DOI:
10.1039/D4QM00668B
(Research Article)
Mater. Chem. Front., 2025,
9, 131-146
New heater@luminescent thermometer nano-objects: Prussian blue core@silica shell loaded with a β-diketonate Tb3+/Eu3+ complex†
Received
6th August 2024
, Accepted 6th November 2024
First published on 20th November 2024
Abstract
We report on the synthesis and investigation of new multifunctional Prussian blue (PB) nanoparticles coated by a mesoporous silica shell and loaded with a luminescent [(Tb/Eu)9(acac)16(μ3-OH)8(μ4-O)(μ4-OH)]·H2O complex. These multifunctional nano-objects work as efficient photothermal nano-heaters able to provide macroscopic temperature rises remotely triggered by light irradiation at 808 nm (ΔT = 20.4 °C under irradiation for 3 min with a laser power of 1.83 W cm−2). Their specific heat capacity, the primary parameter influencing the heating properties of nanoparticles, was determined by using the photothermal properties and the measured heat capacity of PB nanoparticles, yielding a value of 1.13 ± 0.03 J g−1 K−1. This moderate value indicates that once heated, the nanoparticles can retain heat effectively, making them suitable for applications requiring sustained and controlled thermal effects. On the other hand, these multifunctional nanoparticles exhibit the characteristic temperature-dependent luminescence of Tb3+ and Eu3+ with improved Tb3+-to-Eu3+ energy transfer, making them efficient as luminescent ratiometric thermometers. These nanothermometers operate in the 20–80 °C range exhibiting a maximal relative thermal sensitivity of 0.75% °C−1 at 20 °C.
1. Introduction
Photothermal nano-objects, renowned for their adeptness in efficiently converting light into heat and generating a significant temperature elevation at the macroscopic level, have garnered considerable interest over the past several decades.1,2 This interest extends beyond fundamental exploration, encompassing a spectrum of technological applications. We can cite the most investigated biomedical area as hyperthermia therapy of cancer,3–6 bacteria-related diseases,7 or thermally enhanced drug delivery.8,9 On the other hand, these nano-objects are widely employed for photothermal catalysis,10–12 water desalination and purification,13–15 energy conversion,16 heat-mediated optical manipulation,17,18 and optical devices,19,20 among other applications. Over the years, various nano-objects have been revealed as efficient photothermal agents, including plasmonic metal nanoparticles,21–24 carbon-based nanomaterials,25,26 nanostructured semiconductors,27 up-conversion nanoparticles,28–30 metal–organic frameworks,31–33 covalent organic frameworks34–36 and more recently iron oxide nanoparticles.37 Among these, Prussian blue (PB) nano-objects have emerged as promising alternatives, showcasing remarkable potential for photothermia-linked applications.
PB nanoparticles, characterized by the general formula A1−xFeIII[FeII(CN)6]1−x (where A denotes an alkaline ion), represent a distinctive class of inorganic nanomaterials with versatile composition, well determined structure and promising physico-chemical properties.38 PB nanoparticles present interesting advantages including: (i) the possibility to control both size and morphology, (ii) the possibility to dope the PB network with mono (Cs+, Tl+), divalent (Mn2+ or Zn2+) or lanthanide ions (Gd3+, Dy3+…) to endow or improve targeted properties, (iii) remarkable photo and thermal stability especially in aqueous solutions at low pH, and (v) biocompatibility, underscored by FDA approval for using bulk PB as an antidote for Cs+ and Tl+ poisoning. More specifically, PB nanoparticles are well-known for their strong light absorption with a high molar extinction coefficient in the near-infrared (NIR) spectral range (I window) spanning from 650 to 850 nm, due to the presence of a Fe2+-to-Fe3+ charge transfer band. Furthermore, they exhibit high efficiency in converting light energy into heat associated with important photothermal stability, making them highly attractive for nanoparticle-assisted photothermal heating. Photothermal properties of PB nanoparticles and PB analogues have been extensively proposed for applications in the medical field, particularly for cancer therapy,39–44 tissue repair and wound healing,45–48 as well as for antibacterial or anti-inflammatory applications,49,50 related to Alzheimer's disease,51 thrombolytic management,52 or the development of biosensors.53,54 It is also interesting to note that their photothermal effect has also been studied for other applications, such as photocatalysis,55 deicing,56 actuators,57 self-healing materials58 or coatings,59 and crude oil adsorption.60 Several investigations have been devoted to the determination of photothermal conversion efficiency (η) of PB nanoparticles. However, due to the fact that the latter significantly depends on nanoparticles’ parameters (size, surface agents, solvent, environment etc.), and the experimental conditions used, different values ranging from 50 to 80% have been provided.61–64 Moreover, a comprehensive understanding of PB nanoparticles' heating properties has not been reached, particularly concerning their heating capacity, which has yet to be investigated. The latter is a crucial factor because it influences how quickly and efficiently the nano-heater can keep and transfer heat, impacting the overall performance and suitability for specific applications.
In the realm of photothermal heat generation by inorganic nanoparticles under light irradiation, a significant challenge lies in accurately regulating temperature not only at the macroscopic level but also in the immediate proximity of the surface of the nano-heater. In this context, considering that conventional temperature measurement instruments are ineffective at the nanoscale due to limitations in sensitivity, accuracy, and spatial resolution, precise temperature measurements are essential. They are necessary for understanding the behaviour of nanoparticles at this scale, where rapid fluctuations and nanoscale-related effects occur. Additionally, precise temperature measurements are crucial for assessing photothermal efficacy, ensuring safety, optimizing parameters, and maintaining quality control across various applications.
Luminescent thermometry is an emerging solution, which leverages the emission properties of various materials to provide highly accurate, non-contact, and localized temperature measurements, even at the micro and nanoscale. It offers several advantages, including high spatial precision (below 10 μm), temporal resolution (0.1 °C), short acquisition times (less than 10 μs), and high maximal relative sensitivity (Srmax > 1% °C−1).65–67 Different types of luminescent thermometers including up-conversion nanoparticles or lanthanide ion-based coordination complexes, hybrid nanoparticles, organic dyes, quantum dots, and nano-diamonds have been widely investigated in recent decades. However, despite significant advancements in luminescent thermometry, examples of well-defined multifunctional nanoparticles that combine inorganic heaters with luminescent thermometers in close contact, capable of providing efficient heating triggered by external stimuli and cohesive temperature readouts, remain relatively scarce. Indeed, the development of multifunctional nano-systems combining nano-heaters and nanothermometers has evolved significantly over the past decade. Initially, research was conducted with the objective of measuring the temperature during the photo-thermal process using simple two-component systems comprising a separated nano-heater and thermometer dispersed together in a colloidal solution68 or introduced within cells.69 Plasmonic gold nanorods have been employed primarily as nano-heaters, and upconversion nanoparticles or quantum dots serving as nanothermometers. Similarly, gold or silver nanoparticles have been deposited on a surface and covered by a layer of silica or polymers containing Ln3+-based complexes as nanothermometers.70,71 The next step in development involved more structured assemblies, where gold nano-heaters (mainly plasmonic Au nano-objects) were paired with nanothermometers through electrostatic interactions. These assemblies often featured core–satellite structures, with a central nano-heater core surrounded by thermometer satellites72–76 or with a central thermometer core surrounded by nano-heater satellites,77 or both components situated on the surface of a support.78 However, these nano-objects often lack well-defined component structures, leading to aggregation and non-uniform distribution of satellites around the core or the components leaching, which can preclude the optimal functionality and reliability of the nano-heater/thermometer system. A significant advancement came with the creation of well-defined nano-systems where heating and temperature-sensing components were either integrated into a single nanoparticle79,80 or assembled into core–shell structures.81,82 These designs ensured precise, non-aggregated multifunctional nano-objects, with components positioned in close contact at exact distances, which is crucial for accurately determining the surface temperature of the nano-heaters. However, such sophisticated systems are relatively rare, highlighting an area of ongoing research and development.
In this article, we report on the design and investigation of new multifunctional heater/thermometer nano-objects containing a PB heater core enwrapped by a mesostructured silica shell loaded with a luminescent thermometer. Surprisingly, despite a few studies on the employment of luminescent species with PB nanoparticles (Ln3+-doped fluoride nanoparticles covered by a thin shell of PB in order to improve their heating ability83 or PB nanoparticles with anchored/loaded fluorescent organic dyes for imaging84–89), the combination of a PB heater with a luminescent thermometer has never been reported to date. The Tb3+/Eu3+ based nonanuclear luminescent coordination compound [(Tb/Eu)9(acac)16(μ3-OH)8(μ4-O)(μ4-OH)]·H2O (acac = acetylacetonate)90,91 was selected as the emissive thermometer for several reasons: (i) it possesses nine lanthanide ions and may be considered as a “lanthanide molecular aggregate” recognized for its improved photophysical properties,92 (ii) the combination of Tb3+ and Eu3+ ions (Tb3+/Eu3+ ratio = 9
:
1) within the same molecule ensures self-referenced capabilities and promotes Tb3+-to-Eu3+ energy transfer which is beneficial for temperature-dependent emission, (iii) it already demonstrated excellent thermometric capacity when inserted into dendritic silica nanoparticles with a high maximum relative thermal sensitivity (1.4% °C−1 at 43 °C), good photothermal stability and repeatability.93 Therefore, PB@SiO2 core@shell nanoparticles were functionalized with acac functions and then loaded with the luminescent [(Tb/Eu)9(acac)16(μ3-OH)8(μ4-O)(μ4-OH)]·H2O compound. The obtained hybrid nano-objects possess an excellent heating ability under irradiation at 808 nm, as well as a moderate specific heat capacity of 1.13 ± 0.03 J g−1 K−1, rendering them particularly compelling for applications requiring sustained and controlled thermal effects. Moreover, they exhibit bright luminescence in the visible region characteristic of Tb3+ and Eu3+ ions, which makes them multifunctional. The observed emission is temperature-dependent allowing the use of these nanoparticles as temperature nanoprobes in close proximity to the PB core with a satisfactory maximal relative sensitivity of 0.75% °C−1 at 20 °C.
2. Materials and methods
All the chemicals were purchased commercially and used without further purification. Sodium hexacyanoferrate decahydrate (Na4[Fe(CN)6]·10H2O, 99.99%), iron(III) chloride hexahydrate (FeCl3·6H2O, 97%), ammonium nitrate (NH4NO3, 99%) and cetyltrimethylammonium bromide (CTAB, 99%) were purchased from Sigma Aldrich (Steinheim, Germany); tetraethyl orthosilicate, Si(OEt)4 (TEOS, 99%), was purchased from Abcr GmbH (Karlsruhe, Germany); sodium hydroxide (NaOH, 98%) was purchased from Honeywell (Charlotte, North Carolina, US); ammonia (NH4OH, 28%) was purchased from VWR Chemicals (Radnor, Pensylvania, US), and ethanol (EtOH, 96%) was purchased from Merck (Darmstadt, Germany). The 3-[3-(triethoxysilyl)propyl]pentane-2,4-dione (acac-Si) was synthesized according to a previously published method.94 The synthesis of the [(Tb/Eu)9(acac)16(μ3-OH)8(μ4-O)(μ4-OH)]·H2O complex (Tb3+/Eu3+ = 9/1) was performed following a previously published method.90,95
2.1. Syntheses
Formation of pristine PB nanoparticles.
Pristine cubic PB nanoparticles of ca. 90 nm were prepared by using a co-precipitation method as previously reported.43,64,96,97 For this, aqueous solutions of FeCl3·6H2O (10.00 mM, 100 mL) and Na4[Fe(CN)6]·10H2O (11.25 mM, 100 mL) were added simultaneously to 100 mL of ultrapure water at a rate of 4 mL h−1, using a peristaltic pump (at 25 °C). Once the addition was complete, the NPs were collected by centrifugation (20
000 rpm, 10 min). The supernatant was removed and the NPs were washed 3 times with water and stored in water.
