can be calculated using DFT, which is believed to be useful for quickly selecting doping elements for enhancing the thermal stability of Mg3Sb2−xBix materials. Additionally, the plasticity and flexibility of Mg3Sb2−xBix thermoelectrics are discussed, demonstrating its promising application in wearable devices. It is believed that this review provides insights into advancing Mg3Sb2−xBix materials and devices, which can also be useful for other thermoelectrics.
Data availability
Data are available on reasonable request from the authors.
Conflicts of interest
There are no conflicts to declare.
Acknowledgements
This work is supported by the Research Grants Council of Hong Kong (C7002-22Y and 17318122).
References
- X. Zhang, Z. Bu, S. Lin, Z. Chen, W. Li and Y. Pei, GeTe Thermoelectrics, Joule, 2020, 4(5), 986–1003 CrossRef CAS.
- Y. Sun, F. Guo, Y. Feng, C. Li, Y. Zou, J. Cheng, X. Dong, H. Wu, Q. Zhang, W. Liu, Z. Liu, W. Cai, Z. Ren and J. Sui, Performance Boost for Bismuth Telluride Thermoelectric Generator via Barrier Layer Based on Low Young's Modulus and Particle Sliding, Nat. Commun., 2023, 14(1), 8085 CrossRef CAS PubMed.
- G. Yang, R. Niu, L. Sang, X. Liao, D. R. G. Mitchell, N. Ye, J. Pei, J. F. Li and X. Wang, Ultra–High Thermoelectric Performance in Bulk BiSbTe/Amorphous Boron Composites with Nano–Defect Architectures, Adv. Energy Mater., 2020, 2000757 CrossRef CAS.
- Y. X. Wu, Z. W. Chen, P. F. Nan, F. Xiong, S. Q. Lin, X. Y. Zhang, Y. Chen, L. D. Chen, B. H. Ge and Y. Z. Pei, Lattice Strain Advances Thermoelectrics, Joule, 2019, 3(5), 1276–1288 CrossRef CAS.
- B. Ding, Y. Ding, J. Peng, J. Romano-deGea, L. E. K. Frederiksen, H. Kanda, O. A. Syzgantseva, M. A. Syzgantseva, J. N. Audinot, J. Bour, S. Zhang, T. Wirtz, Z. Fei, P. Dörflinger, N. Shibayama, Y. Niu, S. Hu, S. Zhang, F. F. Tirani, Y. Liu, G. J. Yang, K. Brooks, L. Hu, S. Kinge, V. Dyakonov, X. Zhang, S. Dai, P. J. Dyson and M. K. Nazeeruddin, Dopant-Additive Synergism Enhances Perovskite Solar Modules, Nature, 2024, 628(8007), 299–305 CrossRef CAS.
- Q. Wang, Z. Yao, J. Wang, H. Guo, C. Li, D. Zhou, X. Bai, H. Li, B. Li, M. Wagemaker and C. Zhao, Chemical Short-Range Disorder in Lithium Oxide Cathodes, Nature, 2024, 629(8011), 341–347 CrossRef CAS.
-
A. F. Ioffe, Semiconductor Thermoelements, and Thermoelectric Cooling, Infosearch, London, 1957 Search PubMed.
- Y. Pei, X. Shi, A. LaLonde, H. Wang, L. Chen and G. J. Snyder, Convergence of Electronic Bands for High Performance Bulk Thermoelectrics, Nature, 2011, 473, 66–69 CrossRef CAS PubMed.
- Q. Zhang, F. Cao, W. Liu, K. Lukas, B. Yu, S. Chen, C. Opeil, D. Broido, G. Chen and Z. Ren, Heavy Doping and Band Engineering by Potassium to Improve the Thermoelectric Figure of Merit in p-Type PbTe, PbSe, and PbTe1−ySey, J. Am. Chem. Soc., 2012, 134(24), 10031–10038 CrossRef CAS PubMed.
- Y. Pei, A. D. LaLonde, N. A. Heinz and G. J. Snyder, High Thermoelectric Figure of Merit in PbTe Alloys Demonstrated in PbTe–CdTe, Adv. Energy Mater., 2012, 2(6), 670–675 CrossRef CAS.
- Y. Pei, Z. M. Gibbs, B. Balke, W. G. Zeier and G. J. Snyder, Optimum Carrier Concentration in n-Type PbTe Thermoelectrics, Adv. Energy Mater., 2014, 4, 1400486 CrossRef.
- M. Zhou, Z. M. Gibbs, H. Wang, Y. Han, C. Xin, L. Li and G. J. Snyder, Optimization of Thermoelectric Efficiency in SnTe: The Case for the Light Band, Phys. Chem. Chem. Phys., 2014, 16(38), 20741–20748 RSC.
- X. Zhang, J. Li, X. Wang, Z. Chen, J. Mao, Y. Chen and Y. Pei, Vacancy Manipulation for Thermoelectric Enhancements in GeTe Alloys, J. Am. Chem. Soc., 2018, 140(46), 15883–15888 CrossRef CAS.
- S. Roychowdhury, R. K. Biswas, M. Dutta, S. K. Pati and K. Biswas, Phonon Localization and Entropy-Driven Point Defects Lead to Ultralow Thermal Conductivity and Enhanced Thermoelectric Performance in (SnTe)1–2x(SnSe)x(SnS)x, ACS Energy Lett., 2019, 4(7), 1658–1662 CrossRef CAS.
