DOI:
10.1039/D4RA06909A
(Paper)
RSC Adv., 2025,
15, 2562-2570
Surface treatment of ZnO:Sb microwires to improve their persistent photo-conductance response†
Received
25th September 2024
, Accepted 7th January 2025
First published on 27th January 2025
Abstract
Chemical and biological sensors based on ZnO microwires usually rely on the change in the wire conductance with the ambient gas composition. Yet, sensitivity and recovery time of the conductance are important limitations in these applications. We treated ZnO:Sb micro-wires with single droplets of solvents for very short times and found a significant enhancement of the persistent photo-conductance and a reduction of the recovery time of the resistance by more than an order of magnitude when treated with isopropanol droplets. Placing a solvent droplet on a ZnO:Sb micro-wire in situ during measurement allowed us to make a direct comparison between the behavior before, during, and after solvent treatment. Isopropyl alcohol (IPA) has the most significant response among the solvents that we studied. Two possible mechanisms have been proposed as possibly being responsible for this huge enhancement of the wire resistance: surface transformations of isopropanol and the catalyzing effect of Sb atoms. Both mechanisms seem to combine and enhance the oxygen adsorption to the wire surface causing a very large rise in the resistance.
1. Introduction
Micro- and nano-sensors play a vital role in modern health and technological applications. ZnO is a thoroughly studied wide-band-gap semiconductor1,2 that has also been proposed and investigated as a chemical sensor. It is relatively inexpensive and easy to grow in multiple useful geometries, like thin films3 nano-wires4 and rods.5 While Sb-doped ZnO (or ZnO:Sb) is predicted to be a p-type semiconductor, most grown ZnO:Sb structures actually turn out to be n-type.6 This is due to oxygen vacancies and zinc interstitials that are not stable at room temperature.1 These imperfections more than cancel out the holes produced by the Sb-doping and give an overall n-type behavior.1
Our thermally-grown ZnO:Sb micro-wires previously showed clear n-type behavior.7 Moreover, these wires have a core–shell structure with a mostly insulating ZnO core and an Sb-rich ZnO:Sb shell,2 where conduction seems to only take place at or near the outer-most layer at the surface of the micro-wire.2,8 This surface conduction is affected by adsorbed atoms, so it makes the resistance (conductance) of the wires quite sensitive to the surrounding chemical environment in addition to its sensitivity to temperature and light.2 The rise in resistance after light is removed is much slower than the more familiar behavior due to the excitation of electrons to the conduction band of a semiconductor.9 This slow but significant change in the resistance of ZnO after exposure to light is therefore referred to as ‘persistent photo-conductance’.2 This ‘surface-originating’ photo-conductance is most likely caused by oxygen vacancies, which are in turn caused by the desorption of oxygen atoms at the surface.10 Surface oxygen atoms are loosely bound to the ZnO wire surface (with energy about 60 meV (ref. 11)) and can get desorbed due to an increase in temperature, exposure to light, or a reduction in the concentration of ambient oxygen.2 The persistent photoconductance is especially pronounced in micro- and nano-wires due to their very large surface area.12
ZnO sensitivity to light and chemical ambient made it a natural candidate to be used as a sensor. So, it has been extensively studied as a potential sensor of various solvents, ultraviolet (UV) light,13 as well as oxidizing and reducing gases. While selectivity is still an unresolved problem, the photoconductivity of ZnO wires displays significant changes upon exposure to small traces of gases of such materials. More specifically, oxidizers cause a rise in the resistance of ZnO wires, while reducers cause a drop in the resistance since they free some conduction electrons. Likewise, solvent gases like acetone,14 isopropyl alcohol,15 benzene and others16 also affect the resistance of ZnO wires by freeing electrons at the surface. The resistance usually goes back slowly to its stable value after the sensed gas is removed.2