IR (KBr): ν(O–H) = 3635 cm−1 (coordinated water), ν(O–H) = 3390 cm−1 (crystallized water), ν(C
N) = 2088 cm−1 (FeIII–C
N–FeII), δ(O–H) = 1607 cm−1 (crystallized water), ν(FeII–CN) = 605 cm−1, δ(FeII–CN) = 503 cm−1. EDS: 11.8/88.2 (Na/Fe). Formula found: Na0.24FeIII[FeII(CN)6]0.81·xH2O. dTEM = 90 ± 12 nm.
Synthesis of PB@SiO2 nano-objects.
The process for coating the PB nanoparticles with a mesoporous silica shell was adapted from a previously published procedure.98 700 mg of cetyltrimethylammonium bromide (CTAB) were dissolved in a mix of ethanol 96% (75 mL) and ultrapure water (450 mL), and then left under stirring (700 rpm) at 35 °C overnight. 80 mg of PB NPs were added to the CTAB solution before the addition of 2 mL of TEOS and 250 μl of ammonia (30%). The mixture was stirred for 2 hours at 35 °C, and 2 h at 80 °C to allow the silica shell growth. Nanoparticles were then collected by centrifugation (20
000 rpm, 10 min). The CTAB extraction was performed through two cycles of dispersion (sonication 30 min) and centrifugation (20
000 rpm, 10 min) using a 6 g L−1 ammonia nitrate solution in EtOH. The nanoparticles were then washed with ultrapure water and centrifuged at 20
000 rpm for 10 min and redispersed in water and centrifuged at low speed (8000 rpm, 15 min) to remove side silica nanoparticles. Three cycles of dispersion and low speed centrifugation were performed. The obtained PB@SiO2 nanoparticles were then stored in water.
IR (KBr): δ(Si–O–Si) = 476 cm−1 (SiO2), ν(Si–O–Si) = 800–1087 cm−1 (SiO2), ν(C–H) = 2800–3000 cm−1 (residual CTAB), δ(CH2,CH3) = 1415 cm−1 (CTAB), ν(C
N) = 2088 cm−1 (FeIII–C
N–FeII), δ(O–H) = 1607 cm−1 (crystallized water), ν(FeII–CN) = 605 cm−1, δ(FeII–CN) = 503 cm−1. dTEM = 132 ± 16 nm. EDS: Si/Fe = 62.5/37.5.
Synthesis of PB@SiO2-acac nano-objects.
The post-functionalization of the silica shell by the acetylacetonate (acac) moieties was performed by dispersing 10 mg of PB@SiO2 nanoparticles in 4 mL of ethanol and 40 mL of toluene, and adding 100 μL of acac-Si (0.31 mmol). The suspension was then refluxed (110 °C) under magnetic stirring (700 rpm) overnight. The nanoparticles were then collected by centrifugation (20
000 rpm, 10 min) and washed three times in EtOH. The obtained nanoparticles were redispersed and stored in EtOH.
IR (KBr): δ(Si–O–Si) = 475 cm−1 (SiO2), ν(Si–O–Si) = 801–1092 cm−1 (SiO2), ν(C
C) = 1635 cm−1 (acac-Si), ν(C
O) = 1695 cm−1 (acac-Si, keto), ν(C–H) = 2800–3000 cm−1 (acac-Si, residual CTAB), ν(C
N) = 2088 cm−1 (FeIII–C
N–FeII), δ(O–H) = 1607 cm−1 (crystallized water), ν(FeII–CN) = 605 cm−1, δ(FeII–CN) = 503 cm−1. dTEM = 139 ± 16 nm. EDS: Si/Fe = 65.1/34.9.
Synthesis of PB@SiO2-acac/(Tb/Eu)9 nano-objects.
The encapsulation of the [(Tb/Eu)9(acac)16(μ3-OH)8(μ4-O)(μ4-OH)]·H2O (Tb3+/Eu3+ = 9/1) compound, symbolized here as (Tb/Eu)9, was performed by adding 15.6 mg of the complex to the PB@SiO2-acac nanoparticles (5 mg) dispersed in 20 mL of MeOH. The mixture was stirred at 65 °C under reflux for 2 h. The (Tb/Eu)9 complex-containing nanoparticles, PB@SiO2-acac/(Tb/Eu)9, were washed twice with water (20
000 rpm, 10 min) and finally dried at 80 °C for 48 h.
IR (KBr): δ(Si–O–Si) = 477 cm−1 (SiO2), ν(Si–O–Si) = 800–1091 cm−1 (SiO2), ν(C
C) = 1655 cm−1 (acac-Si), ν(C
O) = 1523 cm−1, ν(C
O) = 1695 cm−1 (acac-Si), ν(C–H) = 2800–3000 cm−1 (acac-Si, residual CTAB), ν(C
N) = 2088 cm−1 (FeIII–C
N–FeII), δ(O–H) = 1607 cm−1 (crystallized water), ν(FeII–CN) = 604 cm−1, δ(FeII–CN) = 503 cm−1. dTEM = 138 ± 17 nm. EDS: Si/Fe = 71.0/28.9; Fe/Tb/Eu = 76.5/21.4/2.1.
2.2. Physical methods
Characterization.
Transmission electron microscopy (TEM) images were recorded at 100 kV (JEOL 1200 EXII). Samples for TEM measurements were deposited from suspensions onto copper grids and allowed to dry before observation. The size distribution histograms were determined using enlarged TEM micrographs taken at a magnification of 100 K on a statistical sample of 200 to 300 nanoparticles. SEM/EDS microscopy was performed on an FEI Quanta FEG 200 instrument (Hillsboro, Oregon, US). The powders were deposited on an adhesive carbon film and analysed under high vacuum. The quantification of heavy elements was carried out using Oxford Instruments AZTEC software (Abingdon-on-Thames, UK), with a dwell time of 3 μs. HRTEM and STEM-EDS measurements were performed on a JEOL 2200 FS microscope (Tokyo, Japan) and an Oxford Instruments SDD EDX detector XMaxN 100 TLE (100 mm2 – windowless) (Abingdon-on-Thames, UK). Infrared (IR) spectra using attenuated total reflectance (KBr-IR) were recorded using powdered samples with a PerkinElmer Spectrum Two FT-IR Spectrometer (Waltham, Massachusetts, US) with four acquisitions at a resolution of 4 cm−1. Ultraviolet-visible spectroscopy (UV-vis) spectra were collected on a JASCO V-650 (Tokyo, Japan) spectrometer. X-Ray diffraction (XRD) powder patterns were recorded using a PANalycal X’pert MDP-Pro diffractometer in Bragg Brentano geometry with Ni filtered Cu-Kα radiation (Kα1 = 1.54059 Å, Kα2 = 1.54443 Å). Measurements were performed at room temperature in a 2θ range of 10°–60° using a step size of 0.033° (2θ) and a counting time per step of 1 s. The XRD patterns were analyzed by pattern matching using the Fullprof software.99 The crystallite size was determined using the Williamson–Hall formula implemented in the same software using a pseudo-Voigt Cox–Thompson function while taking into consideration the instrumental broadening obtained using a reference (Y2O3 powder annealed at 1400 °C for 36 h). Nitrogen adsorption and desorption isotherms at 77 K were measured using a TriStar 3000 (V6.06 A) instrument. The samples were first dried under vacuum at 80 °C for 12 hours. The specific surface area (SBET) was determined using the Brunauer–Emmett–Teller (BET) method, while the pore size distribution was derived from the desorption branch of the nitrogen isotherms based on the Barrett–Joyner–Halenda (BJH) equation.
Photoluminescence and thermometry measurements.
Emission and excitation spectra were measured at room temperature (20 °C) in the solid state, using a Edinburgh FLS-1000 spectrofluorometer (Edinburgh, UK). The excitation source was a 450 W ozone free Xe arc lamp. The spectra were corrected for the detection and optical spectral response of the spectrofluorometer. Photoluminescence measurements as a function of temperature (luminescent thermometry) were performed by using the temperature setup incorporated into the Edinburgh spectrofluorometer. Emission spectra were recorded in the temperature range from 20 to 80 °C. At each temperature step, a period of 5 min was given to allow the temperature to stabilize, and then 1 emission spectrum was recorded with a dwell time of 0.2 s and a step of 1 nm.
Specific heat.
Specific heat was determined under an argon atmosphere using a differential scanning calorimeter, DSC 404C Pegasus manufactured by NETZSCH. The samples were pelletized (6 mm diameter with a load of 1.5 T for 30 s) and loaded into an aluminium crucible covered with a lid. The lid is pierced to allow gas exchange during the experiment. The temperature profile is as follows: an isotherm at 40 °C is maintained for 10 minutes and is followed by an increase at a rate of 20 °C min−1 to 250 °C. The setpoint is maintained for 10 min. At minimum 7 cycles are measured. The first cycle is not considered due to losses of water from the samples. The data are exploited following the ratio method on the linear temperature range of 60–150 °C to determine the specific heat.
Photothermia.
Photothermia experiences were realized using a laser with a wavelength of 808 nm and laser powers of 0.80 W cm−2, 1.00 W cm−2, 1.33 W cm−2 and 1.83 W cm−2. A 100 μg mL−1 PB core solution was deposited onto the glass surface as a thin film and left to dry before the measurements. The temperature was measured by using a thermal infrared camera with points taken every second during all thermal cycles.
Light-to-heat conversion coefficient (η).
To determine the photothermal conversion efficiency 54 μg of PB@SiO2-acac/(Tb/Eu)9 nanoparticles were deposited on a glass surface. The deposit had a circular shape with an area of 0.32 cm2. It was irradiated with a power of 2.58 W cm−2 at a wavelength of 808 nm. The temperature data obtained from the thermal camera was processed using a model of a glass slide with a thin layer on top, developed and solved in COMSOL software.100 The resulting fit was used to extract η.
3. Results and discussion
3.1. Synthesis of multifunctional PB@SiO2 core@shell nanoparticles loaded with a luminescent [(Tb/Eu)9(acac)16(μ3-OH)8(μ4-O)(μ4-OH)] compound
The synthesis of multifunctional core@shell PB@SiO2 nanoparticles loaded with the luminescent compound [(Tb/Eu)9(acac)16(μ3-OH)8(μ4-O)(μ4-OH)]·H2O inside the porosity of the silica shell was designed using a four-step approach as depicted in Scheme 1. It consists of: (i) the synthesis of the pristine cubic PB nanoparticles, (ii) their coating by a mesoporous silica shell to obtain PB@SiO2 nano-objects, (iii) the functionalization of the silica pores with the acetylacetonate moiety, and (iv) the loading of the luminescent [(Tb/Eu)9(acac)16(μ3-OH)8(μ4-O)(μ4-OH)]·H2O compound inside the silica porosity. The mesoporous silica shell was used to enwrap the PB heater core and encapsulate the luminescent thermometer because: (i) its porosity permits loading of the luminescent complex inside the pores assuring its homogeneous distribution, (ii) the possibility of functionalizing the pores to render them less hydrophilic and ensure the retention of the lanthanide complex inside the pores, (iii) a good thermal shock resistance and a near zero thermal expansion, and (iv) the optical transparency required for both light activation and luminescent thermometry.101–103
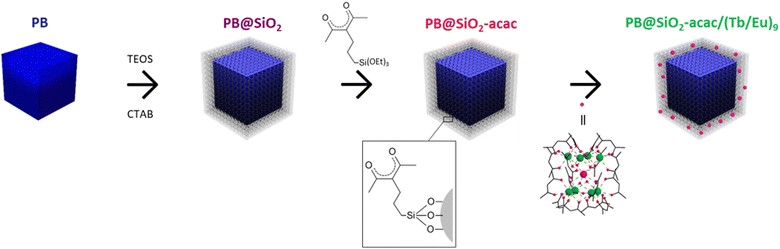 |
| Scheme 1 Schematic representation of the four-step approach employed for the synthesis of multifunctional core@shell PB@SiO2-acac nanoparticles loaded with luminescent [(Tb/Eu)9(acac)16(μ3-OH)8(μ4-O)(μ4-OH)] compound. | |
Pristine cubic PB nanoparticles were prepared by using a co-precipitation method in water with controlled addition of Na4[Fe(CN)6]·10H2O and FeCl3·6H2O precursors.43,64,96,97 This method permits cubic nanoparticles of the formula Na0.24FeIII[FeII(CN)6]0.81·xH2O to be obtained (see the Materials and methods section for details). Secondly, the mesoporous silica shell was grown on the surface of the PB nanoparticles by using a sol–gel process adapting a previously reported procedure.98 The subsequent post-grafting with the acac moiety was performed by adding acac-siloxane to give PB@SiO2-acac nanoparticles. The acac functionalization of the silica is necessary since the complex is not retained in the silica pores without functionalization. Then, the [(Tb/Eu)9(acac)16(μ3-OH)8(μ4-O)(μ4-OH)]·H2O complex with the Tb/Eu ratio = 9/1 (which is denoted as (Tb/Eu)9) was loaded into the silica pores by its impregnation during 2 h in methanol at 65 °C to obtain PB@SiO2-acac/(Tb/Eu)9 nano-objects. They present the loading of 0.13 units of (Tb/Eu)9 complex per SiO2 unit (i.e. 0.11 of Ln3+ per unit of SiO2).