- Z. Chen, B. Ge, W. Li, S. Lin, J. Shen, Y. Chang, R. Hanus, G. J. Snyder and Y. Pei, Vacancy-Induced Dislocations within Grains for High-Performance PbSe Thermoelectrics, Nat. Commun., 2017, 8, 13828 CrossRef CAS PubMed.
- S. I. Kim, K. H. Lee, H. A. Mun, H. S. Kim, S. W. Hwang, J. W. Roh, D. J. Yang, W. H. Shin, X. S. Li and Y. H. Lee, Dense Dislocation Arrays Embedded in Grain Boundaries for High-Performance Bulk Thermoelectrics, Science, 2015, 348(6230), 109–114 Search PubMed.
- G. Ribeiro, L. Paulatto, R. Bianco, I. Errea, F. Mauri and M. Calandra, Strong Anharmonicity in the Phonon Spectra of PbTe and SnTe from First Principles, Phys. Rev. B, 2018, 97, 014306 CrossRef CAS.
- U. Aseginolaza, R. Bianco, L. Monacelli, L. Paulatto, M. Calandra, F. Mauri, A. Bergara and I. Errea, Strong Anharmonicity and High Thermoelectric Efficiency in High-Temperature SnS from First Principles, Phys. Rev. B, 2019, 100, 214307 CrossRef CAS.
- G. Snyder, M. Christensen, E. Nishibori, T. Caillat and B. Iversen, Disordered Zinc in Zn4Sb3 with Phonon-Glass and Electron-Crystal Thermoelectric Properties, Nat. Mater., 2004, 3(7), 458–463 CAS.
- S. Lin, W. Li, S. Li, X. Zhang, Z. Chen, Y. Xu, Y. Chen and Y. Pei, High Thermoelectric Performance of Ag9GaSe6 Enabled by Low Cutoff Frequency of Acoustic Phonons, Joule, 2017, 1(4), 816–830 CrossRef CAS.
- B. Jiang, P. Qiu, J. Huang, T. Mao, Y. Wang, Q. Song, D. Ren, X. Shi and L. Chen, Entropy Optimized Phase Transitions and Improved Thermoelectric Performance in N-Type Liquid-Like Ag9GaSe6 Materials, Mater. Today Phys., 2018, 8, 20 Search PubMed.
- H. Liu, X. Shi, F. Xu, L. Zhang, W. Zhang, L. Chen, Q. Li, C. Uher, T. Day and G. J. Snyder, Copper Ion Liquid-Like Thermoelectrics, Nat. Mater., 2012, 11(5), 422–425 CAS.
- K. Zhao, P. Qiu, Q. Song, A. B. Blichfeld, E. Eikeland, D. Ren, B. Ge, B. B. Iversen, X. Shi and L. Chen, Ultrahigh Thermoelectric Performance in Cu2−ySe0.5S0.5 Liquid-Like Materials, Mater. Today Phys., 2017, 1, 14 Search PubMed.
- J. Li, X. Zhang, Z. Chen, S. Lin, W. Li, J. Shen, I. T. Witting, A. Faghaninia, Y. Chen, A. Jain, L. Chen, G. J. Snyder and Y. Pei, Low-Symmetry Rhombohedral GeTe Thermoelectrics, Joule, 2018, 2(5), 976–987 CrossRef CAS.
- C. Fu, S. Bai, Y. Liu, Y. Tang, L. Chen, X. Zhao and T. Zhu, Realizing High Figure of Merit in Heavy-Band p-Type Half-Heusler Thermoelectric Materials, Nat. Commun., 2015, 6, 8144 CrossRef PubMed.
- A. Li, M. K. Brod, Y. Wang, K. Hu, P. Nan, S. Han, Z. Gao, X. Zhao, B. Ge, C. Fu, S. Anand, G. J. Snyder and T. Zhu, Opening the Bandgap of Metallic Half–Heuslers Via the Introduction of D–D Orbital Interactions, Adv. Sci., 2023, 10(23), 2302086 CrossRef CAS PubMed.
- Y. Tang, Z. M. Gibbs, L. A. Agapito, G. Li, H.-S. Kim, M. B. Nardelli, S. Curtarolo and J. Snyder, Convergence of Multi-Valley Bands as the Electronic Origin of High Thermoelectric Performance in CoSb3 Skutterudites, Nat. Mater., 2015, 14, 1223–1228 CrossRef CAS.
- H. Zhao, J. Sui, Z. Tang, Y. Lan, Q. Jie, D. Kraemer, K. McEnaney, A. Guloy, G. Chen and Z. Ren, High Thermoelectric Performance of MgAgSb-Based Materials, Nano Energy, 2014, 7(0), 97–103 CrossRef CAS.
- K. Cheng, Z. Bu, J. Tang, X. Zhang, X. Meng, W. Li and Y. Pei, Efficient Mg2Si0.3Sn0.7 Thermoelectrics Demonstrated for Recovering Heat of About 600 k, Mater. Today Phys., 2022, 28, 100887 CrossRef CAS.
- S. Ohno, K. Imasato, S. Anand, H. Tamaki, S. D. Kang, P. Gorai, H. K. Sato, E. S. Toberer, T. Kanno and G. J. Snyder, Phase Boundary Mapping to Obtain n-Type Mg3Sb2-Based Thermoelectrics, Joule, 2018, 2(1), 141–154 CrossRef CAS.
