DOI:
10.1039/D4QI01944J
(Research Article)
Inorg. Chem. Front., 2025,
12, 171-178
Glacial acetic acid as a resolution solvent for growing enantiopure crystals from racemic mixtures †
Received
1st August 2024
, Accepted 8th October 2024
First published on 9th October 2024
Abstract
Chirality is pervasive in nature, yet chiral separation continues to be a real challenge. Herein we report the synthesis and structure of [Pd4S2(dppm)3(CNtBu)2][H(OAc)2]2 (abbre. Pd4S2, HOAc = acetic acid), with an intrinsically chiral Pd–S core. The as-prepared clusters are racemates crystallized in the centric space group P21/n or as twins of acentric space groups P43212 and P41212, both with co-crystallized acetic acid molecules. Surprisingly, when re-crystallized from glacial acetic acid, optically pure enantiomeric crystals of P43212 or P41212 were obtained simultaneously, with the same amount in one pot. In this regard, glacial acetic acid functions both as a recrystallization solvent and as a resolution agent. It also effectively eliminates twinning. This unexpected finding suggests an easy and economical way to separate a racemic mixture into enantiomers which may find applications in chiral separation technologies.
Introduction
Chirality (or handedness) is ubiquitous in nature.1,2 The chirality of matter has dramatic consequences in both living and non-living systems, ranging from biology, medicine to materials science and beyond.3 While many protocols for the synthesis of chiral molecules using chiral precursors4–18 or catalysts19 and the separation of racemic chiral molecules20–28 have been developed, it is still a challenge to separate enantiomers without the influence of exogenous chiral factors such as chiral counterions.29 Since Louis Pasteur30 discovered the spontaneous resolution of racemic sodium ammonium tartrate tetrahydrate in 1848, the potential of using spontaneous resolution to separate enantiomers has been well recognized. It has the advantages of easy operation, cost-effectiveness, and simple conditions.31 However, only about 10% of racemic crystals exhibit spontaneous resolution behavior,32 which prevents its widespread industrial application.33
Although predicting and controlling the spontaneous resolution behavior of racemic crystals has long been considered a formidable task,33–35 there are nevertheless two controversial rules of thumb: (1) Wallach's rule: racemic crystals are denser than their chiral counterparts36,37 and (2) chiral salts have a higher propensity of spontaneous resolution than their neutral counterparts.38 In this regard, “counterion engineering” may be an effective means of inducing spontaneous resolution of racemic chiral charged molecules.
In this work, we discovered that spontaneous resolution of the as-prepared title clusters can be achieved simply by recrystallization from glacial acetic acid. We further demonstrate that a unique solvent such as glacial acetic acid could be used, simultaneously as a recrystallization medium (purification process) and as a resolution agent (enantiomeric separation) for racemic nanoclusters.39,40 In addition, glacial acetic acid can effectively eliminate twinning. This 3-in-1 attribute of glacial acetic acid is unmatched by any other solvent which may find applications in chiral separation technologies of importance ranging from pharmaceutics to materials sciences.
Results and discussion
Synthesis, characterization, and structure analysis
The title cluster, [Pd4S2(dppm)3(CNtBu)2]2+ (abbre. as Pd4S2), as the [H(OAc)2]− salt, was synthesized by adding, consecutively, t-butylisonitrile (CNtBu), bis(diphenylphosphino)methane (dppm), and (Me3Si)2S to a solution of Pd4(OAc)4(CO)4 in CH2Cl2 (cf. synthesis in the ESI†). The ligand CNtBu, with a stronger σ-donation power than that of CO,41 and the chelating ligand (diphenylphosphino)methane (dppm) were chosen to replace CO and PR3, respectively, to overcome the well-known intrinsic instability of Pd NCs42 co-protected by the latter ligands. In addition, hexamethyldisilathiane (Me3Si)2S was introduced as the S2− source to further enhance the stability. As a result, the title cluster is extremely stable, even surviving in harsh solvents such as glacial acetic acid.
Crystals suitable for X-ray diffraction were obtained by diffusing diethyl ether into the reaction mixture. Pd4S2 can be obtained in a high yield (62.5%) when the ratio of the reactants, viz., CNtBu, dppm and (Me3Si)2S, is 3
:
1
:
1 (relative to Pd). Pd4S2 crystallizes in three different space groups P43212 and P41212, and P21/n (trace amount), respectively (Fig. 1). P43212 and P41212 constitute a pair of enantiomorphic space groups while P21/n is achiral. Two acetates and two acetic acids per Pd4S2 cluster (Fig. 2) were found in crystals with the P43212 and P41212 space groups ([Pd4S2(dppm)3(CNtBu)2][H(OAc)2]2, hereafter abbreviated as Pd4S2-P43212 and Pd4S2-P41212). However, due to the serious disorder, only one acetate and one acetic acid per Pd4S2 cluster (Fig. 3a) were found in the P21/n crystal (hereafter abbreviated as Pd4S2-P21/n). The co-existence of acetic acid and acetate was also confirmed by FT-IR (Fig. S1†). Subsequent discussions will be based on the crystal structure of Pd4S2-P41212.