Infrared (IR) spectroscopy was performed to characterize the samples at each step of the PB@SiO2-acac/(Tb/Eu)9 nano-objects’ preparation (Fig. 1). The typical stretching vibrations of the bridging cyanide group ν(FeII–CN–FeIII) at 2090 cm−1, along with the ν(FeII–C) and the δ(FeII–CN) bands at 605 cm−1 and 503 cm−1 are clearly present in the IR spectra of all samples indicating that the PB network was preserved after silica shell coating, acac functionalization and complex loading. The characteristic silica vibrations, ν(Si–O–Si) at 1090 and 800 cm−1, δ(Si–O–Si) at 475 cm−1 and ν(Si–OH) at 972 cm−1 appear in the spectrum recorded for the PB@SiO2 sample after silica coating. The bands from the grafted acac moieties (ν(C
O) at 1700, 1690 and 1630 cm−1 in the keto and enol forms and ν(C
C) in the 1350–1400 cm−1 range, as well as the C–H vibrations between 2900–3000 cm−1 characterize their successful anchoring into the silica porosity. The IR spectrum of PB@SiO2-acac/(Tb/Eu)9 containing the incorporated luminescent [(Tb/Eu)9(acac)16(μ3-OH)8(μ4-O)(μ4-OH)]·H2O complex shows the decrease in the relative intensity of ν(C
O) vibrations of the free acac moiety at 1700 cm−1 in comparison to the one of the band at 1630 cm−1, as well as the appearance of the ν(C
O) at 1520 cm−1 band (the coordinated enol form of acac in the complex) attesting the presence of the complex inside the pores (Fig. 1 and Fig. S1, ESI†). However, the presence of δ(H–O–H) situated in the 1620–1605 cm−1 region renders the interpretation of the IR spectra related to the attribution of the acac form and conclusion on the coordination of the lanthanide of the complex to the acac groups of the silica difficult. Note that similar results have been obtained for stellate-like silica nanoparticles loaded with the same complex.93
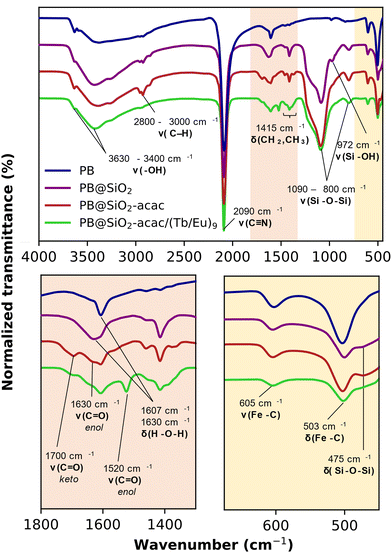 |
| Fig. 1 IR spectra of different steps in the synthesis of PB@SiO2-acac/(Tb/Eu)9 nano-objects. | |
The powder X-ray diffraction (PXRD) pattern showed the typical fcc (space group Fm
m) structure indexed in the Fm
m (01-073-0689) space group with a lattice parameter of a = 10.1633(0) Å for the pristine PB, nano-objects (Fig. 2a).
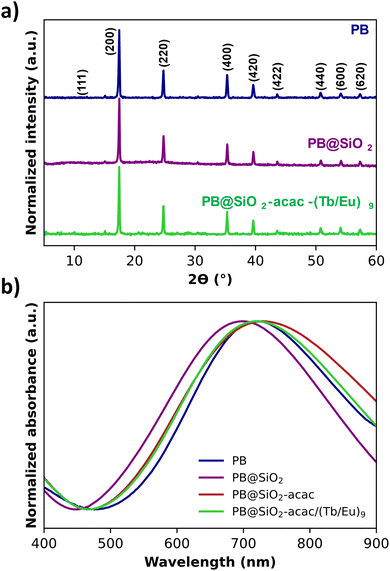 |
| Fig. 2 (a) Powder X-ray diffraction patterns for the pristine PB, PB@SiO2 and PB@SiO2-acac/(Tb/Eu)9 nano-objects, and (b) absorption spectra in water for suspensions of PB, PB@SiO2, PB@SiO2-acac and PB@SiO2-acac/(Tb/Eu)9 nano-objects in the visible region. | |
This value did not drastically change after the silica coating, functionalization and complex loading: the value of 10.1625(2) Å is found for the PB@SiO2-acac/(Tb/Eu)9 sample (Table 1). This fact indicates that the cyano-bridged structure of the PB nanoparticles was preserved during the silica shell coating and the complex loading. The crystalline domains were calculated from the Williamson–Hall formula for the PB@SiO2-acac/(Tb/Eu)9 sample giving an average size value of ca. 90 nm. This size is in agreement with what is determined from the transmission electronic microscopy (TEM) measurements (see later).
Table 1 Main characteristics for PB, PB@SiO2, PB@SiO2-acac and PB@SiO2-acac/(Tb/Eu)9 nano-objects
Nano-objects |
a
(Å) |
PB core sizebc (nm) |
SiO2 shell thicknessb (nm) |
Total sizec (nm) |
Lattice a parameter obtained from PXRD.
The core size and shell thickness were measured directly from TEM images.
Obtained as the mean edge of the cube.
|
PB |
10.1633(0) |
90 ± 12 |
— |
90 ± 12 |
PB@SiO2 |
10.1628(1) |
91 ± 12 |
18 ± 4 |
132 ± 16 |
PB@SiO2-acac |
— |
90 ± 12 |
19 ± 4 |
139 ± 16 |
PB@SiO2-acac/(Tb/Eu)9 |
10.1625(2) |
91 ± 12 |
18 ± 4 |
138 ± 17 |
The absorption electronic spectra measured for all samples showed a classical broad band in the range 400–900 nm with a maximum at 723 nm for the pristine PB nanoparticles (Fig. 2b). This band is usually ascribed to the intervalence Fe2+-to-Fe3+ charge transfer through the cyanide bridge. Its maximum is shifted toward lower wavelengths for PB@SiO2 after silica shell coating, which is in agreement with the previously reported results for the silica coated PB nanoparticles.98 After the subsequential acac functionalization and complex loading, this band is red shifted (Fig. 2b).
TEM observations were carried out in order to evaluate the size and morphology of the obtained nanoparticles. TEM images obtained for the pristine PB nanoparticles show typical well defined cubic shaped nano-objects with the mean edge of the cube of 90 ± 12 nm (Fig. 3a and Fig. S2a, ESI† for size distribution histogram). Each single core was then coated by a mesoporous silica shell to provide core@shell PB@SiO2 nanoparticles with a whole diameter of 132 ± 16 nm (see Fig. 3b and Fig. S2b, ESI† for size distribution histograms). The PB core size was almost not changed after the silica coating (91 ± 12 nm, Fig. S3a, ESI†) and the silica shell thickness obtained from the TEM images is equal to 18 ± 4 nm (Fig. S4a, ESI†). The TEM image for these PB@SiO2 nanoparticles reveals the expected presence of the silica shell's mesoporosity. The grafting of the acac moiety (see Fig. 3c and Fig. S2c, S3b and S4b, ESI† for size distribution histograms) and the loading of the Tb3+/Eu3+ complex inside the silica pores (Fig. 3d and Fig. S2d, S3c and S4c, ESI† for size distribution histograms) do not modify the morphology and the size of the nano-objects. Indeed, the PB core size for the PB@SiO2-acac/(Tb/Eu)9 nanoparticles is equal to 91 ± 12 nm and the silica shell thickness is equal to 18 ± 4 nm. The sizes of the cores and shells and the whole sizes of all obtained nanoparticles are gathered in Table 1.
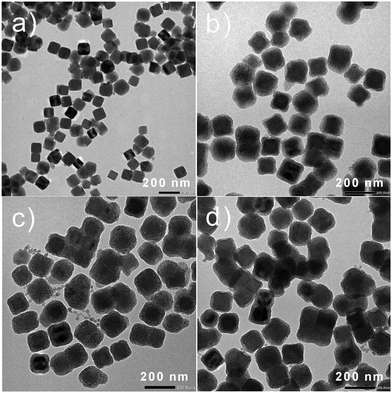 |
| Fig. 3 (a) TEM images for PB, (b) PB@SiO2, (c) PB@SiO2-acac and (d) PB@SiO2-acac/(Tb/Eu)9 nano-objects. | |
The obtained nanoparticles were characterized by HAADF-STEM with EDS mapping, which allowed us to visualize the presence of the silica shell (pink colour for Si atoms) around the PB core (Fe atoms are depicted in yellow), as well as the homogeneity of the atomic distribution of terbium (orange colour) and europium atoms (green colour) confirming the encapsulation of the Tb3+/Eu3+ complex within the silica shell (Fig. 4).
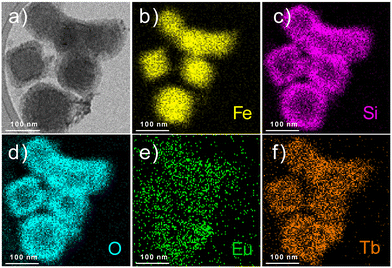 |
| Fig. 4 (a) STEM-bright field image and STEM-EDS elemental mapping of PB@SiO2-acac/(Tb/Eu)9 nano-objects with topochemical distribution of (b) Fe (yellow), (c) Si (pink), (d) O (blue), (e) Eu (green) and (f) Tb (orange). | |
Nitrogen sorption measurements on the nano-objects provide an estimation of the effect of compound incorporation in the porosity of the silica shell. As observed through TEM, PB@SiO2 appears to exhibit porosity, which is confirmed by the specific surface area of 371 m2 g−1 (Fig. S5, ESI†). After the complex loaded, the specific surface area decreased to 245 m2 g−1 for PB@SiO2-acac/(Tb/Eu)9, indicating that the complex was successfully incorporated into the pores of the silica shell.