- K. Imasato, S. D. Kang, S. Ohno and G. J. Snyder, Band Engineering in Mg3Sb2 by Alloying with Mg3bi2 for Enhanced Thermoelectric Performance, Mater. Horiz., 2018, 5(1), 59–64 RSC.
- A. Li, C. Fu, X. Zhao and T. Zhu, High-Performance Mg3Sb2−xBix Thermoelectrics: Progress and Perspective, Research, 2020, 2020, 22 Search PubMed.
- W. Liu, X. Tan, K. Yin, H. Liu, X. Tang, J. Shi, Q. Zhang and C. Uher, Convergence of Conduction Bands as a Means of Enhancing Thermoelectric Performance of N-Type Mg2Si1−xSnx Solid Solutions, Phys. Rev. Lett., 2012, 108(16), 166601 CrossRef PubMed.
- Z. Han, J.-W. Li, F. Jiang, J. Xia, B.-P. Zhang, J.-F. Li and W. Liu, Room-Temperature Thermoelectric Materials: Challenges and a New Paradigm, J. Materiomics, 2022, 8(2), 427–436 CrossRef.
- J. Yu, Y. Xing, C. Hu, Z. Huang, Q. Qiu, C. Wang, K. Xia, Z. Wang, S. Bai, X. Zhao, L. Chen and T. Zhu, Half–Heusler Thermoelectric Module with High Conversion Efficiency and High Power Density, Adv. Energy Mater., 2020, 10(25), 2000888 CrossRef CAS.
-
T. Kajikawa, N. Kimura and T. Yokoyama, Thermoelectric Properties of Intermetallic Compounds: Mg3bi2 and Mg3Sb2 for Medium Temperature Range Thermoelectric Elements. 22nd International Conference on Thermoelectrics 2003, p. 305.
- H. Tamaki, H. K. Sato and T. Kanno, Isotropic Conduction Network and Defect Chemistry in Mg3+dSb2-Based Layered Zintl Compounds with High Thermoelectric Performance, Adv. Mater., 2016, 28(46), 10182–10187 CrossRef CAS PubMed.
- X. Shi, X. Wang, W. Li and Y. Pei, Advances in Thermoelectric Mg3Sb2 and Its Derivatives, Small Methods, 2018, 2, 1800022 Search PubMed.
- J. Zhang, L. Song and B. B. Iversen, Improved Thermoelectric Properties of N-Type Mg3Sb2 through Cation-Site Doping with Gd or Ho, ACS Appl. Mater. Interfaces, 2021, 13(9), 10964–10971 Search PubMed.
- T. Luo, J. J. Kuo, K. J. Griffith, K. Imasato, O. Cojocaru-Mirédin, M. Wuttig, B. Gault, Y. Yu and G. J. Snyder, Nb–Mediated Grain Growth and Grain–Boundary Engineering in Mg3Sb2−Based Thermoelectric Materials, Adv. Funct. Mater., 2021, 31(28), 2100258 Search PubMed.
- J. Zhang, L. Song and B. B. Iversen, Probing Efficient N-Type Lanthanide Dopants for Mg3Sb2 Thermoelectrics, Adv. Sci., 2020, 7(24), 2002867 Search PubMed.
- N. Chen, H. Zhu, G. Li, Z. Fan, X. Zhang, J. Yang, T. Lu, Q. Liu, X. Wu, Y. Yao, Y. Shi and H. Zhao, Improved Figure of Merit (Z) at Low Temperatures for Superior Thermoelectric Cooling in Mg3(Bi,Sb)2, Nat. Commun., 2023, 14(1), 4932 Search PubMed.
- J. Jia, Y. Zhou, X. Chen, W. Xue, H. Yu, J. Li, S. Zhi, C. Chen, J. Wang, S. Hou, X. Liu, Y. Wang, F. Cao, Y. Chen, J. Mao and Q. Zhang, Revealing the Defect-Dominated Electron Scattering in Mg3Sb2-Based Thermoelectric Materials, Research, 2022, 2022, 10 Search PubMed.
- X. Shi, X. Zhang, A. Ganose, J. Park, C. Sun, Z. Chen, S. Lin, W. Li, A. Jain and Y. Pei, Compromise between Band Structure and Phonon Scattering in Efficient n-Mg3Sb2-Bi Thermoelectrics, Mater. Today Phys., 2021, 18, 100362 CrossRef CAS.
- L. Wang, N. Sato, Y. Peng, R. Chetty, N. Kawamoto, D. H. Nguyen and T. Mori, Realizing High Thermoelectric Performance in n–Type Mg3(Sb, Bi)2−Based Materials via Synergetic Mo Addition and Sb–Bi Ratio Refining, Adv. Energy Mater., 2023, 13(35), 2301667 CrossRef CAS.
- X. Li, C. Sun, K. Yang, D. Liang, X. Ye, W. Song, W. Xu, W. Zhao and Q. Zhang, Excellent Room Temperature Thermoelectric Performance in Mg3Sb2-Based Alloys Via Multi-Functional Doping of Nb, Small, 2024, e2311478 CrossRef PubMed.
- A. Bhardwaj, A. Rajput, A. K. Shukla, J. J. Pulikkotil, A. K. Srivastava, A. Dhar, G. Gupta, S. Auluck, D. K. Misra and R. C. Budhani, Mg3Sb2-Based Zintl Compound: A Non-Toxic, Inexpensive and Abundant Thermoelectric Material for Power Generation, RSC Adv., 2013, 3(22), 8504 RSC.
