DOI:
10.1039/D4QI02614D
(Research Article)
Inorg. Chem. Front., 2025,
12, 369-378
Integrating multiple emission centers for photoluminescence regulation in copper–antimony/bismuth halides†
Received
16th October 2024
, Accepted 23rd November 2024
First published on 26th November 2024
Abstract
Zero-dimensional (0-D) heterometallic halides containing multiple metal–halogen units are emerging optoelectronic materials with diverse photophysical properties. In this work, eight 0-D ionic compounds, including heterometallic halides [(Tp)3CuX]MX6 (Tp = protonated thiomorpholine; X = Cl, Br; M = Sb, Bi), monometallic halides (Tp)3MX6 (M = Bi when X = Cl; M = Bi, Sb when X = Br), and (Tp)Br have been synthesized. Their structures and photoluminescence have been comparatively studied. Inserting a CuX unit into the three (Tp)+ cation moieties of (Tp)3MX6 results in the formation of [(Tp)3CuX]MX6 with a complex cation of [(Tp)3CuX]3+. The assembly of two distinct metal–halogen units in the heterometallic halide enables charge transfer between the complex cation and anion unit, as verified by DFT calculations, and meanwhile achieves the wide modulation of the luminescent color (green to red region) resulting from the integration of both complex cationic and anionic emission centers in one single lattice of [(Tp)3CuBr]MBr6. The luminescence mechanisms of monometallic and heterometallic halides are elucidated by detailed structural and spectral comparisons. Moreover, the compound [(Tp)3CuBr]SbBr6 shows significant potential for low-temperature optical temperature sensing applications based on the fluorescence intensity ratio of its dual emissions, with absolute sensitivity (Sa) and relative sensitivity (Sr) values of 0.078 K−1 and 5.38% K−1, respectively. This study not only provides a new strategy for developing heterometallic halide photoluminescence materials, but also offers new ideas for a better understanding of the photophysical mechanism of 0-D heterometallic halides.
Introductions
In recent years, zero-dimensional (0-D) inorganic–organic metal halides (IOMHs) have attracted much attention due to their excellent photophysical properties as well as the designable dual functionality of inorganic and organic moieties.1–7 IOMHs have potential applications in various optoelectronic fields such as light-emitting diodes,8,9 information storage and anti-counterfeiting encryption,10,11 and X-ray scintillation.12–14 IOMHs containing metal ions of the ns2 type (e.g., Sb3+ and Bi3+) are well known for their unique photophysical properties.4,15 The lone-pair stereo-activity of trivalent Sb3+ and Bi3+ leads to the formation of various metal halide anionic units with different geometrical configurations.16,17 However, their corresponding photoluminescence (PL) features are relatively humdrum. For example, Sb3+ usually emits orange-yellow light18,19 and Bi3+ usually emits blue light.20 Thus, to enrich the PL of Sb3+ or Bi3+ based halides, introducing multiple emission centers into them is an expectable strategy.
Indeed, the integration of different metal–halogen units in metal halides can enrich their structural diversity and designability of photophysical properties.21–36 For example, Zhi-Guo Xia et al. presented a series of compounds (C9NH20)9[Pb3X11](MX4)2 (C9NH20 = 1-buty-1-methylpyrrolidinium, X = Br and Cl, M = Mn, Fe, Co, Ni, Cu, and Zn) and emphasized the synergistic effects of the different metal halide units on photophysical properties.37 Bi-Wu Ma et al. reported a novel 0-D (bmpy)9[SbCl5]2[Pb3Cl11] (bmpy = 1-butyl-1-methylpyrrolidinium), in which the warm white light emissions could be obtained by combining green emission from [Pb3Cl11]5− and orange emission from [SbCl5]2− unit.21 Ze-Wei Quan et al. reported a novel 0-D hybrid metal halide [Pb(C12H24O6)Cl]2[Mn2Cl6] with broad yellow emission originating from the d–d transition of the [Mn2Cl6]2− dimer anion.30 However, these reports on 0-D heterometallic halides mainly focus on lead-based heterometallic halides,25,26,28–30,37,38 which are not environmentally friendly due to the biotoxicity of Pb. Cu(I)-based IOMHs (CunXm(L)z; X = Cl, Br, I; L = N, P, S-based ligands) are inexpensive and environmentally friendly, with a diversity of structures and PL properties.8,39–43 Therefore, the combination of ns2 metal–halogen and copper(I)–halogen units would endow IOMHs with interesting photophysical properties and even enable broadband luminescence modulation, which are still blank in the 0-D IOMHs family.
In this work, we synthesized eight 0-D ionic compounds, including [(Tp)3CuX]MX6 (Tp = protonated thiomorpholine; X = Cl, Br; M = Sb, Bi), (Tp)3MX6 (M = Bi when X = Cl; M = Bi, Sb when X = Br) and (Tp)Br to clarify the structure–property relationship in 0-D heterometallic halides. Their crystal structures, stability, and photophysical properties have been studied and compared. The heterometallic halides [(Tp)3CuX]MX6 are formed by introducing a CuX unit into the corresponding monometallic halides. The assembly of two distinct metal–halogen units in the heterometallic halide achieves charge transfer between the complex cation and anion unit, as verified by Density Functional Theory (DFT) calculations. Furthermore, such an assembly allows for the wide modulation of the emission color (green to red region), which results from the integration of both complex cationic and anionic emission centers in one single lattice. Through the detailed structural and spectral comparisons, their photophysical mechanisms are expounded. Our study not only introduces a new strategy for novel metal halides with rich luminescent behiviors, but also provides new insights into the photophysical mechanism of 0-D heterometallic halides.
Results and discussion
Crystal structure and stability
Considering the good coordination ability of S and Cu atoms, we therefore chose a S-containing organic, namely thiomorpholine, as a reactant. Single crystals of (Tp)Br were synthesized in a mixture of thiomorpholine and hydrohalic acid, which was heated to complete dissolution and then cooled naturally. Three monometallic halides (Tp)3MX6 were synthesized by mixing SbBr3 or BiX3 (X = Cl, Br) and thiomorpholine in a hydrohalic acid solution, which was heated to a completely clear solution and then cooled naturally. By using a similar synthetic method, the addition of CuX (X = Cl, Br) as a reactant would result in two copper–antimony heterometallic halides [(Tp)3CuX]SbX6 (X = Cl, Br) and two copper–bismuth heterometallic halides [(Tp)3CuX]BiX6 (X = Cl, Br) (Scheme 1). Detailed synthetic methods for all compounds are given in the Experimental section of the ESI.† The crystal structures of all compounds are determined using single crystal X-ray diffraction at both room temperature and 100 K. Tables S1–S20† provide crystallographic data, structural refinement details, selected bond lengths and bond angles for all title compounds, as well as hydrogen bonding data.