The main hypothesis in this paper is that solvents can leave lasting effects on the surface of ZnO wires and such effects might be utilized in increasing the sensitivity, efficiency, and time response of ZnO:Sb wires to chemical, temperature, or light exposure variations. This prediction is plausible because solvent gases have strong effects on the resistance of ZnO microwires. So, we will immerse ZnO:Sb microwires in (or treat the wires with) various liquids for short periods of time and then study the effects on the photo-conductance properties. We will also compare and contrast the effects of treatment with an alkene (olefin) versus an alkane solvent on the persistent photo-conductance. This treatment is especially useful in determining the route for the surface transformations responsible for the photo-conductance enhancement and the role of hydrogen atoms and water molecules on the photo-conductance. This problem received little attention from researchers and still needs elaborate studies due to its significant implications for enhancing the performance of these wires as sensors.17,18
2. Experimental setup
ZnO:Sb microwires are thermally grown by placing a 3
:
2 mixture of 3 g of Zn and 2 g of Sb2O3 powder in a crucible, mixing them thoroughly, and then heating in an oven to 900 °C for 2 hours. The oven is then turned off and the crucible and contents are allowed to cool down to room temperature overnight. This produces a huge number of ZnO:Sb core–shell micro-wires with ZnO cores and Sb-rich shells. An individual wire is then attached to a simple voltage divider circuit via conductive epoxy and a battery is connected in series to a known resistor and the ZnO:Sb wire, as described in detail elsewhere.2 A data acquisition (NI MyDAQ) device is then used to measure the voltage difference across the ZnO:Sb wire under different conditions. The electrical resistance of the microwires is then recorded and studied under various light and solvent exposure conditions, where a solvent drop is placed directly on the wire and allowed to dry/evaporate naturally during the measurements.
3. Data and analysis
We start with imaging and structural analysis, where it is seen from the scanning electron microscope (SEM) image in Fig. 1A that the ZnO:Sb microwires are polycrystalline, have a rough surface and vary in diameter slightly from place to place. This particular wire has a diameter of about 8 μm and is approximately 2 mm long, as also seen from Fig. 1B, which is an optical microscope image taken via the CCD camera (ZWO ASI1600). The images also show the two conductive epoxy (H20E EPO-TEK) drops used to connect the ZnO:Sb microwire to the measurement circuit.
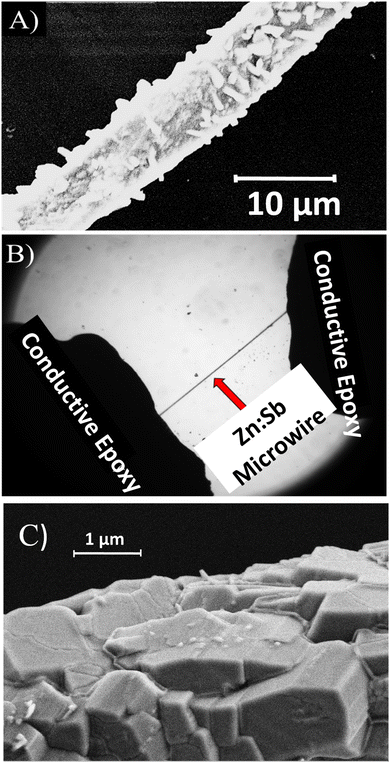 |
| Fig. 1 (A) SEM image for ZnO:Sb micro-wire. The surface of the wire is not smooth and has many nano-crystals on it (B) optical microscope image of a ZnO:Sb micro-wire that has been connected to the measurement circuit using two conductive epoxy drops. (C) High-resolution SEM image showing the rough, polycrystalline nature of the ZnO:Sb wires surface. | |
As evidenced by the SEM images in Fig. 1A and C, as well as many other images,2 X-ray diffraction (XRD), X-ray photoelectron spectroscopy (XPS), electron backscattered diffraction (EBSD), and photoluminescence (PL) spectra (that we published elsewhere) are all consistent with a polycrystalline, core–shell structure of the ZnO:Sb wires with a pure ZnO core and a Sb-rich ZnO:Sb shell having a rough surface.6,7,19 While the core of the wire is basically free of Sb atoms, the shell is quite rich in Sb with an atomic abundance of about 15%.
