DOI:
10.1039/D4QI01910E
(Research Article)
Inorg. Chem. Front., 2025,
12, 355-368
Tailoring electronic structure to enhance the ammonium-ion storage properties of VO2 by molybdenum doping toward highly efficient aqueous ammonium-ion batteries†
Received
29th July 2024
, Accepted 18th November 2024
First published on 19th November 2024
Abstract
Recently, research on ammonium-ion storage has gained widespread interest, and it is still a major problem and a popular research area to produce high-performance electrode materials for aqueous ammonium ion batteries (AAIBs). Herein, the electronic structure of tunnel-like vanadium dioxide (VO2) is tailored by molybdenum doping (denoted as VO2-Mo) to enhance ammonium-ion storage properties toward highly efficient AAIBs. VO2-Mo with a unique nanobelt structure is designed and synthesized by adjusting the content of Mo via a facile hydrothermal method. Density functional theory (DFT) simulations and experimental data both demonstrate that molybdenum atoms in the VO2 structure can improve mass transfer, speed up ion transport, and accelerate kinetics, showing boosted NH4+-storage properties. With 2% Mo doping, at 0.1 A g−1, VO2-Mo exhibits a specific discharge capacity of around 370 mA h g−1, surpassing VO2 (232 mA h g−1) and the vanadium oxide-based materials that have been reported for NH4+-storage. After approximately 6000 successive charging and discharging cycles at 2 A g−1, it essentially maintains the specific capacity of 140 mA h g−1. Using VO2-Mo, polyaniline (PANI) and 1 M (NH4)2SO4 as the anode, cathode, and electrolyte, respectively, a VO2-Mo//PANI full battery was further built, and at 0.2 A g−1, it reached a specific discharge capacity of up to 232 mA h g−1, surpassing the performances of the most state-of-the-art AAIBs. At 89 W kg−1, the VO2-Mo//PANI battery can achieve an energy density (E) up to 133 W h kg−1. This study provides new ideas for tailoring electrode materials with enhanced NH4+-storage for AAIBs.
1. Introduction
In the face of the growing energy crisis and climate change, there is an urgent need to reduce the burning of fossil fuels and develop sustainable energy sources. One of these is rechargeable batteries, which are considered to be a clean and sustainable solution.1–5 The high energy density of conventional lithium-ion batteries (LIBs) has led to their widespread use but the safety issues of LIBs are also prominent, especially the thermal runaway problem triggered by their flammable and toxic organic electrolytes.6–8 As a result, new batteries built on aqueous electrolytes have drawn a lot of interest recently because of their excellent security, affordability, and environmental friendliness in addition to their numerous application possibilities.9–16 Aqueous batteries usually use metal ions as charge carriers, such as Li+,17,18 K+,19 Na+,20 Mg2+,21 and Zn2+.22–24 These metal-ion carrier batteries have many advantages, such as abundant resource reserves and superior theoretical capacity. However, the excessive focus on metal ions has led to the neglect of non-metal ion carriers, which has resulted in insufficient research on novel non-metal ion water-based batteries and supercapacitors.25 In fact, non-metal ions tend to have some unique advantages compared to metal ions, such as higher abundance, lower hydration radius and lighter molar mass.26 Of these, NH4+ has garnered significant interest because of its quick kinetics, lower molar mass (18 g mol−1), and smaller hydration radius (3.31 Å).27–30 What's more, the unique H-bond energy storage mechanism and tetrahedral geometry confer the topological electrochemical chemistry of NH4+. Despite these advantages, the development of water-based NH4+ rechargeable devices is still at a basic stage, and exploring suitable electrode materials is still a huge challenge.7
Previous studies on aqueous ammonium ion batteries (AAIBs) are mainly focused on cathode materials. Since Toshima's pioneering exploration of the feasibility of embedding NH4+ in Prussian blue analogues (PBAs),31 a series of major breakthroughs have been made in the field of NH4+ electrolyte energy storage. Cui et al. later conducted groundbreaking work on the use of PBAs for NH4+ storage.32 In addition to PBAs, Dong et al. developed α-MnO2 with a tunnel-like structure applied for the electrochemical storage of NH4+, which had excellent electrochemical properties.33 In addition to the above materials, other electrode materials, such as Berlin Green,34 Ti3C2-Mxene,28 MnOx,12 and vanadium-based materials,27,35–37 can also be used for efficient NH4+ storage. Among these, materials based on vanadium have garnered a lot of interest because of their distinctive shape, numerous changeable oxidation states, and vast resource reserves.38–42 However, their poor electrical conductivity, slow ion diffusion kinetics and unavoidable dissolution in aqueous solutions are drawbacks that severely limit their NH4+ storage performance.26 For this reason, some effective strategies can be adopted to circumvent the above problems, and the common methods include cladding, interpolation, doping and defect engineering. For example, Zhang et al. developed polypyrrole intercalated VOH for efficient aqueous ammonium ion storage.43 Chen et al. reported the use of interlayer engineering to promote aqueous ammonium ion storage by synergizing K+ and polyaniline (PANI) during vanadium oxide hydration.30 Liu et al. explored a defect engineering strategy to promote ammonium vanadate electrode materials for high-performance aqueous ammonium ion supercapacitors.41 These modification strategies have made great contributions to aqueous ammonium ion energy storage. Notably, this is a particularly interesting approach in terms of defect engineering, which can change the nature of the electronic structure without significantly affecting the lattice, thus improving the electrochemical properties of the sample. In detail, oxygen defects can initiate an unbalanced distribution of electric charges, attracting more electric charges to be incorporated into the off-domain electron cloud, thus stimulating electric charge transfer and increasing the electrical conductivity.44 Meanwhile, oxygen defects significantly facilitate the creation of additional active sites and regulated migration paths for carrier ions (NH4+), which effectively accelerates the electrochemical reaction kinetics, reduces the polarization effect, and ultimately achieves reversible storage of ions.39,41,45
Among the vanadium oxides, vanadium dioxide (VO2) exhibits significant promise in a range of aqueous metal ion batteries due to its tunnel-like structure (Fig. 1b),46,47 and the research on VO2 for AAIBs has also received attention.39,40,48 Gong et al. developed electrode materials with power densities up to 4540 W kg−1 for aqueous ammonium ion batteries by modifying the oxygen defect content in VO2·xH2O nanoarrays.40 As an anode material for AAIBs, Dong et al.39 produced faulty VO2, which showed a moderate transport channel with 200 mA h g−1 (0.1 A g−1). The energy density of the entire battery was 96 W h kg−1. Tan et al. constructed a VO2@C composite and it showed good NH4+ storage characteristics.48 These works demonstrate that the electrochemical properties are boosted by the composite and defects, whereas, they may also be improved by other methods, such as doping, which can tailor the electronic structure of VO2.46 Therefore, herein, we focus on the NH4+-storage of VO2 for AAIBs enhanced by the doping strategy. Although recent works have been reported for Mo doped into VO2 for NH4+ storage, the capacity achieved by Wang et al.49 is not high enough and the reason why this method can boost the electrochemical properties is not clearly demonstrated.50 Besides, the latter work focused on its electrochemical properties for supercapacitors, not for AAIBs.
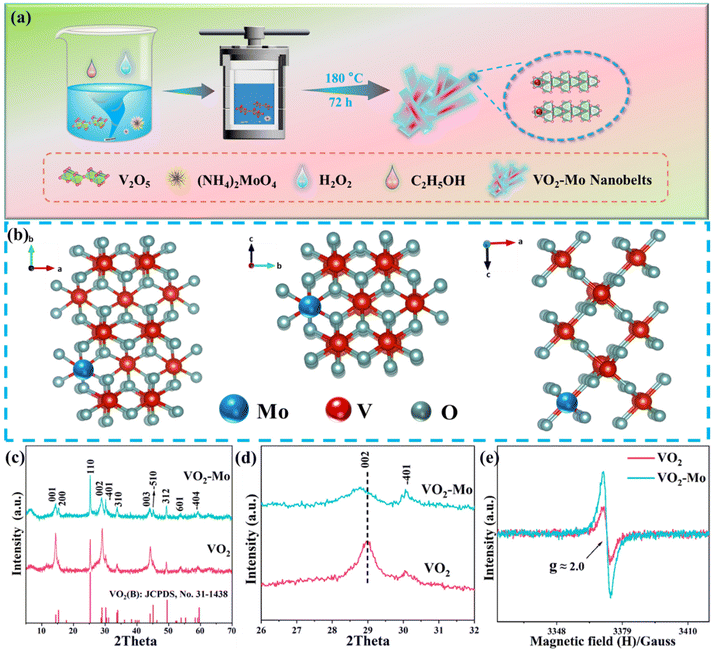 |
| Fig. 1 Characterization of VO2-Mo: (a) sample synthesis process diagram; (b) the VO2-Mo crystal structure from several angles; (c) XRD patterns of VO2-Mo and VO2; (d) the small 2θ range; and (e) EPR spectra of VO2 and VO2-Mo. | |
Based on the above statements, a simple process is developed in this study to produce appropriate oxygen defects based on a mild reaction environment by introducing an appropriate amount of Mo into VO2 (denoted as VO2-Mo). When Mo is doped successfully, VO2's lattice spacing increases, which is advantageous for NH4+ storage that is effective. The guest Mo atom in the VO2 crystal structure can boost mass transfer, accelerate ion transport, and accelerate kinetics, as demonstrated by both experimental results and DFT simulations. This results in increased NH4+-storage properties of the VO2-Mo composite. It is proved that the Mo-doped sample VO2-Mo has superior electrochemical properties and cycling stability compared to VO2. With an excellent specific capacity of 370 mA h g−1 under 0.1 A g−1, the former still maintains a high capacity retention rate of 85% even after 6000 cycles. Excellent NH4+ storage performance is also demonstrated by the full-cell VO2-Mo//PANI, which is composed of polyaniline (PANI) as the cathode material and VO2-Mo as the anode material. This strategy may open more paths in advanced NH4+ energy storage materials, driving the future boom of aqueous nonmetallic ion storage systems.