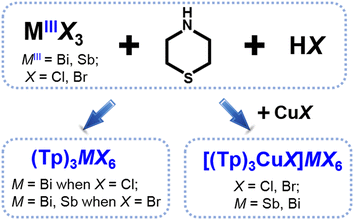 |
| Scheme 1 Synthesis scheme of title compounds. | |
All title compounds are ionic 0-D structures. The asymmetric unit of (Tp)Br consists of a protonated thiomorpholine cation and a bromide anion (Fig. S1a and b†). The asymmetric unit of monometallic halides consists of three protonated thiomorpholine cations and an octahedral [MX6]3− unit (X = Cl or Br, M = Sb or Bi) (Fig. 1a and b). The asymmetric unit of heterometallic halides has a complex cation [(Tp)3CuX]3+ and an octahedral anionic unit [MX6]3− (Fig. 1d and e). Detailed structural descriptions are presented in ESI.†
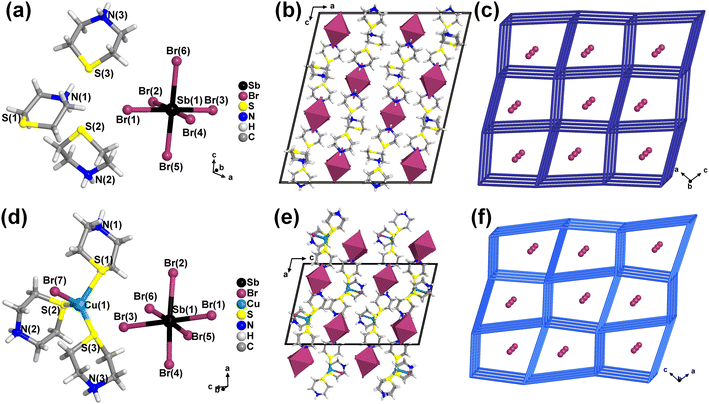 |
| Fig. 1 Single-crystal molecular structural diagrams of (Tp)3SbBr6 (a) and [(Tp)3CuBr]SbBr6 (d). Packing diagrams of (Tp)3SbBr6 (b) and [(Tp)3CuBr]SbBr6 (e) viewed along the b axis. Topological nets of pcu type for (Tp)3SbBr6 (c) and [(Tp)3CuBr]SbBr6 (f), in which the reddish-purple ball represents [SbBr6]3− and the dark blue balls represents three (Tp)+ moieties and the light blue ball represents the [(Tp)3CuX]3+. | |
Comparing the structures of monometallic and heterometallic halides using (Tp)3SbBr6 and [(Tp)3CuBr]SbBr6 as examples, it is found that their anionic unit [SbBr6]3− is arranged in a similar manner in the packing structure (Fig. 1a–f). However, the coordination of CuBr reduces the shortest distance of organic components from 10.03 Å in (Tp)3SbBr6 to 7.21 Å in [(Tp)3CuBr]SbBr6. By contrast, the shortest distance between the anionic [SbBr6]3− units in [(Tp)3CuBr]SbBr6 increases by 0.21–0.78 Å compared to that in (Tp)3SbBr6 (Fig. S2a–f†). More molecular structural diagrams and structure packing diagrams for each compound are given in Fig. S3–S5.† The bond lengths of Bi–X and Sb–X in all compounds are comparable to those previously reported for antimony(III) and bismuth(III) halides.44–48 In addition, the degree of distortion of the anionic octahedra of all compounds at 100 and 297 K is evaluated using eqn (S1) and (S2),† and their σ2 and Δd values are listed in Table S5.† All of the experimental powder X-ray diffraction (PXRD) patterns for all compounds match well with the corresponding simulated ones (Fig. S6a–d and S7a–d†), indicating that the synthesized compounds are high-purity phases.
The PXRD patterns (Fig. S8a–d†) for the powder samples of [(Tp)3CuCl]SbCl6 and [(Tp)3CuCl]BiCl6 after storing at ambient condition for 6 months and [(Tp)3CuBr]SbBr6 and [(Tp)3CuBr]BiBr6 after storing at ambient condition 12 months are consistent with that of corresponding as-synthesized samples, indicating their good stability. The high stability of four heterometallic halides can be attributed to their abundant hydrogen bonding in the structures.12,49 The thermal stability of all compounds was also investigated through thermogravimetric (TG) analysis. Compounds [(Tp)3CuCl]SbCl6, [(Tp)3CuCl]BiCl6, and (Tp)3BiCl6 have no weight loss until around 225 °C (Fig. S9a and S9c†). Compounds [(Tp)3CuBr]SbBr6 and (Tp)3SbBr6 have no weight loss until around 250 °C (Fig. S9b and S9d†). Similarly, compounds [(Tp)3CuBr]BiBr6 and (Tp)3BiBr6 have no weight loss until around 280 °C (Fig. S9b and S9d†). This is consistent with that of other reported 0D metal halides with good thermal stability.30,50,51 Based on the above characterizations, the title compounds have excellent stability.
Optical absorption and DFT calculation
The [(Tp)3CuCl]SbCl6 and [(Tp)3CuCl]BiCl6 are colorless and transparent under ambient light, while [(Tp)3CuBr]SbBr6 and [(Tp)3CuBr]BiBr6 show a similar yellowish transparency. (Tp)3SbBr6 and (Tp)Br are yellowish transparent and compounds (Tp)3BiCl6 and (Tp)3BiBr6 are light-pink transparent, which is consistent with their optical absorption spectra shown in Fig. S10a–d and S11a–d.† The optical absorption edges for [(Tp)3CuCl]SbCl6, [(Tp)3CuCl]BiCl6, [(Tp)3CuBr]SbBr6, and [(Tp)3CuBr]BiBr6 are 385, 380, 450, and 440 nm, respectively (Fig. S10a–d†). The optical absorption edges for (Tp)3BiCl6, (Tp)3BiBr6, (Tp)3SbBr6, and (Tp)Br are 373, 422, 425, and 426 nm, respectively (Fig. S11a–d†).
To further understand the effect of organic components on the bandgap transition and their electronic structure, DFT calculations were performed for [(Tp)3CuCl]SbCl6, [(Tp)3CuBr]SbBr6, and (Tp)3SbBr6. The calculated band gaps for [(Tp)3CuCl]SbCl6, [(Tp)3CuBr]SbBr6, and (Tp)3SbBr6 are 3.01 eV, 2.71 eV, and 2.94 eV, respectively, all with an indirect band gap (Fig. S12a–c†). Their DFT calculated band gap results show that the introduction of CuBr narrows the band gap of [(Tp)3CuBr]SbBr6, which is comparable to the experimental absorption edge. The density of states (DOSs) for heterometallic halides [(Tp)3CuCl]SbCl6 and [(Tp)3CuBr]SbBr6 were analysed (Fig. 2a and d). The valence-band maximum (VBM) of [(Tp)3CuCl]SbCl6 and [(Tp)3CuBr]SbBr6 is mainly contributed by Cu d electrons, halogen p electrons and protonated thiomorpholine (Fig. 2b and e), while the conduction-band minimum (CBM) is dominated by Sb p electrons, halogen p electrons and protonated thiomorpholine (Fig. 2c and f). The DOSs of the monometallic bromide (Tp)3SbBr6 (Fig. 2g) reveal that the VBM is mainly contributed by Sb s electrons, Br p electrons, and protonated thiomorpholine, whereas the CBM is mainly contributed by Sb p electrons and Br p electrons (Fig. 2h and i).