- A. Bhardwaj and D. K. Misra, Enhancing Thermoelectric Properties of a p-Type Mg3Sb2-Based Zintl Phase Compound by Pb Substitution in the Anionic Framework, RSC Adv., 2014, 4(65), 34552–34560 RSC.
- A. Bhardwaj, N. S. Chauhan and D. K. Misra, Significantly Enhanced Thermoelectric Figure of Merit of p-Type Mg3Sb2-Based Zintl Phase Compounds Via Nanostructuring and Employing High Energy Mechanical Milling Coupled with Spark Plasma Sintering, J. Mater. Chem. A, 2015, 3(20), 10777–10786 RSC.
- J. Shuai, Y. Wang, H. S. Kim, Z. Liu, J. Sun, S. Chen, J. Sui and Z. Ren, Thermoelectric Properties of Na-Doped Zintl Compound: Mg3−xNaxSb2, Acta Mater., 2015, 93, 187–193 CrossRef CAS.
- S. Kim, C. Kim, Y.-K. Hong, T. Onimaru, K. Suekuni, T. Takabatake and M.-H. Jung, Thermoelectric Properties of Mn-Doped Mg–Sb Single Crystals, J. Mater. Chem. A, 2014, 2(31), 12311–12316 RSC.
- L. Song, J. Zhang and B. B. Iversen, Simultaneous Improvement of Power Factor and Thermal Conductivity Via Ag Doping in p-Type Mg3Sb2 Thermoelectric Materials, J. Mater. Chem. A, 2017, 5(10), 4932–4939 RSC.
- J. Zhang, L. Song, A. Mamakhel, M. R. V. Jørgensen and B. B. Iversen, High-Performance Low-Cost n-Type Se-Doped Mg3Sb2-Based Zintl Compounds for Thermoelectric Application, Chem. Mater., 2017, 29(12), 5371–5383 CrossRef CAS.
- J. Mao, J. Shuai, S. Song, Y. Wu, R. Dally, J. Zhou, Z. Liu, J. Sun, Q. Zhang, C. Cruz, S. Wilson, Y. Pei, D. Singh, G. Cheng, C. Chu and Z. Ren, Manipulation of Ionized Impurity Scattering for Achieving High Thermoelectric Performance in n-Type Mg3Sb2-Based Materials, Proc. Natl. Acad. Sci. U. S. A., 2017, 114, 10548 CrossRef CAS.
- J. Zhang, L. Song, S. H. Pedersen, H. Yin, L. T. Hung and B. B. Iversen, Discovery of High-Performance Low-Cost n-Type Mg3Sb2-Based Thermoelectric Materials with Multi-Valley Conduction Bands, Nat. Commun., 2017, 8, 13901 CrossRef CAS PubMed.
- Z. Ren, J. Shuai, J. Mao, Q. Zhu, S. Song, Y. Ni and S. Chen, Significantly Enhanced Thermoelectric Properties of p-Type Mg3Sb2 Via Co-Doping of Na and Zn, Acta Mater., 2018, 143, 265–271 CrossRef CAS.
- X. Shi, T. Zhao, X. Zhang, C. Sun, Z. Chen, S. Lin, W. Li, H. Gu and Y. Pei, Extraordinary n-Type Mg3SbBi Thermoelectrics Enabled by Yttrium Doping, Adv. Mater., 2019, 31, 1903387 CrossRef.
- X. Chen, H. Wu, J. Cui, Y. Xiao, Y. Zhang, J. He, Y. Chen, J. Cao, W. Cai, S. J. Pennycook, Z. Liu, L.-D. Zhao and J. Sui, Extraordinary Thermoelectric Performance in n-Type Manganese Doped Mg3Sb2 Zintl: High Band Degeneracy, Tuned Carrier Scattering Mechanism and Hierarchical Microstructure, Nano Energy, 2018, 52, 246–255 CrossRef CAS.
- J. Zhang, L. Song, K. A. Borup, M. R. V. Jørgensen and B. B. Iversen, New Insight on Tuning Electrical Transport Properties Via Chalcogen Doping in n–Type Mg3Sb2−Based Thermoelectric Materials, Adv. Energy Mater., 2018, 8(16), 1702776 CrossRef.
- K. Imasato, S. Ohno, S. D. Kang and G. J. Snyder, Improving the Thermoelectric Performance in Mg3+xSb1.5Bi0.49Te0.01 by Reducing Excess Mg, APL Mater., 2018, 6(1), 016106 CrossRef.
- S. W. Song, J. Mao, M. Bordelon, R. He, Y. M. Wang, J. Shuai, J. Y. Sun, X. B. Lei, Z. S. Ren, S. Chen, S. Wilson, K. Nielsch, Q. Y. Zhang and Z. F. Ren, Joint Effect of Magnesium and Yttrium on Enhancing Thermoelectric Properties of n-Type Zintl Mg3+dY0.02Sb1.5Bi0.5, Mater. Today Phys., 2019, 8, 25–33 CrossRef.
- Z. Han, Z. Gui, Y. B. Zhu, P. Qin, B.-P. Zhang, W. Zhang, L. Huang and W. Liu, The Electronic Transport Channel Protection and Tuning in Real Space to Boost the Thermoelectric Performance of Mg3+dSb2−yBiy near Room Temperature, Research, 2020, 2020, 12 Search PubMed.