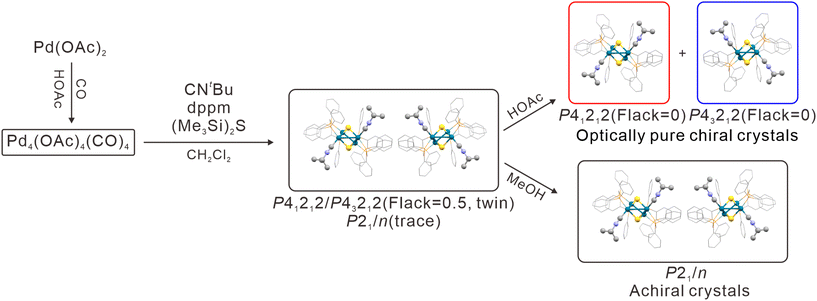 |
| Fig. 1 Schematic illustration of the synthesis and spontaneous resolution of Pd4S2. Color legend: deep sky-blue, Pd; yellow, S; orange, P; light purple, N; and grey, C. All hydrogen atoms, solvents and counterions are omitted for clarity. | |
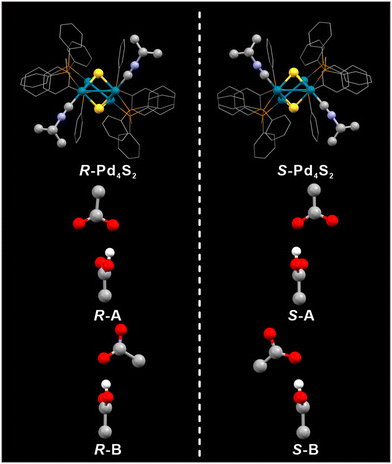 |
| Fig. 2 Enantiomer pair (R and S) of Pd4S2 (top), along with HOAc/OAc− aggregates A (middle) and B (bottom) were derived from the crystal structures of Pd4S2-P41212 and Pd4S2-P43212, respectively. Color legend: deep sky-blue, Pd; yellow, S; orange, P; red, O; light purple, N; grey, C; and white, H. | |
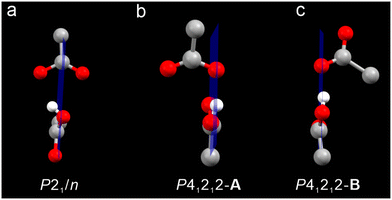 |
| Fig. 3 Different structures of HOAc/OAc− aggregates were observed in different crystal structures of space groups: (a) Pd4S2-P21/n and (b and c) Pd4S2-P41212. Mirror images of (b) and (c) were observed in Pd4S2-P43212. Color legend: red, O; grey, C; and white, H. | |
Pd4S2 displays a twisted planar metal core with four Pd–Pd bonds. The dihedral angle formed by Pd(1)–Pd(1)′–Pd(2) and Pd(1)′–Pd(2)–Pd(2)′ is 126.21° (Fig. 4a). The shortest Pd(1)–Pd(1)′ bond length is 2.5875(9) Å, shorter than the 2.75 Å in palladium metal,43 indicating a strong Pd–Pd interaction. The longest Pd(2)–Pd(2)′ bond length is 3.0375(9) Å, which is ligated by two μ3-S atoms from above and below the twisted Pd4 plane (Fig. 4a). Two more Pd–S bonds exist between these two S atoms and the other two Pd atoms (Pd(1)–S(1) and Pd(1)′–S(1)′). All Pd–Pd bonds are bridged by dppm except the longest one (Fig. 4b), which is terminally coordinated by two CNtBu ligands (Fig. 4c). There is a two-fold axis passing through the midpoints of the longest and shortest Pd–Pd bonds and the methylene carbon of the middle dppm (Fig. 4c). As a result, the entire Pd4S2 cluster conforms to the crystallographically imposed symmetry of C2.