3.2. Photothermal properties and determination of the specific heat capacity
The macroscopic photothermal properties were investigated for the PB@SiO2-acac/(Tb/Eu)9 nano-objects and compared with the ones of the pristine PB nanoparticles. Note that while the macroscopic photothermal properties of PB nanoparticles coated by silica shell have already been reported in the literature,98,104,105 a clear comparison between PB and silica coated PB nano-objects has never been published before. The nanoparticles were deposited on a glass surface and submitted to laser irradiation under 808 nm with different laser powers ranging from 0.8 to 1.83 W cm−2 and the macroscopic temperature rise was monitored by using a thermal camera.106,107 An important temperature increase was observed for PB@SiO2-acac/(Tb/Eu)9 nano-objects under irradiation after only a few minutes. For instance, ΔT of 20.4 °C was observed under 3 min irradiation with the laser power of 1.83 W cm−2, while no obvious heating effect was detected on the surface without nanoparticles (Fig. 5a). In comparison, PB nanoparticles without a silica shell provide higher macroscopic temperature elevation with ΔT of 40.1 °C under the same conditions. Of note, the absorption levels of both the bare PB nanoparticles and the silica coated ones were similar, 29% and 27%, respectively. This result is in agreement with the work of S. Merabia et al., which demonstrated that the thickness of the silica shell highly impacts the heat transfer of Au core silica shell nanoparticles at nano- and macroscales.108 In this work, the authors showed that a very thin silica shell is most favourable for efficient heat transfer even in comparison with the pristine Au nanoparticles, while a thicker silica shell decreases macroscopic photothermal efficiency. As expected, both PB@SiO2-acac/(Tb/Eu)9 and PB nano-objects present a linear increase in the ΔT value as a function of laser power (Fig. 5b), which is rather common with other photothermal nano-objects. The photothermal conversion efficiency η of 69% was extracted using a deposit of 54 μg of PB@SiO2-acac/(Tb/Eu)9 nano-objects on a glass surface (see the Materials and methods section and Fig. S6, ESI†). Note that this efficiency depends on several factors, including the nature of the nanoparticles, their size, shape, interface (core/shell), environment, solvents, etc. For instance, in our previous studies, we showed that PB nanoparticles of ca. 81 nm in aqueous solution presented a light-to-heat conversion efficiency of 57%,64 and those deposited on the surface demonstrated an efficiency of 50%.109 Note that the value of 69% is comparable to what is found for Au nanocages, regarded as a benchmark for photothermal agents.110
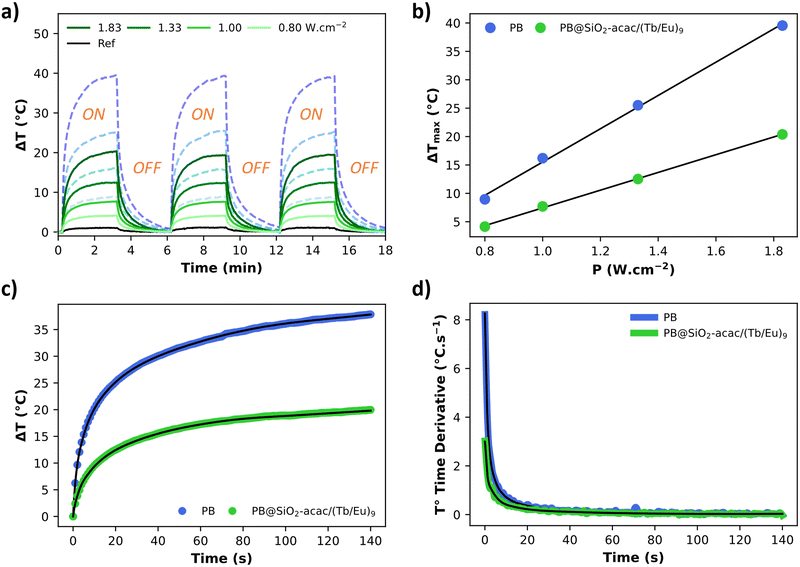 |
| Fig. 5 (a) Photothermal properties presented as ΔT vs. time curve for PB (blue dotted lines) and PB@SiO2-acac/(Tb/Eu)9 (green full lines) nano-objects deposited on the glass surface under irradiation at 808 nm (0.80 W cm−2, 1.00 W cm−2, 1.33 W cm−2 and 1.83 W cm−2), measured over three cycles of irradiation/relaxation with the laser source, (b) ΔT measured for PB and PB@SiO2-acac/(Tb/Eu)9 nano-objects after 3 min of laser irradiation at 808 nm as a function of laser power, (c) experimental data for ΔT vs. time curve measured for PB and PB@SiO2-acac/(Tb/Eu)9 nano-objects under irradiation at 808 nm with the laser power of 1.83 W cm−2 and its numerical solution of eqn (1) obtained from the fit of the thermal derivative curve fit (black lines), and (d) derivative of the temperature as a function of the time with its fit obtained using eqn (3)–(5). | |
In order to obtain a deeper understanding of the heat transfer of the PB and PB@SiO2-acac/(Tb/Eu)9 nanoparticles, we determined their specific heat capacities. This property corresponds to the amount of heat energy required to raise the temperature of a unit mass of a substance by one degree celsius (°C) or one kelvin (K). It varies significantly between different materials and inversely affects the temperature change resulting from light absorption. Consequently, it influences the efficiency and dynamics of the light-to-heat transfer process and the heat retention. First, the specific heat capacity (Cp) of the PB nanoparticles was measured by using DSC in the linear temperature range 60–150 °C by using 5 heating/cooling cycles (Fig. S7, ESI†) (see the Methods and materials section for details). The obtained Cp value is equal to 0.92 ± 0.03 J g−1 K−1, which is close to the values usually given for bulk metal organic framework materials (0.75–0.85 J g−1 K−1). In comparison, metallic gold provides a much lower Cp value of 0.129 J g−1 K−1. It signifies that gold and PB nanoparticles behave differently regarding their photothermal properties. Indeed, without taking into account the interface thermal resistivity, gold nanoparticles very rapidly convert light to heat and transfer it to the macroscopic environment. In contrast, PB nano-objects require more energy to reach the same temperature as Au nanoparticles, but due to their higher heat capacity, once heated, they can store more thermal energy and release this stored heat at a slower pace compared to gold nanoparticles. Therefore, PB nanoparticles could have advantages for prolonged or localized heating phenomena, such as enhanced hot spot effect formation or maintaining stable local thermal conditions.
The specific heat capacity for PB@SiO2-acac/(Tb/Eu)9 nanoparticles was determined from their photothermal properties by taking an approximation that the PB core keeps its initial Cp value unchanged after the silica coating and the complex loading. The absorption of the silica shell is neglected. It is also important to note that Cp and Cv are equivalent for a solid. We used the heat equation:
| 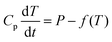 | (1) |
where
Cp is the heat capacity of the sample,
P is the heat source power and
f is the heat exchange function which takes into account the exchange of energies between the system and the environment. In our case, the heat equation is applied to a thin layer of nano-objects in contact with 2 environments, the glass layer and the air. These two interfaces contribute to a high degree of complexity in the exchange function. If we consider that the exchange function is
N times derivable, then it can be expressed as a Taylor series of the
f function at the point
T0:
| 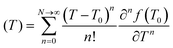 | (2) |
The thermodynamic principles indicate that the first order of this series is zero. Then, this equation can be rewritten with unknown constants
an:
| 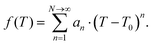 | (3) |
Fig. 5c shows experimental data of Δ
T vs. time curve for heating of PB and PB@SiO
2-acac/(Tb/Eu)
9 nano-objects under laser irradiation with a laser power
P of 1.83 W cm
−2. The temperature variation as a function of time for PB nanoparticles could be written as:
| 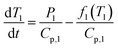 | (4) |
and for PB@SiO
2-acac/(Tb/Eu)
9 nano-objects as:
| 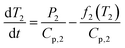 | (5) |
It is established that the
f function is zero at the point
t = 0 s. Furthermore, if the derivative of the temperature is taken (see
Fig. 5d), the ratio
P/
Cp can be extracted from the initial point. Therefore,
P1 and
P2 depend on: (i) the source light power (1.83 W cm
−2), (ii) the absorption of the layer and (iii) the light-to-heat conversion coefficient
η. The absorption of a layer, designated as layer
i, will be referred to as
Ai. Therefore,
P1 and
P2 can be expressed as:
| P1 = A1·Plaser·η1 and P2 = A2·Plaser·η2 | (6) |
In the case of PB, the total heat capacity depends on its mass and its mass-specific heat capacity:
In the case of PB@SiO
2-acac/(Tb/Eu)
9 nano-objects, the total heat capacity can take into account the mass of PB and SiO
2 and the mass-specific heat capacity of PB and SiO
2, while the contribution of organic function and luminescent compound loaded could be neglected:
| Cp,2 = mPB,2·Cp,PB + mSiO2·Cp,SiO2 | (8) |
From the thermal derivative curves displayed in
Fig. 5d we can extract the following ratio:
|  | (9) |
If we assume that the light-to-heat conversion efficiency is the same for the PB nanoparticles and the PB core in the PB@SiO
2-acac/(Tb/Eu)
9 nano-objects, and that the mass of PB is proportional of the light absorption for both samples, we can write:
| 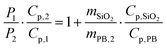 | (10) |
The aforementioned equation can be employed in conjunction with the photothermal experiment and the EDS, which permits the extraction of the mass ratio between silica and PB, in order to ascertain the mass heat capacity of PB.
| 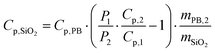 | (11) |
With
eqn (8) and (11) we obtain specific heat capacities of 1.31 ± 0.04 J g
−1 K
−1 and 1.13 ± 0.03 J g
−1 K
−1 for the silica shell and the PB@SiO
2-acac/(Tb/Eu)
9 nano-objects, respectively.
It is noteworthy that the derivative thermal curve can be fitted using eqn (3)–(5) (black lines in Fig. 5d). Utilizing the extracted f function from the fit, the solution to eqn (1) can be derived. Subsequently, the temperature as a function of time can be obtained (black lines in Fig. 5c). Note that the obtained specific heat capacity for the silica shell is in agreement with the values given for porous silica in the literature. Therefore, the increased Cp value of the PB@SiO2-acac/(Tb/Eu)9 nano-objects relative to bare PB nanoparticles is consistent with the enhanced heat retention offered by the silica shell, which explains the lower macroscopic heating of these nano-objects.
