DOI:
10.1039/D4QM00636D
(Research Article)
Mater. Chem. Front., 2025,
9, 55-64
Asymmetric deep-blue tetrafluorobenzene-bridged fluorophores with hybridized local and charge-transfer characteristics for efficient OLEDs with low efficiency roll-off†
Received
28th July 2024
, Accepted 21st October 2024
First published on 23rd October 2024
Abstract
High dynamic range technology places greater demands on organic light-emitting diode (OLED) displays, particularly blue emitters, which face significant challenges in meeting the wide-color-gamut BT.2100 standard and achieving high efficiency at high brightness. Here, we propose a design strategy for constructing bipolar deep-blue materials by combining an asymmetric donor–acceptor–donor′ (D–A–D′) type structure with a novel tetrafluorobenzene acceptor. The resulting molecules feature typical hybridized local and charge-transfer state characteristics, with high oscillator strengths, achieving high fluorescence efficiencies exceeding 80% and fast radiative rates that surpass 6 × 108 s−1. Consequently, the doped device emits a deep-blue light with color coordinates of (0.159, 0.048), and demonstrates a maximum external quantum efficiency (EQE) of 6.35%, maintaining efficiencies of 5.95% and 5.61% at 500 and 1000 cd m−2, respectively. Remarkably, the non-doped OLED boasts a superior EQE of 7.44%, retaining an impressive 6.99% even at 1000 cd m−2 and maintaining a high 6.19% up to a brightness of 10
000 cd m−2, demonstrating minimal efficiency roll-off. These findings underscore the great potential of the tetrafluorobenzene-based D–A–D′ type molecular design strategy in developing efficient blue materials and their optoelectronic applications.
Introduction
Organic light-emitting diode (OLED), is revolutionizing the world of display technology, due to its superiorities over traditional display technology, with advantages including low power consumption, exceptional image quality, lightweight flexibility, and so on,1–5 making it applicable for a wide range of applications, from smartphones and televisions to large-screen displays and beyond. To deliver a more immersive and intricate visual experience, high dynamic range (HDR) technology has placed stringent demands on OLED displays, primarily focusing on achieving a broad color gamut that adheres to the International Telecommunication Union's 2016 Broadcast Service Television 2100 (BT.2100) standard, as well as ensuring high efficiency under intense brightness conditions (known as low efficiency roll-off). This is intimately tied to the inherent properties of organic electroluminescent (EL) materials, which ultimately dictate the ceiling of device performance. However, despite their inherently wide bandgap, achieving efficient deep-blue emitters that comply with the BT.2100 blue standard, corresponding to the Commission Internationale de l’Éclairage (CIE) coordinates of (0.131, 0.046), while maintaining low efficiency roll-off at high brightness, poses a formidable challenge in comparison to red and green emitters.6–9
Donor–acceptor (D–A) type molecules, containing an electron-rich donor (D) and an electron-deficient acceptor (A), have emerged as a prevalent strategy to streamline hole/electron injection and transport in emitters. This design not only broadens the exciton recombination zone but also mitigates exciton quenching effects, ultimately alleviating the efficiency roll-off in OLEDs, enhancing their overall performance. The intramolecular charge transfer (CT) state occurring in D–A type emitters can also promote the conversion from nonradiative triplet excitons to radiative singlet excitons through the reverse intersystem crossing (RISC) or high-lying RISC processes, as seen in thermally activated delayed fluorescence (TADF) and hybridized local and charge-transfer (HLCT) emitters.10–16 Furthermore, the photoluminescence quantum yield (PLQY) of the D–A type emitter is one of the most important parameters for achieving efficient deep-blue OLEDs. Typically, achieving a high PLQY necessitates a rapid exciton decay process, concurrently orchestrated by both singlet radiative and non-radiative transitions. For the former to be effective, a substantial oscillator strength is essential, which can be augmented by extending the conjugation length via enhancements in molecular rigidity and coplanarity. However, this extension may inadvertently induce a minor bathochromic shift. Conversely, in the pursuit of deep-blue emission, the conjugation lengths of chromophores must be restricted, which unfortunately degrades the oscillator strength and PLQY. High luminescence efficiency and wide-bandgap deep-blue emission are two contradictory factors that exhibit contrary dependence on the conjugation extension. Therefore, to unlock the potential of efficient deep-blue emitters and OLEDs, it is important to adopt an appropriate molecular design strategy to break this trade-off.
Several successful strategies have been developed to aim to improve the PLQYs, for example, adjusting the number and positions of substituents, employing an intramolecular-lock strategy,17,18 introducing some particular electron groups with heteroatoms (N, O, S),14,19,20 and preventing intermolecular aggregation and stacking.9,13 Typically, HLCT molecules are characterized by highly mixed or hybridized CT and local excited (LE) states.10–16 Notably, the LE state possesses a large transition moment, contributing to considerable fluorescence efficiency, while the CT state ensures an effective conversion from triplet excitons to singlets enhancing the triplet exciton utilization. This combination renders HLCT molecules highly appealing for potential applications in blue and deep-blue OLEDs. To date, various acceptor moieties including triazole,19,21–23 benzonitrile,18,24,25 diphenylsulfone,26 oxazole,27 and imidazole12,13,28–32 have been applied to construct efficient deep-blue HLCT materials and devices. However, HLCT-OLEDs that either approach or fulfill the BT.2100 standard's blue gamut are notably scarce, particularly when it comes to sustaining a low efficiency roll-off, which remains a formidable challenge.