3.1 Persistent photo-conductance effects due to light exposure
We study next the persistent photo-conductance of the wires: in Fig. 2A, we illuminated a white light on a ZnO:Sb microwire for 5 minutes causing its resistance to decrease from about 35 MΩ to 8 MΩ. This decrease in resistance to less than 25% of its original value is most likely due to the desorption of oxygen atoms from the wire surface. The energy of the absorbed light frees some oxygen atoms leaving behind more and more free electrons, thus causing a significant rise (drop) in this surface-dominated photo-conductance (resistance).2
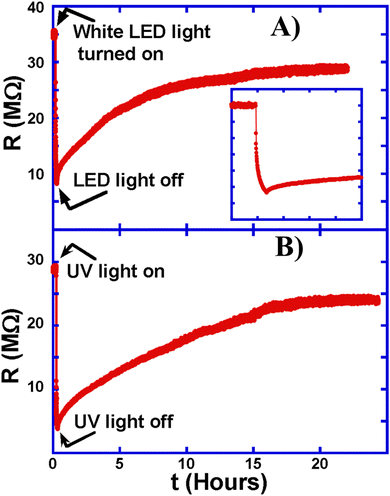 |
| Fig. 2 (A) White LED light causes the microwires resistance to decrease relatively quickly. On the other hand, turning the light off leads to a rather slow increase in resistance. The inset shows the same data, has the same axes labels and units, but shows the change in resistance during the first hour of data collection. (B) Illuminating UV light on the ZnO:Sb microwire causes the resistance to drop rapidly, while the resistance rises quite slowly when turning the UV light off, just like the effect of white light exposure. | |
The resistance of the ZnO:Sb microwire starts to rise back immediately after the light is turned off, starting with a relatively fast increase during the first few minutes, but increasing much slower after that, where it took more than 24 hours to return to the original resistance from which it started. This is called persistent photoconductance.2 As oxygen atoms from the ambient get adsorbed back onto the wire surface, they ‘fill’ oxygen vacancies thus eliminating some free electrons ‘donated’ by these vacancies. This diffusive process takes a relatively long time and depends on the abundance of oxygen in the surroundings. A concise representation of this behavior is as follows:12,20
|
O2(gas) ↔ O2(adsorbed)
| (1) |
|
O2(adsorbed) + e− ↔ O2(adsorbed)− ⇒ surface resistance increases
| (2) |
So, when there is no light at room temperature, oxygen molecules get adsorbed on the surface of the wires and capture some of the free electrons from the surface causing the resistance to increase. On the other hand, exposure to light (or increasing the temperature) provides the activation energy needed for the O2 molecules to get desorbed and released back as gas, thus freeing an electron for each desorbed molecule. This rise in free electrons density causes the resistance to decrease significantly, see eqn (3).
|
O2(adsorbed)− + hν(light) → O2(gas) + e− ⇒ surface resistance decreases
| (3) |
Fig. 2B shows the effect of exposing a ZnO:Sb micro-wire to ultraviolet (UV) light for 5 minutes. The resistance of the wire decreased from about 28 MΩ to below 4 MΩ, which is less than 15% of the original value. Even though the intensity of the UV light is about 1000 times less than that of the white light, as shown in Fig. 3, the resistance drop due to UV light exposure is quite similar to that caused by the white light. The higher energy of the UV light gets absorbed more and releases adsorbed oxygen from the ZnO:Sb wire more efficiently, leading to a much higher sensitivity to UV light. This extreme sensitivity to UV light makes ZnO:Sb wires excellent UV sensors. It is important, however, to notice that the resistance takes the same long time to rise back to its original value, both after exposure to white and to UV lights. This clearly shows that this change in ZnO:Sb wire resistance is due to the persistent photo-conductance through the surface-oxygen desorption,21 rather than due to exciting ZnO valence electrons to the conduction band and increasing the conductance through the well-known semiconductor photoconductance mechanism. This happens even though the energy of the UV light (3.45 eV) is larger than the energy gap of 3.37 eV in ZnO, so these ‘messy’ polycrystalline wires clearly do not behave like the single crystal ZnO semiconductor.