2. Results and discussion
2.1. Composition and structure of VO2-Mo
As described in Fig. 1a, molybdenum doped vanadium dioxide (denoted as VO2-Mo) with 2% Mo atom percentage was synthesized directly by a simple hydrothermal method using V2O5 and (NH4)2MoO4 as the raw sources, as well as anhydrous ethanol as the reductant. H2O2, here, plays a vital role in converting the solid V2O5 to the soluble coordination compound obeying the following chemical equation:51 V2O5 + 4H2O2 → 2[VO(O2)2]− + 3H2O + 2H+. This is very important for doping because the soluble 2[VO(O2)2]− and ammonium molybdate can form a homogeneous solution and they can coprecipitate to form VO2-Mo under hydrothermal conditions. VO2-Mo has a typical tunneling structure, displayed in Fig. 1b, which is good for rapid ionic migration.52 The tunnel-like VO2 has been demonstrated to be a promising material for Li-ion and Zn-ion storage,53 as well as ammonium-ion storage.39 The performance of VO2 is enhanced because the guest atom optimized its electronic structure46 and this property may also be suitable for ammonium-ion storage. Fig. 1c displays the X-ray diffraction (XRD) patterns, and all peaks are able to match perfectly with the standard card of VO2 (JCPDS, No. 31-1438). Comparing VO2 with VO2-Mo (Fig. 1d), the (002) crystal plane (2θ about 29°) is significantly shifted to a lower angle by the introduction of Mo. The fact that the massive Mo atom is effectively doped into the crystalline structure of VO2 in place of the V position is demonstrated by the increased crystal plane spacing. The tuned structure of VO2 can favor the insertion and detachment of ammonium ions like other vanadium oxides.54 It is worth noting that the introduction of Mo modulates the structure of VO2 under hydrothermal conditions, which may make the exposure of some of its crystal facets not exactly the same. For example, the diffraction peaks at 28.8° and 30.1° correspond to the (002) and (−401) crystal facets, respectively, where the (−401) crystal facet is relatively more exposed for VO2-Mo. The introduction of Mo does not create any impurity, but the degree of VO2-Mo crystallinity is decreased because the XRD peaks become weak (Fig. 1c and d), which suggest that there are more oxygen defects in VO2-Mo. In order to verify the above conjecture, we performed electron paramagnetic resonance (EPR) measurements on VO2-Mo and VO2, as seen in Fig. 1e, where VO2-Mo exhibits two substantially symmetric peaks at g = 2.0, which is attributed to anionic vacancy trapping of electrons. These prove that the introduction of Mo can induce more oxygen defects, which will improve the charge storage of VO2.40
Using N2 adsorption/desorption isotherms, the porous structural information of VO2 and VO2-Mo is examined and is displayed in Fig. 2a. The isotherms are basically assigned to III and IV with an H3 hysteresis loop as defined by IUPAC. The specific surface area (SBET) of VO2-Mo, measured by the Brunauer–Emmett–Teller (BET) method, is around 43 m2 g−1, greater than the value of VO2 (24 m2 g−1). The pore distributions of VO2 and VO2-Mo (Barrett–Joyner–Halenda (BJH) method) are depicted in Fig. S1 (ESI†). It can be seen that the introduction of Mo in VO2 can induce some pores. The total pore volumes of VO2 and VO2-Mo are found to be 0.283 cm3 g−1 and 0.394 cm3 g−1, respectively. The results from the N2 adsorption/desorption isotherms furthermore confirm that the Mo-doping can tune the structure of VO2. Fourier transform infrared spectroscopy (FTIR) was used on the substances both before and after doping to investigate the FTIR spectra of VO2 and VO2-Mo, displayed in Fig. S2 (ESI†), in order to further confirm the chemical bonding composition of the products. The V
O and V–O–V bonds are responsible for the vibrational peaks at roughly 1000 cm−1 and 500 cm−1,40 respectively. Compared with VO2, the vibrational peaks in VO2-Mo are slightly red-shifted, indicating the chemical environment change induced by Mo-doping. It is noteworthy to observe that a wavenumber of about 815 cm−1 is indexed to the characteristic Mo–O vibrational peak, which strongly proves the successful doping of Mo. Fig. S3 (ESI†) depicts the Raman spectra of VO2 and VO2-Mo. The stretching vibration of (V2O2)n, the bending vibration of V
O, the bending vibration of V–O3–V, the V2–O stretching vibration, and the V–O stretching vibration are represented by the Raman peaks that are present in both of them at around 140 cm−1, 280 cm−1, 407 cm−1, 695 cm−1, and 992 cm−1.55 As seen in Fig. S4 (ESI†) and Fig. 2b and c, XPS spectra are used to further explore and offer information on the chemical composition and oxidation states of VO2 and VO2-Mo. The full XPS spectra (Fig. S4†) clearly show that the Mo 3p and Mo 3d peaks appear in VO2-Mo, and the remaining peaks align with every one of the VO2 peaks. The atomic percentage of Mo/V is about 1.9%, which is in line with the used raw materials. The V 2p XPS spectra of VO2 and VO2-Mo are compared and shown in Fig. 2b, where V 2p1/2 and V 2p3/2 have binding energies of about 524.2 eV and 516.8 eV for VO2 and 523.8 eV and 516.5 eV for VO2-Mo, respectively. What's more, the difference of V 2p1/2 and V 2p3/2 in these two samples is 7.3 eV. The above results prove that the V in the above samples is in the +4 oxidation state,40 and the doping of Mo leads to a slight shift of V 2p binding energies in VO2-Mo. The Mo 3d XPS spectra of VO2-Mo in Fig. 2c are divided into Mo 3d3/2 (235.8 eV) and Mo 3d5/2 (232.6 eV). These values combined with the peak difference of 3.2 eV prove that Mo is in the +4 oxidation state in VO2-Mo.56 The XPS results further prove the successful doping of Mo. Scanning electron microscopy (SEM) images show that both VO2 (Fig. S5a, ESI†) and VO2-Mo (Fig. 2d and e) have the structures of nanobelts. The SEM elemental mapping images provide additional confirmation of the elemental composition of VO2 and VO2-Mo, as shown in Fig. S6 (ESI†) and Fig. 2f, respectively. They reveal the presence of elements V, O and Mo in VO2-Mo (Fig. 2f), while only elements V and O are present in VO2 (Fig. S6, ESI†). The elemental distributions are very homogeneous in both, which confirms the successful doping of Mo and the absence of impurities introduced during the doping process. The TEM pictures provide additional confirmation of the VO2 and VO2-Mo nanobelt shape (Fig. S5b, ESI;†Fig. 2g and h). A high-resolution TEM (HRTEM) picture of VO2-Mo is displayed in Fig. 2i. It is evident that good crystallization is present, and the clear crystalline lattice spacing is around 0.357 nm, in accordance with the VO2 (110) plane, suggestive of the successful synthesis of VO2. In summary, the above characterization results show that the introduction of Mo atoms modulates the crystal facet index of tunneling VO2, enlarges its spacing, and generates appropriate oxygen defects, while it also increases the specific surface area of VO2 and enriches its microscopic pore structure, which are conducive to the enhancement of the ion transport kinetics of the carriers (NH4+) during the electrochemical process and favors efficient ammonium-ion storage, and ultimately to the enhancement of the material's electrochemical performance.40
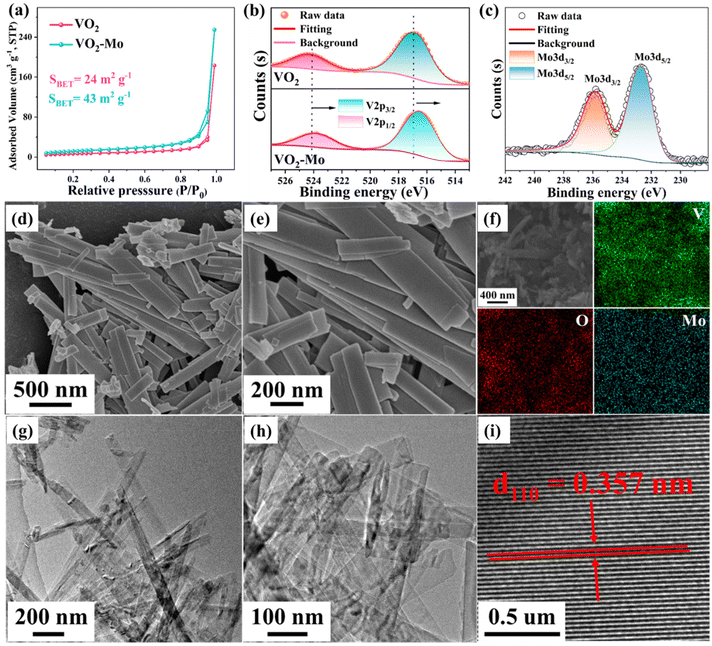 |
| Fig. 2 Structure and morphology of VO2 and VO2-Mo: (a) N2 adsorption–desorption isotherms; (b) V 2p XPS spectra; (c) Mo 3d XPS spectra of VO2-Mo; (d and e) SEM images of VO2-Mo; (f) SEM elemental mapping images of VO2-Mo; (g and h) TEM images and (i) HRTEM image of VO2-Mo. | |
2.2. The VO2-Mo electrode's NH4+ storage properties and mechanism
