DOI:
10.1039/D4QI02426E
(Research Article)
Inorg. Chem. Front., 2025,
12, 179-190
Hierarchical FeCo LDH/NiSe heterostructure electrocatalysts with rich heterointerfaces for robust water splitting at an industrial-level current density†
Received
26th September 2024
, Accepted 5th November 2024
First published on 6th November 2024
Abstract
Sustainable hydrogen production by electrocatalytic water splitting is a promising energy storage technology yet challenging due to the sluggish kinetics of the oxygen evolution reaction (OER). Rationally designed robust and high-efficiency non-noble metal electrocatalysts for large-current-density OER are highly desirable for industrial-grade hydrogen production. Herein, a unique hierarchical heterostructure electrocatalyst composed of FeCo layered double hydroxide nanosheets and a NiSe nanowire array grown on Ni foam (FeCo LDH/NiSe@NF) is demonstrated. The optimized FeCo LDH/NiSe@NF exhibits an excellent OER activity with low overpotentials of 230, 266 and 298 mV at current densities of 100, 500 and 1000 mA cm−2 in an alkaline solution. In particular, it can deliver 1000 mA cm−2 without much decay after the long-term stability test for 100 h. Both the experimental results and theoretical calculations reveal that reasonable construction of a hierarchical heterostructure, as well as the strong interaction at the heterointerface between NiSe and FeCo LDH can not only offer sufficient exposure of the surface active sites, better electrochemical conductivity and structural stability, but also effectively optimize electronic configurations and lower the reaction energy barriers, consequently facilitating the reaction kinetics especially in large-current-density alkaline water electrolysis. Moreover, a two-electrode electrolyzer using FeCo LDH/NiSe@NF and NiCoP/NiCoSx@NF as the anode and cathode only needs a cell voltage of 1.849 V to reach 1000 mA cm−2 in 30 wt% KOH solution at 80 °C. The high catalytic activity and long-term stability of the catalyst at large current densities have exceeded most electrocatalysts reported, highlighting its great potential in large-scale applications.
1 Introduction
As a promising energy storage technology, hydrogen production from water electrolysis is widely used to store intermittent energy sources such as solar and wind energy. In particular, alkaline water electrolysis (AWE) has the characteristics of low cost, a simple equipment structure, and high commercial maturity and has been applied on a large-scale industrial application.1,2 Conventional alkaline electrolyzers generally operate at a cell voltage of about 1.8–2.4 V to achieve a low current density of 250–400 mA cm−2 at 50–80 °C, 30 wt% KOH solution, which has been plagued by low energy conversion efficiency and poor stability.3,4 The anodic oxygen evolution reaction (OER) is always considered to be the main bottleneck for industrial AWE because it often requires a high overpotential due to the slow four-electron reaction kinetics especially at large current densities. Thus, the rational design of robust and highly efficient electrocatalysts is extremely urgent for improving electrocatalytic OER activity and reducing the cell voltage of AWE under industrial conditions.
In search of non-noble metal electrocatalysts that meet the above conditions, researchers have focused on a number of 3d transition metal (especially Ni, Co, and Fe)-based hybrid electrocatalysts with different structures and compositions by constructing a three-dimensional (3D) heterostructure.5,6 Recently, transition metal selenides (TMSes) have attracted much attention as a candidate component for the fabrication of heterostructured catalysts.7 Since the anion size of Se2− in selenides is larger, and the energy level of the 3d orbital of Se is close to that of the 3s orbital and the 3p orbital, it's conducive to bonding with metal atoms. Such electronic arrangement results in better metallicity and electrical conductivity of TMSes, which facilitates electron transport and enhances the electrical conductivity.8 Besides, the nanowire/nanorod nanoarray morphology of TMSes with open frameworks can promote the formation of a large number of heterointerfaces as well as provide a large electrochemically active surface area, thus providing more electrochemically active sites. For example, Lee et al. reported a 3D hierarchical WCoSe/WCo3O4 heterostructure grown on Ni foam as a bifunctional electrocatalyst in alkaline solution, which can tune the electronic structures and increase active catalyst sites, thereby promoting the electrocatalytic performance for bifunctional water splitting.9 Zhang et al. prepared NiMoO4@Mo15Se19/NiSe2 core–shell nanostructures that can accelerate the electron transfer and expand more active sites, and the unique d–p orbital hybridization further decreased the adsorption energy of oxygen-containing species.10 However, the high adsorption energy barriers of oxygen intermediates in TMSes still limit the further improvement of their electrocatalytic efficiency.
It is noteworthy that metal layered double hydroxides (LDHs) based on Fe, Co, and Ni elements are perceived to have great potential in heterostructure electrocatalysts because of their rich metal sites, easily adjustable components and flexible tailoring of the two-dimensional layered nanosheet structure.11–14 It has been reported that surface reconstruction of metal LDHs during the oxidation process to form MOOH as a true active species can optimize the adsorption energy of intermediates in the OER process.15 Nevertheless, the catalyst performance of metal LDHs alone is still limited by the poor conductivity.16,17 Therefore, it is expected to design a heterostructure composed of materials with good electrical conductivity and metal LDHs for further improving the catalytic performance. For instance, Yu et al. proposed NiFe LDH nanosheets grown on Cu nanowires (Cu@NiFe LDH) as a bifunctional electrocatalyst for water splitting, where the Cu NWs and Cu@NiFe LDH showed smaller ohmic resistances (Rs), indicating good electrical contact with the Cu substrate.18 Zhai et al. demonstrated that the hierarchical bimetal nitride/hydroxide (NiMoN/NiFe LDH) array had faster kinetics for adsorption of the *OH intermediate due to fast charge transport through operando electrochemical impedance spectroscopy (EIS).19
Based on the above, herein, a NiSe nanowire surrounded by a FeCo LDH nanosheet 3D heterostructure array on Ni foam (FeCo LDH/NiSe@NF) was fabricated via a two-step hydrothermal–electrodeposition strategy. Benefiting from this unique hierarchical system, a large number of FeCo LDH/NiSe heterointerfaces enable more active sites to be exposed to the reactants and accelerate the reaction kinetics for OER. Consequently, the optimized FeCo LDH/NiSe@NF exhibits a remarkable OER activity with low overpotentials of 230, 266 and 298 mV at current densities of 100, 500 and 1000 mA cm−2, respectively, along with an outstanding long-term stability (100 h) in 1 M KOH, which outperforms most of the catalysts for alkaline large-current-density OER. Furthermore, a two-electrode alkaline electrolyzer with a FeCo LDH/NiSe@NF anode and a NiCoP/NiCoSx@NF cathode also demonstrates exceptional overall water splitting performance under harsh conditions of 30 wt% KOH strong alkaline and high temperatures up to 80 °C. Theoretical calculations demonstrate that the strong coupling effect between FeCo LDH and NiSe at the heterointerface could effectively regulate the local electronic structure and the d-band center, which is favorable for balancing the adsorption of H2O and thus reducing the energy barrier of the OER process.
2 Experimental methods
2.1 Chemicals
Co(NO3)2·6H2O (99.99% metals basis), FeSO4·7H2O (≥99%), Se powder (≥99.99% metals basis), Pt/C (20%) and commercial IrO2 were purchased from Aladdin Reagent (Shanghai) Co., Ltd. NaBH4 (98%), KOH (AR), HCl (36–38%), ethanol and acetone were purchased from Sinopharm Chemical Reagent Co., Ltd. Nafion solution (5 wt%) was purchased from Du Pont Company. Ni foam (NF, thickness: 1 mm, porosity: ca. 95%) was purchased from Jiashide Foam Metal Co., Ltd. All chemicals were used directly without further purification. Ultrapure Milli-Q water (18.2 MΩ cm−1) was used in all experiments.
2.2 Synthesis of NiSe@NF
Firstly, Ni foam was cut into 1 × 4 cm2 plates, followed by ultrasonically cleaning with acetone, 3 M HCl, ultrapure water and ethanol for 15 min, respectively, and then dried at 40 °C for 6 h. NiSe@NF was obtained by a hydrothermal method. Specifically, excess NaBH4 was dissolved in 30 mL ethanol under stirring. Next, 59 mg Se powder was added and stirred for several minutes. The precursor solution was then poured into a 50 mL Teflon-lined stainless-steel autoclave where a cleaned Ni foam was placed at the bottom. The autoclave was transferred to an oven and maintained at 140 °C for 12 h. The obtained material was washed with ethanol and dried at 60 °C for 1 h.