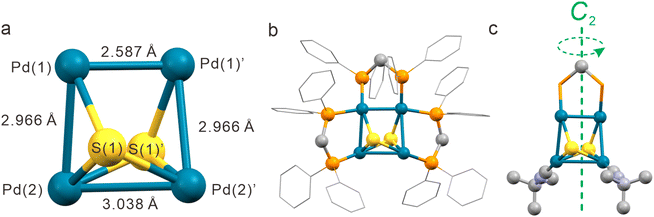 |
| Fig. 4 (a) Arrangement of S atoms on Pd4S2. (b) Arrangement of dppm ligands on Pd4S2. (c) The crystallographic C2 symmetry axis and the arrangement of CNtBu ligands on Pd4S2. Color legend: deep sky-blue, Pd; yellow, S; orange, P; light purple, N; and grey, C. | |
The UV/Vis absorption spectrum of Pd4S2 in a methanol solution displays two peaks at 323 and 415 nm. Additionally, the UV/Vis spectra indicate that Pd4S2 remains stable, with no noticeable decomposition under ambient conditions for at least 3 days (Fig. S2†). As shown in Fig. S3,† there is a prominent peak at m/z = 904.0408, which corresponds to the dication [Pd4S2(dppm)3(CNtBu)2]2+. There is a perfect match between the simulated isotopic patterns and experimental data. The absence of other impurity peaks indicates the high purity of Pd4S2. Since Pd4S2 is a dication, and there are two S2− in Pd4S2, the four palladium atoms carry six positive charges. In this context, due to the rather short bond length, Pd(1)–Pd(1)′ can be considered as a bona fide Pd(I) dimer with a strong Pd–Pd bond, while Pd(2)–Pd(2)′ is a nonbonded Pd(II) dimer. Here I and II represent the formal oxidation states of +1 and +2 of Pd atoms, respectively. XPS analysis also confirmed the coexistence of Pd(I) and Pd(II)44 (Fig. S4†). The two dimers are connected by Pd(1)–Pd(2) and Pd(1)′–Pd(2)′ bonds of length 2.9659(7) Å (Fig. 4a). The two dimers are twisted by 40.48° about the crystallographic C2 axis, thereby lowering the symmetry of Pd4S2 from D4h (hypothetical intermediate structure, vide infra) to C2, turning it into a chiral nanocluster (Fig. 7c).
Spontaneous resolution induced by glacial acetic acid
The as-grown crystals from dichloromethane belong to either the P41212 or P43212 chiral space group, but with a Flack parameter of 0.5, along with a trace amount of achiral crystals of the space group P21/n (as proved by PXRD, Fig. S5†). The Flack parameter of 0.5 of the chiral crystals indicates that they are inversion twins.45 They are in fact identical and will be hereafter labeled as Pd4S2-P41212-twin and Pd4S2-P43212-twin. To our surprise, by adding increasing amounts of acetic acid to the dichloromethane solution, equal proportions of optically pure chiral crystals of space groups P41212 and P43212, both with a Flack parameter of zero (0), could be obtained. They will be referred to as Pd4S2-P41212 and Pd4S2-P43212 (see Tables S3 and S4†). The relative yields of different crystals depend on the ratio of the reactants as well as the acetic acid
:
CH2Cl2 solvent mixture. Subsequently, glacial acetic acid was used as the sole solvent in the recrystallization process and optically pure enantiomeric crystals of space groups P41212 or P43212, both with a Flack parameter of zero (0), were obtained (Table S10†). The electronic circular dichroism (ECD) spectrum also confirmed the spontaneous resolution of Pd4S2 clusters (Fig. S6†). On the other hand, recrystallization from methanol yielded achiral crystals of the space group P21/n as the sole product.
Obviously, the solvent plays a crucial role in determining whether chiral or achiral crystals are formed. However, the underlying mechanisms driving the spontaneous resolution of racemic mixtures are far more intricate. Many factors can influence the spontaneous resolution of racemic mixtures, including but not limited to space filling (Wallach's rule36), hydrogen bonding,3 symmetry,46 stacking,47 coulombic interactions,38etc. In fact, these factors are difficult to completely distinguish because they are not mutually independent.
From the perspective of space filling, homochiral stacking is less efficient (Wallach's rule), suggesting that voids in homochiral stacking are larger than voids in heterochiral stacking. How to fill these larger voids becomes the key in inducing homochiral stacking. Consistent with this notion, in our case, acentric space groups have larger voids to accommodate the anions and solvent molecules, as reflected in the (unit cell volume)/Z listed in Table S11.† Crystallographically, for spontaneously resolved chiral Pd4S2 crystals (Flack = 0), each Pd4S2 cluster has four acetic acids and four acetates with an occupancy of 0.5. They form two independent sets of HOAc/OAc− aggregates labeled as A and B depicted in Fig. 3b and c. This is also the case in the twin crystals (Flack = 0.5). However, only one molecule of acetic acid was found in the crystals of Pd4S2-P21/n. Furthermore, recrystallization of the as-grown Pd4S2 crystals in methanol leads to the replacement of one acetic acid by two methanols per cluster (Fig. S8†), as confirmed by SC-XRD, giving rise to the formula [Pd4S2(dppm)3(CNtBu)2](OAc)2 (MeOH)2 (labeled as Pd4S2-P21/n-MeOH, one of the two OAc− anions was not found due to the serious disorder). This reflects smaller voids in the achiral crystals (cf. Table S11†). To rule out the influence of disorder, we performed liquid-state NMR tests (Fig. S13–16†) on crystals with different space groups. The results once again demonstrated that the chiral space group contains more acetic acid molecules compared to the achiral space group (Table S13,† entries 1–3). Therefore, we believe the larger voids in the chiral crystals accommodate the extra acetic acid molecule, helping to stabilize the chiral crystals.