It is widely believed that the generation of appropriate oxygen vacancies in the lattice is beneficial to increase the active sites for ion storage, accelerate ion diffusion, increase the material's electrical conductivity, and ultimately enhance the comprehensive electrochemical performances of materials for NH4+ storage.39–41 In this work, a standard three-electrode system was assembled utilizing 1 mol L−1 (NH4)2SO4 as the electrolyte in order to investigate the electrochemical characteristics of VO2 and VO2-Mo for the NH4+ storage. As shown in Fig. S7 (ESI†), the three electrodes in the system were a working electrode (VO2-Mo or VO2), a reference electrode (Ag/AgCl), and a counter electrode (carbon rod). To investigate how the amount of Mo doping affects VO2's electrochemical performance, the Mo contents were adjusted to 0%, 1%, 2% and 4% (details in the ESI†). The XRD patterns in Fig. S8 (ESI†) confirm their successful preparation. The galvanostatic charge–discharge (GCD) curves of these samples at 0.5 A g−1 are shown in Fig. S9 (ESI†), which proves that the most significant performance enhancement of VO2 is achieved at a Mo content of 2%. To show the superiority of Mo doping, we focus on the comparison of VO2 and VO2-Mo for the NH4+ storage using various tests. The cyclic voltammetry (CV) curves at various scan speeds within the potential window of −0.7–0.3 V are displayed in Fig. S10 (ESI†). The insertion/extraction of NH4+, which results in vanadium's valence change (IV ↔ III), is what causes the evident redox peaks to be seen. The CV curves at 1 mV s−1 are shown in Fig. 3a. Both VO2 and VO2-Mo have two pairs of redox peaks although a reduction peak is not obvious, which coincide with the two steps of NH4+ (de)intercalation. The redox peaks of VO2 are located at about −0.539/–0.659 V and −0.029/–0.356 V, whereas, the redox peaks of VO2-Mo are located at about −0.570/–0.631 V and −0.172/–0.422 V. Compared with VO2, VO2-Mo exhibits smaller potential gaps (0.061 V and 0.250 V vs. 0.120 V and 0.327 V) and higher peak current densities. Such phenomena reveal that VO2-Mo shows a smaller polarization because of better redox reaction kinetics and more rapid ion diffusion.57 The redox couples of VO2-Mo move to the center position compared to VO2, demonstrating that Mo-doping is not only good for the redox reaction in kinetics, but also reduces the Gibbs free energy in thermodynamics, thus leading to better electrochemical performances. Fig. 3b and Fig. S11 (ESI†) show the GCD curves for VO2 and VO2-Mo at various current densities, respectively, which reflect their rate performances summarized in Fig. 3c. The platforms in these GCD curves correspond with the redox reactions in their CV curves. The specific discharge capacities of VO2-Mo are around 370, 285, 225, 180, 137, and 87 mA h g−1, which are higher than those of VO2, which are about 232, 192, 143, 110, 78, and 44 mA h g−1, respectively, at 0.1, 0.2, 0.5, 1, 2, and 5 A g−1. After 50 cycles, the specific discharge capacities are approximately 210 mA h g−1 and 310 mA h g−1, respectively, when the current density reaches 0.1 A g−1. The GCD curves in Fig. S11† and Fig. 3b support poor coulombic efficiency (CE), which is the cause of the decrease in particular capacities. Notably, as shown in Fig. 3d and Table S1 (ESI†), the obtained specific capacities of VO2-Mo surpass those of many reported anode materials for ammonium ion storage, including defective VO2 (220 mA h g−1, 0.1 A g−1),40 VOPO4·2H2O (154.6 mA h g−1, 0.1 A g−1),58 d-VO (∼200 mA h g−1, 0.1 A g−1),39 MnAl-LDH (84 mA h g−1, 0.1 A g−1),59 FVO (88.6 mA h g−1, 0.1 A g−1),60 MoO3 (115 mA h g−1, 0.1 A g−1),29etc. In addition to the excellent specific capacity and multiple performances, the VO2-Mo anode material also has better cycling stability compared with VO2. As demonstrated in Fig. 3e, after approximately 6000 consecutive charging and discharging cycles at a current density of 2 A g−1, the specific capacity of VO2-Mo essentially stays constant, reaching approximately 140 mA h g−1 (98% of the initial value). In contrast, the specific capacity of VO2 stays at 37 mA h g−1 (51% of the initial value). Vanadium oxide materials’ inherent features, such as their slow breakdown and structural deterioration, are what lead to the decrease in the specific capacity of VO2.3,8,61 Such a result illustrates that the introduced Mo can stabilize the structure of VO2, thus leading to enhanced cycling durability. Compared with regular CE of VO2-Mo, the coulombic efficiency (CE) of VO2 fluctuates acutely (Fig. 3e), which also supports the conclusion of the stable structure of VO2-Mo for highly reversible NH4+ (de)intercalation. All results affirm that the overall electrochemical performances of VO2 can be well enhanced by the introduced Mo to produce appropriate oxygen defects.
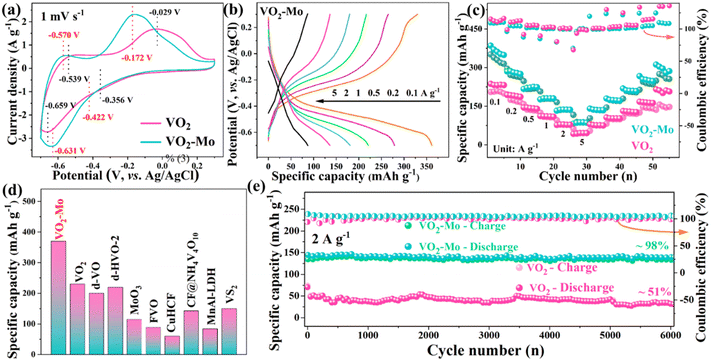 |
| Fig. 3 Electrochemical properties of VO2 and VO2-Mo: (a) comparison of CV curves at 1 mV s−1; (b) GCD curves of VO2-Mo at different current densities; (c) rate performances; (d) comparison of the capacity with literature studies; and (e) cycling performances. | |
Fig. 4a displays the CV curves at five distinct scanning rates of 0.2–1 mV s−1 in order to better evaluate the kinetics of VO2-Mo for NH4+ intercalation/deintercalation. The oxidation and reduction peaks invariably change slightly at different scanning speeds because of the polarization effect, but the shapes of the CV curves maintain a high degree of similarity, indicating that the material has good stability and remarkable electrochemical kinetics.62 The previous studies proved that these CV curves’ peak current (i) and scanning rate (v) should match with the following two equations:63
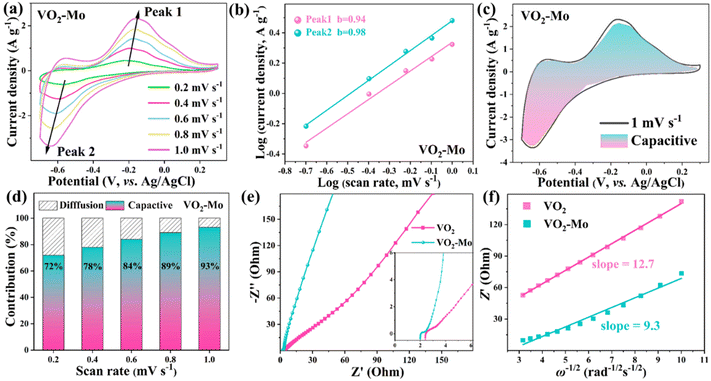 |
| Fig. 4 Electrochemical kinetic studies of VO2-Mo: (a) CV curves obtained at various scan rates; (b) the fitting log(i) vs. log(v) curves; (c) the capacitive contribution graph; (d) contribution ratios; (e) Nyquist charts and (f) the relationship of Z‘ − ω−1/2. | |
When a and b are both constants, then eqn (1) can be changed to:
The relationship between i and v is evident in eqn (2), where b denotes the slope relationship between log(i) and log(v). In particular, it is demonstrated that surface capacitance predominates when b is close to 1, and that ion diffusion's contribution to capacity prevails when b is close to 0.5. As shown in Fig. 4b, Peak 1 and Peak 2's computed b-values of 0.98 and 0.94, respectively, demonstrate that the surface capacitance contribution and a small amount of diffusion characteristics are the primary drivers of the ammonium ion storage process in VO2-Mo. The diffusion and capacitance contribution ratios of VO2-Mo in ammonium ion storage at different scanning rates can be calculated using eqn (3):30
where
k1v represents capacitive behavior,
k2v1/2 represents ion diffusion control,
k1 represents percentage contribution in response to capacitive behavior, and
k2 represents diffusion control percentage. The contribution ratios of diffusion and capacitance are shown in
Fig. 4d, while
Fig. 4c displays the capacitive contribution at 1 mV s
−1. When the CV scanning rate increases from 0.2 mV s
−1 to 1 mV s
−1, the percentage of capacitive contribution progressively increases from 72% to 93%. This is also similar to the trend when the
b value is close to 1. The result proves that VO
2-Mo has excellent charge reaction kinetics, which is an important reason for its excellent rate performance and highly reversible capacity. In order to reveal the intrinsic reason for this phenomenon, the conductivity of the VO
2-Mo and VO
2 electrode materials was examined using electrochemical impedance spectroscopy (EIS), as shown in
Fig. 4e. The electrode's charge transfer resistance (
Rct) is represented by the circular section of the Nyquist curve at high frequency, and the Warburg diffusion impedance associated with NH
4+ diffusion is represented by the linear portion at low frequencies.