2.3 Synthesis of FeCo LDH/NiSe@NF
FeCo LDH was grown on NiSe@NF by an electrodeposition method. NiSe@NF (deposition area of 0.4 cm2), Ag/AgCl and graphite rod were used as working, reference and counter electrodes, respectively. Typically, the electrodeposition experiment was carried out at a cathodic current density of 50 mA cm−2 for 300 s in 50 mL solution containing various molar ratios of FeSO4 and Co(NO3)2 (2
:
3, 1
:
1 and 3
:
2 for Fe/Co). The total amount of Co2+ and Fe2+ was 2.5 mmol. Co(OH)2/NiSe@NF was synthesized by the same method without the addition of FeSO4·7H2O. Additionally, the FeCo LDH/NiSe@NF (1
:
1) electrodes deposited at 10, 25 and 100 mA cm−2 for 300 s, as well as for 150 and 600 s at 50 mA cm−2 were also synthesized. The loading of the catalyst on NF was about 5.6 mg cm−2.
2.4 Fabrication of IrO2@NF and Pt/C@NF
To fabricate the IrO2 or Pt/C loaded on Ni foam for comparison, 11.2 mg commercial IrO2 or Pt/C (20%) and 60 μL Nafion solution were dispersed in 600 μL ethanol and 340 μL water by sonication for 1 h. The 200 μL of ink was then coated onto NF and dried in air.
2.5 Characterization
The XRD patterns of the as-synthesized electrodes were characterized using a PANalytical B.V. X-pert Powder X-ray diffractometer equipped with Cu Kα radiation (λ = 1.5406 Å). The morphologies were analyzed by field emission scanning electron microscopy (SEM, Hitachi SU-70) and transmission electron microscopy (TEM, FEI Tecnai G2 F20 S-TWIN). The XPS measurements were performed using a Thermo Scientific K-Alpha apparatus with an Al Kα excitation source, and the obtained binding energies were corrected for specimen charging by referencing C 1s to 284.6 eV. The Raman spectra were performed on a LabRAM HR Evolution spectrometer (λ = 532 nm) in the range of 100–1000 cm−1. The atomic force microscopy (AFM) measurement was carried out on a Bruker Dimension ICON, and three positions were taken for parallel testing. In addition, the contact angle (CA) measurements were conducted on a LAUDA OSA200-T instrument.
2.6 Electrochemical measurements
All OER electrochemical measurements were performed on a Bio-Logic potentiostat with a standard three-electrode system using 1.0 M KOH aqueous solution as the electrolyte. The as-synthesized electrodes (0.4 cm2) were directly employed as the working electrode, a graphite rod served as the counter electrode, and an Hg/HgO electrode was used as the reference electrode. Polarization curves were obtained using linear sweep voltammetry (LSV) with a scan rate of 5 mV s−1. All the LSV curves under the three-electrode system were corrected by 100% iR compensation and all the potentials were referred to the reversible hydrogen electrode (RHE): ERHE = EHg/HgO + 0.098 + 0.0592 pH − iR. The electrochemical impedance spectra (EIS) with an amplitude of 5 mV were recorded in the frequency range of 10 mHz–100 kHz. The chronopotentiometry test was conducted at current densities of 100, 500 and 1000 mA cm−2. The faradaic efficiency (FE) was tested by applying a constant current density of 300 mA cm−2 for 60 minutes, and the volume of oxygen was measured by the water drainage method. The double layer capacitance (Cdl) values were assessed by cyclic voltammetry (CV) measurements in the potential range from 0.71 to 0.81 V vs. RHE at different scan rates (20, 40, 60, 80 and 100 mV s−1).
For the two-electrode overall water splitting system, FeCo LDH/NiSe@NF was used as both anode and cathode. The LSV (without iR compensation) and chronopotentiometry tests were carried out in 1.0 M KOH and 30 wt% KOH solution at 25, 50 and 80 °C.
2.7 Computational methods
The Vienna Ab initio Package (VASP)20,21 was used for all the density functional theory (DFT) calculations within the generalized gradient approximation (GGA) using the Perdew–Burke–Ernzerhof (PBE) functional.22,23 The projected augmented wave (PAW) method24 was chosen to describe the exchange–correlation effects and the energy cutoff for plane wave basis was set to 400 eV. The FeCo LDH (001)/NiSe (101) heterostructure was constructed. For comparison, the NiSe (101) slab in a 3 × 4 supercell, FeCo LDH (001) slab and Co(OH)2 (001)/NiSe (101) heterostructure were also constructed. The structural optimization was completed until energy and force convergence on each atom were less than 1.0 × 10−4 eV and 0.05 eV Å−1, respectively. The Brillouin zone integration was sampled using a 3 × 3 × 1 Monkhorst–Pack k-point grid. The Gibbs free energies of oxygen intermediates during the OER were calculated as follows:where ΔE, ΔZPE and ΔS are the total energy difference, the zero point energy difference, and the entropy change, respectively. T was set to room temperature (298.15 K). When the electrode potential U = 0 V, the catalytic activity was estimated by the energy barrier of the rate-determining step (RDS): | ΔGRDS = max[ΔG1, ΔG2, ΔG3, ΔG4] | (2) |
where ΔG1 = ΔG*OH, ΔG2 = ΔG*O − ΔG*OH, ΔG3 = ΔG*OOH − ΔG*O, ΔG4 = 4.92 − ΔG*OOH.
And the theoretical overpotential is equal to:
| ηOER = ΔGRDS/e − 1.23 V. | (3) |
3 Results and discussion
We used a two-step method for preparing FeCo LDH nanosheets covered on the NiSe nanowire hierarchical heterostructure on Ni foam (FeCo LDH/NiSe@NF), as indicated in the schematic illustration (Fig. 1a). Initially, the NiSe nanowire array was vertically grown on the NF substrate uniformly (NiSe@NF) with an average width of 48 nm by hydrothermal treatment at 140 °C for 12 h as shown in scanning electron microscopy (SEM) and transmission electron microscopy (TEM) images (Fig. S1†). Then NiSe@NF served as the skeleton for the subsequent electrochemical deposition of outer ultrathin FeCo LDH nanosheets to form a nanosheet–nanowire hierarchical 3D array structure (Fig. S2† and Fig. 1b). Unlike stacked pieces in chunks for FeCo LDH@NF (Fig. S3†) and scaly flakes for Co(OH)2/NiSe@NF (Fig. S4†), the morphology of FeCo LDH in the upper layer of FeCo LDH/NiSe@NF presents flower-like ultrathin nanosheet clusters (Fig. S2b† and Fig. 1b1), which can protect the lower layer from falling off the NF skeleton. SEM (Fig. 1b2) and TEM images (Fig. 1c) show that the NiSe nanowire array in the lower layer is further uniformly covered with interconnected ultrathin FeCo LDH nanosheets after decoration with FeCo LDH, which is favorable for forming a large number of heterointerfaces of FeCo LDH/NiSe to provide more active sites for OER and accelerate the mass and charge transfer. Meanwhile, the ultrathin nature of the FeCo LDH nanosheets with a thickness of about 1.45 nm was also confirmed using an atomic force microscope (AFM) (Fig. S5†). As displayed in Fig. 1d and f and Fig. S6,† the energy dispersive X-ray spectroscopy (EDS) elemental line scanning and mapping images further identify the core–shell structure of the lower layer, clearly indicating that NiSe is located in the core while FeCo LDH is homogeneously distributed throughout the whole catalyst. Besides, the molar content of elements in NiSe@NF and FeCo LDH/NiSe @NF were determined by EDS, suggesting the Co/Fe molar ratio of 1
:
1 (Fig. S7 and Table S1†). The high-resolution TEM (HRTEM) images in Fig. 1e show that the lattice spacings of 0.21 and 0.26 nm correspond to the (107) crystal plane of FeCo LDH (PDF #50-0235) and the (101) crystal plane of hexagonal NiSe (PDF #02-0892), respectively. And the contact between FeCo LDH and NiSe is close.