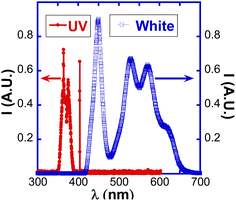 |
| Fig. 3 The spectrum of both the white light LED (open blue squares) and the UV light (filled red circles) used to illuminate the ZnO:Sb wires in this study. It is important to know that the intensity of the UV light is 1000 times less than that of the white LED, so the y-axis to the left (the intensity of the UV light) has been multiplied by 1000 for easier display of the spectrum. | |
The recovery of the resistance of the ZnO:Sb microwires happens in two stages: the first stage is relatively fast, and is due to the heterojunction regarding electron–hole recombination leads, while the second stage happens due to the adsorption of oxygen molecules at the surface.22 For example, photoconductance studies on ZnO wires in vacuum showed the rise in resistance to be much slower than when in air because of the reduced concentration of oxygen atoms that can adsorb to the surface and cause the resistance to increase.23
3.2 Surface treatment effects
Oxygen adsorption and desorption also play a crucial role in changing the wire resistance when the surroundings of the ZnO:Sb wires change: exposure to reducing gases causes the resistance to decrease, while oxidizers cause it to increase.24,25 This sensitivity of their electric resistance to oxygen concentration makes the ZnO wires potential oxygen sensors.2 Solvents also have a strong effect on ZnO persistent photoconductance, just like oxidizers and reducers in general. For example, acetone acts as a reducing gas where it affects the free carrier density (and thus the resistance) at room temperature through reacting with the adsorbed oxygen ions forming according to eqn (2) above. More specifically, each acetone molecule reacts with FOUR O2− giving three molecules each of water and carbon dioxide and releasing four free electrons as conduction electrons on the wire surface according to the reaction:20 |
CH3COCH3 + 4O2(adsorbed)− → 3CO2 + 3H2O + 4e−
| (4) |
Thus, each acetone molecule frees 4 electrons and strongly decreases the surface resistance. Other solvents have a similar effect, so their presence can be ‘sensed’ by measuring the resistance changes in ZnO. In general, reducers free electrons and increase surface conductivity, while oxidizers capture free electrons thus they reduce conductivity.
One problem with such sensors, however, is that the resistance takes a very long time to go back to its original value after it drops due to any stimulant, making it desirable to speed up resistance-recovery process. Surface treatment is a promising tool to speed up the resistance recovery because it can affect the oxygen adsorption/desorption chemistry. While acetone and other solvents have a clear effect on the abundance of free electrons on the surface, as shown in eqn (4) above, the main question that this article addresses is: Do solvents leave some lasting effects on the surfaces of ZnO:Sb wires?
3.2.1 Isopropyl alcohol treatment. We investigate first the isopropyl alcohol (IPA)-treatment effects and start by connecting a fresh ZnO:Sb wire, left it in darkness for two days, and then recorded its UV photo-conductance response, shown in Fig. 4A. Even though the initial resistance (R0) for this particular wire is about two times higher than the resistance of the wire that produced Fig. 2A, yet the overall behavior is the same. The sample was then left in darkness for about two days in order for its resistance to go back to its initial large value. The inset in Fig. 4A shows the same resistance graph as Fig. 4A but for a longer time of two days while in darkness. We then started collecting the resistance data shown in Fig. 4B. The blue line with filled circles shows the typical drop in resistance associated with turning on the UV light after about 5 minutes from the beginning. UV light is then turned off after about 1 minute of exposure and a droplet of IPA with volume ≈90 μL is placed directly on the ZnO:Sb wire. The IPA causes the resistance to drop to below 1 MΩ, most likely due to conduction through the liquid itself rather than through the wire.