3.3. Luminescence and luminescent thermometry
The photoluminescence of PB@SiO2-acac/(Tb/Eu)9 nanoparticles was investigated in the solid state first at room temperature in order to confirm the encapsulation of the luminescent compound within silica mesoporosity, and secondly in the 20–80 °C temperature range in order to demonstrate the thermometric ability of the nanoparticles. The solid-state excitation spectra at room temperature were recorded by monitoring the main 5D0 → 7F2 and 5D4 → 7F5 transitions of Eu3+ (at 615 nm) and Tb3+ (at 545 nm), respectively (Fig. 6). They exhibit a dominant broad band with a maximum at 308 nm, which can be ascribed to the acac ligand excited state. This fact attests the efficient excitation through the acac “antenna” sensitization. Besides, sharp low intensity bands can be observed, which can be attributed to the 4f transitions of both lanthanide ions. Note also that the Tb3+ characteristic transitions appear when the spectra are recorded by monitoring the main emission of Eu3+ at 615 nm. All these characteristics appear in the excitation spectrum of the free [(Tb/Eu)9(acac)16(μ3-OH)8(μ4-O)(μ4-OH)]·H2O compound as illustrated in Fig. S8, ESI.† However, for the latter, 4f transitions appear much more pronounced. Previously observed with the [(Tb/Eu)9(acac)16(μ3-OH)8(μ4-O)(μ4-OH)]·H2O compound encapsulated inside stellate silica nanoparticles,93 this effect was interpreted as an antenna effect enhancement through the acetylacetonate-functionalized silica matrix sensitization. The solid-state emission spectra of PB@SiO2-acac/(Tb/Eu)9 nanoparticles were recorded at room temperature upon excitation at 308 nm (in the acac-linked sensitization) since this excitation band is operational for both lanthanide ions (Fig. 6). The emission spectrum demonstrates a series of expected Tb3+ 5D4 → 7F6-0 characteristic emission lines (labelled in green) with the most intense 5D4 → 7F5 transition, as well as Eu3+ 5D0 → 7F0-4 characteristic transitions (labelled in red) with the most intense band situated at ca. 615 nm (5D0 → 7F2). In comparison with the solid-state emission spectrum of the free [(Tb/Eu)9(acac)16(μ3-OH)8(μ4-O)(μ4-OH)]·H2O compound, the relative intensities of the main Tb3+ 5D4 → 7F5 transition decreases, while the intensities of two main Eu3+ characteristic transitions (5D0 → 7F2 and 5D0 → 7F4) increase. For instance, the relative intensities of the Tb3+ emissive transitions to the Eu3+ emissive ones at room temperature are much lower in PB@SiO2-acac/(Tb/Eu)9 nanoparticles compared to the free complex: I550/I615 goes from 2.2 to 0.65 and I550/I700 goes from 9 to 1.5 for [(Tb/Eu)9(acac)16(μ3-OH)8(μ4-O)(μ4-OH)]·H2O and PB@SiO2-acac/(Tb/Eu)9 respectively (Fig. 6 and Fig. S8, ESI†). This means that the relative signals from the Eu3+ transitions have been multiplied by 3.4 at 615 nm and by 6 at 700 nm, compared to the one at 550 nm from the Tb3+. These modifications in the emission spectrum after complex loading can be attributed to the action of three potential effects occurring in the investigated system: (i) the expected boost of the Tb3+-to-Eu3+ energy transfer after insertion of the complex into the silica shell. This effect has previously been observed for acac-functionalized stellates silica nanoparticles loaded by [(Tb/Eu)9(acac)16(μ3-OH)8(μ4-O)(μ4-OH)]·H2O and explained by the slight modifications in the complex geometry occurred after encapsulation,93 (ii) the possible energy transfer from Tb3+ to the silica host, which has previously been observed in the case of lanthanide-based complexes inserted into the silica matrix,111 and (iii) the presence of PB nanoparticle absorption, occurring between 600 and 900 nm.
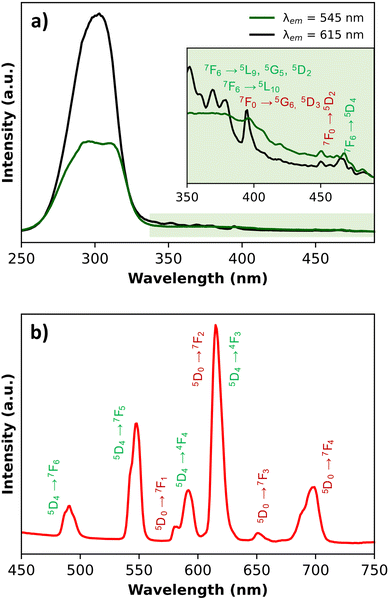 |
| Fig. 6 (a) Excitation spectra of PB@SiO2-acac/(Tb/Eu)9 nanoparticles monitored at λem = 545 (green) and λem = 615 nm (black) measured in the solid state at room temperature. Inset: Magnification of the excitation spectra between 350 and 480 nm showing the 4f-transitions; (b) emission spectra of PB@SiO2-acac/(Tb/Eu)9 nanoparticles obtained at room temperature (red) in the solid state with excitation at λexc = 308 nm. Eu3+ and Tb3+ linked transitions are shown in red and green, respectively. | |
Secondly, the photoluminescence was investigated in the 20–80 °C temperature range to verify the potential of these nano-objects to act as luminescent temperature probes. Fig. 7a demonstrates the emission spectra of PB@SiO2-acac/(Tb/Eu)9 nanoparticles measured under 308 nm excitation in the 20–80 °C range (Fig. 7a), where the intensities are temperature dependent. The corresponding temperature dependence of the luminescence intensity ratio (LIR) between 5D4 → 7F6 of Tb3+ (the integrated areas 480–505 nm) and 5D0 → 7F4 of Eu3+ (the integrated areas 603–635 nm) emissions providing the best results for thermometry is shown in Fig. 7b.
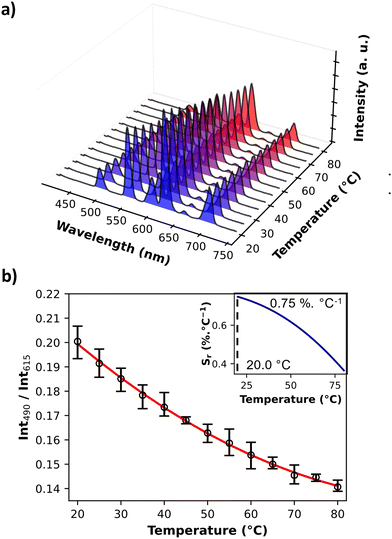 |
| Fig. 7 (a) Emission spectra (λex = 308 nm) of PB@SiO2-acac/(Tb/Eu)9 nanoparticles in the 20 to 80 °C temperature range; (b) corresponding LIR between the 5D4 → 7F6 (Tb3+) and 5D0 → 7F2 (Eu3+) transitions. The solid line represents a second-degree polynomial fitting. Integrated areas: 480–505 nm (Tb3+: 5D4 → 7F6) and 603–635 nm (Eu3+: 5D0 → 7F2). Inset: Temperature dependence of Sr. The error bars correspond to the standard error of the mean determined from three consecutive temperature cycles. | |
It is important to note that the temperature dependent amplitude of 5D4 → 4F3 of Tb3+ is significantly less than that of the 5D0 → 7F4 of Eu3+. The error bars represent the standard deviation of average values obtained upon three consecutive temperature cycles. The temperature-dependent variation of the LIR parameters shows a second-degree polynomial correlation in the full temperature range 20–80 °C, while a linear correlation occurs between 20 and 40 °C. The relative thermal sensitivity value (Sr) is usually used as the characteristic, allowing a comparison of the different luminescent thermometer efficiencies.112 The Sr parameter is the variation of LIR per degree of temperature, expressed by the equation:
Sr(T) = |∂LIR(T)/∂T|/LIR(T) |
The temperature dependence of
Sr is shown in
Fig. 7b. The maximum on this curve obtained from the calibration data corresponds to the maximal relative sensitivity (
Srmax), which is equal to 0.75% °C
−1 at 20 °C. This value is rather close to the maximal relative thermal sensitivity of ∼1% °C
−1 frequently considered as the benchmark for efficient luminescent thermometers.
66
The thermal uncertainty was also calculated as the smallest temperature change that can be measured, i.e.:
δT = |δLIR(T)/LIR(T)|/Sr(T) |
The measured thermal uncertainty is equal to 1.01 °C. The calibration parameters for PB@SiO
2-acac/(Tb/Eu)
9 nanoparticles are gathered in
Table 2.
Table 2 Calibration parameters for thermometry measurements for PB@SiO2-acac/(Tb/Eu)9 nanoparticles
|
PB@SiO2-acac/(Tb/Eu)9 |
Temperature operating range |
20–80 °C |
Maximal relative sensitivity (Sr) with LIR490/615 |
0.75% °C−1 at 20 °C |
Maximal relative sensitivity (Sr) with LIR545/615 |
0.63% °C−1 at 20 °C |
Best uncertainty of LIR (δLIR) |
0.0012 |
Best uncertainty of temperature (δT) |
1.01 °C |
4. Conclusion
This work presents the synthesis and investigation of new multifunctional heater@thermometer nano-objects obtained by coating PB nanoparticles with a mesoporous silica shell, followed by their covalent grafting with the acetylacetonate moieties and the loading of the luminescent compound [(Tb/Eu)9(acac)16(μ3-OH)8(μ4-O)(μ4-OH)]·H2O inside the pores. The functionalized silica shell plays a crucial role in hosting and protecting the luminescent compound and allowing its homogeneous distribution around the PB core.
The as-obtained multifunctional nanoparticles present a whole size of ca. 138 nm with a cubic PB core of ca. 91 nm and a well distinguished silica shell of ca. 18 nm. The PB nanoparticles retain their initial cubic morphology and size after the silica shell coating, its functionalization and luminescent compound loading. It is worth noting that the obtained hybrid nano-objects present a well-defined single core coated by a well-defined mesoporous silica shell. The encapsulation of [(Tb/Eu)9(acac)16(μ3-OH)8(μ4-O)(μ4-OH)]·H2O complexes ca. 13% (vs. SiO2, i.e. 0.11 unit of Ln3+ per unit of SiO2) was attested by an ensemble of characterizations, including IR spectroscopy, STEM-EDS atomic mapping, elemental and EDS analyses. The STEM-bright field images attest the homogenous distribution of the [(Tb/Eu)9(acac)16(μ3-OH)8(μ4-O)(μ4-OH)] compound inside the silica shell. The investigation of the luminescence properties also proved the presence of the complex inside the silica porosity. Indeed, the nanoparticles exhibit similar Tb3+ and Eu3+ characteristic excitations and emissions in comparison with the spectra of the free compound. However, some modifications in the emission spectra of nanoparticles compared to the ones of the non-loaded complexes indicate the occurrence of a slight geometry modification of the latter, which can be induced by interactions with acetylacetonate functions of the silica. In particular, the encapsulation boosted the Tb3+-to-Eu3+ energy transfer already occurring in the compound. This effect has already been observed in the case of acac-functionalized stellate silica nanoparticles hosting this luminescent complex and explained thanks to theoretical calculations. Indeed, the encapsulation induces a slight shortening of intermolecular Tb3+–Eu3+ distances, which results in a drastic increase in the pairwise energy transfer rate.
Thanks to the presence of the PB heater core, the obtained multifunctional nano-objects exhibit photothermal properties allowing an important temperature rise under irradiation at 808 nm within a few minutes (for instance ΔT is 20.4 °C within 3 min of irradiation with a laser power of 1.83 W cm−2). The observed difference in the macroscopic temperature change (ΔT) between the bare PB nanoparticles and the multifunctional PB@SiO2-acac/(Tb/Eu)9 nano-objects (0.92 ± 0.03 vs. 1.13 ± 0.03 J g−1 K−1) can be attributed to an increased specific heat capacity due to the presence of the silica shell, which retains the transferred heat from the PB core. A more complex model should be developed in order to investigate the thermal resistivity at the PB/silica interface.
The PB@SiO2-acac/(Tb/Eu)9 nano-objects also exhibit temperature-dependent luminescence of the Tb3+/Eu3+ complex offering the function of a luminescent thermometer in the visible region in the 20–80 °C range displaying a maximal relative thermal sensitivity of 0.75% °C−1 at 20 °C. This work opens up a route to new efficient heater/thermometer systems and investigations of the hot spot effect.
Author contributions
Conceptualization, S. S., G. F., J. L. and Y. G.; methodology, A. L., H. B., S. S., G. F., M. B., T. P., B. B., J. L., and Y. G.; validation, J. L., G. F., S. S.; formal analysis, A. L., H. B., M. B., S. S., Y. G., G. F.; investigation, A. L., H. B., G. F., S. S.; resources, S. S., J. L. and Y. G.; data curation, A. L., H. B., M. B., S. S., G. F., writing – original draft preparation, J. L., A. L., H. B., G. F.; writing – review and editing, S. S., G. F., J. L., and Y. G.; visualization, J. L., and Y. G.; supervision, S. S., G. F., J. L., and Y. G.; project administration, S. S., J. L. and Y. G.; funding acquisition, S. S. All authors have read and agreed to the published version of the manuscript.
Data availability
All data generated or analyzed during this study are included in the ESI† accompanying this article. Additional details and data that support the findings of this study are available from the corresponding author upon reasonable request.
Conflicts of interest
There are no conflicts to declare.