Non-covalent interactions have been considered to play a crucial role in the spontaneous resolution of enantiomers.3,35,39,40 In our case, a manifold of hydrogen bonds, π⋯π, or C–H⋯π interactions was observed, as tabulated in Table S12.† Particularly worth noting is the H⋯O distances of 2.148 Å between the methylene groups of the wingtip dppm ligands and HOAc/OAc− aggregate B, as portrayed in Fig. 5. The latter represents significant hydrogen bonding. Considering the disordered nature of aggregate B (related by two-fold rotation axes), it appears that aggregate B keeps switching between two positions. In this way, aggregate B may function as a “linker”, connecting two adjacent Pd4S2 clusters of the same chirality to form a homochiral “chain” (Fig. S7†).
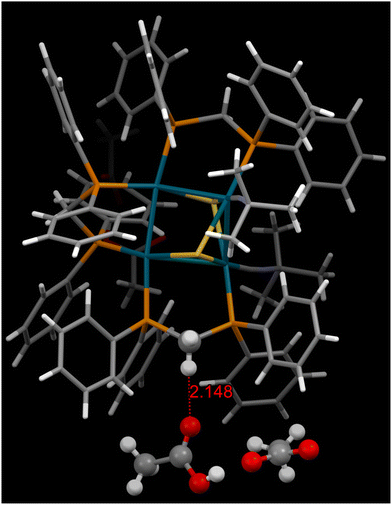 |
| Fig. 5 The weak but significant hydrogen bonding interaction between the carbonyl oxygen atom of acetic acid in aggregate B and one hydrogen atom of the methylene group of the dppm ligand in Pd4S2-P41212. Color legend: deep sky-blue, Pd; yellow, S; orange, P; red, O; light purple, N; grey, C; and white, H. | |
Remarkably, the symmetry of the counterions also seems to affect the spontaneous resolution of Pd4S2. As shown in Fig. 3, the HOAc/OAc− aggregates in Pd4S2-P21/n have a noncrystallographic mirror plane passing through them. In contrast, the aggregates A and B in Pd4S2-P41212 or Pd4S2-P43212 have their planes moved to one side, making the anions chiral as well. Consistent with this observation, the introduction of PF6− as the counteranions, with a smaller volume and spherical symmetry, also led to the centric space group P21/n (see Fig. S9 and Table S7†). Therefore, we believe the symmetry of these chiral anions also contributes to the spontaneous resolution of the racemates to form the respective enantiomers (vide infra).
Finally, it is also interesting to note that glacial acetic acid can effectively eliminate crystal twinning. We note that there are no structural differences between Pd4S2-P41212/P43212 and Pd4S2-P41212/P43212-twin except for the Flack parameters (zero (0) for the former and 0.5 for the latter twin). In this regard, glacial acetic acid can eliminate twinning. Rasmuson48et al. studied the crystal aggregation of paracetamol crystallized in different solvents and found that the aggregation of crystallites can be reduced by using highly polar solvents. They believed that there were strong interactions between highly polar solvents and the crystal surface, thus reducing “crystalline bridging”, thereby promoting the formation of single crystals rather than the twin crystals. We believed that the same effect or a similar effect may be operational here.
Enantioseparation of Pd4S2 by chiral anions
In order to illustrate the importance of the symmetry factor in the spontaneous resolution process, we also performed enantioseparation of Pd4S2 by using chiral counteranions. Here (S)-1,1′-binaphthyl-2,2′-diyl hydrogen phosphate or (R)-1,1′-binaphthyl-2,2′-diyl hydrogen phosphate (abbre. as S-Phos and R-Phos, Fig. S10†) were used in separate experiments to grow the respective enantiomers. The resulting SC-XRD structure determination confirmed that chiral phosphates were successfully introduced into the crystal lattice (see S-Phos&R-Pd4S2 and R-Phos&S-Pd4S2 in Fig. 6 and Tables S14, S15†). Additionally, we also identified the crystal structures of S-Phos&S-Pd4S2 and R-Phos&R-Pd4S2 (Tables S8, S9 and Fig. S18†), although these were only minor (ca. 10%) products. In addition to the ECD peaks at 325 nm (and the accompanying shoulders) of the chiral anions, there are additional peaks at 444 nm and 396 nm due to the chiral Pd4S2 clusters (Fig. 7a). Subsequently, a circular dichroism spectrometer was used to study the racemization of Pd4S2. As can be seen in Fig. S11 and Fig. S12,† the CD signal decreased with increasing temperature and the racemization activation energy obtained from weighted linear fit of the Arrhenius plot (Fig. 7b) was determined to be 31.55 ± 0.82 kcal mol−1.