In our previous work, a deep-blue HLCT emitter, DCZ2F,33 with an EQE of 5.62% and CIE coordinates of (0.163, 0.035), was reported based on a novel p-difluorobenzene acceptor. Its fluorination not only enhanced orbital overlaps, leading to a high fluorescence efficiency, but also contributed to the electron injection and transportation in the EL process. Subsequently, a new HLCT emitter (PICZ2F) with a maximum EQE of 6.16% and a high value of 5.80% at a high brightness of 15
000 cd m−2 was achieved by integrating an asymmetric donor–acceptor–donor′ (D–A–D′) molecule design strategy.34 Its large oscillator strength (1.2987), fast radiation transition rate (kr, 4.97 × 108 s−1) and bipolar transporting ability facilitated the low efficiency roll-off. On their basis, here we further replaced a difluoro-substituted acceptor with tetrafluoro-substituted benzene to construct an asymmetric D–A–D′ type molecular system, where tetrafluorobenzene was employed as a novel acceptor bridging phenylcarbazole and phenanthroimidazole donors. As a result, the target materials 2-(4′′-(9H-carbazol-9-yl)-2′,3′,5′,6′-tetrafluoro-[1,1′:4′,1′′-terphenyl]-4-yl)-1-phenyl-1H-phenanthro[9,10-d]imidazole (PICZ4F) and 1-phenyl-2-(2′,3′,5′,6′-tetrafluoro-4′-(9-phenyl-9H-carbazol-3-yl)-[1,1′-biphenyl]-4-yl)-1H-pphenanthro[9,10-d]imidazole (PI3CZ4F) were designed and synthesized by regulating the connection positions of the phenylcarbazole unit. They both not only exhibit high oscillator strengths and rapid krs, but also possess exceptional bipolar transporting abilities. Consequently, in the doped devices, the EQEs of over 6.3% with CIE coordinates of (0.159, 0.049) and (0.159, 0.048) were achieved based on PICZ4F and PI3CZ4F. Interestingly enough, due to the higher PLQY and good carrier mobilities, the PI3CZ4F-based non-doped device achieved a better EQE value of 7.44% and a small efficiency roll-off with EQEs of 6.99% and 6.19% at high brightness levels of 1000 and 10
000 cd m−2, which provides the possibility for OLED applications under high brightness conditions.
Results and discussion
Molecular design and synthesis
The target molecules of PICZ4F and PI3CZ4F were designed with a D–A–D′ type architecture. Here, 1,2,4,5-tetrafluorobenzene, a weak electron-withdrawing moiety, served as a novel acceptor (A), while rigid planar phenanthroimidazole with good thermal stability and fluorescence efficiency, a typical HLCT building group, was utilized as the donor (D). Additionally, phenylcarbazole, a weak electron donor, was chosen as the second donor (D′) (Fig. 1a). Phenanthroimidazole was para-linked with the tetrafluorobenzene acceptor to expand the π-conjugation length and reduce non-radiation energy loss. Meanwhile, the phenylcarbazole unit was incorporated with tetrafluorobenzene at the para-position of the benzene ring and the C3-position of the carbazole for PICZ4F and PI3CZ4F, respectively. The existence of multiple C–H⋯F non-covalent interactions formed between the F atom and the H atom of adjacent benzenes can effectively promote the delocalization of electrons and charge transfer transitions,35,36 thereby enhancing both the PLQY and bipolar transporting ability.33,37–39 The synthetic routes for PICZ4F and PI3CZ4F are outlined in Scheme S1 (ESI†). Both PICZ4F and PI3CZ4F were synthesized via the tetrakis(triphenylphosphine)palladium catalyzed Suzuki coupling reaction, achieving yields exceeding 80%. Following purification through column chromatography and recrystallization in chloroform, their molecular structures were further characterized using 1H and 13C nuclear magnetic resonance (NMR) spectroscopy and high-resolution mass spectrometry. The detailed experimental process, synthesis route, and characterization data are shown in the Experimental section (ESI†). As shown in Fig. S1 (ESI†), the thermal decomposition temperatures with 5% weight loss are as high as 446 and 471 °C for PICZ4F and PI3CZ4F, respectively; PI3CZ4F has a high glass transition temperature (Tg) of 153.2 °C, while no obvious Tg is observed for PICZ4F in the temperature range from 30 to 300 °C. These results demonstrate that both of them exhibit good thermal stability, fulfilling the prerequisite for the preparation of OLEDs through thermal evaporation.
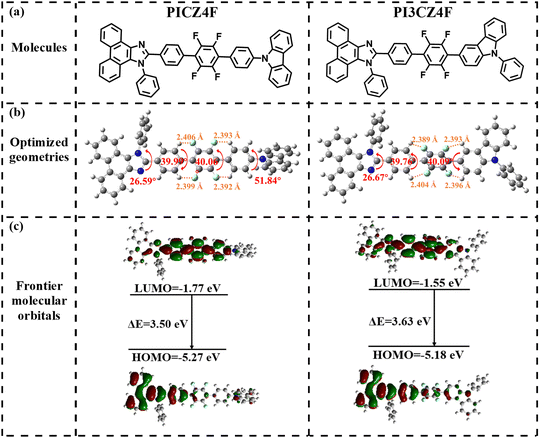 |
| Fig. 1 Molecular structures (a), geometries in the ground state (b), and HOMO and LUMO distributions and calculated energy levels (c) for PICZ4F and PI3CZ4F. | |
Theoretical calculation
To gain insight into the ground state electronic structures of PICZ4F and PI3CZ4F, the optimization of molecular configuration and frontier molecular orbital (FMO) distributions were performed using density functional theory (DFT) calculations at the B3LYP/6-31G(d,p) basis set. As depicted in Fig. 1b, both PICZ4F and PI3CZ4F exhibit moderately distorted molecular conformations, characterized by twist angles of approximately 40° between the tetrafluorobenzene and neighboring groups. Additionally, the angles between the imidazole and linked benzene in the phenanthroimidazole unit are 26.59° for PICZ4F and 26.67° for PI3CZ4F, respectively. The calculated distances between F atoms and adjacent benzene H atoms in PICZ4F and PI3CZ4F range from 2.389 to 2.406 Å, falling below the sum of their van der Waals radii (2.55 Å). This suggests the presence of four intramolecular C–H⋯F non-covalent interactions in both molecules, which contribute to increased molecular rigidity and, consequently, enhanced fluorescence efficiency. Furthermore, the analysis of reduced density gradient (RDG) isosurface regions for the optimized geometries of the ground state reveals the presence of obvious intramolecular C–H⋯F non-covalent interactions (Fig. S2, ESI†).35 As shown in Fig. 1c, the highest occupied molecular orbital (HOMO) distributions of the two isomers exhibit similarities, with the majority concentrated on the phenanthroimidazole unit and a residual presence on the tetrafluorobenzene. Whereas, the lowest unoccupied molecular orbitals (LUMOs) are predominantly delocalized on the tetrafluorobenzene acceptor, imidazole and neighboring benzene rings, exhibiting substantial orbital overlaps with the proportions of 52.94% and 61.74% for PICZ4F and PI3CZ4F, facilitating a rapid radiative-transition rate. Compared to PICZ4F, PI3CZ4F shows slightly shallower HOMO and LUMO energy levels, corresponding to a larger bandgap of 3.63 eV, due to the large dihedral angles in the A–D′ segment.