Acknowledgements
The authors thank the University of Montpellier and CNRS and the ANR within the frame of the HotSpot project (ANR-23-CE09-0017) for funding. H. B. is grateful to the LabUM program, which funded her Master 2 internship (ANR-16-IDEX-0006). The authors are grateful to the Platform of Analysis and Characterization of ICGM and platform Electronic and Analysis microscopy at the University of Montpellier for analysis and measurements.
Notes and references
- X. Cui, Q. Ruan, X. Zhuo, X. Xia, J. Hu, R. Fu, Y. Li, J. Wang and H. Xu, Photothermal Nanomaterials: A Powerful Light-to-Heat Converter, Chem. Rev., 2023, 123, 6891–6952 CrossRef CAS.
- P. Pallavicini, G. Chirico and A. Taglietti, Harvesting Light To Produce Heat: Photothermal Nanoparticles for Technological Applications and Biomedical Devices, Chem. – Eur. J., 2021, 27, 15361–15374 CrossRef CAS PubMed.
- Y. Liu, P. Bhattarai, Z. Dai and X. Chen, Photothermal therapy and photoacoustic imaging via nanotheranostics in fighting cancer, Chem. Soc. Rev., 2019, 48, 2053–2108 RSC.
- M. Kim, J.-H. Lee and J.-M. Nam, Plasmonic Photothermal Nanoparticles for Biomedical Applications, Adv. Sci., 2019, 6, 1900471 CrossRef.
- W. Bian, Y. Wang, Z. Pan, N. Chen, X. Li, W.-L. Wong, X. Liu, Y. He, K. Zhang and Y.-J. Lu, Review of Functionalized Nanomaterials for Photothermal Therapy of Cancers, ACS Appl. Nano Mater., 2021, 4, 11353–11385 CrossRef CAS.
- K. Yang, S. Zhao, B. Li, B. Wang, M. Lan and X. Song, Low temperature photothermal therapy: Advances and perspectives, Coord. Chem. Rev., 2022, 454, 214330 CrossRef CAS.
- J. Huo, Q. Jia, H. Huang, J. Zhang, P. Li, X. Dong and W. Huang, Emerging photothermal-derived multimodal synergistic therapy in combating bacterial infections, Chem. Soc. Rev., 2021, 50, 8762–8789 RSC.
- Y. Tao, H. F. Chan, B. Shi, M. Li and K. W. Leong, Light: A Magical Tool for Controlled Drug Delivery, Adv. Funct. Mater., 2020, 30, 2005029 CrossRef CAS.
- W. Zhao, Y. Zhao, Q. Wang, T. Liu, J. Sun and R. Zhang, Remote Light-Responsive Nanocarriers for Controlled Drug Delivery: Advances and Perspectives, Small, 2019, 15, 1903060 CrossRef CAS PubMed.
- D. Mateo, J. L. Cerrillo, S. Durini and J. Gascon, Fundamentals and applications of photo-thermal catalysis, Chem. Soc. Rev., 2021, 50, 2173–2210 RSC.
- J. Zhang, H. Chen, X. Duan, H. Sun and S. Wang, Photothermal catalysis: From fundamentals to practical applications, Mater. Today, 2023, 68, 234–253 CrossRef CAS.
- X. Song, X. Shan, H. Xue, X. Li, R. Liu, J. Kong, Z. Zuo, X. Su, Q. Zhang, Y. Yin and Y. Cai, Advances in Photothermal Catalysis: Mechanisms, Materials, and Environmental Applications, ACS Appl. Nano Mater., 2024 DOI:10.1021/acsanm.4c00598.
- F. Zhao, Y. Guo, X. Zhou, W. Shi and G. Yu, Materials for solar-powered water evaporation, Nat. Rev. Mater., 2020, 5, 388–401 CrossRef.
- M. Gao, L. Zhu, C. K. Peh and G. W. Ho, Solar absorber material and system designs for photothermal water vaporization towards clean water and energy production, Energy Environ. Sci., 2019, 12, 841–864 RSC.
- L. Zhu, M. Gao, C. K. N. Peh and G. W. Ho, Recent progress in solar-driven interfacial water evaporation: Advanced designs and applications, Nano Energy, 2019, 57, 507–518 CrossRef CAS.
- P. Cheng, D. Wang and P. Schaaf, A Review on Photothermal Conversion of Solar Energy with Nanomaterials and Nanostructures: From Fundamentals to Applications, Adv. Sustainable Syst., 2022, 6, 2200115 CrossRef CAS.
- Z. Chen, J. Li and Y. Zheng, Heat-Mediated Optical Manipulation, Chem. Rev., 2022, 122, 3122–3179 CrossRef CAS PubMed.
- L. Lin, E. H. Hill, X. Peng and Y. Zheng, Optothermal Manipulations of Colloidal Particles and Living Cells, Acc. Chem. Res., 2018, 51, 1465–1474 CrossRef CAS.
- Y. Zhao, H. Ji, M. Lu, J. Tao, Y. Ou, Y. Wang, Y. Chen, Y. Huang, J. Wang and Y. Mao, Thermochromic Smart Windows Assisted by Photothermal Nanomaterials, Nanomaterials, 2022, 12, 3865 CrossRef CAS PubMed.
- Z. Wang, M. Wang, X. Wang, Z. Hao, S. Han, T. Wang and H. Zhang, Photothermal-based nanomaterials and photothermal-sensing: An overview, Biosens. Bioelectron., 2023, 220, 114883 CrossRef CAS PubMed.
- J. Zheng, X. Cheng, H. Zhang, X. Bai, R. Ai, L. Shao and J. Wang, Gold Nanorods: The Most Versatile Plasmonic Nanoparticles, Chem. Rev., 2021, 121, 13342–13453 CrossRef CAS.
- M. Ha, J.-H. Kim, M. You, Q. Li, C. Fan and J.-M. Nam, Multicomponent Plasmonic Nanoparticles: From Heterostructured Nanoparticles to Colloidal Composite Nanostructures, Chem. Rev., 2019, 119, 12208–12278 CrossRef CAS.
- L. Jauffred, A. Samadi, H. Klingberg, P. M. Bendix and L. B. Oddershede, Plasmonic Heating of Nanostructures, Chem. Rev., 2019, 119, 8087–8130 CrossRef CAS.
- J. Chen, Z. Ye, F. Yang and Y. Yin, Plasmonic Nanostructures for Photothermal Conversion, Small Sci., 2021, 1, 2000055 CrossRef CAS.
-
H. Chi, Y. Wang, Z. Li and E. Ye, in Photothermal Nanomaterials, ed. E. Ye and Z. Li, The Royal Society of Chemistry, 2022, ch. 10, pp. 305–320 Search PubMed.
- Z. Li, H. Lei, A. Kan, H. Xie and W. Yu, Photothermal applications based on graphene and its derivatives: A state-of-the-art review, Energy, 2021, 216, 119262 CrossRef CAS.
- C. Coughlan, M. Ibáñez, O. Dobrozhan, A. Singh, A. Cabot and K. M. Ryan, Compound Copper Chalcogenide Nanocrystals, Chem. Rev., 2017, 117, 5865–6109 CrossRef CAS PubMed.
-
Z. Chu, B. Chen, W. Wang, H. Chen and H. Qian, in Photothermal Nanomaterials, ed. E. Ye and Z. Li, The Royal Society of Chemistry, 2022, ch. 8, pp. 252–285 Search PubMed.
- K. Li, Z. Zhang, D. Zhu and C. Yue, Excellent temperature sensitivities based on the FIR technique of up-conversion luminescence in a novel NaLaTi2O6:Yb3+,Tm3+ material, Inorg. Chem. Front., 2024, 11, 7464–7474 RSC.
- K. Li, D. Zhu and C. Yue, Codoping Pr3+ and Er3+ into NaLaTi2O6 to realize dual-mode FIR temperature sensing properties, Spectrochim. Acta, Part A, 2025, 326, 125224 CrossRef CAS.
- G. Cai, P. Yan, L. Zhang, H.-C. Zhou and H.-L. Jiang, Metal–Organic Framework-Based Hierarchically Porous Materials: Synthesis and Applications, Chem. Rev., 2021, 121, 12278–12326 CrossRef CAS PubMed.
- J.-D. Xiao and H.-L. Jiang, Metal–Organic Frameworks for Photocatalysis and Photothermal Catalysis, Acc. Chem. Res., 2019, 52, 356–366 CrossRef CAS PubMed.
- Q. Zheng, X. Liu, Y. Zheng, K. W. K. Yeung, Z. Cui, Y. Liang, Z. Li, S. Zhu, X. Wang and S. Wu, The recent progress on metal–organic frameworks for phototherapy, Chem. Soc. Rev., 2021, 50, 5086–5125 RSC.
- Q. Sun, K. Tang, L. Song, Y. Li, W. Pan, N. Li and B. Tang, Covalent organic framework based nanoagent for enhanced mild-temperature photothermal therapy, Biomater. Sci., 2021, 9, 7977–7983 RSC.
- R. Xia, X. Zheng, C. Li, X. Yuan, J. Wang, Z. Xie and X. Jing, Nanoscale Covalent Organic Frameworks with Donor–Acceptor Structure for Enhanced Photothermal Ablation of Tumors, ACS Nano, 2021, 15, 7638–7648 CrossRef CAS.
-
X. Zhang, Y. Xu, Z. Qiao and H. Wang, in Photothermal Nanomaterials, ed. E. Ye and Z. Li, The Royal Society of Chemistry, 2022, ch. 9, pp. 286–304 Search PubMed.
- A. V. de Walle, A. Figuerola, A. Espinosa, A. Abou-Hassan, M. Estrader and C. Wilhelm, Emergence of magnetic nanoparticles in photothermal and ferroptotic therapies, Mater. Horiz., 2023, 10, 4757–4775 RSC.
-
Y. Guari and J. Larionova, Prussian Blue-Type Nanoparticles and Nanocomposites: Synthesis, Devices, and Applications, Pan Stanford Publishing Pte Ltd, Singapore, 2019 Search PubMed.
- Z. Liu, H. Xie and T. Wang, Erythrocyte–Cancer Hybrid Membrane-Camouflaged Prussian Blue Nanoparticles with Enhanced Photothermal Therapy in Tumors, ACS Omega, 2023, 8, 23056–23066 CrossRef CAS.
- J. Liang, Y. Sun, K. Wang, Y. Zhang, L. Guo, Z. Bao, D. Wang, H. Xu, J. Zheng and Y. Yuan, Prussian Blue-Derived Nanoplatform for In Situ Amplified Photothermal/Chemodynamic/Starvation Therapy, ACS Appl. Mater. Interfaces, 2023, 15, 18191–18204 CrossRef CAS.
- K. Tang, X. Li, Y. Hu, X. Zhang, N. Lu, Q. Fang, J. Shao, S. Li, W. Xiu, Y. Song, D. Yang and J. Zhang, Recent advances in Prussian blue-based photothermal therapy in cancer treatment, Biomater. Sci., 2023, 11, 4411–4429 RSC.
- W. Zhu, K. Liu, X. Sun, X. Wang, Y. Li, L. Cheng and Z. Liu, Mn2+-Doped Prussian Blue Nanocubes for Bimodal Imaging and Photothermal Therapy with Enhanced Performance, ACS Appl. Mater. Interfaces, 2015, 7, 11575–11582 CrossRef CAS PubMed.
- L. M. A. Ali, E. Mathlouthi, M. Kajdan, M. Daurat, J. Long, R. Sidi-Boulenouar, M. Cardoso, C. Goze-Bac, N. Amdouni, Y. Guari, J. Larionova and M. Gary-Bobo, Multifunctional manganese-doped Prussian blue nanoparticles for two-photon photothermal therapy and magnetic resonance imaging, Photodiagn. Photodyn. Ther., 2018, 22, 65–69 CrossRef CAS.