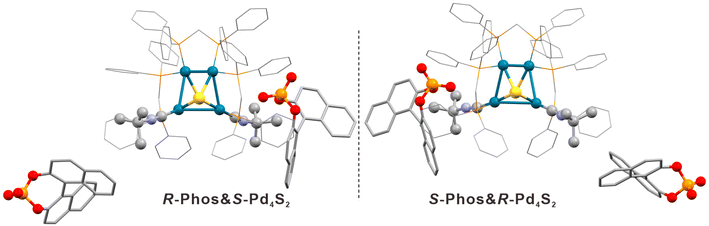 |
| Fig. 6 Enantiomer pair of R-Phos&S-Pd4S2 and S-Phos&R-Pd4S2. Color legend: deep sky-blue, Pd; yellow, S; orange, P; light purple, N; red, O; and grey, C. All hydrogen atoms are omitted for clarity. | |
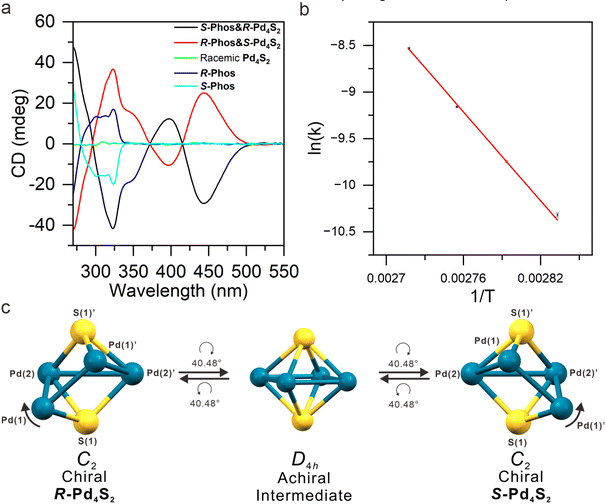 |
| Fig. 7 (a) The ECD spectra of methanol solutions of the enantiomeric pairs S-Phos&R-Pd4S2 and R-Phos&S-Pd4S2, S-Phos and R-Phos and racemic Pd4S2. (b) Arrhenius plot ln(k) vs. 1/T for the racemization of S-Phos&R-Pd4S2 at 353, 358, 363, and 368 K. (c) The origin of chirality of the two enantiomers of Pd4S2. Middle figure: hypothetical intermediate structure (D4h symmetry) consisting of four Pd atoms and two μ4 sulfur atoms. Left and right figures: the clockwise rotation of the Pd(1)–Pd(1)′ bond about the crystallographic C2 axis by 2 × 40.48° relative to the Pd(2)–Pd(2)′ bond results in the conversion of R to S enantiomers, respectively. Color legend: deep sky-blue, Pd and yellow, S. | |
Finally, we wish to propose a plausible racemization mechanism with an octahedral Pd4S2 structure as the intermediate, as follows. First, it occurs to us that the observed Pd4S2 framework may be described as a distorted trigonal prism (tp) with an additional Pd(2)–Pd(2)′ bond across the diagonal of the distorted square face (formed by Pd(2), Pd(2)′, S(1), and S(1)′ as shown in Fig. 7c, left). A clockwise rotation of 40.48° about the two-fold symmetry axis of the enantiomer R-Pd4S2 (Fig. 7c, left) produces two additional Pd–S bonds (Pd(1)–S(1)′ and Pd(1)′–S(1)), making the four Pd atoms coplanar and the S atoms quadruply bridging (μ4-S). The Pd4S2 structure then becomes a distorted octahedron of D4h symmetry (Fig. 7c, middle). Further clockwise rotation of 40.48° gives rise to the enantiomer S-Pd4S2 (Fig. 7c, right), completing the racemization process.
Conclusions
In conclusion, the title cluster [Pd4S2(dppm)3(CNtBu)2]2+ was synthesized and fully characterized. It has a peculiar structure rendering it chiral and crystallizes either as a racemate in a centric space group (P21/n) or as twin crystals of acentric space groups (P41212/P43212). When recrystallized from glacial acetic acid, optically pure crystals of P41212 or P43212 can be obtained. We believe that, in addition to preventing the formation of twin crystals, the number and structural characteristics of the anionic acetic acid/acetate (HOAc/OAc−) aggregates formed in glacial acetic acid may have contributed, in a significant way, to the formation of the optically pure chiral crystals. The use of glacial acetic acid, both as a recrystallization solvent and a resolution agent, as demonstrated in this work, represents an easy and economical route in separating racemic mixtures. This unexpected finding may find applications in chiral separation technologies ranging from pharmaceutics to materials sciences.
Author contributions
Hongwen Deng: conceptualization, data curation, formal analysis, investigation, methodology, validation, visualization, writing – original draft, and writing – review & editing; Peng Yuan: methodology; Kejie Lao: data curation; Qijun Fu: data curation; Boon K. Teo: conceptualization, data analysis, experiment supervision, and writing – review & editing; Nanfeng Zheng: conceptualization, data analysis, funding acquisition, resources, supervision, and writing – review & editing.