The natural transition orbitals (NTOs) of singlet and triplet excited states for PICZ4F and PI3CZ4F were further investigated using time-dependent DFT calculations. As depicted in Fig. S3 (ESI†), during the transition from the ground state to lowest singlet state (S1), the “hole” and “particle” distributions of PICZ4F and PI3CZ4F exhibit partial overlap on the imidazole ring, tetrafluorobenzene and adjacent groups, accompanied by partial separation on phenanthrene and carbazole segments. This pattern is indicative of typical hybrid locally excited and charge transfer (HLCT) characteristics,11,12 with high oscillator strengths of 1.1738 for PICZ4F and 1.3908 for PI3CZ4F (see Tables S1 and S2, ESI†). The calculated energy splits (ΔES1T1) between the lowest triplet state (T1) and S1 for PICZ4F and PI3CZ4F are 0.60 and 0.69 eV, respectively, hindering the RISC process from T1 to S1. Nevertheless, the energy splits between S1 and high-lying triplet states (T3 for PICZ4F with a HLCT state characteristic, T4 for PI3CZ4F with a LE state characteristic) are notably small, at 0.11 and 0.02 eV, respectively, coupled with high spin–orbit coupling (SOC) matrix elements of 0.117 and 0.139 cm−1 (Fig. S4, ESI†). According to Fermi's golden and Kasha's rules,40 this indicates the existence of a favorable “hot exciton” channel facilitating the hRISC processes from T3 or T4 to S1, potentially enhancing exciton utilization efficiency in the EL process.
Photophysical properties
In order to investigate the photophysical properties of PICZ4F and PI3CZ4F, ultraviolet-visible (UV-vis) absorption and photoluminescence (PL) spectra in a dilute tetrahydrofuran (THF) solution were measured. As presented in Fig. 2a and b, the absorption peak at approximately 291 nm can be attributed to the n–π* transition of the carbazole group, while the absorption bands of 300–365 nm can be ascribed to the π–π* transition of the phenanthroimidazole and phenylcarbazole groups.9,41,42 According to the onset of the absorption spectra, the optical bandgaps (Egs) were estimated to be 3.17 and 3.19 eV for PICZ4F and PI3CZ4F, respectively. Upon photoexcitation, PICZ4F exhibits a blue emission with a maximum peak wavelength at 443 nm and a high PLQY value of 99%, whereas PI3CZ4F displays a slight blue-shifted emission with the peak at 429 nm, due to the reduced conjugation length caused by different connection modes of phenylcarbazole. The calculated energy levels of S1 and T1 for PICZ4F are 2.80 and 2.35 eV, respectively, while for PI3CZ4F, they are 2.88 and 2.35 eV, derived from the emission peaks observed in low-temperature fluorescence and phosphorescence spectra at 77 K. The ΔES1T1 values of up to 0.45 and 0.53 eV reveal that the typical TADF mechanism involving the RISC process from T1 to S1 did not occur in PICZ4F and PI3CZ4F. As shown in Fig. 2c, PICZ4F and PI3CZ4F neat films emit pure-blue light, with the peak wavelengths of 453 and 455 nm and PLQYs of 80% and 87%, respectively, while the doped films show blue-shifted and enhanced emission, with the PL peaks at 432 and 441 nm, and PLQYs of 97% and 90%, due to the attenuation of the aggregation effect. It should be noted that such high PLQYs achieved in the films are rare, especially in neat films. As shown in Fig. 2d and Fig. S5 (ESI†), and summarized in Table S3 (ESI†), PICZ4F and PI3CZ4F exhibit nanosecond fluorescence lifetimes below 2 ns and high PLQYs over 95% in both high polarity and low polarity solvents, further ruling out the TADF process. Upon integrating the fluorescence lifetimes measured in the films, which are 1.216 and 1.568 ns for PICZ4F neat and doped films, respectively, and 0.971 and 0.905 ns for PI3CZ4F neat and doped films, the kr values of 6.58 × 108 and 6.19 × 108 s−1 for PICZ4F neat and doped films are achieved using the formula: kr = PLQ Y/τ, Notably, PI3CZ4F exhibits superior kr values, reaching 8.96 × 108 and 9.94 × 108 s−1, respectively. The photophysical and electrochemical data are summarized in Table 1.
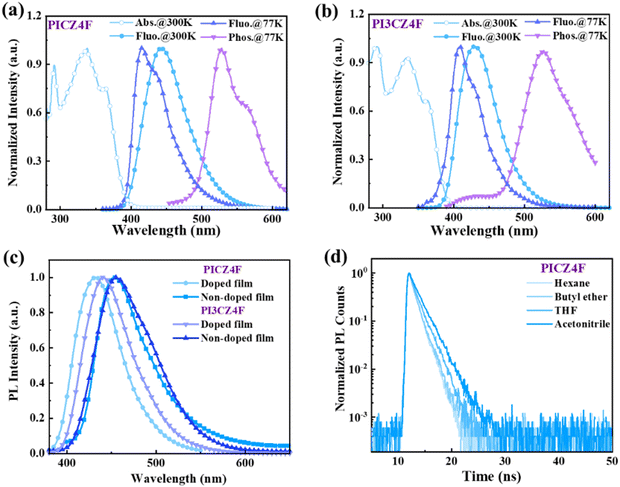 |
| Fig. 2 Room temperature UV-vis absorption and emission spectra in THF solvent (10 μM) and low temperature fluorescence and phosphorescence spectra for PICZ4F (a) and PI3CZ4F (b). (c) Emission spectra of neat films, and doped films with 5 wt% dispersed into polymethyl methacrylate (PMMA) for PICZ4F and PI3CZ4F. (d) Transient PL decay spectra of PICZ4F in the hexane, butyl ether, THF, and acetonitrile solvents (10 μM). | |
Table 1 The photophysical and electrochemical data of PICZ4F and PI3CZ4F
Material |
λ
Fluo (nm) |
PLQY (%) |
HOMO/LUMO/Egd (eV) |
E
S1/ET1e (eV) |
τ
(ns) |
k
r
(×108 s−1) |
Measured in THF solvent (10−5 μM) at 300 K.
Measured in neat films at 300 K.
Measured in doped films with 5 wt% dispersed into PMMA at 300 K.
HOMO energy level: obtained from CV measurement. Eg: calculated from the absorption onset. LUMO energy level: calculated by ELUMO = EHOMO + Eg.
S1 and T1 energy levels.
Fluorescence lifetime.