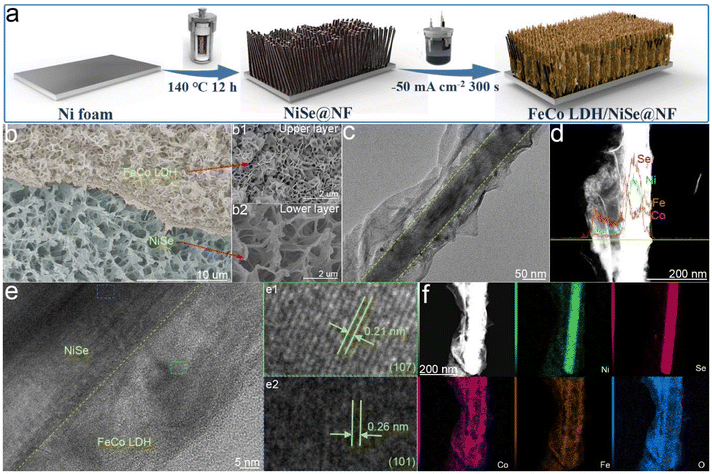 |
| Fig. 1 (a) Schematic illustration of the fabrication process of FeCo LDH/NiSe@NF heterostructure electrode. (b) Low- and (b1 and b2) high-magnification SEM images, (c) TEM image, (d) EDS line scan image, (e) HRTEM image and (e1 and e2) the lattice fringe images of the squared part, and (f) HAADF-STEM and corresponding EDS elemental mapping images of FeCo LDH/NiSe@NF. | |
The crystal structures of the as-prepared catalysts were studied by X-ray diffraction (XRD). As shown in Fig. 2a, all XRD patterns have two strong peaks at 44.4° and 51.7°, which belong to NF substrate. The NiSe@NF prepared by hydrothermal method consists of the two mixed crystal phases. The diffraction peaks at 30.6°, 46.5°, 48.1°, 54.1° and 63.7° could be indexed to the (113), (117), (152), (200) and (0410) planes of orthorhombic NiSe (PDF #29-0935), and the diffraction peaks at 32.8°, 49.9°, 59.5° and 61.3° could be indexed to the (101), (110), (103) and (201) planes of hexagonal NiSe (PDF #02-0892). The Co(OH)2 grown on the NF substrate was confirmed according to the PDF #30-0443. The introduction of Fe during the electrodeposition step leads to the formation of FeCo LDH, where the main diffraction peaks at 33.6°, 39.2° and 59.8° correspond to PDF #50-0235 of FeCo LDH. When FeCo LDH was deposited on the NiSe@NF, the main XRD diffraction peaks of both FeCo LDH and hexagonal NiSe could still be observed, illustrating the successful preparation of FeCo LDH/NiSe heterostructure. The Raman spectrum of FeCo LDH/NiSe@NF (Fig. S8†) shows two characteristic peaks at 455 and 534 cm−1 arising from M–O–M bonds (M = Fe, Co).25,26
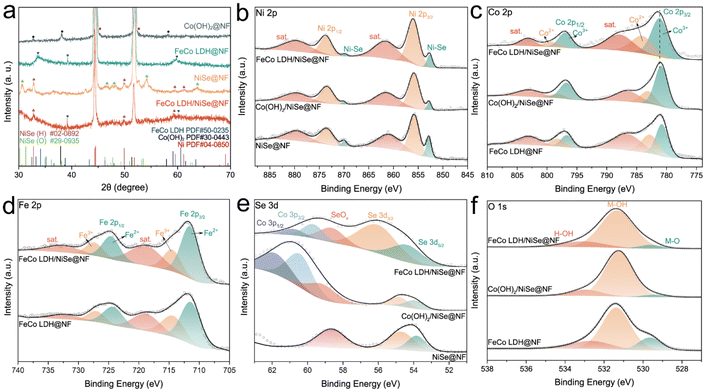 |
| Fig. 2 (a) XRD patterns of NiSe@NF, FeCo LDH@NF, Co(OH)2/NiSe@NF and FeCo LDH/NiSe@NF. High resolution XPS spectra of (b) Ni 2p, (c) Co 2p, (d) Fe 2p, (e) Se 3d and (f) O 1s for different samples. | |
X-ray photoelectron spectroscopy (XPS) analysis was further utilized to investigate the chemical composition and chemical state of NiSe@NF, FeCo LDH@NF, Co(OH)2/NiSe@NF and FeCo LDH/NiSe@NF. The Ni 2p XPS spectra in Fig. 2b shows two peaks at 855.95 and 873.68 eV together with their satellite peaks at 861.68 and 879.88 eV, which can be ascribed to Ni 2p3/2 and Ni 2p1/2 of Ni2+ in NiSe, respectively.27,28 Additionally, the peaks located at 852.68 and 869.78 eV are assigned to the Ni–Se bonds.29–31 The Co 2p XPS spectra are shown in Fig. 2c, in which two sets of doublet peaks at 781.18/796.98 eV and 784.28/799.38 eV are attributed to Co 2p3/2/Co 2p1/2 of Co3+ and Co2+, respectively. Compared to Co(OH)2/NiSe@NF, FeCo LDH/NiSe@NF has a positive shift in binding energy after introducing Fe, revealing the charge transfer to Fe. In Fig. 2d, Fe 2p spectra could be deconvoluted to two sets of doublet peaks of Fe2+ (711.68/724.68 eV) and Fe3+ (714.68/727.48 eV) accompanied by two satellite peaks at 719.28 and 732.98 eV. For the Se 3d spectra in Fig. 2e, there are two peaks appeared at around 53.84 and 54.72 eV for NiSe@NF, which are attributed to Se 3d5/2 and Se 3d3/2. And the peak at about 58.70 eV indicates the existence of SeOx due to the surface Se species oxidation when NiSe@NF was exposed to air.32,33 After the decoration of Co(OH)2 or FeCo LDH, the wider peak can be observed at 59–61 eV, indicating the overlap of SeOx and the Co 3p.34–36 The O 1s XPS spectra (Fig. 2f) indicate three oxygen species of M–O (529.58 eV), M–OH (531.38 eV), and H–OH (533.08 eV). The XPS together with XRD and TEM results show conclusive evidence that the prepared catalyst consists of NiSe and FeCo LDH.
The electrocatalytic OER activity of FeCo LDH/NiSe@NF in 1.0 M KOH was studied in a standard three-electrode system in which Co(OH)2/NiSe@NF, FeCo LDH@NF, NiSe@NF, com-IrO2 dispersed on NF (IrO2@NF) and NF substrate were simultaneously investigated for comparison. All potentials were referenced to the reversible hydrogen electrode (RHE) with the iR-correction. In order to obtain optimal preparation conditions, we first assessed the electrocatalytic activity of FeCo LDH/NiSe@NF prepared under different Fe/Co feeding ratios, electrodeposition current densities and time. From the linear sweep voltammetry (LSV) curves in Fig. S9–11,† we can find that the FeCo LDH deposited on NiSe@NF with the Fe/Co molar ratio of 1
:
1 performs best under the electrodeposition condition of 50 mA cm−2 for 300 s. As shown in Fig. 3a, the OER activity of NiSe@NF is significantly improved after the decoration of FeCo LDH nanosheets. Apparently, FeCo LDH/NiSe@NF requires the lowest overpotential of 230 mV at the current density of 100 mA cm−2, much lower than that of Co(OH)2/NiSe@NF (287 mV), FeCo LDH@NF (288 mV), NiSe@NF (335 mV), bare NF (415 mV), and even commercial IrO2 on NF (295 mV). Besides, the weak anodic peaks near 1.38 V vs. RHE are associated with the oxidation from Ni(II) to Ni(III).26,37 Moreover, the large-current-density OER catalytic activity of as-prepared electrodes was further compared to assess the commercial viability. Again, FeCo LDH/NiSe@NF processes the lowest overpotentials of 266 and 298 mV to deliver the large current densities of 500 and 1000 mA cm−2, respectively, which is far superior to Co(OH)2/NiSe@NF (331 and 372 mV), FeCo LDH@NF (351 and 417 mV), and NiSe/NF (423 and 593 mV), as shown in Fig. 3b. The electrocatalytic kinetic profiles were investigated by steady–state Tafel slope and electrochemical impedance spectroscopy (EIS). The Tafel slope of NiSe@NF and FeCo LDH@NF are 104 and 81 mV dec−1, respectively (Fig. 3c). While the Tafel slope of FeCo LDH/NiSe@NF drastically reduces to 48 mV dec−1 after combining FeCo LDH with NiSe, suggesting the strong synergy of FeCo LDH/NiSe heterostructure and an accelerated reaction kinetics on FeCo LDH/NiSe@NF for the OER process. The Nyquist plot is fitted in Fig. 3d, and compared to other electrodes, the FeCo LDH/NiSe@NF exhibits a much smaller semicircle. It means that the FeCo LDH/NiSe@NF has the smallest charge transfer resistance (Rct) and excellent electrochemical conductivity, which is consistent with the results of Tafel slopes.