64 It can be seen that the radius of the VO
2-Mo electrode is much smaller and its slope is steeper than that of VO
2, proving the low intrinsic resistance, VO
2-Mo's higher mass-transfer resistance and quick ion migration for NH
4+ storage.
65 The ion diffusion rate is indicated by the slope
b generated by the diffusion of ions in the low-frequency domain, as demonstrated by
Z′ −
ω−1/2 fitting curves (
Fig. 4f) with
b values of 12.7 and 9.3. The aforementioned findings clearly show that in the VO
2-Mo material, ammonium ions migrate more quickly and the rate of redox reaction is likewise faster. This indicates that the oxygen vacancies generated in VO
2 by employing Mo are favorable for electron transfer and accelerate ion diffusion, which explains well why the integrated electrochemical performances of VO
2-Mo are much better than that of VO
2.
Several ex situ characterization techniques, including XRD, FTIR, and XPS tests, were used to reveal the mechanism of NH4+-storage behavior in VO2-Mo electrodes during charging and discharging. Fig. 5f shows the five chosen states of A/D and pristine labeling on the GCD curves of the VO2-Mo electrode. The ex situ XRD patterns in different states (Fig. 5a) reveal that the VO2-Mo electrode shows the characteristic diffraction peaks of VO2 (JCPDS, No. 31-1438) before and after applying the potential except some peaks from the substrate Ti foil. This proves the highly stable structure of VO2-Mo for the ammoniation/deammoniation processes. The enlarged XRD patterns show the shift of the (110) peak during charging and discharging (Fig. 5b). When fully discharged to −0.7 V (from pristine to A), the (110) peak of the electrode material shifts to a higher angle, indicating that the ammonium ions are clearly inserted into the lattice structure of the material, and conversely, at a full charge state of 0.3 V (A to B), the (001) peak returns to a lower angle, indicating that the ammonium ions are deintercalated from the lattice of VO2-Mo. More impressively, when the applied potential is again reduced to −0.7 V (B to C) and improved to 0.3 V (C to D), the corresponding peak also returns to a higher (lower) degree, which corresponds to the state of A (B), strongly demonstrating that the NH4+ insertion/deinsertion reaction is highly reversible. It is also noteworthy that no impurities are detected in all XRD patterns, which implies that the whole charging/discharging process is very efficient and does not produce any by-products. Fig. 5e shows the ex situ FTIR spectra in different selected states, and the peaks associated with the V–O stretching vibrations appear in the low-wave number region (below 1100 cm−1). When NH4+ enters the tunneling structure of VO2-Mo during the discharge process, H atoms combine with the O atoms to form hydrogen bonds. The nitrogen–hydrogen bond's bending vibration is attributed to the peak at 1394 cm−1, and its stretching vibration is attributed to the peak at 3195 cm−1. The peaks at 2800 and 1078 cm−1 represent the change of the crystal structure during charging and discharging and correspond to hydrogen atoms in NH4+ bound to oxygen atoms in the VO2-Mo main skeleton.66 When ammonium ions deintercalate (charge) or intercalate (discharge), their vibrational peaks’ intensity correspondingly reduces or increases. To further investigate the bonding mechanism of the VO2-Mo electrode for storing NH4+, we performed ex situ XPS tests for both full charge and full discharge processes. The ex situ entire XPS spectra of full charging and full discharging procedures are displayed in Fig. S12a (ESI†) and the results show that there are slight differences in the strengths and positions of the peaks corresponding to N 1s, V 2p, and Mo 3d under the full charging and full discharging conditions, which suggests that they may be related to the mechanism of ammonium ion storage. The ex situ XPS spectra of N 1s, V 2p, and Mo 3d during full charging and full discharging are shown in Fig. 5c, d and Fig. S12 (ESI†), respectively. The intensity of N 1s is obviously much weaker in the fully charged state than that in the fully discharged state, and the spectra of N 1s are mainly attributed to the protonated state (–NH+–, 400.4 eV) and the –NH– segment (402.0 eV) (Fig. 5c).41 The strong evidence that the charging and discharging processes are accompanied by the embedding (discharge) and detachment (charge) of NH4+ by hydrogen bond is provided by the intensity variation of NH+–, which is consistent with the results reported by the previous report.62 In addition, the oxidation state changes reflected by the ex situ XPS spectra of V 2p (Fig. 5d) and Mo 3d (Fig. S12†) can again confirm the above mechanism: accompanied by NH4+ embedding in the discharge process, the contents of +3 (V/Mo) increase. Nevertheless, the contents of +4 (V/Mo) decrease accompanied by NH4+ detachment in the charging process.12 Based on the above characterization, it can be fully demonstrated that the energy storage mechanism of the VO2-Mo electrode is as follows: during charging and discharging, NH4+ is delocalized/embedded from the tunneling structure and accompanied by the breaking and generation of hydrogen bonds, during which the metal V and Mo also produce corresponding valence changes to balance the charge. The VO2-Mo electrode's NH4+ storage mechanism can be visually represented as illustrated in Fig. 5f, which is based on a thorough conclusion presented above. The reaction during charging and discharging is expressed by the following equation: VO2-Mo + xNH4+ + xe− ↔ Mo-(NH4)xVO2-Mo. The NH4+ storage mechanism of the VO2-Mo electrode is demonstrated by the creation and disruption of hydrogen bonds, which is in keeping with the previously reported V-based materials for ammonium ion storage.27,38,41,54,67
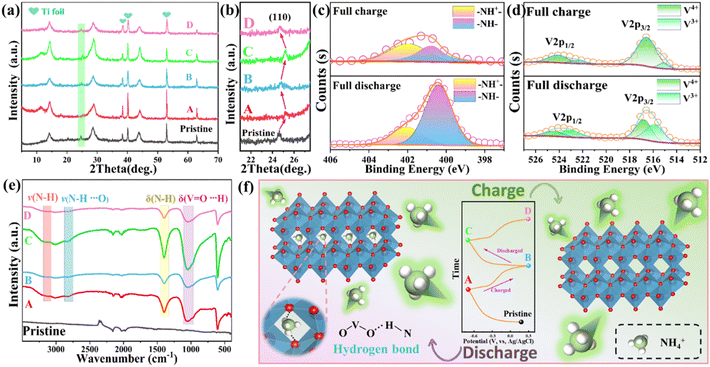 |
| Fig. 5 Electrochemical mechanism of VO2-Mo for ammonium ion storage: (a and b) the five points’ equivalent ex situ XRD patterns; (c and d) the ex situ XPS spectra; (e) the ex situ FTIR spectra corresponding to the five points; and (f) mechanism diagram of ammonium ion storage during charging/discharging processes, inserting the GCD curves. | |
2.3. VO2-Mo//PANI aqueous NH4+ batteries