Data availability
Crystallographic data: Pd4S2-P41212-twin (CCDC 2343119), Pd4S2-P43212-twin (CCDC 2343114), Pd4S2-P41212 (CCDC 2343112), Pd4S2-P43212 (CCDC 2343113), Pd4S2-P21/n (CCDC 2343120), Pd4S2-P21/n-MeOH (CCDC 2343115), Pd4S2-P21/n-PF6− (CCDC 2343116), S-Phos&S-Pd4S2 (CCDC 2343118), R-Phos&R-Pd4S2 (CCDC 2343117), S-Phos&R-Pd4S2 (CCDC 2382579) and R-Phos&S-Pd4S2 (CCDC 2382580).†
Conflicts of interest
There are no conflicts to declare.
Acknowledgements
We acknowledge the financial support from the National Natural Science Foundation of China (grant no. 92261207, and NSFC Center for Single-Atom Catalysis under grant no. 22388102) and the New Cornerstone Science Foundation. Boon K. Teo acknowledges the support from the State Key Laboratory for Physical Chemistry of Solid Surfaces (PCOSS).
References
- L. D. Barron, Chirality and Life, Space Sci. Rev., 2008, 135, 187–201 CrossRef CAS.
- M. Zhang, G. Qing and T. Sun, Chiral biointerface materials, Chem. Soc. Rev., 2012, 41, 1972–1984 RSC.
- L. Pérez-García and D. B. Amabilino, Spontaneous resolution under supramolecular control, Chem. Soc. Rev., 2002, 31, 342–356 RSC.
- G. Deng, S. Malola, J. Yan, Y. Han, P. Yuan, C. Zhao, X. Yuan, S. Lin, Z. Tang, B. K. Teo, H. Hakkinen and N. Zheng, From Symmetry Breaking to Unraveling the Origin of the Chirality of Ligated Au13Cu2 Nanoclusters, Angew. Chem., Int. Ed., 2018, 57, 3421–3425 CrossRef CAS PubMed.
- H. Shen, Z. Xu, L. Wang, Y. Z. Han, X. Liu, S. Malola, B. K. Teo, H. Hakkinen and N. Zheng, Tertiary Chiral Nanostructures from C−H⋯F Directed Assembly of Chiroptical Superatoms, Angew. Chem., Int. Ed., 2021, 60, 22411–22416 CrossRef CAS PubMed.
- S. Takano and T. Tsukuda, Amplification of the Optical Activity of Gold Clusters by the Proximity of BINAP, J. Phys. Chem. Lett., 2016, 7, 4509–4513 CrossRef CAS PubMed.
- J. Wang, Z. Guan, W. Liu, Y. Yang and Q. Wang, Chiroptical Activity Enhancement via Structural Control: The Chiral Synthesis and Reversible Interconversion of Two Intrinsically Chiral Gold Nanoclusters, J. Am. Chem. Soc., 2019, 141, 2384–2390 CrossRef CAS PubMed.
- Y. Kong, Z. Yan, S. Li, H. Su, K. Li, Y. Zheng and S. Zang, Photoresponsive Propeller-like Chiral AIE Copper(I) Clusters, Angew. Chem., Int. Ed., 2020, 59, 5336–5340 CrossRef CAS PubMed.
- W. Si, K. Sheng, C. Zhang, Z. Wang, S. Zhang, J. Dou, L. Feng, Z. Gao, C. Tung and D. Sun, Bicarbonate insertion triggered self-assembly of chiral octa-gold nanoclusters into helical superstructures in the crystalline state, Chem. Sci., 2022, 13, 10523–10531 RSC.
- B. Li, Y. Kong, S. Li, X. Liu, P. Luo and S. Zang, Synthesis of Atom-Precise Chiral Ag14 Clusters Protected by Penicillamine Ligands, Part. Part. Syst. Charact., 2019, 36, 1900069 CrossRef.
- J. Chai, S. Yang, T. Chen, Q. Li, S. Wang and M. Zhu, Chiral Inversion and Conservation of Clusters: A Case Study of Racemic Ag32Cu12 Nanocluster, Inorg. Chem., 2021, 60, 9050–9056 CrossRef CAS PubMed.
- S. Li, X. Dong, K. Qi, S. Zang and T. C. W. Mak, Full-Color Tunable Circularly Polarized Luminescence Induced by the Crystal Defect from the Co-assembly of Chiral Silver(I) Clusters and Dyes, J. Am. Chem. Soc., 2021, 143, 20574–20578 CrossRef CAS PubMed.
- Y. Yang, X. Pei and Q. Wang, Postclustering Dynamic Covalent Modification for Chirality Control and Chiral Sensing, J. Am. Chem. Soc., 2013, 135, 16184–16191 CrossRef CAS PubMed.
- G. Deng, S. Malola, P. Yuan, X. Liu, B. K. Teo, H. Hakkinen and N. Zheng, Enhanced Surface Ligands Reactivity of Metal Clusters by Bulky Ligands for Controlling Optical and Chiral Properties, Angew. Chem., Int. Ed., 2021, 60, 12897–12903 CrossRef CAS PubMed.