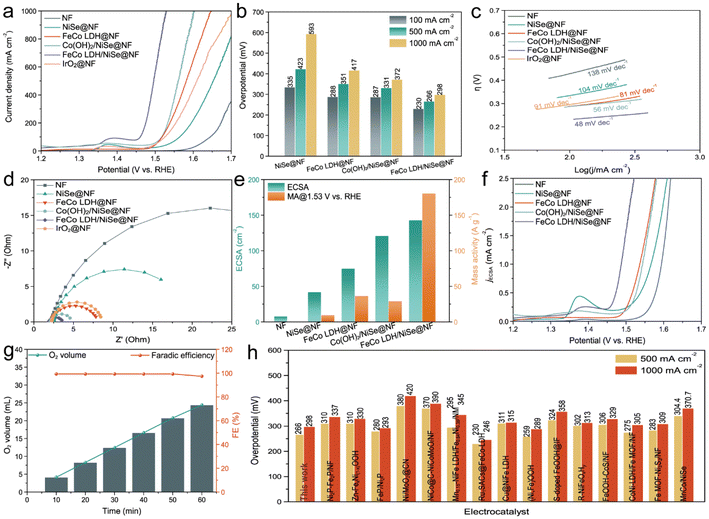 |
| Fig. 3 (a) LSV polarization curves, (b) overpotentials at the current densities of 100, 500, and 1000 mA cm−2, (c) Tafel slope plots, (d) Nyquist plot, (e) ECSA (left) and mass activity at 1.53 V vs. RHE (right), and (f) ECSA-normalized LSV curves of NiFe@NF, FeCo LDH@NF, Co(OH)2/NiSe@NF, FeCo LDH/NiSe@NF and IrO2@NF. (g) Volume of produced O2 as a function of time (left) and faradaic efficiency of OER (right) for FeCo LDH/NiSe@NF. (h) Comparison of the overpotentials of FeCo LDH/NiSe@NF and the other electrocatalysts recently reported at current densities of 500 and 1000 mA cm−2 for OER in 1.0 M KOH.18,38–51 | |
To evaluate the inherent catalytic activity, the electrochemical active surface area (ECSA) of the electrodes was estimated via the double-layer capacitance (Cdl), considering their proportional relationship. And the Cdl could be measured in terms of the linear slope of current density and scan rate in the CV curves (Fig. S12†). As expected, the FeCo LDH/NiSe@NF has the ECSA of 143 cm2, which is larger than that of Co(OH)2/NiSe@NF (121 cm2), FeCo LDH@NF (75 cm2) and NiSe@NF (42 cm2) (Fig. 3e). Therefore, the FeCo LDH/NiSe heterostructure exposes abundant active sites due to the plentiful interfaces in the 3D hierarchical heterostructure, which is beneficial for the electrode to have full contact with the electrolyte, thereby accelerating the mass transfer process and boosting the OER activity. Fig. 3e also further compares their mass activity to elucidate intrinsic activity. The mass activity of FeCo LDH/NiSe@NF is 180.6 A g−1 at 1.53 V vs. RHE, which is about 6.2 times, 5.0 times and 18.7 times higher than those of Co(OH)2/NiSe@NF, FeCo LDH@NF and NiSe@NF, demonstrating a high OER intrinsic activity of the FeCo LDH/NiSe@NF electrode. The specific activity normalized by ECSA was estimated as shown in Fig. 3f. It could be observed that there is a change in the activity trend, and FeCo LDH@NF and Co(OH)2/NiSe@NF exhibit approximate ECSA-normalized activities. Nevertheless, the intrinsic activity of FeCo LDH/NiSe@NF is still superior to other electrodes, implying that the Fe introduction and heterostructure engineering improve the intrinsic catalytic activity of electrode active sites.
The oxygen production efficiency in 1.0 M KOH was measured by the water drainage method (Fig. S13†). As displayed in Fig. 3g, the faradaic efficiency of FeCo LDH/NiSe@NF toward the OER is close to 100%. The slight loss of faradaic efficiency may be induced by the oxidation of metal species during the OER process as well as the dissolution of part O2 in the electrolyte. The above electrochemical test results strongly show the enhancement of the synergistic activation effect in FeCo LDH/NiSe@NF toward the OER. Consequently, the electrocatalytic activity of our FeCo LDH/NiSe@NF electrode outperforms most of the recently reported OER anodes at large current densities (Fig. 3h and Table S2†). The long-term stability of FeCo LDH/NiSe@NF was further evaluated using the chronopotentiometry (CP) test. As illustrated in Fig. 4, FeCo LDH/NiSe@NF can operate stably without significant degradation for 100 h at either a small current density of 100 mA cm−2 or large current densities of 500 and 1000 mA cm−2. The excellent long-term stability of FeCo LDH/NiSe@NF for OER, especially at large current densities, makes its use possible in industrial water electrolysis.
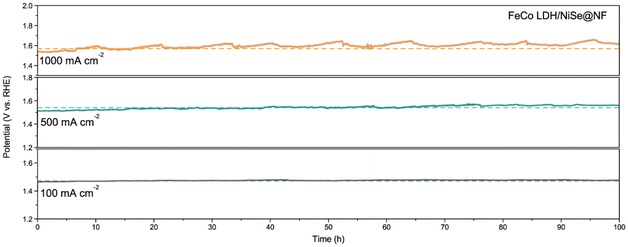 |
| Fig. 4 Chronopotentiometric stability test of the FeCo LDH/NiSe@NF at current densities of 100, 500 and 1000 mA cm−2. | |
The structure and chemical states of FeCo LDH/NiSe@NF after the stability test were analyzed again by SEM, TEM, Raman, and XPS. It is found that the macroscopic nanosheet-nanowire layered array structure is largely preserved (Fig. S14a and b†). Noticeably, according to enlarged SEM images (Fig. S14b1 and b2†), the FeCo LDH nanosheet becomes thicker as abundant wrinkly secondary nanosheets proliferate throughout the nanosheet surface, which offers a larger specific surface area and number of active sites. Additionally, the EDS mapping images in Fig. S14d† further confirm that the lower layer of FeCo LDH/NiSe@NF retains the core–shell structure with a uniform distribution of elements. The Raman spectra are shown in Fig. S15,† and only one broad peak at around 573 cm−1 could be observed after the OER, which is assigned to the M–O vibration mode of MOOH (M = Fe, Co or Ni).52–55 Fig. S16a† shows the high-resolution Ni 2p XPS spectra of FeCo LDH/NiSe@NF before and after the OER test. A couple of new doublet peaks attributed to Ni3+ can be observed, indicating the structural transition from NiSe to NiOOH.52,56 The change in the proportion of EDS elements before and after the reaction also indicates that NiSe is transformed into NiOOH or NiO (Table S1†). The Co 2p XPS spectra indicate that part of Co2+ species were transformed into Co3+ species (Fig. S16b†), which confirms the formation of CoOOH.57,58 Similarly, the Fe 2p XPS spectra (Fig. S16c†) also exhibit increased Fe3+, suggesting the possible reconstruction from FeCo LDH to Fe, Co-OOH, which is consistent with the SEM and Raman results.59,60 In the case of O 1s spectra (Fig. S16e†), the characteristic peak intensity of M–O increases significantly. These characterization results confirm the partial structural surface reconstruction of catalyst active sites into oxyhydroxides during the OER process.