To further evaluate the practicality of VO2-Mo electrodes, as shown in Fig. 6a, we attempted to fabricate an aqueous NH4+ cell. The structural characterization and electrochemical characterization of PANI are described in Fig. S13 (ESI†). PANI's CV curves display a few redox reactions, which indicates that it can store NH4+. Its operating potential range is −0.3–0.7 V and PANI's specific discharge capacities at current densities of 0.2, 0.5, 1, 2, and 5 A g−1 are approximately 110, 98, 89, 80, and 67 mA h g−1, respectively. The above results predict that PANI as a cathode can be better paired with an anode composed of VO2-Mo.68Fig. 6b and Fig. S14a (ESI†) show the CV curves of VO2-Mo//PANI and VO2//PANI aqueous NH4+ batteries at different scanning speeds, respectively, and both of them can operate well in the selected voltage window up to 1.4 V. The redox peaks correspond with the (de)intercalation of NH4+ discussed above. The integrated area of the VO2-Mo//PANI battery is significantly bigger than that of the VO2//PANI battery, as shown by the comparison of the CV curves of the two batteries at 1 mV s−1 (Fig. S14b†), proving the superior performances of VO2-Mo//PANI battery. In order to investigate the intrinsic reasons for the advanced VO2-Mo//PANI battery, the conductivities of VO2-Mo//PANI and VO2//PANI are respectively investigated by EIS (Fig. S15, ESI†). As expected, the VO2-Mo//PANI battery has lower electrical conductivity and better charge/ion transfer behavior, which can achieve efficient and rapid ammonium ion storage. The rate performances and corresponding GCD curves of the VO2-Mo/PANI battery at various current densities are shown in Fig. 6c and d. The redox peaks in CV curves match with the platforms in these GCD curves (Fig. 6b). The complete battery shows reversible specific discharge capacities of approximately 232, 171, 135, 102, and 62 mA h g−1 for current densities of 0.2, 0.5, 1, 2, and 5 A g−1, respectively, which is encouraging. The specific discharge capacities of the full battery rapidly recover to about 220 mA h g−1 and 235 mA h g−1 when the current densities are restored to 0.2 and 0.1 A g−1. The VO2-Mo//PANI battery achieves the specific discharge capacity as high as ca. 232 mA h g−1 at 0.2 A g−1, which surpasses that of the state-of-the-art AAIBs, such as, MnAlLDH//PTCDI (60 mA h g−1, 0.1 A g−1),69 MnO2//d-VO (181 mA h g−1, 0.1 A g−1),39 γ-MnO2//WO3 (106 mA h g−1, 0.5 A g−1),70 Mn3(PO4)2·7H2O//MoOx (98 mA h g−1, 0.1 A g−1),71 NiHCF//1,5-NAPD (143 mA h g−1, 0.1 A g−1)72 and so on. The cycling stability with CE at 5 A g−1 is shown in Fig. 6g. After 6000 cycles, the complete battery reaches a specific discharge capacity of roughly 55 mA h·g−1, with an 85% cycle retention, as well as the CE around 100% during the repeated charge/discharge processes. Such results prove the superior cycling stability of the VO2-Mo//PANI battery, in line with the results of the three-electrode system (Fig. 3e). To further illustrate the merits of the VO2-Mo//PANI battery, Ragone plots compared with the previously reported batteries for ammonium ion storage are shown in Fig. 6e. Based on the weight of VO2-Mo, the discharge energy density (E) of VO2-Mo/PANI battery can reach up to 133 W h kg−1 at a power density (P) of 89 W kg−1. As shown in Table S2 (ESI†), the achieved E and P values are superior to those of previous NH4+ storage devices, including ACC@VPP//PTCDI,35 MoO3@C//MoO3@C,73 NVO//AC,54 MnAlLDH//PTCDI,69 KVO/PANI//AC,30 MoO3//CuFe PBA,29 PVO//AC,74 MnO2//d-VO,39etc. The above characteristics prove the good energy output of the designed VO2-Mo//PANI battery. Furthermore, a LED is powered by a series battery system to demonstrate the viability of this ammonium ion battery's practical application. As shown in Fig. 6f, the red LED has been successfully lit continuously for more than 30 minutes, showing the stable operating characteristics of the designed VO2-Mo//PANI battery. In summary, the entire battery exhibits strong electrochemical properties and stable cycling, making it suitable for large-scale energy storage system production in the future.
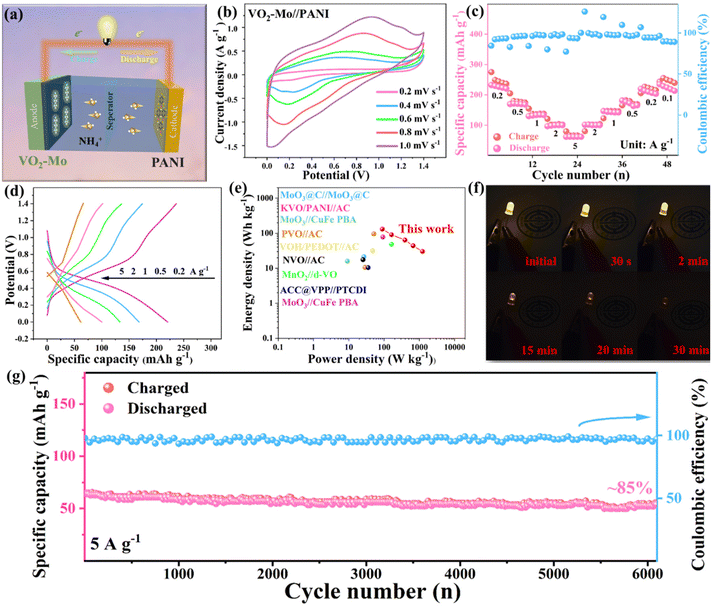 |
| Fig. 6 The complete cell performance of VO2-Mo/PANI: (a) schematic diagram of a VO2-Mo/PANI battery; (b) CV curves of a VO2-Mo//PANI battery at different scan rates; (c) rate performances of a VO2-Mo//PANI battery; (d) GCD curves of a VO2-Mo//PANI battery at different voltage windows; (e) Ragone plots; (f) demonstration of the VO2-Mo//PANI battery to power an LED light; and (g) cycling stability and CE of a VO2-Mo//PANI battery. | |
2.4. DFT calculations
Both the three- and two-electrode systems attest that the Mo atom in VO2 can boost the NH4+-storage properties of VO2. We conducted the density functional theory (DFT) calculations to uncover the underlying reason, whose information is shown in the ESI† in detail. The structures of VO2 and VO2-Mo models at different orientated views are depicted in Fig. 7a–d, which clearly represent the tunnel-like structure of VO2. They are built, optimized and used to calcaulate the band structures, density of state (DOS), partial DOS (PDOS) and electron density difference during the DFT calculations. The band structures of VO2 and VO2-Mo are shown in Fig. 7e and f. The bandgap of VO2-Mo (Fig. 7f) decreases to 1.81 eV compared with VO2 (1.99 eV, Fig. 7e), which demostrates that the introduction of Mo increases the electronic conductivity and lowers the electron transport barrier, in line with the EIS spectra disucssed in Fig. 4e, f and Fig. S15.† Besides, in the band structures of VO2-Mo, the energy bands become flat and the bandwidth decreases, which imply the current carrier concentration increases. It is good for the flow of electrons, enhancing the electrochemcial properteis of VO2-Mo.75 Fig. S16a (ESI†) shows the DOS curves of VO2 and VO2-Mo and Fig. S16b and c (ESI†) depicts their corresponding PDOS curves of O 2p and V 3d. After doping with the element Mo, we can find that both vanadium 3d orbitals and oxygen 2p orbitals move closer to the Fermi energy level, and the increase in the energy level of the electrons in the two orbitals means that their increased activity is one of the causative factors for the instability of the binding of positively charged protons with the ammonium ion. This feature can decrease the strength of the H-bond and the weak H-bond can greatly boost the NH4+-storage.76 The electron density differences of VO2 and VO2-Mo are compared in Fig. 7g and h, which reflect the change of electron cloud. After the introduction of Mo, both positive and negative charges are increased, which implies the electrons’ localization of VO2-Mo. This feature can modulate the strength of the H-bond. Therefore, the electronic conductivity is enhanced and the electron transport barrier is lowered by Mo atoms in VO2, resulting in the boosted NH4+ kinetics during the charge and discharge processes. The structural models of VO2 and VO2-Mo for calculating the adsorption energy with ammonium ion are shown in Fig. S17 (ESI†). VO2-Mo exhibits a higher adsorption energy than that of VO2, which supports the point that the H-bond becomes weak after the introduction of Mo atoms, thus enhancing the NH4+ kinetics. All the experimental and DFT results unequivocally prove that the Mo-doping strategy can serve as a useful tool to boost ammonium-ion storage properties of materials for AAIBs.