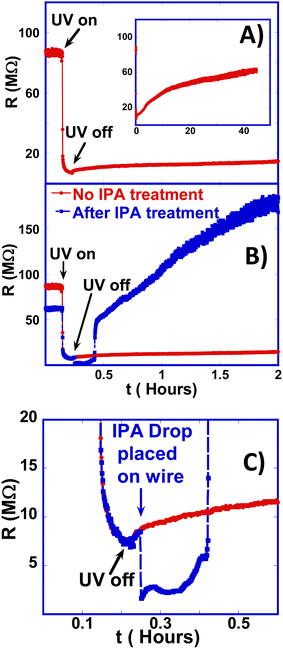 |
| Fig. 4 Persistent photo-resistance behavior of a ZnO:Sb wire when (A) not treated with any solvents, where the inset is a replot of the same data for a longer period of two full days without light exposure. (B) When treated by immersion in an IPA drop for few minutes until the drop dries. The effect of IPA is quite apparent, where the resistance returns to its initial value within minutes rather than days. The resistance even increases to more than 200% of its original value when treated with IPA. (C) Is a zoomed-in plot of the resistance as the IPA droplet is placed on the wire and as it evaporates. | |
The most important behavior is that as the IPA evaporates and eventually dries at around 0.4 hours (25 minutes), the resistance rises abruptly and becomes larger than its initial value within 10 minutes of IPA drying. The resistance then continues to rise to more than twice its initial value within 1 hour, which is in sharp contrast with the extremely slow resistance rise in untreated ZnO:Sb wires. We observed a rise in the resistance to more than 200% ± 20% of its untreated value in four different samples that we treated with IPA. For direct comparison between untreated and IPA-treated wires, the red line with filled circles in Fig. 3B is a replot of Fig. 3A showing the persistent photo-resistance behavior in the un-treated wire, while again the blue line with solid squares is for the IPA-treated wire. Comparison shows that the two most important features of the IPA-treated wire are: (1) the very quick ‘recovery’ of the wire resistance after exposure to UV light. (2) The huge enhancement of the resistance of the wire. This faster and larger response can have significant implications for improving the photo-conductance response of these wires and utilizing them in real applications as gas sensors.
This effect on the resistance following the complete evaporation of IPA is also quite different from that reported in the literature when exposing ZnO to moderate concentrations of IPA gas: in these reports, the presence of IPA causes the resistance to decrease, while turning the IPA off leads to a rise in resistance.26 The reported behavior in the literature is consistent with the reaction in eqn (4) above because it leads to the ‘liberation’ of larger numbers of free electrons. Yet, our results suggest a completely different effect or mechanism that likely causes lasting (long-term) changes to the surface of the wire. Fig. 4C is a zoomed-in version of Fig. 4B that focuses on the behavior of the resistance as the IPA drop is placed on the ZnO:Sb wire and as it dries out. It shows the huge and abrupt effect of IPA treatment on increasing the wire resistance. We observed this effect of short IPA treatment consistently on multiple other samples, but only show one data set here.
3.2.2 Surface treatment in other solvents. An important question is whether other common solvents have the same effects on the ZnO:Sb wires or not. Fig. 5 shows a comparison of the effects of four different solvents, as well as the case without any solvent treatment. It is quite apparent that only IPA treatment has a strong effect on the wire conduction properties. The mechanism leading to the enhancement of photo-conductance effects seems to be specific to isopropanol, among all four tested solvents.