- C. Chen, X. Li, S. Li, X. Wang, Z. Zhang, J. Sui, F. Cao, X. Liu and Q. Zhang, Enhanced Thermoelectric Performance of P-Type Mg3Sb2 by Lithium Doping and Its Tunability in an Anionic Framework, J. Mater. Sci., 2018, 53(23), 16001–16009 CrossRef CAS.
- H. Wang, J. Chen, T. Lu, K. Zhu, S. Li, J. Liu and H. Zhao, Enhanced Thermoelectric Performance in p-Type Mg3Sb2 Via Lithium Doping, Chin. Phys. B, 2018, 27(4), 047212 CrossRef.
- X. Tang, B. Zhang, X. Zhang, S. Wang, X. Lu, G. Han, G. Wang and X. Zhou, Enhancing the Thermoelectric Performance of p-Type Mg3Sb2 Via Codoping of Li and Cd, ACS Appl. Mater. Interfaces, 2020, 12(7), 8359–8365 CrossRef CAS.
- K. Imasato, M. Wood, J. J. Kuo and G. J. Snyder, Improved Stability and High Thermoelectric Performance through Cation Site Doping in n-Type La-Doped Mg3Sb1.5Bi0.5, J. Mater. Chem. A, 2018, 6(41), 19941–19946 RSC.
- F. Zhang, C. Chen, S. Li, L. Yin, B. Yu, J. Sui, F. Cao, X. Liu, Z. Ren and Q. Zhang, Enhanced Thermoelectric Performance in n–Type Mg3.2Sb1.5Bi0.5 by La or Ce Doping into Mg, Adv. Electron. Mater., 2020, 6(3), 1901391 CAS.
- J. Zhang, L. Song and B. B. Iversen, Rapid One–Step Synthesis and Compaction of High–Performance n–Type Mg3Sb2 Thermoelectrics, Angew. Chem., Int. Ed., 2020, 59(11), 4278–4282 CAS.
- J. Li, S. Zhang, K. Han, B. Sun and L. Cao, Large Improvement in Thermoelectric Performance of Pressure-Tuned Mg3Sb2, RSC Adv., 2022, 12(2), 1149–1156 CAS.
- K. Liu, C. Chen, X. Li, J. Jia, C. Xia, J. Mao, Q. Huang, J. Sui, F. Cao, X. Liu, Y. Chen and Q. Zhang, Tuning the Carrier Scattering Mechanism by Rare-Earth Element Doping for High Average zT in Mg3Sb2-Based Compounds, ACS Appl. Mater. Interfaces, 2022, 14(5), 7022–7029 CrossRef CAS PubMed.
- H. Shang, Q. Zou, L. Zhang, Z. Liang, S. Song, B. Hong, H. Gu, Z. Ren and F. Ding, Improving Thermal Stability and Revealing Physical Mechanism in N-Type Mg3Sb2-Bi for Practical Applications, Nano Energy, 2023, 109, 108270 CAS.
- J. Hu, J. Zhu, X. Dong, M. Guo, Y. Sun, W. Shi, Y. Zhu, H. Wu, F. Guo, Y. X. Zhang, Z. H. Ge, Q. Zhang, Z. Liu, W. Cai and J. Sui, Breaking the Minimum Limit of Thermal Conductivity of Mg3Sb2 Thermoelectric Mediated by Chemical Alloying Induced Lattice Instability, Small, 2023, 19(33), e2301382 Search PubMed.
- L. Yu, X. L. Shi, Y. Mao, W. D. Liu, Z. Ji, S. Wei, Z. Zhang, W. Song, S. Zheng and Z. G. Chen, Simultaneously Boosting Thermoelectric and Mechanical Properties of n-Type Mg3Sb1.5Bi0.5-Based Zintls through Energy-Band and Defect Engineering, ACS Nano, 2024, 18(2), 1678–1689 CrossRef CAS PubMed.
- J. S. Liang, X. L. Shi, Y. Peng, W. D. Liu, H. Q. Yang, C. Y. Liu, J. L. Chen, Q. Zhou, L. Miao and Z. G. Chen, Synergistic Effect of Band and Nanostructure Engineering on the Boosted Thermoelectric Performance of n–Type Mg3+d(Sb, Bi)2 Zintls, Adv. Energy Mater., 2022, 12(26), 2201086 CrossRef CAS.
- M. Jin, S. Lin, W. Li, X. Zhang and Y. Pei, Nearly Isotropic Transport Properties in Anisotropically Structured n-Type Single-Crystalline Mg3Sb2, Mater. Today Phys., 2021, 21, 100508 CrossRef CAS.
- A. Li, C. Hu, B. He, M. Yao, C. Fu, Y. Wang, X. Zhao, C. Felser and T. Zhu, Demonstration of Valley Anisotropy Utilized to Enhance the Thermoelectric Power Factor, Nat. Commun., 2021, 12(1), 5408 CrossRef CAS.
- J. Mao, Y. Wu, S. Song, J. Shuai, Z. Liu, Y. Pei and Z. Ren, Anomalous Electrical Conductivity of n-Type Te-Doped Mg3.2Sb1.5Bi0.5, Mater. Today Phys., 2017, 3, 1–6 CrossRef.
- R. Shu, Y. Zhou, Q. Wang, Z. Han, Y. Zhu, Y. Liu, Y. Chen, M. Gu, W. Xu, Y. Wang, W. Zhang, L. Huang and W. Liu, Mg3+dSbxBi2−x Family: A Promising Substitute for the State-of-the-Art n-Type Thermoelectric Materials near Room Temperature, Adv. Funct. Mater., 2019, 29, 1807235 CrossRef.