- M. Zhang, X. Dong, Z. Wang, H. Li, S. Li, X. Zhao and S. Zang, AIE Triggers the Circularly Polarized Luminescence of Atomically Precise Enantiomeric Copper(I) Alkynyl Clusters, Angew. Chem., Int. Ed., 2020, 59, 10052–10058 CrossRef CAS PubMed.
- M. Zhang, X. Dong, Z. Wang, X. Luo, J. Huang, S. Zang and T. C. W. Mak, Alkynyl-Stabilized Superatomic Silver Clusters Showing Circularly Polarized Luminescence, J. Am. Chem. Soc., 2021, 143, 6048–6053 CrossRef CAS PubMed.
- R. W. Y. Man, H. Yi, S. Malola, S. Takano, T. Tsukuda, H. Häkkinen, M. Nambo and C. M. Crudden, Synthesis and Characterization of Enantiopure Chiral Bis NHC-Stabilized Edge-Shared Au10 Nanocluster with Unique Prolate Shape, J. Am. Chem. Soc., 2022, 144, 2056–2061 CrossRef CAS PubMed.
- X. Pei, P. Zhao, H. Ube, Z. Lei, K. Nagata, M. Ehara and M. Shionoya, Asymmetric Twisting of C-Centered Octahedral Gold(I) Clusters by Chiral N-Heterocyclic Carbene Ligation, J. Am. Chem. Soc., 2022, 144, 2156–2163 CrossRef CAS.
- J. Wang, R. He, W. Liu, Q. Feng, Y. Zhang, C. Liu, J. Ge and Q. Wang, Integration of Metal Catalysis and Organocatalysis in a Metal Nanocluster with Anchored Proline, J. Am. Chem. Soc., 2023, 145, 12255–12263 CrossRef CAS.
- I. Dolamic, S. Knoppe, A. Dass and T. Bürgi, First enantioseparation and circular dichroism spectra of Au38 clusters protected by achiral ligands, Nat. Commun., 2012, 3, 798 CrossRef PubMed.
- H. Yoshida, M. Ehara, U. D. Priyakumar, T. Kawai and T. Nakashima, Enantioseparation and chiral induction in Ag29 nanoclusters with intrinsic chirality, Chem. Sci., 2020, 11, 2394–2400 RSC.
- H. Yi, K. M. Osten, T. I. Levchenko, A. J. Veinot, Y. Aramaki, T. Ooi, M. Nambo and C. M. Crudden, Synthesis and enantioseparation of chiral Au13 nanoclusters protected by bis-N-heterocyclic carbene ligands, Chem. Sci., 2021, 12, 10436–10440 RSC.
- N. Barrabés, B. Zhang and T. Bürgi, Racemization of Chiral Pd2Au36(SC2H4Ph)24: Doping Increases the Flexibility of the Cluster Surface, J. Am. Chem. Soc., 2014, 136, 14361–14364 CrossRef.
- B. Zhang and T. Bürgi, Doping Silver Increases the Au38(SR)24 Cluster Surface Flexibility, J. Phys. Chem. C, 2016, 120, 4660–4666 CrossRef CAS.
- S. Knoppe, O. A. Wong, S. Malola, H. Häkkinen, T. Bürgi, T. Verbiest and C. J. Ackerson, Chiral Phase Transfer and Enantioenrichment of Thiolate-Protected Au102 Clusters, J. Am. Chem. Soc., 2014, 136, 4129–4132 CrossRef CAS PubMed.
- G. Deng, B. K. Teo and N. Zheng, Assembly of Chiral Cluster-Based Metal-Organic Frameworks and the Chirality Memory Effect during their Disassembly, J. Am. Chem. Soc., 2021, 143, 10214–10220 CrossRef CAS PubMed.
- Y. Zhu, H. Wang, K. Wan, J. Guo, C. He, Y. Yu, L. Zhao, Y. Zhang, J. Lv, L. Shi, R. Jin, X. Zhang, X. Shi and Z. Tang, Enantioseparation of Au20(PP3)4Cl4 Clusters with Intrinsically Chiral Cores, Angew. Chem., Int. Ed., 2018, 57, 9059–9063 CrossRef CAS PubMed.
- J. Yan, H. Su, H. Yang, C. Hu, S. Malola, S. Lin, B. K. Teo, H. Häkkinen and N. Zheng, Asymmetric Synthesis of Chiral Bimetallic [Ag28Cu12(SR)24]4− Nanoclusters via Ion Pairing, J. Am. Chem. Soc., 2016, 138, 12751–12754 CrossRef CAS PubMed.
- T. Buhse, J. M. Cruz, M. E. Noble-Terán, D. Hochberg, J. M. Ribó, J. Crusats and J. C. Micheau, Spontaneous Deracemizations, Chem. Rev., 2021, 121, 2147–2229 CrossRef CAS PubMed.
- H. Flack, Louis Pasteur's discovery of molecular chirality and spontaneous resolution in 1848, together with a complete review of his crystallographic and chemical work, Acta Crystallogr., Sect. A: Found. Adv., 2009, 65, 371–389 CrossRef CAS.