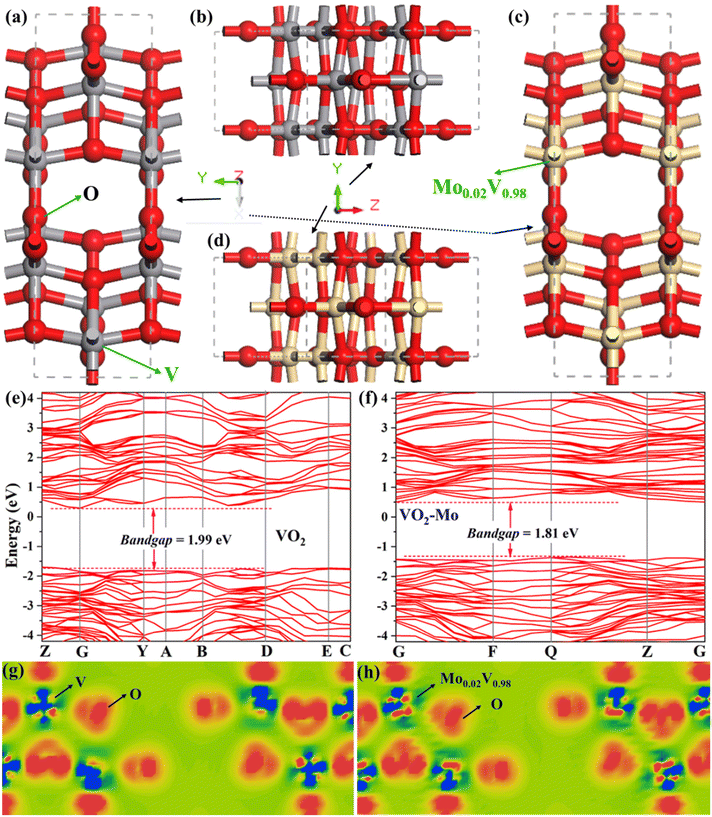 |
| Fig. 7 The results of DFT calculations: (a and b) the structures of VO2; (c and d) the structures of VO2-Mo; (e) the band structure of VO2; (f) the band structure of VO2-Mo; (g) the electron density difference of VO2; and (h) the electron density difference of VO2-Mo. | |
3. Conclusion
In conclusion, we demonstrate that VO2's electronic structure is tailored by Mo doping (VO2-Mo) to enhance ammonium-ion storage properties toward highly efficient AAIBs. VO2-Mo is successfully prepared by adjusting the content of Mo via a facile hydrothermal method. The introduction of Mo can increase the oxygen defects in VO2-Mo, which improves the electronic structure properties and provides more active sites and smooth migration paths for ammonium ions. Both experimental results and DFT calculations reveal that the guest Mo atom in the VO2 crystal structure can speed up ion transport, increase mass transfer, and improve kinetics, leading to improved NH4+-storage properties. VO2-Mo (2% Mo) exhibits a specific discharge capacity of about 370 mA h g−1 at 0.1 A g−1, higher than that of VO2 (232 mA h g−1) and the reported vanadium oxides for NH4+-storage. The capacity of 140 mA h g−1 basically remains unchanged after about 6000 consecutive charging and discharging processes at 2 A g−1. The creation of a hydrogen bond between NH4+ and VO2-Mo, which is corroborated by ex situ XRD, FTIR, and XPS, is the mechanism for storing NH4+. The VO2-Mo//PANI complete cell outperforms the most advanced electrode materials for AAIBs, achieving a specific discharge capacity of up to 232 mA h g−1 at 0.2 A g−1. At 89 W kg−1, the E value of the VO2-Mo//PANI battery can reach up to 133 W h kg−1. In addition to meeting the anticipated future need for the large-scale production of aqueous NH4+ energy storage systems, our work offers fresh insights into the creation of tailored electrode materials with increased NH4+-storage for AAIBs.
Data availability
The data supporting this article have been included as part of the ESI.†
Conflicts of interest
There are no conflicts to declare.
Acknowledgements
This work is supported by the Doctoral Research Start-up Fund of Hubei University of Science and Technology (BK202504) and the Natural Science Foundation of Liaoning Province (2023-MS-115).
References
- J. Han, A. Varzi and S. Passerini, The Emergence of Aqueous Ammonium-Ion Batteries, Angew. Chem., Int. Ed., 2022, 61, e202115046 CrossRef CAS PubMed.
- Z. Tian, J. Yin, T. Guo, Z. Zhao, Y. Zhu, Y. Wang, J. Yin, Y. Zou, Y. Lei, J. Ming, O. Bakr, O. F. Mohammed and H. N. Alshareef, A Sustainable NH4+ Ion Battery by Electrolyte Engineering, Angew. Chem., Int. Ed., 2022, 61, e202213757 CrossRef CAS PubMed.
- H. Jiang, Y. Zhang, M. Waqar, J. Yang, Y. Liu, J. Sun, Z. Feng, J. Sun, Z. Pan, C. Meng and J. Wang, Anomalous Zn2+ Storage Behavior in Dual-Ion-In-Sequence Reconstructed Vanadium Oxides, Adv. Funct. Mater., 2023, 33, 2213127 CrossRef CAS.
- X. Dong, Y. Peng, Y. Wang, H. Wang, C. Jiang, C. Huang, C. Meng and Y. Zhang, Hemimorphite /C interface layer with dual-effect methodically redistricted Zn2+ deposition behavior for dendrite-free zinc metal anodes, Energy Storage Mater., 2023, 62, 102937 CrossRef.
- H. Yu, Z. Wang, R. Zheng, L. Yan, L. Zhang and J. Shu, Toward Sustainable Metal-Iodine Batteries: Materials, Electrochemistry and Design Strategies, Angew. Chem., Int. Ed., 2023, 62, e202308397 CrossRef CAS PubMed.
- M. Li, J. Lu, Z. Chen and K. Amine, 30 Years of Lithium-Ion Batteries, Adv. Mater., 2018, 30, 1800561 CrossRef PubMed.
- Q. Chen, W. Liang, Z. Tang, J. Jin, J. Zhang, G. Hou, L. Mai and Y. Tang, Aqueous ammonium ion storage materials: A structure perspective, Mater. Today, 2024, 72, 359–376 CrossRef CAS.
- Z. Feng, Y. Zhang, Z. Gao, D. Hu, H. Jiang, T. Hu, C. Meng and Y. Zhang, Construction interlayer structure of hydrated vanadium oxides with tunable P-band center of oxygen towards enhanced aqueous Zn-ion batteries, Adv. Powder Mater., 2024, 3, 100167 CrossRef.
- H. Hong, J. Zhu, Y. Wang, Z. Wei, X. Guo, S. Yang, R. Zhang, H. Cui, Q. Li, D. Zhang and C. Zhi, Metal-Free Eutectic Electrolyte with Weak Hydrogen Bonds for High-Rate and Ultra-Stable Ammonium-Ion Batteries, Adv. Mater., 2024, 36, 2308210 CrossRef CAS PubMed.
- A. Kulkarni, C. Padwal, J. MacLeod, P. Sonar, T. Kim and D. Dubal, Toward Safe and Reliable Aqueous Ammonium Ion Energy Storage Systems, Adv. Energy Mater., 2024, 14, 2400702 CrossRef CAS.
- Q. Chen, J. Jin, M. Song, X. Zhang, H. Li, J. Zhang, G. Hou, Y. Tang, L. Mai and L. Zhou, High-Energy Aqueous Ammonium-Ion Hybrid Supercapacitors, Adv. Mater., 2022, 34, 2107992 CrossRef CAS PubMed.
- Y. Song, Q. Pan, H. Lv, D. Yang, Z. Qin, M. Y. Zhang, X. Sun and X. X. Liu, Ammonium-Ion Storage Using Electrodeposited Manganese Oxides, Angew. Chem., Int. Ed., 2021, 60, 5718–5722 CrossRef CAS PubMed.
- D. Chao, W. Zhou, F. Xie, C. Ye, H. Li, M. Jaroniec and S.-Z. Qiao, Roadmap for advanced aqueous batteries: From design of materials to applications, Sci. Adv., 2020, 6, eaba4098 CrossRef CAS PubMed.
- W. Guo, Z. Wu, S. Zhao, J. Zhao, X. Han, B. Wanyan, J. Wang, L. Yan, L. Zhang, H. Yu, T.-F. Yi and J. Shu, Zinc hexacyanoferrate with open framework for efficient aqueous cobalt ions storage, Chem. Eng. J., 2024, 499, 156480 CrossRef CAS.
- W. Guo, T. Zhang, G. Shu, L. Fan, Z. Wu, L. Yan, L. Zhang, H. Yu, T.-F. Yi and J. Shu, Nanocarbon armor reinforced Ag particles to build a high-rate and long-lifespan aqueous Zn2+/Cl− dual-ion battery, Inorg. Chem. Front., 2024, 11, 2798–2806 RSC.
- B. Wanyan, Y. Zhan, Z. Miao, W. Guo, J. Wang, L. Yan, L. Zhang, H. Yu, T.-F. Yi and J. Shu, Energetic aqueous zinc-sulfur battery achieved via co-solvent and redox mediator synergistic regulation, Composites, Part B, 2024, 284, 111709 CrossRef CAS.
- Y. Wang, T. Ou, Y. Dong, L. Chen, Y. Huang, D. Sun, W. Qiang, X. Pei, Y. Li and Y. Tan, A Green Asymmetric Bicyclic Co-Solvent Molecule for High-Voltage Aqueous Lithium-Ion Batteries, Adv. Mater., 2024, 36, 2311009 CrossRef CAS PubMed.
- C. Yang, J. Chen, X. Ji, T. P. Pollard, X. Lü, C.-J. Sun, S. Hou, Q. Liu, C. Liu, T. Qing, Y. Wang, O. Borodin, Y. Ren, K. Xu and C. Wang, Aqueous Li-ion battery enabled by halogen conversion–intercalation chemistry in graphite, Nature, 2019, 569, 245–250 CrossRef CAS PubMed.
- L. Jiang and Y.-C. Lu, Building a Long-Lifespan Aqueous K-Ion Battery Operating at −35 °C, ACS Energy Lett., 2024, 9, 985–991 CrossRef CAS.