- J. W. Li, W. Liu, W. Xu, H. L. Zhuang, Z. Han, F. Jiang, P. Zhang, H. Hu, H. Gao, Y. Jiang, B. Cai, J. Pei, B. Su, Q. Li, K. Hayashi, H. Li, Y. Miyazaki, X. Cao, Q. Zheng and J. F. Li, Bi-Deficiency Leading to High-Performance in Mg3 (Sb,Bi)2-Based Thermoelectric Materials, Adv. Mater., 2023, 35(23), e2209119 CrossRef PubMed.
- X. Sun, X. Li, J. Yang, J. Xi, R. Nelson, C. Ertural, R. Dronskowski, W. Liu, G. J. Snyder, D. J. Singh and W. Zhang, Achieving Band Convergence by Tuning the Bonding Ionicity in n-Type Mg3Sb2, J. Comput. Chem., 2019, 40(18), 1693–1700 CrossRef CAS PubMed.
- J. Hu, J. Zhu, F. Guo, H. Qin, Y. Liu, Q. Zhang, Z. Liu, W. Cai and J. Sui, Electronic Orbital Alignment and Hierarchical Phonon Scattering Enabling High Thermoelectric Performance p-Type Mg3Sb2 Zintl Compounds, Research, 2022, 2022, 12 CrossRef.
- J. Li, S. Zhang, F. Jia, S. Zheng, X. Shi, D. Jiang, S. Wang, G. Lu, L. Wu and Z.-G. Chen, Point Defect Engineering and Machinability in n-Type Mg3Sb2-Based Materials, Mater. Today Phys., 2020, 15, 100269 CrossRef.
- J. Li, F. Jia, S. Zhang, S. Zheng, B. Wang, L. Chen, G. Lu and L. Wu, The Manipulation of Substitutional Defects for Realizing High Thermoelectric Performance in Mg3Sb2-Based Zintl Compounds, J. Mater. Chem. A, 2019, 7(33), 19316–19323 RSC.
- J. Shuai, J. Mao, S. Song, Q. Zhu, J. Sun, Y. Wang, R. He, J. Zhou, G. Chen, D. J. Singh and Z. Ren, Tuning the Carrier Scattering Mechanism to Effectively Improve the Thermoelectric Properties, Energy Environ. Sci., 2017, 10(3), 799–807 Search PubMed.
- K. Imasato, C. Fu, Y. Pan, M. Wood, J. J. Kuo, C. Felser and G. J. Snyder, Metallic n-Type Mg3Sb2 Single Crystals Demonstrate the Absence of Ionized Impurity Scattering and Enhanced Thermoelectric Performance, Adv. Mater., 2020, 32(16), e1908218 Search PubMed.
- F. Jiang, X. Wu, Y. Zhu, C. Xia, Z. Han, H. Yu, C. Chen, T. Feng, J. Mao, Y. Chen and W. Liu, Boosting Room-Temperature Thermoelectric Performance of Mg3Sb1.5Bi0.5 Material through Breaking the Contradiction between Carrier Concentration and Carrier Mobility, Acta Mater., 2024, 265, 119636 Search PubMed.
- W. Zhu, P. Zheng, Y. Shao, W. Fang, H. Wu and J. Si, Enhanced Average Thermoelectric Properties of n-Type Mg3Sb2 Based Materials by Mixed-Valence Ni Doping, J. Alloys Compd., 2022, 924, 166598 CrossRef CAS.
- Z. Liang, C. Xu, H. Shang, M. Ning, T. Tong, S. Song, W. Ren, X. Shi, X. Liu, F. Ding, J. Bao, D. Wang and Z. Ren, Near–Room–Temperature Thermoelectric Performance Enhancement Via Phonon Spectra Mismatch in Mg3(Sb, Bi)2−Based Material by Incorporating Multi–Walled Carbon Nanotubes, Adv. Energy Mater., 2023, 13(25), 2301107 Search PubMed.
- X. Shi, C. Sun, X. Zhang, Z. Chen, S. Lin, W. Li and Y. Pei, Efficient Sc-Doped Mg3.05−xScxSbBi Thermoelectrics near Room Temperature, Chem. Mater., 2019, 31(21), 8987–8994 Search PubMed.
- Y. Pan, M. Yao, X. Hong, Y. Zhu, F. Fan, K. Imasato, Y. He, C. Hess, J. Fink, J. Yang, B. Büchner, C. Fu, G. J. Snyder and C. Felser, Mg3(Bi,Sb)2 Single Crystals Towards High Thermoelectric Performance, Energy Environ. Sci., 2020, 13(6), 1717–1724 Search PubMed.
- Z. Liu, N. Sato, W. Gao, K. Yubuta, N. Kawamoto, M. Mitome, K. Kurashima, Y. Owada, K. Nagase, C.-H. Lee, J. Yi, K. Tsuchiya and T. Mori, Demonstration of Ultrahigh Thermoelectric Efficiency of ∼7.3% in Mg3Sb2/MgAgSb Module for Low-Temperature Energy Harvesting, Joule, 2021, 5(5), 1196–1208 Search PubMed.
- F. Jiang, T. Feng, Y. Zhu, Z. Han, R. Shu, C. Chen, Y. Zhang, C. Xia, X. Wu, H. Yu, C. Liu, Y. Chen and W. Liu, Extraordinary Thermoelectric Performance, Thermal Stability and Mechanical Properties of n-Type Mg3Sb1.5Bi0.5 through Multi-Dopants at Interstitial Site, Mater. Today Phys., 2022, 27, 100835 Search PubMed.