Radiative rate.
|
PICZ4F
|
443a/453b/432c |
99a/80b/97c |
−5.56/−2.39/3.17 |
2.80/2.35 |
1.216b/1.568c |
6.58b/6.19c |
PI3CZ4F
|
429a/455b/441c |
99a/87b/90c |
−5.61/−2.42/3.19 |
2.88/2.35 |
0.971b/0.905c |
8.96b/9.94c |
To investigate the excited-state properties of PICZ4F and PI3CZ4F, the solvatochromic effect in various solvents was measured and summarized in Tables S4 and S5 (ESI†). As can be observed from Fig. 3 and Fig. S6 (ESI†), the absorption profiles of both PICZ4F and PI3CZ4F exhibit only minor changes with the increase in solvent polarity, whereas the PL spectra of PICZ4F and PI3CZ4F, respectively, display significant red-shifts of 57 and 48 nm. The emission of both compounds shows a fine structure in the low-polarity solvent of n-hexane but exhibits broad and structureless profiles in mid-polarity and high-polarity solvents, confirming the presence of an intrinsic CT component in the excited state. According to the Lippert–Mataga model, plots of the Stokes shift (va − vf) against the orientation polarizability (f(ε,n)) were applied to estimate the dipole moment of the S1 state. As shown in Fig. S7 (ESI†), PICZ4F displays a two-section fitting line in low- and high-polar solvents, while PI3CZ4F shows a linear relationship with a dipole moment of 11.79 Debye, lower than that of the pure CT compound 4-(N,N-dimethylamine)benzonitrile (23 Debye),43 signifying that the excited states of PICZ4F and PI3CZ4F are non- and quasi-equivalent HLCT states.8,18,44,45 This is also proven by the single-exponential fluorescence lifetimes in both low- and high-polar solvents.
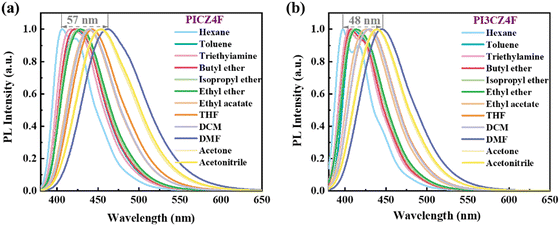 |
| Fig. 3 PL spectra in various solvents for PICZ4F (a) and PI3CZ4F (b) with the concentrations of 10 μM. | |
Electrochemical and electrical properties
Based on the cyclic voltammetry measurements, the oxidation potentials were estimated to be 0.95 and 1.0 V (Fig. S8, ESI†), corresponding to the HOMO energy levels of −5.56 and −5.61 eV for PICZ4F and PI3CZ4F, respectively. Their LUMO energy levels can be calculated as −2.39 and −2.42 eV, respectively, from the energy difference between the Egs and HOMO energy levels. Furthermore, single-carrier devices were fabricated to investigate the carrier transporting capabilities of PICZ4F and PI3CZ4F. The device architectures are indium tin oxide (ITO)/lithium fluoride (LiF, 1 nm)/1,3,5-tris(1-phenyl-1H-benzimidazol-2-yl)-benzene (TPBi, 10 nm)/PICZ4F or PI3CZ4F (80 nm)/TPBi (10 nm)/LiF (1 nm)/aluminium (Al, 100 nm) for electron-only devices (EODs), and ITO/N,N′-bis(1-naphthalenyl)-N,N′-bisphenyl-(1,1′-biphenyl)-4,4′-diamine (NPB, 10 nm)/PICZ4F or PI3CZ4F (80 nm)/NPB (10 nm)/Al (100 nm) for hole-only devices (HODs), respectively. As depicted in Fig. 4, the electron and hole current densities of PICZ4F and PI3CZ4F devices display a steady escalation in concert with the rising applied voltage, unequivocally showcasing their bipolar transport capabilities. This distinctive feature holds immense potential for broadening the exciton recombination zone and effectively suppressing exciton quenching effects, which plays a crucial role in the efficiency roll-off behavior in the non-doped OLEDs with them as pure emissive layers. Under an electric field of 5 × 105 V cm−1, PICZ4F boasts electron and hole mobilities of 5.37 × 10−5 and 4.08 × 10−8 cm2 V−1 s−1, respectively, whereas PI3CZ4F demonstrates even more impressive electron and hole mobilities, reaching 6.44 × 10−5 and 3.40 × 10−7 cm2 V−1 s−1. Notably, both materials exhibit more excellent electron and hole transport properties than that of DCZ2F and PICZ2F (Fig. S9, ESI†),33,34 illustrating the superiority of tetrafluorobenzene-bridged asymmetric D–A–D′ type molecular design strategy.
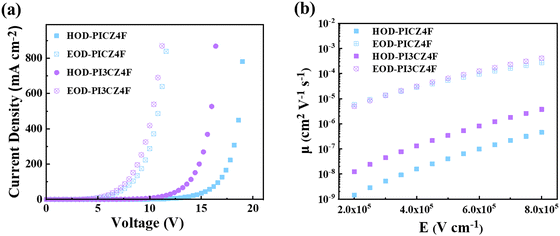 |
| Fig. 4 (a) The current density–voltage curves of electron- and hole-only devices for PICZ4F and PI3CZ4F. (b) Carrier mobilities versus electric field curves of PICZ4F and PI3CZ4F. | |
EL performance
In pursuit of adhering to the BT.2100 blue standard, we embarked on an initial exploration of the EL performance of the doped OLEDs incorporated PICZ4F and PI3CZ4F as emitters. Taking into account both the singlet and triplet energy levels, the widely utilized material, 4,4′-bis(N-carbazolyl)-1,1′-biphenyl (CBP), was selected as the host matrix for PICZ4F and PI3CZ4F. Devices with the following structures were processed: ITO/HATCN (20 nm)/TAPC (45 nm)/TCTA (10 nm)/CBP: XX (5 wt%, 20 nm)/TPBi (30 nm)/LiF (1 nm)/Al (100 nm). Thereinto, the doping concentrations of PICZ4F- and PI3CZ4F-based devices (D1 and D2) are 5 wt%, dipyrazino[2,3-f:2′,3′-h]quinoxaline-2,3,6,7,10,11-hexacarbonitrile (HATCN) and LiF served as the hole- and electron-injection layers, 4,4′-(cyclohexane-1,1-diyl)bis(N,N-di-ptolylaniline) (TAPC) was used as the hole transporting layer, tris(4-carbazoyl-9-ylphenyl)amine (TCTA) was employed as hole transporting and exciton-blocking layers, and TPBi functioned as electron transporting, hole-blocking, and exciton-blocking layers, respectively. The molecular structures and energy level diagram of the functional layer materials are shown in Fig. S10 (ESI†). The device structure and EL performance are shown in Fig. 5 and Fig. S11 (ESI†), and summarized in Table 2.