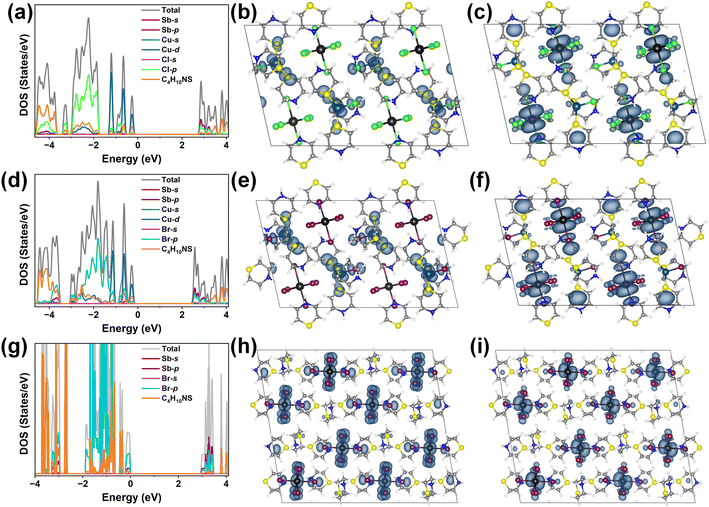 |
| Fig. 2 Density of states (DOSs) for [(Tp)3CuCl]SbCl6 (a), [(Tp)3CuBr]SbBr6 (d), and (Tp)3SbBr6 (g). The C4H10NS refers to the protonated thiomorpholine. The valence-band maximum (VBM) diagram of [(Tp)3CuCl]SbCl6 (b), [(Tp)3CuBr]SbBr6 (e), and (Tp)3SbBr6 (h). The conduction-band minimum (CBM) diagram of [(Tp)3CuCl]SbCl6 (c), [(Tp)3CuBr]SbBr6 (f), and (Tp)3SbBr6 (i). The round-like electronic cloud represents the s-electrons, the spindle-like electronic cloud represents the p-electrons, and the four-dumbbell-shaped electronic cloud represent the d-electrons. | |
Photoluminescence
The PL spectra, the photoluminescence excitation (PLE) spectra, and time-resolved PL spectra have been performed for all compounds. As shown in Fig. 3a, the PLE bands of [(Tp)3CuCl]SbCl6 around 365 nm can be attributed to the triplet state transition of Sb3+.52–56 [(Tp)3CuCl]SbCl6 shows orange-yellow emission at ambient temperature and orange-red emission at 80 K (Fig. 3a). At 300 K, [(Tp)3CuCl]SbCl6 has a maximum emission peak at 590 nm, and a large Stokes shift of 225 nm with full width at half maximum (FWHM) of 133 nm (Fig. S13a†). At 80 K, the maximum peak of [(Tp)3CuCl]SbCl6 emission is 615 nm with FWHM of 95 nm and a large Stokes shift of 250 nm (Fig. 3a). Notably, upon cooling to 80 K, the emission peaks show a variable redshift of 25 nm compared to that at room temperature. The PL lifetimes of the emission peaks of [(Tp)3CuCl]SbCl6 at 590 nm was 15.57 ns at 300 K, and that of [(Tp)3CuCl]SbCl6 at 615 nm was 4.21 μs at 80 K (Fig. S13b and c†), which suggested that the orange-yellow emission may originate from a partially permissive 3P1 → 1S0 transition of the [SbCl6]3− units.44,46,56 The thermal quenching effect should be the reason of the decreased PL lifetime along with the increased temperature.44 It is noteworthy that the isomeric heterometallic bromide [(Tp)3CuBr]SbBr6 exhibits distinct luminescence at different temperatures. [(Tp)3CuBr]SbBr6 emits an orange light at room temperature, which changes to a red light as the temperature decreases to about 160 K, and to a yellow-green light when the temperature reaches 80 K (Fig. 3b and g). At 300 K, the maximum emission peak of [(Tp)3CuBr]SbBr6 is at 625 nm under 380 nm and 430 nm excitation (Fig. S14a and b†). At 80 K, under 380 nm or 355 nm excitation, the maximum emission peak of [(Tp)3CuBr]SbBr6 is at 650 nm with a shoulder peak at 545 nm (Fig. 3b). Interestingly, the excitation spectra of the two emission peaks (650 nm and 545 nm) are different, suggesting that the origin of the two emission peaks is quite different. Different excitation wavelengths and temperatures result in different intensity ratios of the main and shoulder peaks.
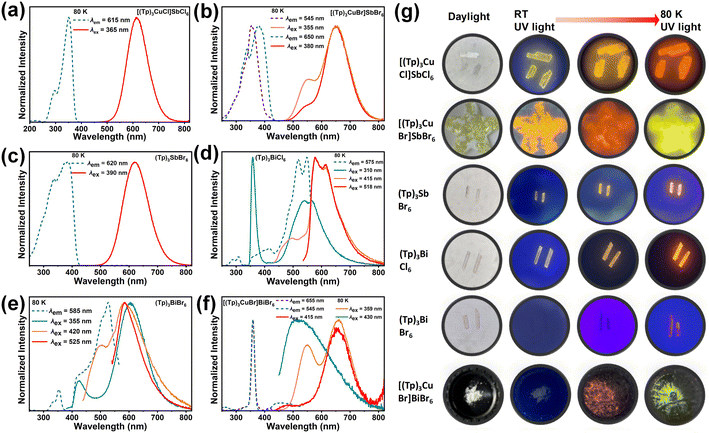 |
| Fig. 3 The PLE and PL spectra for [(Tp)3CuCl]SbCl6 (a), [(Tp)3CuBr]SbBr6 (b), (Tp)3SbBr6 (c), (Tp)3BiCl6 (d), (Tp)3BiBr6 (e), and [(Tp)3CuBr]BiBr6 (f). (g) Luminescence of six title compounds under 365 nm UV lamp at room temperature and low temperature. | |
To further determine the origin of the red and green emissions in [(Tp)3CuBr]SbBr6, the PL spectra of the monometallic halide (Tp)3SbBr6 and the organic bromide salt (Tp)Br are tested. At 300 K, the highest emission peak of (Tp)3SbBr6 is at 610 nm (Fig. S15a†), whereas at 80 K, the highest emission peak of (Tp)3SbBr6 is slightly red-shifted to 620 nm (Fig. 3c). At 300 K, (Tp)Br exhibits broadband emission with a maximum peak of 575 nm at 355 nm excitation and 545 nm at 450 nm excitation (Fig. S16a†). Comparison of the luminescence behavior of compounds [(Tp)3CuBr]SbBr6, [(Tp)3CuCl]SbCl6, and (Tp)3SbBr6 shows that the source of their emission around ∼620 nm can be attributed to the 3P1 → 1S0 transition of the anionic octahedral [SbX6]3− unit.55–58 and the difference in the positions of their emission peaks may be due to the different halogens in the anionic octahedral [SbX6]3− unit.59 Based on the structural features and luminescent properties of the complex cation, it is suggested that the 545 nm shoulder PL peak of [(Tp)3CuBr]SbBr6 should come from the metal–halide-to-ligand charge transfer [(M + X)LCT] of the complex cation,39,60–62 which is in good agreement with the results of DFT calculation (Fig. 2d–f).
The PL properties of Bi-based halides have been studied as well. There are three bands around 310 nm, 415 nm, and 518 nm in the PLE of (Tp)3BiCl6 at 80 K (Fig. 3d). Compared with the PLE of (Tp)Br (Fig. S16a†), we can infer that the major contribution of (Tp)3BiCl6 PLE should be from the organic component. Under the excitation of these three PLE bands, there are four emission bands in the PL spectra, that is, a narrow peak at 355 nm, a shoulder band around 490 nm, and two broad peaks around 575 and 615 nm (Fig. 3d). The PL band around 575 nm is mirror-symmetric with PLE band around 518 nm, which is similar with the PLE of (Tp)Br (Fig. S16a†). Thus, the two broad emission bands (i.e., 575 nm and 615 nm) should originate from the organic cation. The 490 nm shoulder peak should be from the Bi3+ ion, which is consistent with the previous reports.20,44,51 The sharp peak at 355 nm should have originated from the free exciton emission,44 whose absorption can be observed in the PLE spectrum of [(Tp)3CuBr]BiBr6 (Fig. 3e). The PL and PLE of (Tp)3BiBr6 at 80 K are similar to those of (Tp)3BiCl6 as shown in Fig. 3e. There are three emission bands around 420, 495, and 585 nm under the excitation wavelengths of 355, 420, and 525 nm. The sharp peak around 420 nm should be from the free exciton emission.51 The shoulder peak around 495 nm is from the Bi3+ ion. The emission band around 585 nm is from the organic component.