Furthermore, we compared the wettability of the FeCo LDH/NiSe@NF electrode with the NiSe@NF electrode by contact angles (CA) measurement using 10 μL of water droplet. As displayed in Fig. S16a,† NiSe@NF shows a CA of 129°. Note that for FeCo LDH/NiSe@NF, the water droplet spreads out as soon as it comes into contact with the electrode surface, so the photo of CA is not captured (Fig. S17b and Video S1†). The superhydrophilic properties of FeCo LDH/NiSe@NF can facilitate rapid electrolyte penetration and mass transfer, which is conducive to the adsorption of H2O and the release of O2 during the reaction.61,62 Therefore, FeCo LDH/NiSe@NF can maintain rapid catalytic reaction kinetics and remarkable stability for alkaline OER at large current densities.
In view of the excellent catalytic performance of FeCo LDH/NiSe@NF at large current densities, we assembled a two-electrode alkaline electrolyzer for overall water splitting by employing FeCo LDH/NiSe@NF||FeCo LDH/NiSe@NF, Pt/C@NF||IrO2@NF, NiSe@NF||NiSe@NF, and NF||NF as the cathode and anode, respectively. Meanwhile, the hierarchical NiCoP/NiCoSx@NF heterostructure for HER from our previous work63 was also prepared and used as a cathode to construct NiCoP/NiCoSx@NF||FeCo LDH/NiSe@NF. As shown in Fig. 5a, NiCoP/NiCoSx@NF||FeCo LDH/NiSe@NF achieves current densities of 100, 500, and 1000 mA cm−2 at the lowest cell voltages of 1.718, 2.087, and 2.480 V without iR compensation in 1.0 M KOH at 25 °C, which even surpasses those of FeCo LDH/NiSe@NF serving as both anode and cathode, as well as some other reported electrodes (Table S3†). Apart from the above, to satisfy the actual commercial AWE demand (highly concentrated and high temperature alkaline electrolyte), the large-current-density electrocatalytic activities for overall water splitting at 50/80 °C + 30 wt% KOH were also performed. It is noted that the cell voltage of NiCoP/NiCoSx@NF||FeCo LDH/NiSe@NF is significantly lower than that of FeCo LDH/NiSe@NF||FeCo LDH/NiSe@NF under harsh conditions of 50 °C + 30 wt% KOH, demonstrating the better HER activity for NiCoP/NiCoSx (Fig. 5b). And when the electrolyte was heated to 80 °C, it only needed a small cell voltage of 1.849 V to reach the industrial current density of 1000 mA cm−2. Moreover, the NiCoP/NiCoSx@NF||FeCo LDH/NiSe@NF also displays satisfactory stability. As we can see from Fig. 5c, the cell voltage remains stable after continuous water splitting at a current density of 500 mA cm−2 for 200 h under 25 °C + 1.0 M KOH, except for some fluctuations due to vigorous gas generation on both electrodes (Fig. 5c inset).
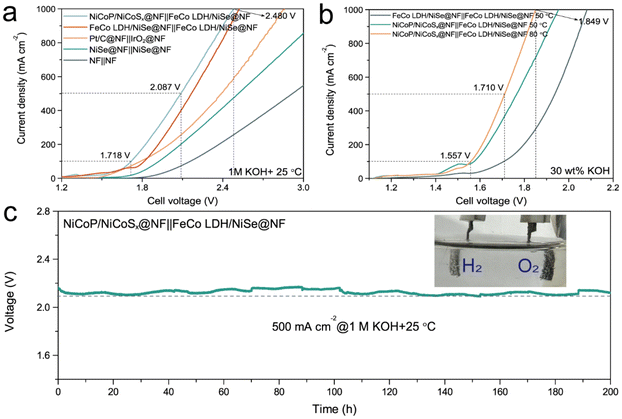 |
| Fig. 5 (a) LSV polarization curves of as-prepared electrodes for overall water splitting at 25 °C in 1.0 M KOH, (b) LSV polarization curves of as-prepared electrodes for overall water splitting at 50/80 °C in 30% KOH, (c) chronopotentiometric test of the NiCoP/NiCoSx@NF||FeCo LDH/NiSe@NF toward water splitting at current density of 500 mA cm−2 (inset: digital photograph of the two-electrode water splitting). | |
To better understand the heterogeneous interface between NiSe and FeCo LDH and the influence of their synergistic coupling on the OER, we conducted density functional theory (DFT) calculations. To simplify the model, the structural models of NiSe, FeCo LDH, Co(OH)2/NiSe and FeCo LDH/NiSe were constructed (Fig. S18†). As shown in Fig. 6a, the charge redistribution at the interface of FeCo LDH/NiSe was analyzed by the charge density difference. It can be found that a strong electron interaction is observed after the formation of the FeCo LDH/NiSe heterostructure. And the charge accumulation/depletion could be readily discernible at the heterointerface, which indicates that there is an obvious electron transfer in the interface region, as also evidenced by Bader charge analysis. As shown in Fig. 6c–e, the Bader charges analysis of the local atoms on NiSe, FeCo LDH and FeCo LDH/NiSe was conducted. The Ni atoms in the NiSe average have 9.62–9.75e− (Table S4†), and the charge number of the Co and Fe atoms in the FeCo LDH are 7.82–7.84e− and 6.57–6.73e− (Table S5†). After the formation of the FeCo LDH/NiSe heterostructure, the charge of Ni, Co and Fe atoms change to 9.65–9.77, 7.77–7.78 and 6.48–6.51e− (loss of 0.23–0.35, 1.22–1.23 and 1.49–1.52e−), respectively (Table S6†). Compared with the original NiSe and FeCo LDHs, the Bader charges of Ni and Se atoms increase at the heterointerface on the NiSe side while the FeCo LDH side loses more charge. This further illustrates the electron transfer from FeCo LDH to NiSe at the interface, which in turn induces the redistribution of electron configuration and facilitates the adsorption of oxygen intermediates.32,64
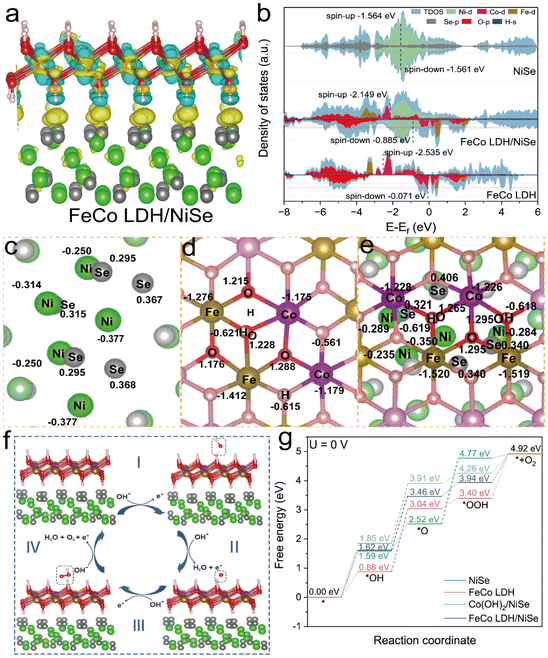 |
| Fig. 6 (a) Charge density difference at the FeCo LDH/NiSe interface (yellow and cyan contours represent charge accumulation and charge depletion, respectively, the isosurface value was set to 0.003 e Bohr−3). (b) The projected density of states of NiSe, FeCo LDH, and FeCo LDH/NiSe. The Bader charge numbers of atoms in (c) NiSe, (d) FeCo LDH and (e) FeCo LDH/NiSe. (f) Four electrons reaction process over optimized structure models with oxygen intermediates (*OH, *O, and *OOH) adsorbed on the surface active sites of FeCo LDH/NiSe for alkaline OER. (g) The Gibbs free energy diagram of NiSe, FeCo LDH, Co(OH)2/NiSe and FeCo LDH/NiSe for OER (U = 0). Atom colors are as follows: Ni in green, Co in purple, Fe in brown, Se in gray, O in red and H in white. | |
The projected density of states (PDOS) of NiSe, FeCo LDH, and FeCo LDH/NiSe were calculated to discern the electronic structure change. Fig. 6b shows obvious metallic characteristics of these three structures, demonstrating high electrical conductivity and fast electron transfer rate, which is favorable for the reduction of the OER overpotential.65 It is found that the coupling of FeCo LDH and NiSe could tune the d-band center, leading to moderate binding strength of intermediates. The d-band center values of NiSe and FeCo LDHs are −1.564 and −2.535 eV, respectively. The d-band center of FeCo LDH/NiSe shifts to −2.149 eV, indicating that the adsorption interaction between reaction intermediates and FeCo LDH/NiSe is neither too weak nor too strong.66 The Gibbs free energy was then calculated to verify that and the corresponding four-electrons OER reaction pathways over the surface of optimized structures with oxygen intermediates (*OH, *O, and *OOH) are illustrated in Fig. 6f and Fig. S19–21.†Fig. 6g demonstrates the calculated OER free energy diagram for various electrocatalysts based on the adsorption evolution mechanism (AEM). It indicated that the rate-determining step (RDS) is the formation of *OOH from *O (ΔG3) for NiSe, which has an energy barrier of 2.25 eV. Notably, FeCo LDH, Co(OH)2/NiSe and FeCo LDH/NiSe all show the same RDS of *OH deprotonation to *O intermediates with the reduced energy barriers (ΔG2) of 2.16, 2.06 and 1.85 eV, respectively. Obviously, FeCo LDH/NiSe can theoretically provide the best electrocatalytic activity with the thermodynamic OER overpotential value of 0.62 V, which is significantly lower than those of the NiSe (1.02 V) and FeCo LDH (0.93 V) alone. This emphasizes that the heterogeneous interface cooperation between FeCo LDH and NiSe effectively optimizes the adsorption of H2O and thus reduces the energy barrier of RDS for the OER process.