- H. Wu, J. Hao, Y. Jiang, Y. Jiao, J. Liu, X. Xu, K. Davey, C. Wang and S.-Z. Qiao, Alkaline-based aqueous sodium-ion batteries for large-scale energy storage, Nat. Commun., 2024, 15, 575 CrossRef CAS PubMed.
- M. Huang, X. Wang, J. Wang, J. Meng, X. Liu, Q. He, L. Geng, Q. An, J. Yang and L. Mai, Proton/Mg2+ Co-Insertion Chemistry in Aqueous Mg-Ion Batteries: From the Interface to the Inner, Angew. Chem., Int. Ed., 2023, 62, e202308961 CrossRef CAS PubMed.
- D. Kundu, B. D. Adams, V. Duffort, S. H. Vajargah and L. F. Nazar, A high-capacity and long-life aqueous rechargeable zinc battery using a metal oxide intercalation cathode, Nat. Energy, 2016, 1, 16119 CrossRef CAS.
- J. Sun, Y. Zhao, Y. Liu, H. Jiang, D. Chen, L. Xu, T. Hu, C. Meng and Y. Zhang, Synthesis of V2O5·nH2O nanobelts@polyaniline core–shell structures with highly efficient Zn2+ storage, J. Colloid Interface Sci., 2023, 633, 923–931 CrossRef CAS PubMed.
- Z. Feng, Y. Zhang, H. Jiang, Y. Liu, J. Sun, T. Hu, J. Sun, C. Meng and J. Wang, On the origin of enhanced electrochemical kinetics in guest-ions pre-intercalated layered vanadium oxides: Interlayer spacing vs lattice distortion, Energy Storage Mater., 2024, 71, 103552 CrossRef.
- R. Zheng, Y. Li, H. Yu, X. Zhang, D. Yang, L. Yan, Y. Li, J. Shu and B.-L. Su, Ammonium Ion Batteries: Material, Electrochemistry and Strategy, Angew. Chem., Int. Ed., 2023, 62, e202301629 CrossRef CAS PubMed.
- Y. Pan, L. Yuan, L. Liu, W. Fang, Y. Hou, L. Fu and Y. Wu, Critical Advances of Aqueous Rechargeable Ammonium Ion Batteries, Small Struct., 2023, 4, 2300201 CrossRef CAS.
- S. Dong, W. Shin, H. Jiang, X. Wu, Z. Li, J. Holoubek, W. F. Stickle, B. Key, C. Liu, J. Lu, P. A. Greaney, X. Zhang and X. Ji, Ultra-fast NH4+ Storage: Strong H Bonding between NH4+ and Bi-layered V2O5, Chem, 2019, 5, 1537–1551 CAS.
- M. R. Lukatskaya, O. Mashtalir, C. E. Ren, Y. Dall'Agnese, P. Rozier, P. L. Taberna, M. Naguib, P. Simon, M. W. Barsoum and Y. Gogotsi, Cation Intercalation and High Volumetric Capacitance of Two-Dimensional Titanium Carbide, Science, 2013, 341, 1502–1505 CrossRef CAS PubMed.
- G. Liang, Y. Wang, Z. Huang, F. Mo, X. Li, Q. Yang, D. Wang, H. Li, S. Chen and C. Zhi, Initiating Hexagonal MoO3 for Superb-Stable and Fast NH4+ Storage Based on Hydrogen Bond Chemistry, Adv. Mater., 2020, 32, e1907802 CrossRef PubMed.
- X. Chen, Z. Feng, X. Dong, H. Jiang, C. Meng and Y. Zhang, Synergistic effect of K+ and PANI in vanadium oxide hydration by interlayer engineering boosts the ammonium ion storage, SusMat, 2023, 3, 263–275 CrossRef CAS.
- K. Itaya, T. Ataka and S. Toshima, Spectroelectrochemistry and electrochemical preparation method of Prussian blue modified electrodes, J. Am. Chem. Soc., 1982, 104, 4767–4772 CrossRef CAS.
- C. D. Wessells, S. V. Peddada, M. T. McDowell, R. A. Huggins and Y. Cui, The Effect of Insertion Species on Nanostructured Open Framework Hexacyanoferrate Battery Electrodes, J. Electrochem. Soc., 2011, 159, A98–A103 CrossRef.
- Y. Wu, Z. Xu, R. Ren, N. Lv, J. Yang, J. Zhang, H. Ren, S. Dong and X. Dong, Flexible Ammonium-Ion Pouch Cells Based on a Tunneled Manganese Dioxide Cathode, ACS Appl. Mater. Interfaces, 2023, 15, 12434–12442 CrossRef CAS PubMed.
- X. Wu, Y. Xu, H. Jiang, Z. Wei, J. J. Hong, A. S. Hernandez, F. Du and X. Ji, NH4+ Topotactic Insertion in Berlin Green: An Exceptionally Long-Cycling Cathode in Aqueous Ammonium-Ion Batteries, ACS Appl. Energy Mater., 2018, 1, 3077–3083 CrossRef CAS.
- X. Chen, P. Wang, Z. Feng, C. Meng and Y. Zhang, Conductive polymer intercalated vanadium oxide on carbon cloth for fast ammonium-ion storage in supercapacitor applications, Chem. Eng. J., 2022, 445, 136747 CrossRef CAS.
- Q. Chen, Z. Tang, H. Li, W. Liang, Y. Zeng, J. Zhang, G. Hou and Y. Tang, Cobalt Ion-Stabilized VO2 for Aqueous Ammonium Ion Hybrid Supercapacitors, ACS Appl. Mater. Interfaces, 2024, 16, 18824–18832 CrossRef CAS PubMed.
- X. Zhang, H. Wei, B. Ren, J. Jiang, G. Qu, J. Yang, G. Chen, H. Li, C. Zhi and Z. Liu, Unlocking High-Performance Ammonium-Ion Batteries: Activation of In-Layer Channels for Enhanced Ion Storage and Migration, Adv. Mater., 2023, 35, 2304209 CrossRef CAS PubMed.
- X. Mu, Y. Song, Z. Qin, J. Meng, Z. Wang and X.-X. Liu, Core-shell structural vanadium Oxide/Polypyrrole anode for aqueous Ammonium-Ion batteries, Chem. Eng. J., 2023, 453, 139575 CrossRef CAS.
- Y. Wu, S. Dong, N. Lv, Z. Xu, R. Ren, G. Zhu, B. Huang, Y. Zhang and X. Dong, Unlocking the High Capacity Ammonium-Ion Storage in Defective Vanadium Dioxide, Small, 2022, 18, 2204888 CrossRef CAS PubMed.
- J. n. Gong, P. Bai, J. Sun, Y. Liu, X. Dong, T. Hu, C. Meng and Y. Zhang, Adjusting oxygen vacancy of VO2·xH2O nanoarray architectures for efficient NH4+ storage, Nano Res., 2024, 17, 2646–2654 CrossRef CAS.
- Y. Liu, Z. Feng, H. Jiang, X. Dong, C. Meng and Y. Zhang, Tailoring NH4+ Storage by Regulating Oxygen Defect in Ammonium Vanadate, Green Energy Environ., 2024, 9, 1171 CrossRef CAS.
- Z. Zhang, Z. Gao, T. Lv, Y. Liu, Z. Zhou, D. Chen, T. Hu, C. Meng and Y. Zhang, Topochemical behavior of ferrocene embedded in V2O5·nH2O with weak hydrogen bonding enhancing ammonium-ion storage, J. Colloid Interface Sci., 2024, 671, 78–87 CrossRef CAS PubMed.
- Z. Zhang, Y. Zhang, Z. Gao, Y. Liu, J. Gong, J. Sun, Z. Feng, T. Hu and C. Meng, Polypyrrole intercalated into the interlayer of vanadium oxide hydration boosts NH4+ storage for aqueous ammonium ion batteries, J. Energy Storage, 2024, 83, 110623 CrossRef.
- Y. Wang, L. Li, S. Wang, X. Dong, C. Ding, Y. Mu, M. Cui, T. Hu, C. Meng and Y. Zhang, Anion Structure Regulation of Cobalt Silicate Hydroxide Endowing Boosted Oxygen Evolution Reaction, Small, 2024, 20, 2401394 CrossRef CAS PubMed.
- Y. Liu, Z. Shao, T. Lv, Z. Zhang, Z. Zhou, T. Hu, C. Meng and Y. Zhang, Conjugated polyaniline as “conveyor” in tungstate boosting cation storage for high-performance aqueous batteries, Green Energy Environ., 2024 DOI:10.1016/j.gee.2024.1006.1004.
- Y. Li, J. Chen, L. Su, X. Zhang, Q. Zheng, Y. Huo and D. Lin, Molybdenum-optimized electronic structure and micromorphology to boost zinc ions storage properties of vanadium dioxide nanoflowers as an advanced cathode for aqueous zinc-ion batteries, J. Colloid Interface Sci., 2023, 652, 440–448 CrossRef CAS PubMed.