The Bi-based heterometallic halides [(Tp)3CuCl]BiCl6 and [(Tp)3CuBr]BiBr6 are non-luminous at room temperature. Even more, [(Tp)3CuCl]BiCl6 is non-luminescent at 80 K. Fig. 3f shows that compound [(Tp)3CuBr]BiBr6 emits yellow-green double emission at 80 K. Under excitation at 359 nm, the highest emission peak of [(Tp)3CuBr]BiBr6 is at 655 nm with a shoulder peak at 545 nm. Under the excitation at 415 nm, [(Tp)3CuBr]BiBr6 has the maximum emission peak at 655 nm and the shoulder peak at 470 nm (Fig. 3f and S17a†). The shoulder peak 470 nm might be from the Bi3+ ion. Under the excitation at 430 nm, the broadband emission peak of [(Tp)3CuBr]BiBr6 is around 525 nm, which is very similar to that of (Tp)Br at 545 nm (Fig. 3f and S16a†). Thus, the peaks around 545 nm should be from the organic component. However, It is hard to explain the peak of 655 nm in [(Tp)3CuBr]BiBr6. In our opinion, the 655 nm peak should be from the organic component, as the same as the 615 nm peak of (Tp)3BiCl6. Typically, 0-D Bi-based bromides exhibit blue emission around 460 nm.20,51 However, (C5N2H14)2[BiBr6]Br·H2O (C5N2H14 = double-protonated homopiperazine) has a red emission (650 nm) at 77 K, which was ascribed to the triplet emission of the [BiBr6]3− anion.45
In order to gain insight into the luminescence mechanism, we have performed the temperature-dependent emission spectroscopy of [(Tp)3CuCl]SbCl6, [(Tp)3CuBr]SbBr6, and [(Tp)3CuBr]BiBr6. As shown in Fig. 4a and b, the intensity of the emission (around 620 nm) of [(Tp)3CuCl]SbCl6 in the temperature range of 80 to 140 K increases gradually with the increase of temperature, and then decreases with the increase of temperature from 140 K to 320 K (Fig. 4a and b). The temperature-dependent PL behavior of [(Tp)3CuCl]SbCl6 can be attributed to thermally activated delayed fluorescence (TADF).61–63 The emission color changes from orange-yellow to orange-red during the whole process (Fig. S18a†). The redshift of the emission peak from 590 nm to 615 nm and the shrinkage of the FWHM from 133 nm to 95 nm with decreasing temperature can be the result of the suppressed thermal expansion and electron–phonon interaction, respectively.56,64 Since the Stokes shift is different for [(Tp)3CuCl]SbCl6 at low temperature (250 nm) and room temperature (225 nm), the distortion of the anionic octahedral [SbCl6]3− at 100 and 297 K is evaluated using eqn (S1) and (S2)† with σ2 of 14.70 and 11.75, Δd of 25.79 and 21.63, respectively (Table S5†). At low temperatures, the greater distortion of the [SbCl6]3− anion in [(Tp)3CuCl]SbCl6 leads to a larger recombination distortion of [SbCl6]3− in the excited state, which results in a smaller radiative transition energy and ultimately in a large Stokes shift.46,65,66
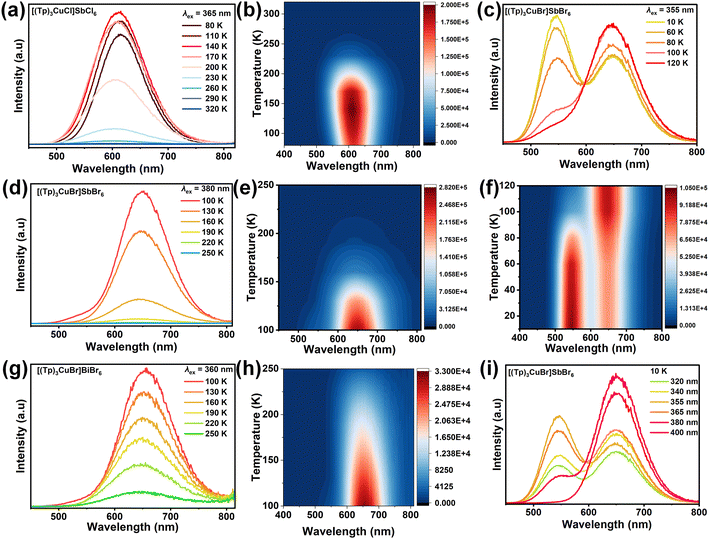 |
| Fig. 4 (a) Temperature-dependent PL spectra of [(Tp)3CuCl]SbCl6 in the temperature range of 80–320 K. (b) Temperature-dependent PL spectra contour map of [(Tp)3CuCl]SbCl6 from 80–320 K. (c) Temperature-dependent PL spectra of [(Tp)3CuBr]SbBr6 from 10–120 K. (d) Temperature-dependent PL spectra of [(Tp)3CuBr]SbBr6 from 100–250 K. (e) Temperature-dependent PL spectra contour map of [(Tp)3CuBr]SbBr6 from 100–250 K. (f) Temperature-dependent PL spectra contour map of [(Tp)3CuBr]SbBr6 from 10–120 K. (g) Temperature-dependent PL spectra of [(Tp)3CuBr]BiBr6 from 100–250 K. (h) Temperature-dependent PL spectra contour map of [(Tp)3CuBr]BiBr6 from 100–250 K. (i) Excitation-dependent PL spectra of [(Tp)3CuBr]SbBr6 at 10 K. | |
The electron–phonon coupling interaction can be evaluated by the Huang–Rhys factor (S) and electron–optical phonon coupling energy (Γop) from eqn (S3) and (S4),† respectively. The temperature-dependent spectral fitting of compound [(Tp)3CuCl]SbCl6 shows S and Γop values of 45.87 and 129 meV, respectively (Fig. S18b and c†). The S value is higher than that of previously reported metal halides, such as [Na(DMSO)2]3SbBr3Cl3 (10.7) and (PPZ)2SbCl7·5H2O (28.0), which indicates that single crystals of [(Tp)3CuCl]SbCl6 exhibit strong electron–phonon coupling.55,64,67 The Arrhenius formula (eqn (S5)†) is used to estimate the activation energy (Ea) for nonradiative recombination. The fitting results indicate that Ea is 253.87 meV (Fig. S18d†). The temperature-dependent PL analysis further suggests the soft lattice and strong electron–phonon coupling in [(Tp)3CuCl]SbCl6.
To investigate the emission phenomena of compound [(Tp)3CuBr]SbBr6, we conducted temperature-dependent spectra of [(Tp)3CuBr]SbBr6 from 100–250 K under the excitation of 380 nm. The emission peak of [(Tp)3CuBr]SbBr6 red shifts from 625 nm to 650 nm with increased intensity and the FWHM narrows from 144 nm to 102 nm, when the temperature decreases from 250 to 100 K (Fig. 4d, e, and S19a†). Similarly, the temperature-dependent PL spectra of [(Tp)3CuBr]BiBr6 from 250 to 100 K shows that the highest emission peak of [(Tp)3CuBr]BiBr6 is slightly red-shifted from 644 nm to 655 nm with improved intensity, and the FWHM narrows from 139 nm to 109 nm (Fig. 4g, h, and S19b†). The temperature-dependent PL behaviors can be attributed to the suppressed thermal expansion and electron–phonon interaction at low temperatures.56,64 Their Stokes shifts are enlarged along with the decreased temperature, which should be related to the enhanced distortion (Table S5†).