- L. M. A. Ali, E. Mathlouthi, M. Cahu, S. Sene, M. Daurat, J. Long, Y. Guari, F. Salles, J. Chopineau, J.-M. Devoisselle, J. Larionova and M. Gary-Bobo, Synergic effect of doxorubicin release and two-photon irradiation of Mn2+-doped Prussian blue nanoparticles on cancer therapy, RSC Adv., 2020, 10, 2646–2649 RSC.
- P. Xu, W. Huang, J. Yang, X. Fu, W. Jing, Y. Zhou, Y. Cai and Z. Yang, Copper-rich multifunctional Prussian blue nanozymes for infected wound healing, Int. J. Biol. Macromol., 2023, 227, 1258–1270 CrossRef CAS.
- J. Wen, Z. Zhao, F. Fang, J. Xiao, L. Wang, J. Cheng, J. Wu and Y. Miao, Prussian Blue Nanoparticle-Entrapped GelMA Gels Laden with Mesenchymal Stem Cells as Prospective Biomaterials for Pelvic Floor Tissue Repair, Int. J. Mol. Sci., 2023, 24, 2704 CrossRef CAS PubMed.
- C. Qi, J. Chen, Y. Zhuang, Y. Zhang, Q. Zhang and J. Tu, PHMB
modified photothermally triggered nitric oxide release nanoplatform for precise synergistic therapy of wound bacterial infections, Int. J. Pharm., 2023, 640, 123014 CrossRef CAS.
- N. Chakraborty, D. Jha, V. P. Singh, P. Kumar, N. K. Verma, H. K. Gautam and I. Roy, White-Light-Responsive Prussian Blue Nanophotonic Particles for Effective Eradication of Bacteria and Improved Healing of Infected Cutaneous Wounds, ACS Appl. Mater. Interfaces, 2023, 15, 50765–50779 CrossRef CAS PubMed.
- X. He, H. Wu, Y. Wang, Y. Xiang, K. Zhang, X. Rao, E.-T. Kang and L. Xu, Bimodal Antimicrobial Surfaces of Phytic Acid–Prussian Blue Nanoparticles–Cationic Polymer Networks, Adv. Sci., 2023, 10, 2300354 CrossRef CAS PubMed.
- L. Doveri, A. Taglietti, P. Grisoli, P. Pallavicini and G. Dacarro, Dual mode antibacterial surfaces based on Prussian blue and silver nanoparticles, Dalton Trans., 2023, 52, 452–460 RSC.
- X. Song, Q. Ding, W. Wei, J. Zhang, R. Sun, L. Yin, S. Liu and Y. Pu, Peptide-Functionalized Prussian Blue Nanomaterial for Antioxidant Stress and NIR Photothermal Therapy against Alzheimer's Disease, Small, 2023, 19, 2206959 CrossRef CAS.
- W. Zhang, M. Sun, Y. Liu, Y. Zhang, L. Xu, Y. Luo, Q. Du, J. Xu, J. Liu, J. Zhou, H. Ran, Z. Wang, J. Wang and D. Guo, Platelet membrane-functionalized hollow mesoporous Prussian blue nanomedicine for comprehensive thrombolytic management by targeted enhanced fibrinolysis and ROS scavenging, Chem. Eng. J., 2023, 474, 145515 CrossRef CAS.
- J. Guan, M. Wang, Y. Xiong, Q. Liu and X. Chen, A luminescent MOF-based nonenzymatic probe for colorimetric/photothermal/fluorescence triple-mode assay of uric acid in body fluids, Talanta, 2024, 267, 125201 CrossRef CAS PubMed.
- H. Liang, S. Chen, A. Qileng, W. Liu, Z. Xu, S. Zhang and Y. Liu, Enhanced Photothermal Activity of Nanoconjugated System via Covalent Organic Frameworks as the Springboard, Small, 2024, 20, 2304720 CrossRef CAS PubMed.
- Y. Yang, Z. Xing, W. Kong, C. Wu, H. Peng, Y. Wang, Z. Li and W. Zhou, Hollow prussian blue analog@defect-rich layered double hydroxide S-scheme heterojunctions toward optimized photothermal-photocatalytic performance, Chem. Eng. J., 2023, 475, 146062 CrossRef CAS.
- W. Xu, H. Zhang, J. Ou, X. Fang, F. Wang, C. Li, W. Li, Y. Hu and A. Amirfazli, Improving durability and photothermal deicing with prussian blue in ptfe superhydrophobic coating, Mater. Lett., 2024, 355, 135579 CrossRef CAS.
- T. Pelluau, T. Brossier, M. Habib, S. Sene, G. Félix, J. Larionova, S. Blanquer and Y. Guari, 4D Printing Nanocomposite Hydrogel Based on PNIPAM and Prussian Blue Nanoparticles Using Stereolithography, Macromol. Mater. Eng., 2024, 309, 2300305 CrossRef CAS.
- S. Han, S. Chen, Z. Hu, Y. Liu, W. Zhang, B. Wang, J. Hu and L. Yang, A near-infrared light-promoted self-healing photothermally conductive polycarbonate elastomer based on Prussian blue and liquid metal for sensors, J. Colloid Interface Sci., 2024, 654, 955–966 CrossRef CAS PubMed.
- D. Gao, Y. Fan, B. Lyu, J. Ma and J. Zhang, Polyacrylate/Prussian blue nanoparticles composite emulsion applied to photothermal self-healing leather coatings, Prog. Org. Coat., 2023, 183, 107753 CrossRef CAS.
- H. Guan, R. Li, R. Lian, J. Cui, M. Ou, L. Liu, X. Chen, C. Jiao and S. Kuang, A biomimetic design for efficient petrochemical spill disposal: CoFe-PBA modified superhydrophobic melamine sponge with mechanical/chemical durability and low fire risk, J. Hazard. Mater., 2023, 459, 132041 CrossRef CAS.
- B. Liu, W. Wang, J. Fan, Y. Long, F. Xiao, M. Daniyal, C. Tong, Q. Xie, Y. Jian, B. Li, X. Ma and W. Wang, RBC membrane camouflaged prussian blue nanoparticles for gamabutolin loading and combined chemo/photothermal therapy of breast cancer, Biomaterials, 2019, 217, 119301 CrossRef CAS PubMed.
- W.-P. Li, C.-H. Su, L.-C. Tsao, C.-T. Chang, Y.-P. Hsu and C.-S. Yeh, Controllable CO Release Following Near-Infrared Light-Induced Cleavage of Iron Carbonyl Derivatized Prussian Blue Nanoparticles for CO-Assisted Synergistic Treatment, ACS Nano, 2016, 10, 11027–11036 CrossRef CAS PubMed.
- Y. Hao, L. Mao, R. Zhang, X. Liao, M. Yuan and W. Liao, Multifunctional Biodegradable Prussian Blue Analogue for Synergetic Photothermal/Photodynamic/Chemodynamic Therapy and Intrinsic Tumor Metastasis Inhibition, ACS Appl. Bio Mater., 2021, 4, 7081–7093 CrossRef CAS PubMed.
- M. Cahu, L. M. A. Ali, S. Sene, J. Long, F. Camerel, M. Ciancone, F. Salles, J. Chopineau, J.-M. Devoisselle, G. Felix, N. Cubedo, M. Rossel, Y. Guari, N. Bettache, J. Larionova and M. Gary-Bobo, A rational study of the influence of Mn2+-insertion in Prussian blue nanoparticles on their photothermal properties, J. Mater. Chem. B, 2021, 9, 9670–9683 RSC.
- C. D. S. Brites, R. Marin, M. Suta, A. N. Carneiro Neto, E. Ximendes, D. Jaque and L. D. Carlos, Spotlight on Luminescence Thermometry: Basics, Challenges, and Cutting-Edge Applications, Adv. Mater., 2023, 35, 2302749 CrossRef CAS PubMed.
- C. D. S. Brites, S. Balabhadra and L. D. Carlos, Lanthanide-Based Thermometers: At the Cutting-Edge of Luminescence Thermometry, Adv. Opt. Mater., 2019, 7, 1801239 CrossRef.
- D. Jaque and F. Vetrone, Luminescence nanothermometry, Nanoscale, 2012, 4, 4301–4326 RSC.
- S. Rohani, M. Quintanilla, S. Tuccio, F. De Angelis, E. Cantelar, A. O. Govorov, L. Razzari and F. Vetrone, Enhanced Luminescence, Collective Heating, and Nanothermometry in an Ensemble System Composed of Lanthanide-Doped Upconverting Nanoparticles and Gold Nanorods, Adv. Opt. Mater., 2015, 3, 1606–1613 CrossRef CAS.
- D. Jaque, B. D. Rosal, E. M. Rodríguez, L. M. Maestro, P. Haro-González and J. G. Solé, Fluorescent nanothermometers for intracellular thermal sensing, Nanomedicine, 2014, 9, 1047–1062 CrossRef CAS PubMed.
- C. D. S. Brites, M. C. Fuertes, P. C. Angelomé, E. D. Martínez, P. P. Lima, G. J. A. A. Soler-Illia and L. D. Carlos, Tethering Luminescent Thermometry and Plasmonics: Light Manipulation to Assess Real-Time Thermal Flow in Nanoarchitectures, Nano Lett., 2017, 17, 4746–4752 CrossRef CAS PubMed.
- T. Cesca, G. Perotto, G. Pellegrini, N. Michieli, B. Kalinic and G. Mattei, Rare-earth fluorescence thermometry of laser-induced plasmon heating in silver nanoparticles arrays, Sci. Rep., 2018, 8, 13811 CrossRef PubMed.
- S. Freddi, L. Sironi, R. D’Antuono, D. Morone, A. Donà, E. Cabrini, L. D’Alfonso, M. Collini, P. Pallavicini, G. Baldi, D. Maggioni and G. Chirico, A Molecular Thermometer for Nanoparticles for Optical Hyperthermia, Nano Lett., 2013, 13, 2004–2010 CrossRef CAS PubMed.
- K. Nigoghossian, S. Ouellet, J. Plain, Y. Messaddeq, D. Boudreau and S. J. L. Ribeiro, Upconversion nanoparticle-decorated gold nanoshells for near-infrared induced heating and thermometry, J. Mater. Chem. B, 2017, 5, 7109–7117 RSC.
- M. L. Debasu, C. D. S. Brites, S. Balabhadra, H. Oliveira, J. Rocha and L. D. Carlos, Nanoplatforms for Plasmon-Induced Heating and Thermometry, ChemNanoMat, 2016, 2, 520–527 CrossRef CAS.
- D. Mendez-Gonzalez, S. Melle, O. G. Calderón, M. Laurenti, E. Cabrera-Granado, A. Egatz-Gómez, E. López-Cabarcos, J. Rubio-Retama and E. Díaz, Control of upconversion luminescence by gold nanoparticle size: from quenching to enhancement, Nanoscale, 2019, 11, 13832–13844 RSC.
- J. He, W. Zheng, F. Ligmajer, C.-F. Chan, Z. Bao, K.-L. Wong, X. Chen, J. Hao, J. Dai, S.-F. Yu and D. Y. Lei, Plasmonic enhancement and polarization dependence of nonlinear upconversion emissions from single gold nanorod@SiO2@CaF2:Yb3+,Er3+ hybrid core–shell–satellite nanostructures, Light: Sci. Appl., 2017, 6, e16217 CrossRef CAS PubMed.
- M. L. Debasu, D. Ananias, I. Pastoriza-Santos, L. M. Liz-Marzán, J. Rocha and L. D. Carlos, All-In-One Optical Heater-Thermometer Nanoplatform Operative From 300 to 2000 K Based on Er3+ Emission and Blackbody Radiation, Adv. Mater., 2013, 25, 4868–4874 CrossRef CAS PubMed.
- M. Quintanilla, I. García, I. de Lázaro, R. García-Alvarez, M. Henriksen-Lacey, S. Vranic, K. Kostarelos and L. M. Liz-Marzán, Thermal monitoring during photothermia: hybrid probes for simultaneous plasmonic heating and near-infrared optical nanothermometry, Theranostics, 2019, 9, 7298–7312 CrossRef CAS PubMed.