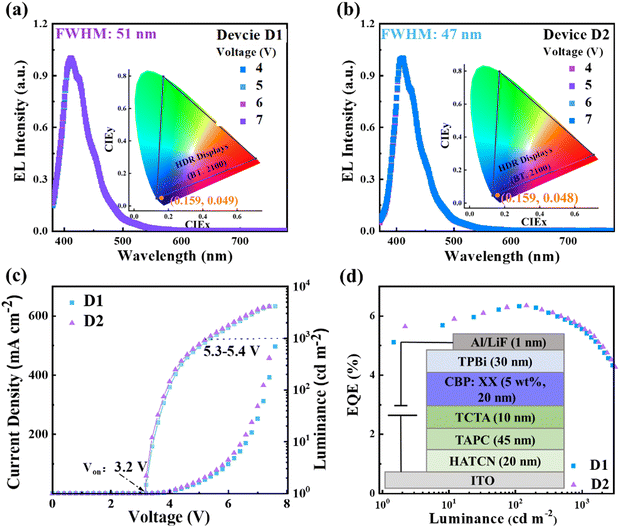 |
| Fig. 5 (a) EL spectra at applied voltages from 4 to 7 V and the CIE chromaticity diagram of device D1. (b) EL spectra at applied voltages from 4 to 7 V and CIE chromaticity diagram of device D2. (c) Current density–voltage-luminance characteristics of devices D1 and D2. (d) EQE versus luminance curves of devices D1 and D2. Insert: device structure. | |
Table 2 Summary of key EL parameters for the D1, D2, N1, and N2 devices
Devices |
Emissive layer |
V
on/1000
[V] |
CEmax/1000b [cd A−1] |
PEmax/1000b [lm W−1] |
EQEmax/1000/10 000c [%] |
λ
EL
[nm] |
FWHMd [nm] |
CIE(x,y)d |
The voltages at 1 and 1000 cd m−2.
Maximum and the value taken at 1000 cd m−2.
Maximum and the values taken at 1000 and 10 000 cd m−2.
Measured at a voltage of 5 V.
|
D1
|
CBP:PICZ4F
|
3.2/5.4 |
1.97/1.73 |
1.65/1.00 |
6.34/5.54/— |
412 |
51 |
(0.159, 0.049) |
D2
|
CBP:PI3CZ4F
|
3.2/5.3 |
1.89/1.68 |
1.65/1.00 |
6.35/5.61/— |
410 |
47 |
(0.159, 0.048) |
N1
|
PICZ4F
|
2.8/3.7 |
9.78/9.19 |
9.95/7.93 |
6.91/6.62/5.97 |
455 |
81 |
(0.160, 0.183) |
N2
|
PI3CZ4F
|
2.85/3.7 |
9.74/8.92 |
10.19/7.76 |
7.44/6.99/6.19 |
455 |
82 |
(0.159, 0.167) |
As displayed in Fig. 5, both devices D1 and D2, emitting deep-blue light, exhibit stable spectral stability across varying voltages. At a voltage of 5 V, EL peaks are achieved at 412 nm and 410 nm, respectively, with narrow full-width at half-maximum (FWHM) of 51 and 47 nm. These correspond to CIE coordinates of (0.159, 0.049) for D1 and (0.159, 0.048) for D2, where the CIEy values are in close proximity to the BT.2100 blue standard of (0.131, 0.046). The narrower emission achieved in the device D2 may be attributed to the restriction of motions of the carbazole group by C–H⋯F interactions. The low turn-on voltages of 3.2 V at 1 cd m−2 signify efficient hole/electron injection and transport in the devices D1 and D2. At 5.3–5.4 V, the brightness reaches 1000 cd m−2, and the maximum brightness of 4179 and 4109 cd m−2 are achieved for D1 and D2, respectively. Notably, the devices D1 and D2 exhibit maximum EQEs of 6.34% and 6.35% at 114 and 146 cd m−2, respectively, maintaining levels as high as 5.54% and 5.61% even at a high brightness of 1000 cd m−2. This low efficiency roll-off can be ascribed to the fast krs.
Inspired by the exceptional bipolar transporting properties of PICZ4F and PI3CZ4F, we further explored their application potential as a pure emissive layer in the simple non-doped OLEDs. For comparison, the non-doped devices (N1 and N2) adopted the same structure as the doped devices. As shown in Fig. 6 and Fig. S11 (ESI†) and Table 2, the non-doped devices N1 and N2 demonstrate higher brightness and lower driving voltages, alongside superior EL efficiencies, in comparison to their doped counterparts. The devices boast turn-on voltages as low as 2.8 and 2.85 V, accompanied by driving voltages of just 3.7 and 5.6 V at brightness levels of 1000 and 10
000 cd m−2, respectively. Even more remarkable, they achieve peak brightness of 37
728 and 40
239 cd m−2 at 7.4 and 7.2 V, respectively, due to their exceptional bipolar transporting abilities and fast krs. Compared to the PICZ4F-based non-doped device N1 with a maximum EQE of 6.91%, device N2 achieves a better EL performance with a maximum EQE, current efficiency (CE), and power efficiency (PE) of 7.44%, 9.74 cd A−1 and 10.19 lm W−1, respectively. At 1000 cd m−2, the EQEs of devices N1 and N2 still remain as high as 6.62% and 6.99% showing a small efficiency roll-off. The superior EL performance achieved in device N2 can be attributed to the high fluorescence efficiency and more balanced bipolar transport capability of PI3CZ4F. Meanwhile, devices N1 and N2 display stable blue emission with EL peaks centered at 455 nm, corresponding to CIE coordinates of (0.160, 0.183) and (0.159, 0.167) (Fig. S12, ESI†). It is particularly noteworthy that the device N2 manages to maintain remarkable efficiency at high brightness. Specifically, it achieves EQEs of 6.19%, 6.07% and 5.87% at 10
000, 20
000, and 30
000 cd m−2, respectively, which is a very rare result in blue OLEDs reported so far. This provides an opportunity for the practical application of OLEDs at a high brightness level. Impressively, the doped devices D1 and D2 exhibit minimal efficiency roll-off, boasting EQE1000 values of 5.54% and 5.61%, respectively, and demonstrate a CIEy color coordinate in close proximity to the BT.2100 blue standard, positioning them among the elite of reported deep-blue OLEDs in terms of both EL performance and color purity (Fig. 7 and Table S6, ESI†).