The temperature-dependent dual emission of [(Tp)3CuBr]SbBr6 is further carried out in the range of 10–120 K under 355 nm excitation. Under 355 nm excitation, the green emission peak at 545 nm gradually decreases, while the red emission peak at 650 nm gradually increases as the temperature increases from 10 K to 120 K (Fig. 4c, f, and S20a†). Different dual peak shapes should be due to the varied energy transfer between cation and anion. At 10 K, the double peaks from the cation and [SbBr6]3− exist simultaneously due to their inhibited energy transfer at low temperatures. At 100 K, the emission peaks of the cations begin to disappear and only red emission of [SbBr6]3− is shown (Fig. 4m). This is due to the activation of energy transfer from the complexing cation to [SbBr6]3−. The possibility of energy transfer is supported by the DOS result of [(Tp)3CuBr]SbBr6 (Fig. 2d). The excitation-dependent spectra of [(Tp)3CuBr]SbBr6 at 10 K show that the green light at 545 nm and the red emission at 650 nm coexist at the excitation wavelength from 320 to 400 nm, while it only displays red emission at the excitation wavelength above 400 nm (Fig. 4i and S19c†). Thus, the lower temperature and shorter excitation wavelength could enhance the emission of [(Tp)3CuBr]3+, thus showing fluorescence emission with dual emission centers (Fig. 4f and i).
The pronounced change in the emission color of [(Tp)3CuBr]SbBr6 with temperature is further used for optical thermometry in the temperature range of 10–120 K (Fig. S20a–c and S19a†). The fluorescence intensity ratio (FIR) of the emission peaks at 545 nm and 650 nm of [(Tp)3CuBr]SbBr6 in the range of 10–120 K was used as an assessment of the sensitivity. The FIR (I620/I545) as a function of temperature was fitted by eqn (1)
68,69 to obtain ΔET:
As important indicators, absolute sensitivity (Sa) and relative sensitivity (Sr) were calculated using the following eqn (2) and (3) to evaluate the properties of the luminescent thermometer.23,68–70
| Sa = Ce−ΔET/kBT × ΔET/kBT2 | (2) |
|  | (3) |
where
B and
C are constants, Δ
ET is the energy difference between two coupled levels,
kB is the Boltzmann constant, and
T is the temperature. The energy gap of Δ
ET is calculated to be 29.66 meV (Fig. S21a
†). At 80 K, the values of
Sa and
Sr calculated from
eqn (2) and (3) are 0.078 K
−1 and 5.38% K
−1, respectively, which are comparable to those of other reported multicolor luminescence-based materials.
23,68,71 It is noteworthy that the emission color of [(Tp)
3CuBr]SbBr
6 changes from yellow-green to red as the temperature rises between 10–120 K (Fig. S20a
†), and then gradually changes from red to orange-red as the temperature increases to 300 K, with the intensity of the light gradually becoming weaker (Fig. S21c
†). This distinct color change can be a useful visual indicator of the temperature change of a substance, and the temperature dependence of the emission color of [(Tp)
3CuBr]SbBr
6 allows optical thermometry and thermochromism at low-temperature in the range 10–120 K (Fig. S20a–c
†).
Conclusions
In summary, we have synthesized three 0-D monometallic halides (Tp)3MX6 using protonated thiomorpholine as the cationic ligand and have successfully synthesized four novel 0-D copper–antimony/bismuth heterometallic halides [(Tp)3CuX]MX6, taking into account that Cu has a better coordination capacity with S atoms. Their crystal structures, thermal stability, and photophysical properties have been investigated. DFT calculations reveal that in monometallic halides the electronic states are highly localized in the anionic octahedral unit, whereas in heterometallic halides charge transfer occurs between the complexing cations and the anionic octahedral unit. The PL data of [(Tp)3CuCl]SbCl6, [(Tp)3CuBr]SbBr6, and (Tp)3SbBr6 show that the PL mechanism of their broadband orange-red emission around 620 nm can be attributed to the 3P1 → 1S0 transition of the anionic octahedral unit. Comparison of heterometallic halides [(Tp)3CuBr]SbBr6 and [(Tp)3CuBr]BiBr6 with the monometallic halides (Tp)3SbBr6, (Tp)3BiCl6, and (Tp)3BiBr6, and (Tp)Br verifies that their green light at 545 nm is attributable to the complex cation [(Tp)3CuBr]3+. Based on the PL characteristics of [(Tp)3CuBr]SbBr6, which changes from yellow-green to red and then to orange-red light with temperature, it can be considered that it has the potential to be used as a low-temperature sensor. To the best of our knowledge, this is the first report on the in-depth study of the relationship of structure and photoluminescence properties for 0-D copper–antimony/bismuth heterometallic halides. This work provides a new strategy for designing 0-D heterometallic halides with low-cost and multiple luminescence centers.
Author contributions
A. A. and X. Y. H. conceived the project. A. A. designed and performed most of the experiments. A. A. performed DFT calculations and analyzed the data. A. A. performed the PL, PLE, PL decay, TG, UV, and XRD experiments. S. M. Z. offered help in the synthesis of compounds. G. Y. C. and J. H. L. offered help with crystal photographs and UV testing. A. A. prepared the manuscript. A. A., H. W. L., Z. P. W., K. Z. D. and X. Y. H. revised the manuscript. All the authors discussed the results and commented on the manuscript. X. Y. H. supervised the project.
Data availability
CCDC no. 2365627–2365634 and 2391052–2391054 contains the supplementary crystallographic data for this paper.
Conflicts of interest
There are no conflicts to declare.
Acknowledgements
This work was supported by the National Natural Science Foundation of China (No. 92261115, 22205236, and 22373014) and the Natural Science Foundation of Fujian Province (No. 2022J05089).
References
- Z. C. Hu, B. J. Deibert and J. Li, Luminescent Metal-Organic Frameworks for Chemical Sensing and Explosive Detection, Chem. Soc. Rev., 2014, 43, 5815–5840 RSC.
- T. T. Xuan and R. J. Xie, Recent Processes on Light-Emitting Lead-Free Metal Halide Perovskites, Chem. Eng. J., 2020, 393, 124757 CrossRef CAS.
- J. C. Jin, N. N. Shen, Z. P. Wang, Y. C. Peng and X. Y. Huang, Photoluminescent Ionic Metal Halides Based on s2 Typed Ions and Aprotic Ionic Liquid Cations, Coord. Chem. Rev., 2021, 448, 214185 CrossRef CAS.
- M. Z. Li and Z. G. Xia, Recent Progress of Zero-Dimensional Luminescent Metal Halides, Chem. Soc. Rev., 2021, 50, 2626–2662 RSC.
- J. W. Lin, X. X. Lu, Z. Liu, Y. P. Lin, J. R. Yang, Y. A. Ge, C. Zhou, X. Z. Wang, J. R. Li, K. Z. Du, F. Tang and X. Y. Huang, Optical Property Regulation through Host-Guest Interaction in a Zero-Dimensional Zr Chloride, Chem. Mater., 2024, 36, 4600–4606 CrossRef CAS.
- Y.-P. Lin, X. Lu, Z. Zhang, X. Qi, J. Jin, J. Xu, Y. Wu, Y. Wu, Z. Deng, X.-Y. Huang, C. Han, S. Hu and K.-Z. Du, Organic Hybrid Tetranuclear Clusteroluminogens: Blue-Light-Excitable LED with Ultrahigh Luminous Efficacy, Chem. Eng. J., 2024, 479, 147523 CrossRef CAS.