- Y. Geng, Z. Li, Z. Lin, Y. Liu, Q. Lai, X. Wu, L. Hu, F. Liu, Y. Yu and C. Zhang, Inhibiting Mg Diffusion and Evaporation by Forming Mg-Rich Reservoir at Grain Boundaries Improves the Thermal Stability of n-Type Mg3Sb2 Thermoelectrics, Small, 2024, 20(2), e2305670 Search PubMed.
- Z. Liang, M. Jian, C. Xu, B.-H. Lei, X. Shi, H. Shang, S. Song, W. Ren, F. Ding, D. J. Singh, Z. Feng and Z. Ren, Intrinsic Thermal Stability Enhancement in n-Type Mg3Sb2 Thermoelectrics toward Practical Applications, Acta Mater., 2023, 247, 118752 Search PubMed.
- H. Shang, Z. Liang, C. Xu, S. Song, D. Huang, H. Gu, J. Mao, Z. Ren and F. Ding, N-Type Mg3Sb2-Bi with Improved Thermal Stability for Thermoelectric Power Generation, Acta Mater., 2020, 201, 572–679 Search PubMed.
- A. Li, P. Nan, Y. Wang, Z. Gao, S. Zhang, Z. Han, X. Zhao, B. Ge, C. Fu and T. Zhu, Chemical Stability and Degradation Mechanism of Mg3Sb2−xBix Thermoelectrics Towards Room-Temperature Applications, Acta Mater., 2022, 239, 118301 CrossRef CAS.
- X. Wu, X. Ma, H. Yao, K. Liang, P. Zhao, S. Hou, L. Yin, H. Yang, J. Sui, X. Lin, F. Cao, Q. Zhang and J. Mao, Revealing the Chemical Instability of Mg3Sb2−xBix-Based Thermoelectric Materials, ACS Appl. Mater. Interfaces, 2023, 15(43), 50216–50224 CrossRef CAS PubMed.
- X. Wu, Z. Han, Y. Zhu, B. Deng, K. Zhu, C. Liu, F. Jiang and W. Liu, A General Design Strategy for Thermoelectric Interface Materials in n-Type Mg3Sb1.5Bi0.5 Single Leg Used in Tegs, Acta Mater., 2022, 226, 117616 CrossRef CAS.
- L. Yin, X. Li, X. Bao, J. Cheng, C. Chen, Z. Zhang, X. Liu, F. Cao, J. Mao and Q. Zhang, Calphad Accelerated Design of Advanced Full-Zintl Thermoelectric Device, Nat. Commun., 2024, 15(1), 1468 CrossRef CAS.
- Z. Bu, X. Zhang, Y. Hu, Z. Chen, S. Lin, W. Li and Y. Pei, An over 10% Module Efficiency Obtained Using Non-Bi2Te3 Thermoelectric Materials for Recovering Heat of <600 K, Energy Environ. Sci., 2021, 14(12), 6506–6513 RSC.
- X. Wu, Y. Lin, Z. Han, H. Li, C. Liu, Y. Wang, P. Zhang, K. Zhu, F. Jiang, J. Huang, H. Fan, F. Cheng, B. Ge and W. Liu, Interface and Surface Engineering Realized High Efficiency of 13% and Improved Thermal Stability in Mg3Sb1.5Bi0.5−Based Thermoelectric Generation Devices, Adv. Energy Mater., 2022, 12(48), 2203039 CrossRef CAS.
- Y. Wang, J. Chen, Y. Jiang, M. Ferhat, S. Ohno, Z. A. Munir, W. Fan and S. Chen, Suppression of Interfacial Diffusion in Mg3Sb2 Thermoelectric Materials through an Mg4.3Sb3Ni/Mg3.2Sb2Y0.05/Mg4.3Sb3Ni-Graded Structure, ACS Appl. Mater. Interfaces, 2022, 14(29), 33419–33428 CrossRef CAS.
- J. Chu, J. Huang, R. Liu, J. Liao, X. Xia, Q. Zhang, C. Wang, M. Gu, S. Bai, X. Shi and L. Chen, Electrode Interface Optimization Advances Conversion Efficiency and Stability of Thermoelectric Devices, Nat. Commun., 2020, 11(1), 2723 CrossRef CAS.
- J. Yang, G. Li, H. Zhu, N. Chen, T. Lu, J. Gao, L. Guo, J. Xiang, P. Sun, Y. Yao, R. Yang and H. Zhao, Next-Generation Thermoelectric Cooling Modules Based on High-Performance Mg3(Bi,Sb)2 Material, Joule, 2022, 6(1), 193–204 CrossRef CAS.
- Q. Zhu and Z. Ren, A Double Four-Point Probe Method for Reliable Measurement of Energy Conversion Efficiency of Thermoelectric Materials, Energy, 2020, 191, 116599 CrossRef CAS.
- L. Yin, C. Chen, F. Zhang, X. Li, F. Bai, Z. Zhang, X. Wang, J. Mao, F. Cao, X. Chen, J. Sui, X. Liu and Q. Zhang, Reliable n-Type Mg3.2Sb1.5Bi0.49Te0.01/304 Stainless Steel Junction for Thermoelectric Applications, Acta Mater., 2020, 198, 25–34 CrossRef CAS.