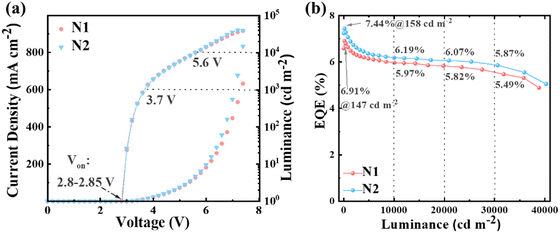 |
| Fig. 6 (a) Current density–voltage–luminance characteristics of the non-doped devices N1 and N2. (b) EQE versus luminance curves of devices N1 and N2. | |
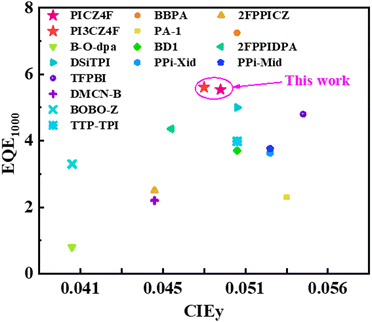 |
| Fig. 7 Summary of the EQE1000–CIEy plots of representative reported deep-blue emitters. | |
Conclusions
In summary, we have successfully designed and synthesized two highly efficient D–A–D′ type blue HLCT emitters, PICZ4F and PI3CZ4F, utilizing a novel tetrafluorobenzene acceptor. These emitters exhibit prominent fluorescence efficiencies in both solutions and films, and furthermore, achieve an enhancement in bipolar transporting properties due to the multiple intramolecular C–H⋯F interactions. The doped OLEDs achieve the maximum EQEs of 6.34% @114 cd m−2 and 6.35% @146 cd m−2, accompanied by a small efficiency roll-off, with CIE coordinates of (0.159, 0.049) and (0.159, 0.048), respectively, closely approximating the BT.2100 blue standard. Additionally, the non-doped device with PI3CZ4F as the pure emissive layer demonstrates even more exceptional EL performance, with an EQE of 7.44%, and maintains remarkably high values exceeding 6% even at a brightness level of 20
000 cd m−2, underscoring the significant potential of OLEDs for high brightness application scenarios. This work introduces a promising tetrafluorobenzene-based asymmetric D–A–D′ molecular design concept, which paves the way for the development of high-performance deep-blue OLEDs with high efficiency and low roll-off.
Data availability
The data supporting this article have been included as part of the ESI.†
Conflicts of interest
The authors declare that they have no known competing financial interests or personal relationships that could have appeared to influence the work reported in this paper.
Acknowledgements
The authors acknowledge the financial support from the Natural Science Foundation of Qingdao (no. 23-2-1-75-zyyd-jch), the Natural Science Foundation of Shandong Provincial (no. ZR2023QE078, ZR2022ZD37, and ZR2019ZD50), the National Natural Science Foundation of China (no. 52103220, 52103017, and 22022501), and the Science and Technology Support Plan for Youth Innovation of Colleges and Universities in Shandong Province (2023KJ097).
Notes and references
- M. A. Baldo, D. F. O’Brien, Y. You, A. Shoustikov, S. Sibley, M. E. Thompson and S. R. Forrest, Highly Efficient Phosphorescent Emission from Organic Electroluminescent Devices, Nature, 1998, 395, 151 CrossRef CAS.
- T. Kim, K. H. Kim, S. Kim, S. M. Choi, H. Jang, H. K. Seo, H. Lee, D. Y. Chung and E. Jang, Efficient and stable blue quantum dot light-emitting diode, Nature, 2020, 586, 385 CrossRef CAS PubMed.
- J. Hwang, P. Nagaraju, M. J. Cho and D. H. Choi, Aggregation-induced emission luminogens for organic light-emitting diodes with a single-component emitting layer, Aggregate, 2023, 4, e199 CrossRef CAS.
- J. Xu, M. Wang, J. Chen, Z. Wu, T. Guo, B. Z. Tang and Z. Zhao, Efficient Blue Carbonyl-Nitrogen Multi-Resonance Molecules for High-Performance Hyperfluorescence OLEDs, Adv. Opt. Mater., 2024, 12, 2400739 CrossRef CAS.
- S. Hirata, Y. Sakai, K. Masui, H. Tanaka, S. Y. Lee, H. Nomura, N. Nakamura, M. Yasumatsu, H. Nakanotani, Q. Zhang, K. Shizu, H. Miyazaki and C. Adachi, Highly efficient blue electroluminescence based on thermally activated delayed fluorescence, Nat. Mater., 2015, 14, 330 CrossRef CAS.
- H.-J. Tan, G.-X. Yang, Y.-L. Deng, C. Cao, J.-H. Tan, Z.-L. Zhu, W.-C. Chen, Y. Xiong, J.-X. Jian, C.-S. Lee and Q.-X. Tong, Deep-Blue OLEDs with Rec.2020 Blue Gamut Compliance and EQE Over 22% Achieved by Conformation Engineering, Adv. Mater., 2022, 34, 2200537 CrossRef CAS.
- X. He, J. Lou, B. Li, X. Dong, F. Zhong, W. Liu, X. Feng, D. Yang, D. Ma, Z. Zhao, Z. Wang and B. Z. Tang, Rational Medium-Range Charge Transfer Strategy Toward Highly Efficient Violet-Blue Organic Light-Emitting Diodes with Narrowed Emission, Adv. Mater., 2024, 36, 2310417 CrossRef CAS PubMed.
- R.-Z. An, Y. Sun, H.-Y. Chen, Y. Liu, A. Privitera, W. K. Myers, T. K. Ronson, A. J. Gillett, N. C. Greenham and L.-S. Cui, Excited-State Engineering Enables Efficient Deep-Blue Light-Emitting Diodes Exhibiting BT.2020 Color Gamut, Adv. Mater., 2024, 4, 2313602 CrossRef.
- L. Peng, J. Lv, S. Xiao, Y. Huo, Y. Liu, D. Ma, S. Ying and S. Yan, High-performance non-doped near ultraviolet OLEDs with the EQE ∼ 6% and CIEy ∼ 0.03 from high-lying reverse intersystem crossing, Chem. Eng. J., 2022, 450, 138339 CrossRef CAS.