4 Conclusion
In brief, we have successfully developed a two-step hydrothermal–electrodeposition method for the synthesis of a FeCo LDH/NiSe heterostructure on Ni foam with abundant heterogeneous interfaces, which serves as a robust electrocatalyst for efficient large-current-density alkaline water splitting. The unique hierarchical nanosheet–nanowire 3D heterostructure allows it to have a large number of surface active sites. And as expected, the synergistic coupling interaction between FeCo LDH and NiSe helps to tune the charge redistribution and electronic structure, thereby accelerating the reaction kinetics and reducing the energy barrier of the RDS in the OER process. The as-prepared FeCo LDH/NiSe@NF displays excellent catalytic performance, achieving low overpotentials of 230, 266 and 298 mV at 100, 500 and 1000 mA cm−2, respectively, and long-term stability for 100 h. More importantly, the two-electrode cell assembled with FeCo LDH/NiSe@NF and NiCoP/NiCoSx@NF as the anode and cathode only needs a cell voltage of 1.849 V to reach 1000 mA cm−2 under industrial conditions of 30 wt% KOH + 80 °C. Based on these results, FeCo LDH/NiSe@NF could be considered a promising candidate for industrial water splitting applications.
Data availability
The data that support the findings of this study are available on request from the corresponding author upon reasonable request.
Conflicts of interest
The authors declare that they have no known competing financial interests or personal relationships that could have appeared to influence the work reported in this work.
Acknowledgements
This work is supported by the Research Funds of the Institute of Zhejiang University-Quzhou (IZQ2022RCZX017) and the City Science and Technology Research Project of Quzhou (2023K008).
References
- Z. Y. Yu, Y. Duan, X. Y. Feng, X. Yu, M. R. Gao and S. H. Yu, Clean and Affordable Hydrogen Fuel from Alkaline Water Splitting: Past, Recent Progress, and Future Prospects, Adv. Mater., 2021, 33, 2007100 CrossRef CAS.
- L. Wan, Z. Xu, Q. Xu, M. Pang, D. Lin, J. Liu and B. Wang, Key components and design strategy of the membrane electrode assembly for alkaline water electrolysis, Energy Environ. Sci., 2023, 16, 1384–1430 RSC.
- H. Sun, X. Xu, H. Kim, W. Jung, W. Zhou and Z. Shao, Electrochemical Water Splitting: Bridging the Gaps Between Fundamental Research and Industrial Applications, Energy Environ. Mater., 2023, 6, e12441 CrossRef CAS.
- Y. Luo, Z. Zhang, M. Chhowalla and B. Liu, Recent Advances in Design of Electrocatalysts for High-Current-Density Water Splitting, Adv. Mater., 2021, 34, 2108133 CrossRef.
- Q. Shao, P. Wang and X. Huang, Opportunities and Challenges of Interface Engineering in Bimetallic Nanostructure for Enhanced Electrocatalysis, Adv. Funct. Mater., 2019, 29, 1806419 CrossRef.
- D. Zheng, L. Yu, W. Liu, X. Dai, X. Niu, W. Fu, W. Shi, F. Wu and X. Cao, Structural advantages and enhancement strategies of heterostructure water-splitting electrocatalysts, Cell Rep. Phys. Sci., 2021, 2, 100443 CrossRef CAS.
- X. Peng, Y. Yana, X. Jin, C. Huang, W. Jin, B. Gao and P. K. Chu, Recent advance and prospectives of electrocatalysts based on transition metal selenides for efficient water splitting, Nano Energy, 2020, 78, 105234 CrossRef CAS.
- X. Xia, L. Wang, N. Sui, V. L. Colvin and W. W. Yu, Recent progress in transition metal selenide electrocatalysts for water splitting, Nanoscale, 2020, 12, 12249 RSC.
- R. Balaji, T. T. Nguyen, K. Harish, N. H. Kim and J. H. Lee, Modulating heterointerfaces of tungsten incorporated CoSe/Co3O4 as a highly efficient electrocatalyst for overall water splitting, J. Mater. Chem. A, 2022, 10, 3782–3792 RSC.
- Q. Zhang, W. Zhang, J. Zhu, X. Zhou, G. Xu, D. Chen, Z. Wu and L. Wang, Tuning d-p Orbital Hybridization of NiMoO4@Mo15Se19/NiSe2 Core-Shell Nanomaterials via Asymmetric Coordination Interaction Enables theWater Oxidation Process, Adv. Energy Mater., 2024, 14, 230546 Search PubMed.
- J. Yu, F. Yu, M. F. Yuen and C. Wang, Two-dimensional layered double hydroxides as a platform for electrocatalytic oxygen evolution, J. Mater. Chem. A, 2021, 9, 9389 RSC.
- S. Jiang, M. Zhang, C. Xu, G. Liu, K. Zhang, Z. Zhang, H. Q. Peng, B. Liu and W. Zhang, Recent Developments in Nickel-Based Layered Double Hydroxides for Photo(-/)electrocatalytic Water Oxidation, ACS Nano, 2024, 18, 16413–16449 CrossRef CAS PubMed.
- Z. Gao, H. Ma, Q. Wang, J. Feng, X. Duan and L. Dianqing, Structure Engineering of Layered Double Hydroxides (LDHs) for Heterogeneous Catalysis, Chem. Res. Chin. Univ., 2024, 40, 590–610 CrossRef CAS.
- H. Wang, H. Wang, Z. Wang, J. Qiu, B. Wang, R. Guo, J. You, X. Lei and X. Liu, Recent advances in NiFe layered double hydroxide electrocatalysts for seawater oxidation, J. Alloys Compd., 2024, 1002, 175368 CrossRef CAS.
- Y. Gong, H. Zhao, Y. Sun, D. Xu, D. Ye, Y. Tang, T. He and J. Zhang, Partially selenized FeCo layered double hydroxide as bifunctional electrocatalyst for efficient and
stable alkaline (sea)water splitting, J. Colloid Interface Sci., 2023, 650, 636–647 CrossRef CAS PubMed.
- Q. Wen, K. Yang, D. Huang, G. Cheng, X. Ai, Y. Liu, J. Fang, H. Li, L. Yu and T. Zhai, Schottky Heterojunction Nanosheet Array Achieving High–Current–Density Oxygen Evolution for Industrial Water Splitting Electrolyzers, Adv. Energy Mater., 2021, 11, 2102353 CrossRef CAS.
- X. Lu, H. Xue, H. Gong, M. Bai, D. Tang, R. Ma and T. Sasaki, 2D Layered Double Hydroxide Nanosheets and Their Derivatives Toward Efficient Oxygen Evolution Reaction, Nano-Micro Lett., 2020, 12, 86 CrossRef CAS.