- Y. Zhong, S.-P. Liu, Y.-P. Lin, X.-H. Qi, B. Yang, Q. Zhang and K.-Z. Du, Multi-Mode Photoluminescence Regulation in a Zero-Dimensional Organic-Inorganic Hybrid Metal Halide Perovskite—[(CH3)4N]2SnCl6, Inorg. Chem., 2023, 62, 14422–14430 CrossRef CAS PubMed.
- K. Zhu, Z. K. Cheng, S. Rangan, M. Cotlet, J. B. Du, L. Kasaei, S. J. Teat, W. Liu, Y. F. Chen, L. C. Feldman, D. M. O'Carroll and J. Li, A New Type of Hybrid Copper Iodide as Nontoxic and Ultrastable LED Emissive Layer Material, ACS Energy Lett., 2021, 6, 2565–2574 CrossRef CAS.
- T. H. Chen, Y. J. Ma and D. P. Yan, Single-Component 0D Metal-Organic Halides with Color-Variable Long-Afterglow toward Multi-Level Information Security and White-Light LED, Adv. Funct. Mater., 2023, 33, 2214962 CrossRef CAS.
- Z. Y. Quan, Q. Zhang, H. J. Li, S. G. Sun and Y. Q. Xu, Fluorescent Cellulose-based Materials for Information Encryption and Anti-Counterfeiting, Coord. Chem. Rev., 2023, 493, 215287 CrossRef CAS.
- Y. R. Shi, S. Y. Zhao, Y. Zhou and Z. G. Zang, Variable halide perovskites: diversification of anti-counterfeiting applications, Mater. Chem. Front., 2023, 7, 6085–6106 RSC.
- Q. Q. He, C. K. Zhou, L. J. Xu, S. J. Lee, X. S. Lin, J. Neu, M. Worku, M. Chaaban and B. W. Ma, Highly Stable Organic Antimony Halide Crystals for X-ray Scintillation, ACS Mater. Lett., 2020, 2, 633–638 CrossRef CAS.
- B. Q. Wang, X. Yang, S. Chen, S. R. Lu, S. Y. Zhao, Q. K. Qian, W. S. Cai, S. H. Wang and Z. G. Zang, Flexible Perovskite Scintillators and Detectors for X-ray Detection, iScience, 2022, 25, 105593 CrossRef CAS PubMed.
- J. X. Wang, O. M. Bakr and O. F. Mohammed, Transparent X-ray imaging scintillators, Matter, 2022, 5, 2547–2549 CrossRef CAS.
- T. V. Sedakova and A. G. Mirochnik, Luminescent and Thermochromic Properties of Tellurium(IV) Halide Complexes with Cesium, Opt. Spectrosc., 2016, 120, 268–273 CrossRef CAS.
- B. Su, S. Geng, Z. Xiao and Z. Xia, Highly Distorted Antimony(III) Chloride [Sb2Cl8]2− Dimers for Near-Infrared Luminescence up to 1070 nm, Angew. Chem., Int. Ed., 2022, 61, e202208881 CrossRef CAS PubMed.
- K. M. McCall, V. Morad, B. M. Benin and M. V. Kovalenko, Efficient Lone-Pair-Driven Luminescence: Structure–Property Relationships in Emissive 5s2 Metal Halides, ACS Mater. Lett., 2020, 2, 1218–1232 CrossRef CAS PubMed.
- V. Morad, S. Yakunin, B. M. Benin, Y. Shynkarenko, M. J. Grotevent, I. Shorubalko, S. C. Boehme and M. V. Kovalenko, Hybrid 0D Antimony Halides as Air-Stable Luminophores for High-Spatial-Resolution Remote Thermography, Adv. Mater., 2021, 33, 2007355 CrossRef CAS PubMed.
- B. Li, J. Jin, M. Yin, X. Zhang, M. S. Molokeev, Z. Xia and Y. Xu, Sequential and Reversible Phase Transformations in Zero-Dimensional Organic-Inorganic Hybrid Sb-based Halides towards Multiple Emissions, Angew. Chem., Int. Ed., 2022, 61, e202212741 CrossRef CAS PubMed.
- Y. Wei, W. Wang, Z. Wang, H. Yang, X. You, Y. Zhao, P. Dang, H. Lian, J. Hao, G. Li and J. Lin, Recent Progress of Bismuth Effect on All-Inorganic Lead-Free Metal Halide Derivatives: Crystals Structure, Luminescence Properties, and Applications, Adv. Funct. Mater., 2022, 33, 2205829 CrossRef.
- C. Zhou, S. Lee, H. Lin, J. Neu, M. Chaaban, L.-J. Xu, A. Arcidiacono, Q. He, M. Worku, L. Ledbetter, X. Lin, J. A. Schlueter, T. Siegrist and B. Ma, Bulk Assembly of Multicomponent Zero-Dimensional Metal Halides with Dual Emission, ACS Mater. Lett., 2020, 2, 376–380 CrossRef CAS.
- D. X. Ju, M. Zhou, Z. C. Liu, P. Ran, Z. W. Dong, S. Hou, H. Li, W. G. Xiao, X. H. Xu, H. F. Li, Y. Yang and T. M. Jiang, Excitation-Selective and Double-Emissive Lead-Free Binary Hybrid Metal Halides for White Light-Emitting Diode and X-Ray Scintillation, Small, 2023, 2305083 Search PubMed.
- J. H. Marayathungal, N. Puthuparambil, D. Das, M. Kalyani, R. Bakthavatsalam and J. Kundu, Bulk Coassembly of Zero-Dimensional Heterometallic Halide Hybrids for Broadband White Light Emission and Optical Thermometry, J. Phys. Chem. C, 2023, 127, 18474–18484 CrossRef CAS.
- C. Zhu, J. B. Jin, Z. Wang, Z. P. Xu, M. C. Folgueras, Y. X. Jiang, C. B. Uzundal, H. K. D. Le, F. Wang, X. Y. Zheng and P. D. Yang, Supramolecular assembly of blue and green halide perovskites with near-unity photoluminescence, Science, 2024, 383, 86–93 CrossRef CAS PubMed.
- C. Zhou, H. Lin, J. Neu, Y. Zhou, M. Chaaban, S. Lee, M. Worku, B. Chen, R. Clark, W. Cheng, J. Guan, P. Djurovich, D. Zhang, X. Lu, J. Bullock, C. Pak, M. Shatruk, M.-H. Du, T. Siegrist and B. Ma, Green Emitting Single-Crystalline Bulk Assembly of Metal Halide Clusters with Near-Unity Photoluminescence Quantum Efficiency, ACS Energy Lett., 2019, 4, 1579–1583 CrossRef CAS.
- M. Li, Y. Li, M. S. Molokeev, J. Zhao, G. Na, L. Zhang and Z. Xia, Halogen Substitution in Zero-Dimensional Mixed Metal Halides toward Photoluminescence Modulation and Enhanced Quantum Yield, Adv. Opt. Mater., 2020, 8, 2000418 CrossRef CAS.
- C. Zhou, L. J. Xu, S. Lee, H. Lin and B. Ma, Recent Advances in Luminescent Zero-Dimensional Organic Metal Halide Hybrids, Adv. Opt. Mater., 2020, 9, 2001766 CrossRef.
- M. Z. Li, J. Zhou, G. J. Zhou, M. S. Molokeev, J. Zhao, V. Morad, M. V. Kovalenko and Z. G. Xia, Hybrid Metal Halides with Multiple Photoluminescence Centers, Angew. Chem., Int. Ed., 2019, 58, 18670–18675 CrossRef CAS PubMed.