- L. Zhang, L. Wang, K. Samedov, M. Chen, D. Chen and Y. Cai, High-Lying Triplet Excitons Utilization of Silole Derivatives Enables their Efficiency Breakthrough in OLEDs, Adv. Funct. Mater., 2024, 2410250 CrossRef.
- Y. Xu, P. Xu, D. Hu and Y. Ma, Recent progress in hot exciton materials for organic light-emitting diodes, Chem. Soc. Rev., 2021, 50, 1030 RSC.
- W. Li, Y. Pan, R. Xiao, Q. Peng, S. Zhang, D. Ma, F. Li, F. Shen, Y. Wang, B. Yang and Y. Ma, Employing ∼100% Excitons in OLEDs by Utilizing a Fluorescent Molecule with Hybridized Local and Charge-Transfer Excited State, Adv. Funct. Mater., 2014, 24, 1609 CrossRef CAS.
- S. Xiao, Y. Gao, R. Wang, H. Liu, W. Li, C. Zhou, S. Xue, S.-T. Zhang, B. Yang and Y. Ma, Highly efficient hybridized local and Charge-transfer (HLCT) Deep-blue electroluminescence with excellent molecular horizontal orientation, Chem. Eng. J., 2022, 440, 135911 CrossRef CAS.
- H. Jiang, H. Li, J. Qiu, J. Jin, C. Xi, P. Tao, B. Zhang, D. Ma and W.-Y. Wong, Manipulating the excited states from charge-transfer to hybridized local and charge-transfer towards high-performance blue electroluminescence, Chem. Eng. J., 2023, 473, 145295 CrossRef CAS.
- Z. Shen, X. Zhu, W. Tang, Y. Zheng, Z. Zhou, X. J. Feng, Z. Zhao and H. Lu, Bis(trimethylsilyl)phenyl-bridged D–A molecules: Synthesis, spectroscopic properties and for achieving deep-blue emitting materials, Dyes Pigm., 2020, 174, 108063 CrossRef CAS.
- Q. Luo, Y. Gao, H. Xu, S. Zhao, W. Dong, Y. Miao, Y. Wang, H. Wang and J. Yu, Applications of hot-exciton anthracene and imidazole derivatives in integrated organic blue-emitting diodes and ultraviolet photodetectors, J. Mater. Chem. C, 2023, 11, 15918 RSC.
- F.-M. Xie, J.-X. Zhou, Y.-Q. Li and J.-X. Tang, Effects of the relative position and number of donors and acceptors on the properties of TADF materials, J. Mater. Chem. C, 2020, 8, 9476 RSC.
- H. Qi, D. Xie, Z. Gao, S. Wang, L. Peng, Y. Liu, S. Ying, D. Ma and S. Yan, A record-high EQE of 7.65%@3300 cd m−2 achieved in non-doped near-ultraviolet OLEDs based on novel D′–D–A type bipolar fluorophores upon molecular configuration engineering, Chem. Sci., 2024, 15, 11053 RSC.
- L. Peng, Y. Huo, S. He, Y. Liu, Z. Ren, S. Ying and S. Yan, A linear deep-blue bipolar fluorescent material with the CIEy < 0.065 serving as the emitter and host for high-performance monochromatic and hybrid white OLEDs, J. Mater. Chem. C, 2022, 10, 11642 RSC.
- J. Lv, Y. Huo, S. Xiao, Z. Zhao, L. Peng, Y. Liu, Z. Ren, D. Ma, S. Ying and S. Yan, Correction: Regulation of excited-state properties of dibenzothiophene-based fluorophores for realizing efficient deep-blue and HLCT-sensitized OLEDs, Mater. Chem. Front., 2023, 7, 183 RSC.
- L. Peng, Y. Huo, L. Hua, J. Lv, Y. Liu, S. Ying and S. Yan, A highly efficient violet-blue OLED with Rec.2020 CIEy based on an orthogonal phenanthroimidazole-substituted 1,2,4-triazole derivative, J. Mater. Chem. C, 2022, 10, 9621 RSC.
- L. Peng, J. Lv, Y. Huo, L. Hua, Y. Liu, S. Ying and S. Yan, High performance deep-blue organic light emitting diodes via excited state regulation of phenanthroimidazole-triazole derivatives, Dyes Pigm., 2022, 206, 110676 CrossRef CAS.
- S. Xue, X. Qiu, S. Ying, Y. Lu, Y. Pan, Q. Sun, C. Gu and W. Yang, Highly Efficient Nondoped Near-Ultraviolet Electroluminescence with an External Quantum Efficiency Greater Than 6.5% Based on a Carbazole–Triazole Hybrid Molecule with High and Balanced Charge Mobility, Adv. Opt. Mater., 2017, 5, 1700747 CrossRef.
- P. Han, C. Lin, H. Xu, E. Xia, D. Yang, A. Qin, D. Ma and B. Z. Tang, Improving the Efficiency of AIEgen-Based Nondoped Blue Organic Light-Emitting Diode by Rational Isomer Engineering, ACS Mater. Lett., 2022, 4, 1087 CrossRef CAS.
- C. Lin, P. Han, S. Xiao, F. Qu, J. Yao, X. Qiao, D. Yang, Y. Dai, Q. Sun, D. Hu, A. Qin, Y. Ma, B. Z. Tang and D. Ma, Efficiency
Breakthrough of Fluorescence OLEDs by the Strategic Management of “Hot Excitons” at Highly Lying Excitation Triplet Energy Levels, Adv. Funct. Mater., 2021, 31, 2106912 CrossRef CAS.
- X. Tang, Q. Bai, Q. Peng, Y. Gao, J. Li, Y. Liu, L. Yao, P. Lu, B. Yang and Y. Ma, Efficient Deep Blue Electroluminescence with an External Quantum Efficiency of 6.8% and CIEy < 0.08 Based on a Phenanthroimidazole–Sulfone Hybrid Donor–Acceptor Molecule, Chem. Mater., 2015, 27, 7050 CrossRef CAS.
- M. Sun, C. Ma, L. Chu, Y. Pan, Q. Sun, W. Yang and S. Xue, Highly efficient deep-blue organic light-emitting diodes (OLEDs) based on hot-exciton materials with multiple triplet exciton conversion channels, J. Mater. Chem. C, 2023, 11, 16258 RSC.