- L. Yu, H. Zhou, J. Sun, F. Qin, F. Yu, J. Bao, Y. Yu, S. Chen and Z. Ren, Cu nanowires shelled with NiFe layered double hydroxide nanosheets as bifunctional electrocatalysts for overall water splitting, Energy Environ. Sci., 2017, 10, 1820–1827 RSC.
- P. Zhai, C. Wang, Y. Zhao, Y. Zhang, J. Gao, L. Sun and J. Hou, Regulating electronic states of nitride/hydroxide to accelerate kinetics for oxygen evolution at large current density, Nat. Commun., 2023, 14, 1873 CrossRef CAS.
- G. Kresse and J. Hafner, Ab initiomolecular dynamics for liquid metals, Phys. Rev. B: Condens. Matter Mater. Phys., 1993, 47, 558–561 CrossRef CAS.
- G. Kresse and J. Hafner, Ab initiomolecular-dynamics simulation of the liquid-metal–amorphous-semiconductor transition in germanium, Phys. Rev. B: Condens. Matter Mater. Phys., 1994, 49, 14251–14269 CrossRef CAS PubMed.
- G. Kresse and D. Joubert, From ultrasoft pseudopotentials to the projector augmented-wave method, Phys. Rev. B: Condens. Matter Mater. Phys., 1999, 59, 1758–1775 CrossRef CAS.
- J. P. Perdew, K. Burke and M. Ernzerhof, Generalized Gradient Approximation Made Simple, Phys. Rev. Lett., 1996, 77, 3865–3868 CrossRef CAS PubMed.
- P. E. Blochl, Projector augmented-wave method, Phys. Rev. B: Condens. Matter Mater. Phys., 1994, 50, 17953–17979 CrossRef.
- R. Li, D. Zhang, H. Chen, S. Wang, Y. Ling and C. Fang, Morphological and Electronic Regulation of Zn,S-Incorporated FeCo–LDH Nanosheets for Boosting the Bi-Electrocatalytic Oxygen Evolution and Urea Oxidation Reactions, ACS Sustainable Chem. Eng., 2023, 11(45), 16098–16107 CrossRef CAS.
- Y. Gan, Z. Li, Y. Ye, X. Dai, F. Nie, X. Yin, Z. Ren, B. Wu, Y. Cao, R. Cai, X. Zhang and W. Song, Doping Mo into NiFe LDH/NiSe Heterostructure to Enhance Oxygen Evolution Activity by Synergistically Facilitating Electronic Modulation and Surface Reconstruction, ChemSusChem, 2022, 15, e202201205 CrossRef CAS.
- F. Zhang, X. Wang, W. Han, Y. Qian, L. Qiu, Y. He, L. Lei and X. Zhang, The Synergistic Activation of Ce-Doping and CoP/Ni3P Hybrid Interaction for Efficient Water Splitting at Large-Current-Density, Adv. Funct. Mater., 2022, 33, 2212381 CrossRef.
- J. Wang, J. Huang, G. Chen, W. Chen, T. Li, A. Meng and K. Ostrikov, In-situ engineered heterostructured nickel tellur-selenide nanosheets for robust overall water splitting, Chem. Eng. J., 2022, 446, 137297 CrossRef CAS.
- S. Ni, H. Qu, Z. Xu, X. Zhu, H. Xing, L. Wang, J. Yu, H. Liu, C. Chen and L. Yang, Interfacial engineering of the NiSe2/FeSe2 p-p heterojunction for promoting oxygen evolution reaction and electrocatalytic urea oxidation, Appl. Catal., B, 2021, 299, 120638 CrossRef CAS.
- H. Fan, D. Jiao, J. Fan, D. Wang, B. Zaman, W. Zhang, L. Zhang, W. Zheng and X. Cui, Kinetically and thermodynamically expediting elementary steps via high-valence Cr-incorporated of nickel selenide for water electrolysis, Nano Res., 2023, 17, 1199–1208 CrossRef.
- J. Zhou, L. Yuan, J. Wang, L. Song, Y. You, R. Zhou, J. Zhang and J. Xu, Combinational modulations of NiSe2 nanodendrites by phase engineering and iron-doping towards an efficient oxygen evolution reaction, J. Mater. Chem. A, 2020, 8, 8113 RSC.
- Y. Yang, Y. Kang, H. Zhao, X. Dai, M. Cui, X. Luan, X. Zhang, F. Nie, Z. Ren and W. Song, An Interfacial Electron Transfer on Tetrahedral NiS(2) /NiSe(2) Heterocages with Dual-Phase Synergy for Efficiently Triggering the Oxygen Evolution Reaction, Small, 2020, 16, e1905083 CrossRef PubMed.
- L. Tan, J. Yu, H. Wang, H. Gao, X. Liu, L. Wang, X. She and T. Zhan, Controllable synthesis and phase-dependent catalytic performance of dual-phase nickel selenides on Ni foam for overall water splitting, Appl. Catal., B, 2022, 303, 120915 CrossRef CAS.
- G. Zhu, X. Xie, X. Li, Y. Liu, X. Shen, K. Xu and S. Chen, Nanocomposites Based on CoSe2-Decorated FeSe2 Nanoparticles Supported on Reduced Graphene Oxide as High-Performance Electrocatalysts toward Oxygen Evolution Reaction, ACS Appl. Mater. Interfaces, 2018, 10, 19258–19270 CrossRef CAS.
- S. Xu, J. Hu, L. Huang, Y. Liu, X. Zheng and D. Jiang, Anchoring RuSe2 on CoSe2 nanoarrays as a hybrid catalyst for efficient and robust oxygen evolution reaction, J. Colloid Interface Sci., 2022, 615, 327–334 CrossRef CAS PubMed.
- S. Xiao, X. Li, W. Zhang, Y. Xiang, T. Li, X. Niu, J. S. Chen and Q. Yan, Bilateral interfaces in In2Se3-CoIn2-CoSe2 heterostructures boosting fast and robust sodium storage properties in MOF-derived hollow nanorods, ACS Nano, 2021, 15, 13307–13318 CrossRef CAS.
- C. Wang, M. Zhu, Z. Cao, P. Zhu, Y. Cao, X. Xu, C. Xu and Z. Yin, Heterogeneous bimetallic sulfides based seawater electrolysis towards stable industrial-level large current density, Appl. Catal., B, 2021, 291, 120071 CrossRef CAS.
- F. Yu, H. Zhou, Y. Huang, J. Sun, F. Qin, J. Bao, W. A. Goddard, S. Chen and Z. Ren, High-performance bifunctional porous non-noble metal phosphide catalyst for overall water splitting, Nat. Commun., 2018, 9, 2551 CrossRef PubMed.
- H. Zhou, F. Yu, Q. Zhu, J. Sun, F. Qin, L. Yu, J. Bao, Y. Yu, S. Chen and Z. Ren, Water splitting by electrolysis at high current densities under 1.6 volts, Energy Environ. Sci., 2018, 11, 2858–2864 RSC.
- G. Deng, J. Zhao, H. Hu, X. Wang, M. Zhu and Y. Feng, FeOOH–CoS composite catalyst with high catalytic performances for oxygen evolution reaction at high current density, Int. J. Hydrogen Energy, 2021, 46, 37333–37339 CrossRef CAS.
- G. Qian, J. Chen, T. Yu, J. Liu, L. Luo and S. Yin, Three-Phase Heterojunction NiMo-Based Nano-Needle for Water Splitting at Industrial Alkaline Condition, Nano-Micro Lett., 2022, 14, 20 CrossRef CAS.
- G. Qian, J. Chen, T. Yu, L. Luo and S. Yin, N-Doped Graphene-Decorated NiCo Alloy Coupled with Mesoporous NiCoMoO Nano-sheet Heterojunction for Enhanced Water Electrolysis Activity at High Current Density, Nano-Micro Lett., 2021, 13, 77 CrossRef PubMed.
- L. Wu, L. Yu, F. Zhang, B. McElhenny, D. Luo, A. Karim, S. Chen and Z. Ren, Heterogeneous Bimetallic Phosphide Ni2P-Fe2P as an Efficient Bifunctional Catalyst for Water/Seawater Splitting, Adv. Funct. Mater., 2020, 31, 2006484 CrossRef.