- Q. Li, Z. W. Chen, M. Z. Li, B. Xu, J. Han, Z. S. Luo, L. Tan, Z. G. Xia and Z. W. Quan, Pressure-Engineered Photoluminescence Tuning in Zero-Dimensional Lead Bromide Trimer Clusters, Angew. Chem., Int. Ed., 2021, 60, 2583–2587 CrossRef CAS PubMed.
- L. M. Zhang, Z. S. Luo, Y. Wei, W. Wang, Y. L. Liu, C. Li, X. He and Z. W. Quan, Zero-Dimensional Hybrid Binuclear Manganese Chloride with Thermally Stable Yellow Emission, Chem. Commun., 2022, 58, 6926–6929 RSC.
- A. V. Artem'ev, M. P. Davydova, A. S. Berezin, D. G. Samsonenko, I. Y. Bagryanskaya, V. K. Brel, X. Hei, K. A. Brylev, O. I. Artyushin, L. E. Zelenkov, I. I. Shishkin and J. Li, New Approach toward Dual-Emissive Organic–Inorganic Hybrids by Integrating Mn(II) and Cu(I) Emission Centers in Ionic Crystals, ACS Appl. Mater. Interfaces, 2022, 14, 31000–31009 CrossRef PubMed.
- L. Lian, P. Zhang, G. Liang, S. Wang, X. Wang, Y. Wang, X. Zhang, J. Gao, D. Zhang, L. Gao, H. Song, R. Chen, X. Lan, W. Liang, G. Niu, J. Tang and J. Zhang, Efficient Dual-Band White-Light Emission with High Color Rendering from Zero-Dimensional Organic Copper Iodide, ACS Appl. Mater. Interfaces, 2021, 13, 22749–22756 CrossRef CAS PubMed.
- A. M. Wheaton, M. E. Streep, C. M. Ohlhaver, A. D. Nicholas, F. H. Barnes, H. H. Patterson and R. D. Pike, Alkyl Pyridinium Iodocuprate(I) Clusters: Structural Types and Charge Transfer Behavior, ACS Omega, 2018, 3, 15281–15292 CrossRef CAS PubMed.
- A. V. Artem'ev, E. P. Doronina, M. I. Rakhmanova, X. Hei, D. V. Stass, O. G. A. Tarasova, I. Y. Bagryanskaya, D. G. Samsonenko, A. S. Novikov, N. A. Nedolya and J. Li, A family of CuI-based 1D polymers showing colorful short-lived TADF and phosphorescence induced by photo- and X-ray irradiation, Dalton Trans., 2023, 52, 4017–4027 RSC.
- A. S. Berezin, D. G. Samsonenko, V. K. Brel and A. V. Artem'ev, “Two-in-one” organic–inorganic hybrid MnII complexes exhibiting dual-emissive phosphorescence, Dalton Trans., 2018, 47, 7306–7315 RSC.
- A. V. Artem'ev, A. S. Berezin, I. V. Taidakov and I. Y. Bagryanskaya, Synthesis of dual emitting iodocuprates: can solvents switch the reaction outcome?, Inorg. Chem. Front., 2020, 7, 2195–2203 RSC.
- M. Li, M. S. Molokeev, J. Zhao and Z. Xia, Optical Functional Units in Zero-Dimensional Metal Halides as a Paradigm of Tunable Photoluminescence and Multicomponent Chromophores, Adv. Opt. Mater., 2020, 8, 1902114 CrossRef CAS.
- L. B. Fan, K. Liu, S. H. He, F. Y. Zhao, J. Zhao, Y. G. Wang and Q. L. Liu, Reversible Mechanically Induced On-Off Photoluminescence in Hybrid Metal Halides, Adv. Funct. Mater., 2022, 32, 2110771 CrossRef CAS.
- W. Liu, Y. Fang and J. Li, Copper Iodide Based Hybrid Phosphors for Energy-Efficient General Lighting Technologies, Adv. Funct. Mater., 2018, 28, 1705593 CrossRef.
- B. B. Su, J. Jin, Y. H. Peng, M. S. Molokeev, X. B. Yang and Z. G. Xia, Zero-Dimensional Organic Copper(I) Iodide Hybrid with High Anti-Water Stability for Blue-Light-Excitable Solid-State Lighting, Adv. Opt. Mater., 2022, 10, 2102619 CrossRef CAS.
- S. Y. Xu, Y. N. Wu, B. C. Chen, P. W. Huang, Z. Z. Zhang, Y. Zhong, Y. Zhao, X. Y. Huang and K. Z. Du, Electrochemical Thin Film Deposition of Copper(I) Halides in Aqueous Solution: Substrate Extension and Structure Transformation, Adv. Mater. Interfaces, 2022, 9, 2102239 CrossRef CAS.
- S. Xu, Y. Hao, X. Xu, L. Huang, Y. Liang, J. Liao, J.-R. Yang, Y. Zhou, M. Huang, K.-Z. Du, C. Zhang and P. Xu, Antitumor Activity and Mechanistic Insights of a Mitochondria-Targeting Cu(I) Complex, J. Med. Chem., 2024, 67, 7911–7920 CrossRef CAS PubMed.
- S.-Y. Xu, J.-R. Yang, Y.-N. Wu, H.-Q. Lin, Y. Chai, J.-W. Lin and K.-Z. Du, Chemical Etching Strategy to Prepare Microtubes of a Hybrid Organic Cuprous Halide, CrystEngComm, 2023, 25, 1053–1057 RSC.
- R. X. He, M. Li, Y. H. Chen, L. Zhou, S. G. Zhou, D. H. You, H. Z. Xiong, Y. H. Hu and Q. L. Chen, Effect of the Host Lattice Environment on the Expression of 5s2 Lone-Pair Electrons in a 0D Bismuth-Based Metal Halide, Inorg. Chem., 2023, 62, 2806–2816 CrossRef PubMed.
- A. V. Bykov, T. A. Shestimerova, M. A. Bykov, K. A. Lyssenko, V. M. Korshunov, M. T. Metlin, I. V. Taydakov and A. V. Shevelkov, Molecular and Supramolecular Structure of a New Luminescent Hybrid Compound: (C5N2H14)2[BiBr6]Br·H2O, Inorganics, 2022, 10, 181 CrossRef CAS.
- D. Chen, F. Dai, S. Hao, G. Zhou, Q. Liu, C. Wolverton, J. Zhao and Z. Xia, Crystal Structure and Luminescence Properties of Lead-free Metal Halides (C6H5CH2NH3)3MBr6 (M = Bi and Sb), J. Mater. Chem. C, 2020, 8, 7322–7329 RSC.
- Q.-L. Li, M. Zhao, R.-J. Hao, J. Wei, X.-X. Wang, C. Yang, M. Zhao, Y.-H. Tan and Y.-Z. Tang, High-Temperature Phase Transition with Switchable Dielectric Behavior and Significant Photoluminescence Changes in a Zero-Dimensional Hybrid SbBr6 Perovskite, Inorg. Chem., 2024, 63, 3411–3417 CrossRef CAS PubMed.
- F. Lin, H. Wang, W. Liu and J. Li, Zero-dimensional Ionic Antimony Halide Inorganic–Organic Hybrid with Strong Greenish Yellow Emission, J. Mater. Chem. C, 2020, 8, 7300–7303 RSC.
- C. Zhou, H. Lin, Q. He, L. Xu, M. Worku, M. Chaaban, S. Lee, X. Shi, M.-H. Du and B. Ma, Low dimensional metal halide perovskites and hybrids, Mater. Sci. Eng., R, 2019, 137, 38–65 CrossRef.