- C. Du, H. Liu, Z. Cheng, S. Zhang, Z. Qu, D. Yang, X. Qiao, Z. Zhao and P. Lu, Ultraefficient Non-Doped Deep Blue Fluorescent OLED: Achieving a High EQE of 10.17% at 1000 cd m−2 with CIEy < 0.08, Adv. Funct. Mater., 2023, 33, 2304854 CrossRef CAS.
- X. Qiu, S. Ying, C. Wang, M. Hanif, Y. Xu, Y. Li, R. Zhao, D. Hu, D. Ma and Y. Ma, Novel 9,9-dimethylfluorene-bridged D–π–A-type fluorophores with a hybridized local and charge-transfer excited state for deep-blue electroluminescence with CIEy ∼ 0.05, J. Mater. Chem. C, 2019, 7, 592 RSC.
- J. Lv, S. Song, J. Li, L. Peng, Y. Li, Y. Liu, D. Ma, S. Ying and S. Yan, High and Balanced Bipolar-Transporting Deep-Blue HLCT Material for Efficient Monochrome and White OLEDs based on a Simple Phenanthroimidazole-Dibenzothiophene Derivative, Adv. Opt. Mater., 2023, 12, 2301413 CrossRef.
- J. Lv, J. Li, S. Song, S. Wang, H. Qi, Y. Huo, Y. Liu, S. Ying and S. Yan, Bipolar Deep-Blue Host for High-Performance Multicolor OLEDs by Peripheral Auxiliary Group Strategy, ACS Appl. Opt. Mater., 2023, 1, 1963 CrossRef CAS.
- F. Liu, G. Cao, Z. Feng, Z. Cheng, Y. Yan, Y. Xu, Y. Jiang, Y. Chang, Y. Lv and P. Lu, Triphenylene-Based Emitters with Hybridized Local and Charge-Transfer Characteristics for Efficient Nondoped Blue OLEDs with a Narrowband Emission and a Small Efficiency Roll-Off, ACS Appl. Mater. Interfaces, 2023, 15, 47307 CrossRef CAS PubMed.
- Y. Huo, J. Lv, M. Wang, Z. Duan, H. Qi, S. Wang, Y. Liu, L. Peng, S. Ying and S. Yan, Simple excited-state modification toward a deep-blue hybridized local and charge-transfer (HLCT) fluorophore and non-doped organic light-emitting diodes, J. Mater. Chem. C, 2023, 11, 6347 RSC.
- S. Wang, L. Peng, F. He, Y. Ming, H. Qi, Y. Liu, D. Ma, S. Ying and S. Yan, A Breakthrough in Color-Tunable All-Fluorescent White OLEDs through the Cooperation Between a New Blue HLCT Fluorophore and a Long-Wavelength TADF Emitter, Adv. Opt. Mater., 2024, 4, 2400503 CrossRef.
- K. Zhang, Z. Zhou, D. Liu, Y. Chen, S. Zhang, J. Pan, X. Qiao, D. Ma, S. Su, W. Zhu and Y. Liu, Boosting External Quantum Efficiency to 12.0% of an Ultraviolet OLED by Engineering the Horizontal Dipole Orientation of a Hot Exciton Emitter, Angew. Chem., Int. Ed., 2024, 5, e202407502 Search PubMed.
- H. Huang, L. Yang, A. Facchetti and T. J. Marks, Organic and Polymeric Semiconductors Enhanced by Noncovalent Conformational Locks, Chem. Rev., 2017, 117, 10291 CrossRef CAS PubMed.
- Q. Fang, B. Xu, B. Jiang, H. Fu, W. Zhu, X. Jiang and Z. Zhang, A novel fluorene derivative containing four triphenylamine groups: Highly thermostable blue emitter with hole-transporting ability for organic light-emitting diode (OLED), Synth. Met., 2005, 155, 206 CrossRef CAS.
- J. Yu, S. Lou, Y. Jiang and Q. Zhang, White organic light emitting diodes show improved performance, SPIE Newsroom, 2008, 10, 1309 Search PubMed.
- Y. Liu, B. Du, X. Han, X. Wu, H. Tong and L. Wang, Intramolecular-locked triazatruxene-based thermally activated delayed fluorescence emitter for efficient solution-processed deep-blue organic light emitting diodes, Chem. Eng. J., 2022, 446, 137372 CrossRef CAS.
- M. A. El-Sayed, Spin—Orbit Coupling and the Radiationless Processes in Nitrogen Heterocyclics, J. Chem. Phys., 1963, 38, 2834 CrossRef CAS.
- Z. Gao, Z. Wang, T. Shan, Y. Liu, F. Shen, Y. Pan, H. Zhang, X. He, P. Lu, B. Yang and Y. Ma, High-efficiency deep blue fluorescent emitters based on phenanthro[9,10-d]imidazole substituted carbazole and their applications in organic light emitting diodes, Org. Electron., 2014, 15, 2667 CrossRef CAS.
- W.-C. Chen, Y. Yuan, S.-F. Ni, Q.-X. Tong, F.-L. Wong and C.-S. Lee, Achieving efficient violet-blue electroluminescence with CIEy < 0.06 and EQE > 6% from naphthyl-linked phenanthroimidazole–carbazole hybrid fluorophores, Chem. Sci., 2017, 8, 3599 RSC.
- Z. R. Grabowski, K. Rotkiewicz and W. Rettig, Structural Changes Accompanying Intramolecular Electron Transfer:
Focus on Twisted Intramolecular Charge-Transfer States and Structures, Chem. Rev., 2003, 103, 3899 CrossRef PubMed.
- H. Zhang, B. Zhang, Y. Zhang, Z. Xu, H. Wu, P.-A. Yin, Z. Wang, Z. Zhao, D. Ma and B. Z. Tang, A Multifunctional Blue-Emitting Material Designed via Tuning Distribution of Hybridized Excited-State for High-Performance Blue and Host-Sensitized OLEDs, Adv. Funct. Mater., 2020, 30, 2002323 CrossRef CAS.
- J. Chen, X. Wu, H. Liu, N. Qiu, Z. Liu, D. Yang, D. Ma, Z. Tang Ben and Z. Zhao, Towards Efficient Blue Delayed-Fluorescence Molecules by Modulating Torsion Angle Between Electron Donor and Acceptor, CCS Chem, 2022, 5, 598 CrossRef.
|
This journal is © the Partner Organisations 2025 |
Click here to see how this site uses Cookies. View our privacy policy here.