- H. Lorenz and A. Seidel-Morgenstern, Processes To Separate Enantiomers, Angew. Chem., Int. Ed., 2014, 53, 1218–1250 CrossRef CAS.
- M. P. Walsh, J. A. Barclay, C. S. Begg, J. Xuan and M. O. Kitching, Conglomerate Crystallization in the Cambridge Structural Database (2020–2021), Cryst. Growth Des., 2023, 23, 2837–2844 CrossRef CAS.
- J. E. Carpenter and M. Grünwald, Heterogeneous Interactions Promote Crystallization and Spontaneous Resolution of Chiral Molecules, J. Am. Chem. Soc., 2020, 142, 10755–10768 CrossRef CAS PubMed.
- A. Dey and E. Pidcock, The relevance of chirality in space group analysis: A database study of common hydrogen-bonding motifs and their symmetry preferences, CrystEngComm, 2008, 10, 1258–1264 RSC.
- X. Lin, B. Kou, J. Cao, P. Weng, X. Yan, Z. Li and Y. Jiang, Spontaneous Resolution of Helical Building Blocks through the Formation of Homochiral Helices in Two Dimensions, Angew. Chem., Int. Ed., 2022, 61, e202205914 CrossRef CAS PubMed.
- O. Wallach, Ueber gebromte Derivate der Carvonreihe, Liebigs Ann. Chem., 1895, 286, 90–143 CrossRef CAS.
- C. P. Brock, W. B. Schweizer and J. D. Dunitz, On the validity of Wallach's rule: on the density and stability of racemic crystals compared with their chiral counterparts, J. Am. Chem. Soc., 1991, 113, 9811–9820 CrossRef CAS.
- J. Jacques, M. Leclercq and M. J. Brienne, Does Salt Formation Increase the Frequency of Spontaneous Resolution, Tetrahedron, 1981, 37, 1727–1733 CrossRef CAS.
- X. Liang, Y. Li, Z. Wang, S. Zhang, Y. Liu, Z. Cao, L. Feng, Z. Gao, Q. Xue, C. Tung and D. Sun, Revealing the chirality origin and homochirality crystallization of Ag14 nanocluster at the molecular level, Nat. Commun., 2021, 12, 4966 CrossRef CAS PubMed.
- J. Huang, Z. Wang, S. Zang and T. C. W. Mak, Spontaneous Resolution of Chiral Multi-Thiolate-Protected Ag30 Nanoclusters, ACS Cent. Sci., 2020, 6, 1971–1976 CrossRef CAS PubMed.
-
M. Lazar and R. J. Angelici, in Modern Surface Organometallic Chemistry, 2009, pp. 513–556 Search PubMed.
- E. G. Mednikov and L. F. Dahl, Palladium: It forms unique nano-sized carbonyl clusters, J. Chem. Educ., 2009, 86, 1135 CrossRef CAS.
- M. Kawano, J. W. Bacon, C. F. Campana and L. F. Dahl, An Unprecedented High-Nuclearity Closest-Packed Bimetallic Palladium Carbonyl Cluster: [Pd33Ni9(CO)41(PPh3)6]4− Containing a Pseudo-D3h hcp Pd33Ni9 Core, J. Am. Chem. Soc., 1996, 118, 7869–7870 CrossRef CAS.
- N. T. Oleg and G. B. Lev, Palladium(I) Complexes in Coordination Chemistry and Catalysis, Russ. Chem. Rev., 1983, 52, 117 CrossRef.
- H. D. Flack and G. Bernardinelli, Reporting and evaluating absolute-structure and absolute-configuration determinations, J. Appl. Crystallogr., 2000, 33, 1143–1148 CrossRef CAS.
- M. C. Etter and K. S. Huang, Induction of noncentrosymmetry by polar hydrogen-bonded chains in nitroaniline crystals, Chem. Mater., 1992, 4, 824–827 CrossRef CAS.
- K. Kinbara, Y. Hashimoto, M. Sukegawa, H. Nohira and K. Saigo, Crystal Structures of the Salts of Chiral Primary Amines with Achiral Carboxylic Acids: Recognition of the Commonly-Occurring Supramolecular Assemblies of Hydrogen-Bond Networks and Their Role in the Formation of Conglomerates, J. Am. Chem. Soc., 1996, 118, 3441–3449 CrossRef CAS.
- E. M. Ålander, M. S. Uusi-Penttilä and Å. C. Rasmuson, Agglomeration of Paracetamol during Crystallization in Pure and Mixed Solvents, Ind. Eng. Chem. Res., 2004, 43, 629–637 CrossRef.
Footnote |
† Electronic supplementary information (ESI) available: Experimental section, physical measurements, additional characterization data, and detailed crystal structure analysis. CCDC 2343112–2343120, 2382579-2382580. For ESI and crystallographic data in CIF or other electronic format see DOI: https://doi.org/10.1039/d4qi01944j |
|
This journal is © the Partner Organisations 2025 |
Click here to see how this site uses Cookies. View our privacy policy here.