- X. Mu, X. Gu, S. Dai, J. Chen, Y. Cui, Q. Chen, M. Yu, C. Chen, S. Liu and S. Mu, Breaking the symmetry of single-atom catalysts enables an extremely low energy barrier and high stability for large-current-density water splitting, Energy Environ. Sci., 2022, 15, 4048–4057 RSC.
- X. Zhang, H. Yi, M. Jin, Q. Lian, Y. Huang, Z. Ai, R. Huang, Z. Zuo, C. Tang, A. Amini, F. Jia, S. Song and C. Cheng, In Situ Reconstructed Zn doped Fe(x)Ni(1(-x)OOH Catalyst for Efficient and Ultrastable Oxygen Evolution Reaction at High Current Densities, Small, 2022, 18, e2203710 CrossRef PubMed.
- X. Zhang, F. Li, Y. Dong, B. Dong, F. Dai, C. Liu and Y. Chai, Dynamic anion regulation to construct S-doped FeOOH realizing 1000 mA cm−2-level-current-density oxygen evolution over 1000 h, Appl. Catal., B, 2022, 315, 121571 CrossRef CAS.
- R. Andaveh, A. S. Rouhaghdam, J. Ai, M. Maleki, K. Wang, A. Seif, G. B. Darband and J. Li, Boosting the electrocatalytic activity of NiSe by introducing MnCo as an efficient heterostructured electrocatalyst for large-current-density alkaline seawater splitting, Appl. Catal., B, 2023, 325, 122355 CrossRef CAS.
- Y. Qian, F. Zhang, L. Qiu, W. Han, Z. Zeng, L. Lei, Y. He, P. Li and X. Zhang, Optimizing electronic structure of NiFe LDH with Mn-doping and Fe0.64Ni0.36 alloy for alkaline water oxidation under industrial current density, Nano Res., 2023, 16, 8953–8960 CrossRef CAS.
- X. Y. Wang, M. Zhu, Q. N. Bian, B. S. Guo, W. Q. Kong, C. B. Wang and Y. Y. Feng, Fe-Based Metal–Organic Framework-Ni3S2 Heterostructure Nanocomposites for Efficient Oxygen Evolution Reaction at High Current Densities, ACS Appl. Nano Mater., 2023, 6, 5200–5210 CrossRef CAS.
- Q. N. Bian, B. S. Guo, D. X. Tan, D. Zhang, W. Q. Kong, C. B. Wang and Y. Y. Feng, Constructing CoNi-LDH/Fe MOF/NF Heterostructure Catalyst for Energy-Efficient OER and UOR at High Current Density, ACS Appl. Mater. Interfaces, 2024, 16, 14742–14749 CrossRef CAS.
- J. Liu, W. Du, S. Guo, J. Pan, J. Hu and X. Xu, Iron-Locked Hydr(oxy)oxide Catalysts via Ion-Compensatory Reconstruction Boost Large-Current-Density Water Oxidation, Adv. Sci., 2023, 10, e2300717 CrossRef PubMed.
- J. Song, Y. Chen, H. Huang, J. Wang, S. C. Huang, Y. F. Liao, A. E. Fetohi, F. Hu, H. Y. Chen, L. Li, X. Han, K. M. El-Khatib and S. Peng, Heterointerface Engineering of Hierarchically Assembling Layered Double Hydroxides on Cobalt Selenide as Efficient Trifunctional Electrocatalysts for Water Splitting and Zinc–Air Battery, Adv. Sci., 2022, 9, 2104522 CrossRef CAS PubMed.
- C. Li, L. Xie, J. Zhao, L. Gu, J. Wu and G. Li, Interfacial electronic modulation by Fe2O3/NiFe-LDHs heterostructures for efficient oxygen evolution at high current density, Appl. Catal., B, 2022, 306, 121097 CrossRef CAS.
- S. Lee, Y. C. Chu, L. Bai, H. M. Chen and X. Hu, Operando identification of a side-on nickel superoxide intermediate and the mechanism of oxygen evolution on nickel oxyhydroxide, Chem Catal., 2023, 3, 100475 CrossRef CAS.
- S. Wang, B. Xu, W. Huo, H. Feng, X. Zhou, F. Fang, Z. Xie, J. K. Shang and J. Jiang, Efficient FeCoNiCuPd thin-film electrocatalyst for alkaline oxygen and hydrogen evolution reactions, Appl. Catal., B, 2022, 313, 121472 CrossRef CAS.
- X. Li, G. Q. Han, Y. R. Liu, B. Dong, W. H. Hu, X. Shang, Y. M. Chai and C. G. Liu, NiSe@NiOOH Core-Shell Hyacinth-like Nanostructures on Nickel Foam Synthesized by in Situ Electrochemical Oxidation as an Efficient Electrocatalyst for the Oxygen Evolution Reaction, ACS Appl. Mater. Interfaces, 2016, 8, 20057–20066 CrossRef CAS PubMed.
- P. Guo, J. Wu, X. B. Li, J. Luo, W. M. Lau, H. Liu, X. L. Sun and L. M. Liu, A highly stable bifunctional catalyst based on 3D Co(OH)2@NCNTs@NF towards overall water-splitting, Nano Energy, 2018, 47, 96–104 CrossRef CAS.
- X. Yang, J. Cheng, Y. Xu, H. Li, W. Tu and J. Zhou, Heterogeneous ultra-thin FeCo-LDH@Co(OH)2 nanosheets facilitated electrons transfer for oxygen evolution reaction, Chem. Eng. J., 2023, 472, 145076 CrossRef CAS.
- Y. Huang, X. Chen, S. Ge, Q. Zhang, X. Zhang, W. Li and Y. Cui, Hierarchical FeCo2S4@CoFe layered double hydroxide on Ni foam as a bifunctional electrocatalyst for overall water splitting, Catal. Sci. Technol., 2020, 10, 1292–1298 RSC.
- S. Niu, W. J. Jiang, Z. Wei, T. Tang, J. Ma, J. S. Hu and L. J. Wan, Se-Doping Activates FeOOH for Cost-Effective and Efficient Electrochemical Water Oxidation, J. Am. Chem. Soc., 2019, 141, 7005–7013 CrossRef CAS.
- Z. Wang, E. Song, N. Wang and J. Dong, Manipulation on active electronic states of metastable phase β-NiMoO4 for large current density hydrogen evolution, Nat. Commun., 2021, 12, 5960 CrossRef CAS.
- J. Ren, L. Chen, H. Wang, W. Tian, S. Zhai, Y. Feng and Z. Yuan, Modulating interfacial charge distribution of Ni2P-NiSe2 by multiple interface engineering for accelerating water splitting with industry-level activity and stability, Appl. Catal., B, 2024, 347, 123817 CrossRef CAS.
- W. Han, F. Zhang, L. Qiu, Y. Qian, S. Hao, P. Li, Y. He and X. Zhang, Interface engineering of hierarchical NiCoP/NiCoS(x) heterostructure arrays for efficient alkaline hydrogen evolution at large current density, Nanoscale, 2022, 14, 15498–15506 RSC.
- Z. Yu, H. Yao, Y. Yang, M. Yuan, C. Li, H. He, T.-S. Chan, D. Yan, S. Ma, P. Zapol and M. G. Kanatzidis, MoOxSy/Ni3S2 Microspheres on Ni Foam as Highly Efficient, Durable Electrocatalysts for Hydrogen Evolution Reaction, Chem. Mater., 2022, 34, 798–808 CrossRef CAS.
- S. Sun, X. Zhou, B. Cong, W. Hong and G. Chen, Tailoring the d-Band Centers Endows (NixFe1−x)2P Nanosheets with Efficient Oxygen Evolution Catalysis, ACS Catal., 2020, 10, 9086–9097 CrossRef CAS.
- J. Y. Xue, F. L. Li, B. B. Chen, H. B. Geng, W. Zhang, W. Y. Xu, H. W. Gu, P. Braunstein and J. P. Lang, Engineering multiphasic MoSe2/NiSe heterostructure interfaces for superior hydrogen production electrocatalysis, Appl. Catal., B, 2022, 312, 121434 CrossRef CAS.
|
This journal is © the Partner Organisations 2025 |
Click here to see how this site uses Cookies. View our privacy policy here.