- S. Wang, H. Zhang, K. Zhao, W. Liu, N. Luo, J. Zhao, S. Wu, J. Ding, S. Fang and F. Cheng, Designing interstitial boron-doped tunnel-type vanadium dioxide cathode for enhancing zinc ion storage capability, Carbon Energy, 2023, 5, e330 CrossRef CAS.
- X. Tan, F. Zhang, D. Chen, J. n. Gong, J. Sun, C. Meng and Y. Zhang, One-step hydrothermal synthesis of vanadium dioxide/carbon core–shell composite with improved ammonium ion storage for aqueous ammonium-ion battery, J. Colloid Interface Sci., 2024, 669, 2–13 CrossRef CAS PubMed.
- L. Chen, J. Zhang, Z. Wang and D. Wang, Enhancing ammonium-ion storage in Mo-doped VO2(B) nanobelt-bundles anode for aqueous ammonium-ion batteries, Nanoscale, 2024, 16, 12624–12634 RSC.
- Z. Zhou, T. Lv, Z. Gao, D. Chen, H. Jiang, C. Meng and Y. Zhang, Mo modulating the structure of monoclinic vanadium dioxide boosting the aqueous ammonium-ion storage for high-performance supercapacitor, J. Colloid Interface Sci., 2024, 676, 947–958 CrossRef CAS PubMed.
- N. Wang, Y. Zhang, T. Hu, Y. Zhao and C. Meng, Facile hydrothermal synthesis of ultrahigh-aspect-ratio V2O5 nanowires for high-performance supercapacitors, Curr. Appl. Phys., 2015, 15, 493–498 CrossRef.
- S. Deng, H. Li, B. Chen, Z. Xu, Y. Jiang, C. Li, W. Xiao and X. Yan, High performance of Mn-doped VO2 cathode for aqueous zinc-ion batteries: An insight into Zn2+ storage mechanism, Chem. Eng. J., 2023, 452, 139115 CrossRef CAS.
- C. Zhao, Y. Liu, X. Wu and S. Luo, Graphene Oxide Wrapped VO2 Nanobelts for Calendar-Life Zn Storage Devices, Adv. Sustainable Syst., 2024, 8, 2400077 CrossRef CAS.
- P. Wang, Y. Zhang, H. Jiang, X. Dong and C. Meng, Ammonium vanadium oxide framework with stable NH4+ aqueous storage for flexible quasi-solid-state supercapacitor, Chem. Eng. J., 2022, 427, 131548 CrossRef CAS.
- Y. Zhang, X. Jing, Y. Cheng, T. Hu and C. Meng, Controlled synthesis of 3D porous VO2(B) hierarchical spheres with different interiors for energy storage, Inorg. Chem. Front., 2018, 5, 2798–2810 RSC.
- S. Peters, C. Rieg, S. Bartling, M. Parlinska-Wojtan, M. Dyballa, S. Wohlrab and A. M. Abdel-Mageed, Accessibility of Reactants and Neighborhood of Mo Species during Methane Aromatization Uncovered by Operando NAP-XPS and MAS NMR, ACS Catal., 2023, 13, 13056–13070 CrossRef CAS.
- J. Zheng, C. Liu, M. Tian, X. Jia, E. P. Jahrman, G. T. Seidler, S. Zhang, Y. Liu, Y. Zhang, C. Meng and G. Cao, Fast and reversible zinc ion intercalation in Al-ion modified hydrated vanadate, Nano Energy, 2020, 70, 104519 CrossRef CAS.
- F. Ye, R. Pang, C. Lu, Q. Liu, Y. Wu, R. Ma and L. Hu, Reversible Ammonium Ion Intercalation/de-intercalation with Crystal Water Promotion Effect in Layered VOPO4·2H2O, Angew. Chem., Int. Ed., 2023, 62, e202303480 CrossRef CAS PubMed.
- C. Li, W. Yan, S. Liang, P. Wang, J. Wang, L. Fu, Y. Zhu, Y. Chen, Y. Wu and W. Huang, Achieving a high-performance Prussian blue analogue cathode with an ultra-stable redox reaction for ammonium ion storage, Nanoscale Horiz., 2019, 4, 991–998 RSC.
- W. Xu, L. Zhang, K. Zhao, X. Sun and Q. Wu, Layered ferric vanadate nanosheets as a high-rate NH4+ storage electrode, Electrochim. Acta, 2020, 360, 137008 CrossRef CAS.
- Y. Liu, Y. Zhang, H. Jiang, J. Sun, Z. Feng, T. Hu, C. Meng and Z. Pan, Synergistic engineering of oxygen-defect and heterojunction boosts Zn2+ (De)intercalation kinetics in vanadium oxide for high-performance zinc-ion batteries, Chem. Eng. J., 2022, 435, 134949 CrossRef CAS.
- D. Yu, Z. Wei, X. Zhang, Y. Zeng, C. Wang, G. Chen, Z. X. Shen and F. Du, Boosting Zn2+ and NH4+ Storage in Aqueous Media via In situ Electrochemical Induced VS2/VOx Heterostructures, Adv. Funct. Mater., 2021, 31, 2008743 CrossRef CAS.
- V. Augustyn, J. Come, M. A. Lowe, J. W. Kim, P.-L. Taberna, S. H. Tolbert, H. D. Abruña, P. Simon and B. Dunn, High-rate electrochemical energy storage through Li+ intercalation pseudocapacitance, Nat. Mater., 2013, 12, 518–522 CrossRef CAS PubMed.
- G. Fang, S. Liang, Z. Chen, P. Cui, X. Zheng, A. Pan, B. Lu, X. Lu and J. Zhou, Simultaneous Cationic and Anionic Redox Reactions Mechanism Enabling High-Rate Long-Life Aqueous Zinc-Ion Battery, Adv. Funct. Mater., 2019, 29, 1905267 CrossRef CAS.
- S. Zhang, K. Zhu, Y. Gao and D. Cao, A Long Cycle Stability and High Rate Performance Organic Anode for Rechargeable Aqueous Ammonium-Ion Battery, ACS Energy Lett., 2023, 8, 889–897 CrossRef CAS.
- H. Li, J. Yang, J. Cheng, T. He and B. Wang, Flexible aqueous ammonium-ion full cell with high rate capability and long cycle life, Nano Energy, 2020, 68, 104369 CrossRef CAS.
- D. Chao and H. J. Fan, Intercalation Pseudocapacitive Behavior Powers Aqueous Batteries, Chem, 2019, 5, 1359–1361 CAS.
- S. F. Kuchena and Y. Wang, Superior Polyaniline Cathode Material with Enhanced Capacity for Ammonium Ion Storage, ACS Appl. Energy Mater., 2020, 3, 11690–11698 CrossRef CAS.
- Q. Liu, F. Ye, K. Guan, Y. Yang, H. Dong, Y. Wu, Z. Tang and L. Hu, MnAl Layered Double Hydroxides: A Robust Host for Aqueous Ammonium-Ion Storage with Stable Plateau and High Capacity, Adv. Energy Mater., 2023, 13, 2202908 CrossRef CAS.
- X. Wen, J. Luo, K. Xiang, W. Zhou, C. Zhang and H. Chen, High-performance monoclinic WO3 nanospheres with the novel NH4+ diffusion behaviors for aqueous ammonium-ion batteries, Chem. Eng. J., 2023, 458, 141381 CrossRef CAS.
- D. Yang, Y. Song, M.-Y. Zhang, Z. Qin, J. Liu and X.-X. Liu, Solid–Liquid Interfacial Coordination Chemistry Enables High-Capacity Ammonium Storage in Amorphous Manganese Phosphate, Angew. Chem., Int. Ed., 2022, 61, e202207711 CrossRef CAS PubMed.
- L. Yan, Y.-e. Qi, X. Dong, Y. Wang and Y. Xia, Ammonium-ion batteries with a wide operating temperature window from −40 to 80 °C, eScience, 2021, 1, 212–218 CrossRef.
- J. Dai, X. Qi, L. Xia, Q. Xue, L. Luo, X. Wang, C. Yang, D. Li, H. Xie, A. Cabot, L. Dai and Y. Xu, Aqueous Ammonium-Ion Supercapacitors with Unprecedented Energy Density and Stability Enabled by Oxygen Vacancy-Enriched MoO3@C, Adv. Funct. Mater., 2023, 33, 2212440 CrossRef CAS.
- P. Wang, Y. Zhang, Z. Feng, Y. Liu and C. Meng, A dual-polymer strategy boosts hydrated vanadium oxide for ammonium-ion storage, J. Colloid Interface Sci., 2022, 606, 1322–1332 CrossRef CAS PubMed.
- X. Tan, F. Zhang, D. Chen, P. Wang, Y. Liu, C. Meng and Y. Zhang, Modulating NH4+ in vanadium oxide framework for high-efficient aqueous NH4+ storage, Chem. Eng. J., 2024, 489, 151119 CrossRef CAS.
- X. Shi, H. Liu, D. Xu, Y. Yu and X. Lu, Advanced Aqueous Ammonium-Ion Batteries Enabled by Hydrogen Bond Modulation, J. Phys. Chem. C, 2023, 127, 6233–6238 CrossRef CAS.
|
This journal is © the Partner Organisations 2025 |
Click here to see how this site uses Cookies. View our privacy policy here.