- Y. Shen, H. D. A. Santos, E. C. Ximendes, J. Lifante, A. Sanz-Portilla, L. Monge, N. Fernández, I. Chaves-Coira, C. Jacinto, C. D. S. Brites, L. D. Carlos, A. Benayas, M. C. Iglesias-de la Cruz and D. Jaque, Ag2S Nanoheaters with Multiparameter Sensing for Reliable Thermal Feedback during In Vivo Tumor Therapy, Adv. Funct. Mater., 2020, 30, 2002730 CrossRef CAS.
- M. Wang, A. Skripka, Y. Zhang, T. Cheng, M. Ng, S. Y. Wong, Y. Zhao, X. Sun, X. Li, K. K. Bhakoo, A. Y. Chang, F. Rosei and F. Vetrone, Theranostic Nanocapsules: Heating, Imaging, and Luminescence Nanothermometry, Chem. Mater., 2024, 36, 3285–3295 CrossRef CAS.
- X. Zhu, W. Feng, J. Chang, Y.-W. Tan, J. Li, M. Chen, Y. Sun and F. Li, Temperature-feedback upconversion nanocomposite for accurate photothermal therapy at facile temperature, Nat. Commun., 2016, 7, 10437 CrossRef CAS PubMed.
- H. Suo, X. Zhao, Z. Zhang and C. Guo, 808 nm Light-Triggered Thermometer–Heater Upconverting Platform Based on Nd3+-Sensitized Yolk–Shell GdOF@SiO2, ACS Appl. Mater. Interfaces, 2017, 9, 43438–43448 CrossRef CAS PubMed.
- X. Wang, H. Li, F. Li, X. Han and G. Chen, Prussian blue-coated lanthanide-doped core/shell/shell nanocrystals for NIR-II image-guided photothermal therapy, Nanoscale, 2019, 11, 22079–22088 RSC.
- M. Shokouhimehr, E. S. Soehnlen, A. Khitrin, S. Basu and S. D. Huang, Biocompatible Prussian blue nanoparticles: Preparation, stability, cytotoxicity, and potential use as an MRI contrast agent, Inorg. Chem. Commun., 2010, 13, 58–61 CrossRef CAS.
- M. Shokouhimehr, E. S. Soehnlen, J. Hao, M. Griswold, C. Flask, X. Fan, J. P. Basilion, S. Basu and S. D. Huang, Dual purpose Prussian blue nanoparticles for cellular imaging and drug delivery: a new generation of T1-weighted MRI contrast and small molecule delivery agents, J. Mater. Chem., 2010, 20, 5251–5259 RSC.
- M. F. Dumont, S. Yadavilli, R. W. Sze, J. Nazarian and R. Fernandes, Manganese-containing Prussian blue nanoparticles for imaging of pediatric brain tumors, Int. J. Nanomed., 2014, 9, 2581–2595 CAS.
- A. Sahu, J. H. Lee, H. G. Lee, Y. Y. Jeong and G. Tae, Prussian blue/serum albumin/indocyanine green as a multifunctional nanotheranostic agent for bimodal imaging guided laser mediated combinatorial phototherapy, J. Controlled Release, 2016, 236, 90–99 CrossRef CAS PubMed.
- E. Mamontova, F. Salles, Y. Guari, J. Larionova and J. Long, Post-synthetic modification of Prussian blue type nanoparticles: tailoring the chemical and physical properties, Inorg. Chem. Front., 2022, 9, 3943–3971 RSC.
- E. Mamontova, J. Long, R. A. S. Ferreira, A. M. P. Botas, F. Salles, Y. Guari, L. D. Carlos and J. Larionova, Making Prussian blue analogues nanoparticles luminescent: effect of the luminophore confinement over the properties, Nanoscale, 2019, 11, 7097–7101 RSC.
- S. Petit, F. Baril-Robert, G. Pilet, C. Reber and D. Luneau, Luminescence spectroscopy of europium(III) and terbium(III) penta-, octa- and nonanuclear clusters with β-diketonate ligands, Dalton Trans., 2009, 6809–6815 RSC.
- D. Guettas, C. M. Balogh, C. Sonneville, Y. Malicet, F. Lepoivre, E. Onal, A. Fateeva, C. Reber, D. Luneau, O. Maury and G. Pilet, Nano-Nonanuclear Mixed-Lanthanide Clusters [Eu9−xTbx] Featuring Tunable Dual Luminescence Properties, Eur. J. Inorg. Chem., 2016, 3932–3938 CrossRef CAS.
- D. A. Gálico, C. M. S. Calado and M. Murugesu, Lanthanide molecular cluster-aggregates as the next generation of optical materials, Chem. Sci., 2023, 14, 5827–5841 RSC.
- T. Pelluau, S. Sene, L. M. A. Ali, G. Félix, A. N. C. Neto, L. D. Carlos, B. Albela, L. Bonneviot, E. Oliviero, M. Gary-Bobo, Y. Guari and J. Larionova, Hybrid multifunctionalized mesostructured stellate silica nanoparticles loaded with beta-diketonate Tb3+/Eu3+ complexes as stable ratiometric emissive thermometer working in water, Nanoscale, 2023, 15, 14409 RSC.
- B. Yuan, X. He, Y. Chen and K. Wang, Preparation of Nanosilica/Polynorbornene Nanocomposite by Covalently Immobilized Silica-Supported Acetylacetonate Palladium(II) Dichloride Catalyst, Macromol. Chem. Phys., 2011, 212, 2378–2388 CrossRef CAS.
- T. Pelluau, S. Sene, B. Garcia-Cirera, B. Albela, L. Bonneviot, J. Larionova and Y. Guari, Multifunctionalized Mesostructured Silica Nanoparticles Containing Mn2 Complex for Improved Catalase-Mimicking Activity in Water, Nanomaterials, 2022, 12, 1136 CrossRef CAS PubMed.
- G. Maurin-Pasturel, E. Rascol, M. Busson, S. Sevestre, J. Lai-Kee-Him, P. Bron, J. Long, J. Chopineau, J.-M. Devoisselle, Y. Guari and J. Larionova, 201Tl-labeled Prussian blue and Au@Prussian blue nanoprobes for SPEC-CT imaging: influence of the size, shape and coating on the biodistribution, Inorg. Chem. Front., 2017, 4, 1737–1741 RSC.
- E. Mamontova, M. Daurat, J. Long, A. Godefroy, F. Salles, Y. Guari, M. Gary-Bobo and J. Larionova, Fashioning Prussian Blue Nanoparticles by Adsorption of Luminophores: Synthesis, Properties, and in Vitro Imaging, Inorg. Chem., 2020, 59, 4567–4575 CrossRef CAS PubMed.
- J.-J. Hu, Y. Chen, Z.-H. Li, S.-Y. Peng, Y. Sun and X.-Z. Zhang, Augment of Oxidative Damage with Enhanced Photodynamic Process and MTH1 Inhibition for Tumor Therapy, Nano Lett., 2019, 19, 5568–5576 CrossRef CAS PubMed.
-
J. Rodriguez-Carvajal, Fullprof: A Program for Rietveld Refinement and Pattern Matching Analysis, Satellite Meeting on Powder Diffraction of the XV Congress of the IUCr, 1990, 127.
- COMSOL Multiphysics® v. 6.0. https://www.comsol.com. COMSOL AB, Stockholm, Sweden.
- X. Du and S. Z. Qiao, Dendritic Silica Particles with Center-Radial Pore Channels: Promising Platforms for Catalysis and Biomedical Applications, Small, 2015, 11, 392–413 CrossRef CAS PubMed.
- V. Polshettiwar, D. Cha, X. Zhang and J. M. Basset, High-Surface-Area Silica Nanospheres (KCC-1) with a Fibrous Morphology, Angew. Chem., Int. Ed., 2010, 49, 9652–9656 CrossRef CAS PubMed.
- K. Zhang, L.-L. Xu, J.-G. Jiang, N. Calin, K.-F. Lam, S.-J. Zhang, H.-H. Wu, G.-D. Wu, B. Albela, L. Bonneviot and P. Wu, Facile Large-Scale Synthesis of Monodisperse Mesoporous Silica Nanospheres with Tunable Pore Structure, J. Am. Chem. Soc., 2013, 135, 2427–2430 CrossRef CAS PubMed.
- Y. Y. Su, Z. Teng, H. Yao, S. J. Wang, Y. Tian, Y. L. Zhang, W. F. Liu, W. Tian, L. J. Zheng, N. Lu, Q. Q. Ni, X. D. Su, Y. X. Tang, J. Sun, Y. Liu, J. Wu, G. F. Yang, G. M. Lu and L. J. Zhang, A Multifunctional PB@mSiO2–PEG/DOX Nanoplatform for Combined Photothermal–Chemotherapy of Tumor, ACS Appl. Mater. Interfaces, 2016, 8, 17038–17046 CrossRef CAS PubMed.
- W. Tian, Y. Su, Y. Tian, S. Wang, X. Su, Y. Liu, Y. Zhang, Y. Tang, Q. Ni, W. Liu, M. Dang, C. Wang, J. Zhang, Z. Teng and G. Lu, Periodic Mesoporous Organosilica Coated Prussian Blue for MR/PA Dual-Modal Imaging-Guided Photothermal-Chemotherapy of Triple Negative Breast Cancer, Adv. Sci., 2017, 4, 1600356 CrossRef PubMed.
- G. Ngo, G. Félix, J. Long, L. Costa, O. Saavedra, P.-E. Milhiet, J.-M. Devoisselle, Y. Guari, J. Larionova and J. Chopineau, A simple approach for controlled deposition of Prussian blue analogue nanoparticles on a functionalised plasmonic gold surface, New J. Chem., 2019, 43, 3660–3664 RSC.
- G. Ngo, G. Félix, C. Dorandeu, J.-M. Devoisselle, L. Costa, P.-E. Milhiet, Y. Guari, J. Larionova and J. Chopineau, A Novel Approach to the Facile Growth and Organization of Photothermal Prussian Blue Nanocrystals on Different Surfaces, Nanomaterials, 2021, 11, 1749 CrossRef CAS PubMed.
- A. Alkurdi, J. Lombard, F. Detcheverry and S. Merabia, Enhanced Heat Transfer with Metal-Dielectric Core-Shell Nanoparticles, Phys. Rev. Appl., 2020, 13, 034036 CrossRef CAS.
- G. Ngo, G. Félix, C. Dorandeu, J.-M. Devoisselle, L. Costa, P.-E. Milhiet, Y. Guari, J. Larionova and J. Chopineau, A Novel Approach to the Facile Growth and Organization of Photothermal Prussian Blue Nanocrystals on Different Surfaces, Nanomaterials, 2021, 11, 1749 CrossRef CAS PubMed.
- J. Zeng, D. Goldfeld and Y. Xia, A Plasmon-Assisted Optofluidic (PAOF) System for Measuring the Photothermal Conversion Efficiencies of Gold Nanostructures and Controlling an Electrical Switch, Angew. Chem., Int. Ed., 2013, 52, 4169–4173 CrossRef CAS PubMed.
- C. D. S. Brites, P. P. Lima, N. J. O. Silva, A. Millán, V. S. Amaral, F. Palacio and L. D. Carlos, A luminescent molecular thermometer for long-term absolute temperature measurements at the nanoscale, Adv. Mater., 2010, 22, 4499–4504 CrossRef CAS PubMed.
- A. Bednarkiewicz, L. Marciniak, L. D. Carlos and D. Jaque, Standardizing luminescence nanothermometry for biomedical applications, Nanoscale, 2020, 12, 14405–14421 RSC.
|
This journal is © the Partner Organisations 2025 |
Click here to see how this site uses Cookies. View our privacy policy here.