- C. Xu, Z. Liang, H. Shang, D. Wang, H. Wang, F. Ding, J. Mao and Z. Ren, Scalable Synthesis of n-Type Mg3Sb2−xBix for Thermoelectric Applications, Mater. Today Phys., 2021, 17, 100336 CrossRef CAS.
- M. Jiang, Y. Fu, Q. Zhang, Z. Hu, A. Huang, S. Wang, L. Wang and W. Jiang, High-Efficiency and Reliable Same-Parent Thermoelectric Modules Using Mg3Sb2-Based Compounds, Natl. Sci. Rev., 2023, 10(6), nwad095 CrossRef CAS PubMed.
- Z. Liang, C. Xu, S. Song, X. Shi, W. Ren and Z. Ren, Enhanced Thermoelectric Performance of p–Type Mg3sb2 for Reliable and Low–Cost All–Mg3Sb2−Based Thermoelectric Low–Grade Heat Recovery, Adv. Funct. Mater., 2022, 33(7), 2210016 CrossRef.
- P. Ying, R. He, J. Mao, Q. Zhang, H. Reith, J. Sui, Z. Ren, K. Nielsch and G. Schierning, Towards Tellurium-Free Thermoelectric Modules for Power Generation from Low-Grade Heat, Nat. Commun., 2021, 12(1), 1121 CrossRef CAS PubMed.
- X. Li, C. Chen, L. Yin, X. Wang, J. Mao, F. Cao and Q. Zhang, Realizing an Excellent Conversion Efficiency of 14.5% in the Mg3Sb2/GeTe-Based Thermoelectric Module for Waste Heat Recovery, Energy Environ. Sci., 2023, 16(12), 6147–6154 RSC.
- Y. Liu, Y. Geng, Y. Dou, X. Wu, L. Hu, F. Liu, W. Ao and C. Zhang, Mg Compensating Design in the Melting-Sintering Method for High-Performance Mg3(Bi, Sb)2thermoelectric Devices, Small, 2023, 19(44), e2303840 CrossRef PubMed.
- J. Mao, H. Zhu, Z. Ding, Z. Liu, G. A. Gamage, G. Chen and Z. Ren, High Thermoelectric Cooling Performance of n-Type Mg3Bi2-Based Materials, Science, 2019, 365, 495 Search PubMed.
- P. Ying, L. Wilkens, H. Reith, N. P. Rodriguez, X. Hong, Q. Lu, C. Hess, K. Nielsch and R. He, A Robust Thermoelectric Module Based on MgAgSb/Mg3(Sb,Bi)2 with a Conversion Efficiency of 8.5% and a Maximum Cooling of 72K, Energy Environ. Sci., 2022, 15(6), 2557–2566 Search PubMed.
- Z. Liu, W. Gao, H. Oshima, K. Nagase, C. H. Lee and T. Mori, Maximizing the Performance of n-Type Mg3Bi2 Based Materials for Room-Temperature Power Generation and Thermoelectric Cooling, Nat. Commun., 2022, 13(1), 1120 Search PubMed.
- A. Li, Y. Wang, Y. Li, X. Yang, P. Nan, K. Liu, B. Ge, C. Fu and T. Zhu, High Performance Magnesium-Based Plastic Semiconductors for Flexible Thermoelectrics, Nat. Commun., 2024, 15(1), 5108 Search PubMed.
- T.-R. Wei, M. Jin, Y. Wang, H. Chen, Z. Gao, K. Zhao, P. Qiu, Z. Shan, J. Jiang, R. Li, L. Chen, J. He and X. Shi, Exceptional Plasticity in the Bulk Single-Crystalline van der Waals Semiconductor Inse, Science, 2020, 369, 542 Search PubMed.
- X. Shi, H. Chen, F. Hao, R. Liu, T. Wang, P. Qiu, U. Burkhardt, Y. Grin and L. Chen, Room-Temperature Ductile Inorganic Semiconductor, Nat. Mater., 2018, 17(5), 421–426 CrossRef CAS PubMed.
- X. D. Wang, J. Tan, J. Ouyang, H. M. Zhang, J. J. Wang, Y. Wang, V. L. Deringer, J. Zhou, W. Zhang and E. Ma, Designing Inorganic Semiconductors with Cold–Rolling Processability, Adv. Sci., 2022, 9(30), 2203776 CrossRef CAS.
- B. Ge, C. Li, W. Lu, H. Ye, R. Li, W. He, Z. Wei, Z. Shi, D. Kim, C. Zhou, M. Zhu, M. Wuttig and Y. Yu, Dynamic Phase Transition Leading to Extraordinary Plastic Deformability of Thermoelectric SnSe2 Single Crystal, Adv. Energy Mater., 2023, 13(27), 2300965 Search PubMed.
- P. Zhao, W. Xue, Y. Zhang, S. Zhi, X. Ma, J. Qiu, T. Zhang, S. Ye, H. Mu, J. Cheng, X. Wang, S. Hou, L. Zhao, G. Xie, F. Cao, X. Liu, J. Mao, Y. Fu, Y. Wang and Q. Zhang, Plasticity in Single-Crystalline Mg3bi2 Thermoelectric Material, Nature, 2024, 631, 777 Search PubMed.
Footnote |
† These two authors contributed equally to this work and are listed alphabetically. |
|
This journal is © The Royal Society of Chemistry 2025 |
Click here to see how this site uses Cookies. View our privacy policy here.