- K. Liu, A. Hou, J. Lin, M. Quan, Y. Xiong, Z. Guo, J. Zhao and Q. Liu, High-Stability Hybrid Antimony Halides for Thermometry in Power System Component or Circuit Monitoring, Adv. Funct. Mater., 2024, 2412529, DOI:10.1002/adfm.202412529.
- R. Zhang, X. Mao, Y. Yang, S. Yang, W. Zhao, T. Wumaier, D. Wei, W. Deng and K. Han, Air-Stable, Lead-Free Zero-Dimensional Mixed Bismuth-Antimony Perovskite Single Crystals with Ultra-broadband Emission, Angew. Chem., Int. Ed., 2019, 58, 2725–2729 CrossRef CAS PubMed.
- H. Nikol and A. Vogler, Photoluminescence of Antimony(III) and Bismuth(III) Complexes in Solution, J. Am. Chem. Soc., 1991, 113, 8988–8990 CrossRef CAS.
- A. Vogler and H. Nikol, Photochemistry and Photophysics of Coordination Compounds of
the Main Group-Metals, Pure Appl. Chem., 1992, 64, 1311–1317 CrossRef CAS.
- A. Vogler and H. Nikol, The Structures of s2 Metal Complexes in the Ground and sp Excited States, Comments Inorg. Chem., 1993, 14, 245–261 CrossRef CAS.
- Y.-C. Peng, S.-H. Zhou, J.-C. Jin, T.-H. Zhuang, L.-K. Gong, H.-W. Lin, Z.-P. Wang, K.-Z. Du and X.-Y. Huang, [PPh3H]2[SbCl5]: A Zero-Dimensional Hybrid Metal Halide with a Supramolecular Framework and Stable Dual-Band Emission, J. Phys. Chem. C, 2022, 126, 17381–17389 CrossRef CAS.
- Y. Zhang, Y. Zhang, Y. Zhao, H. Jia, Z. Yang, B. Yin, Y. Wu, Y. Yi, C. Zhang and J. Yao, Crystal-Liquid-Glass Transition and Near-Unity Photoluminescence Quantum Yield in Low Melting Point Hybrid Metal Halides, J. Am. Chem. Soc., 2023, 145, 12360–12369 CrossRef CAS PubMed.
- G. Zhou, B. Su, J. Huang, Q. Zhang and Z. Xia, Broad-band emission in metal halide perovskites: Mechanism, materials, and applications, Mater. Sci. Eng., R, 2020, 141, 100548 CrossRef.
- J. Jin, Y. Wang, K. Han and Z. Xia, Rigid Phase Formation and Sb3+ Doping of Tin(IV) Halide Hybrids toward Photoluminescence Enhancement and Tuning for Anti-Counterfeiting and Information Encryption, Angew. Chem., Int. Ed., 2024, 63, e202408653 CrossRef CAS PubMed.
- V. Morad, S. Yakunin and M. V. Kovalenko, Supramolecular Approach for Fine-Tuning of the Bright Luminescence from Zero-Dimensional Antimony(III) Halides, ACS Mater. Lett., 2020, 2, 845–852 CrossRef CAS PubMed.
- P. C. Ford, E. Cariati and J. Bourassa, Photoluminescence properties of multinuclear copper(I) compounds, Chem. Rev., 1999, 99, 3625–3647 CrossRef CAS PubMed.
- K. Zhu, Z. K. Cheng, S. Rangan, M. Cotlet, J. B. Du, L. Kasaei, S. J. Teat, W. Liu, Y. F. Chen, L. C. Feldman, D. M. O'Carroll and J. Li, A New Type of Hybrid Copper Iodide as Nontoxic and Ultrastable LED Emissive Layer Material, ACS Energy Lett., 2021, 6, 2565–2574 CrossRef CAS.
- X. M. Liu, Y. Y. Jiang, F. Y. Li, X. L. Xu, R. H. Li, W. J. Zhu, J. F. Ni, C. B. Ding, S. J. Liu and Q. Zhao, Thermally Activated Delayed Fluorescent Scintillators Based on Mononuclear Copper(I) Halide Complexes for High-Resolution X-Ray Imaging, Adv. Opt. Mater., 2023, 11, 2202169 CrossRef CAS.
- H. Yersin, R. Czerwieniec, M. Z. Shafikov and A. F. Suleymanova, TADF Material Design: Photophysical Background and Case Studies Focusing on Cu(I) and Ag(I) Complexes, ChemPhysChem, 2017, 18, 3508–3535 CrossRef CAS PubMed.
- Q. Mo, Q. Qian, Y. Shi, W. Cai, S. Zhao and Z. Zang, High Quantum Efficiency of Stable Sb-Based Perovskite-Like Halides toward White Light Emission and Flexible X-Ray Imaging, Adv. Opt. Mater., 2022, 10, 2201509 CrossRef CAS.
- Y.-C. Peng, Z.-Z. Zhang, Y.-P. Lin, J.-C. Jin, T.-H. Zhuang, L.-K. Gong, Z.-P. Wang, K.-Z. Du and X.-Y. Huang, A Deep-Red-Emission Antimony(III) Chloride with Dual-Cations: Extremely Large Stokes Shift due to High [SbCl6] Distortion, Chem. Commun., 2021, 57, 13784–13787 RSC.
- Q. Wei, T. Chang, R. Zeng, S. Cao, J. Zhao, X. Han, L. Wang and B. Zou, Self-Trapped Exciton Emission in a Zero-Dimensional (TMA)2SbCl5·DMF Single Crystal and Molecular Dynamics Simulation of Structural Stability, J. Phys. Chem. Lett., 2021, 12, 7091–7099 CrossRef CAS PubMed.
- J.-B. Luo, J.-H. Wei, Z.-Z. Zhang and D.-B. Kuang, Water-Molecule-Induced Emission Transformation of Zero-Dimension Antimony-Based Metal Halide, Inorg. Chem., 2021, 61, 338–345 CrossRef PubMed.
- X. Shi, Y. Xue, Q. Mao, L. Pei, X. Li, M. Liu, Q. Zhang and J. Zhong, Eu3+ Single-Doped Phosphor with Antithermal Quenching Behavior and Multicolor-Tunable Properties for Luminescence Thermometry, Inorg. Chem., 2023, 62, 893–903 CrossRef CAS PubMed.
- Y. Gao, F. Huang, H. Lin, J. Zhou, J. Xu and Y. Wang, A Novel Optical Thermometry Strategy Based on Diverse Thermal Response from Two Intervalence Charge Transfer States, Adv. Funct. Mater., 2016, 26, 3139–3145 CrossRef CAS.
- H. Zhang, J. Ye, X. Wang, S. Zhao, R. Lei, L. Huang and S. Xu, Highly reliable all-fiber temperature sensor based on the fluorescence intensity ratio (FIR) technique in Er3+/Yb3+ co-doped NaYF4 phosphors, J. Mater. Chem. C, 2019, 7, 15269–15275 RSC.
- R. Li, G. Wei, Z. Wang, Y. Wang, J. Li, S. He, L. Li, H. Suo, W. Ding and P. Li, Cr3+-Facilitated Ultra-Sensitive Luminescence Ratiometric Thermometry at Cryogenic Temperature, Laser Photonics Rev., 2023, 17, 2200589 CrossRef CAS.
Footnote |
† Electronic supplementary information (ESI) available: Experimental section, crystal structure and bond length analyses, figures for PXRD, TG, and optical properties, and crystallographic data tables. CCDC 2365627–2365634 and 2391052–2391054 for all title compounds. For ESI and crystallographic data in CIF or other electronic format see DOI: https://doi.org/10.1039/d4qi02614d |
|
This journal is © the Partner Organisations 2025 |
Click here to see how this site uses Cookies. View our privacy policy here.