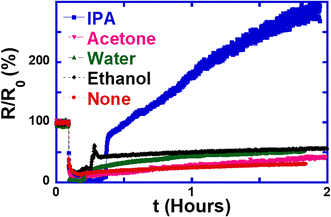 |
| Fig. 5 Comparison of the persistent photoconductance response in ZnO:Sb micro-wires when either untreated or treated by immersion in alcohol, water, acetone, or ethanol for a short time (about 3 minutes). The only treatment that gives a strong effect on the conduction properties of the wire is IPA. | |
We further tested the effect of treating the wire with either an alkane (cyclohexane) or an alkene (cyclohexene) in order to determine the chemical mechanism through which IPA treatment works. Fig. 6 shows that using the non-polar solvent cyclohexane does not have any appreciable effect on the resistance either. This shows that the polarity of the solvent has no significant effect on the gas adsorption at the surface of the ZnO:Sb wires.
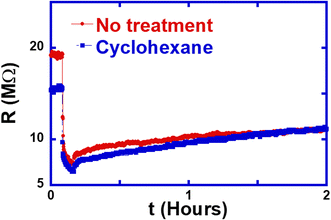 |
| Fig. 6 Cyclohexane treatment effects. Using this nonpolar solvent does not give any appreciable effects on the photoconductance behavior of the ZnO:Sb micro-wires. | |
3.2.3 Solvent transformation to explain solvent-treatment effects. A possible mechanism to explain this long-term effect (i.e. the significant, relatively fast rise in resistance) due to IPA treatment of the ZnO:Sb micro-wires is an increase in the surface roughness of the wires due to immersion in IPA. This added roughness can lead to a larger surface area and allow the adsorption of more oxygen molecules on the surface. While this mechanism was proposed in the literature to explain16,17 an enhancement in the photodetection properties of ZnO nano-wires, the nano-wires used in ref. 17 were immersed in IPA for weeks to achieve sufficient surface roughness. This is much longer than the IPA-treatment time of our wires, which were covered in IPA for two to three minutes only. So, increased surface roughness is unlikely to cause the effects we see in Fig. 4B.The most likely mechanism is the transformations of the solvent (i.e. IPA) on the ZnO:Sb micro-wire surface,27 where IPA goes through a series of reactions producing chemicals such as enolates and allylic species28 that stay on the wire surface after the IPA evaporates. These intermediates react strongly with oxygen thus accelerating (i.e. catalyzing) the incorporation of oxygen on the micro-wire surface and increasing the resistance rapidly. This process becomes especially faster or more efficient due to the presence of Sb on the ZnO wire.
More specifically, IPA adsorbs dissociatively on Zn and Sb (from ZnO:Sb) sites to form surface isopropoxide ((CH3)2HCO) species,28 which is an alkoxide. These alkoxide intermediate compounds go either through dehydration reactions that produce propylene by removing an H2O molecule29 or dehydrogenation reactions that produce acetone when removing an H2 molecule. The actual process that will be favored (i.e. dehydration or dehydrogenation) depends on the nature of the active surface sites: redox or acidic–basic. Redox surface sites yield acetone and acidic–basic surface sites yield propylene.30 A schematic representation/chart of the two possible reaction paths is:
|
 | (5) |
In the dehydrogenation path, an enolate anion is formed by the abstraction of a proton from one of the methyl groups of acetone. This enolate intermediate oxidizes by an ambient oxygen molecule on the ZnO:Sb surface, thus speeding the process of oxygen adsorption to the surface.29,31
The dehydrogenation (acetone-producing) path produces a stable carbonyl compound at room temperature according to the equation:
|
 | (6) |
It is important to notice that acetone treatment did not lead to any significant enhancement in the resistance, as shown in Fig. 4 above. Therefore, the acetone path of the reaction (i.e. the dehydrogenation shown in eqn (5) and (6)) is quite unlikely to be the responsible mechanism for the observed resistance enhancement. This leaves us with one likely reaction path, which is the propylene-producing dehydration path outlined in eqn (7) below.
As eqn (7) below shows, the resulting propylene in the dehydration (propylene-producing) path is adsorbed on the ZnO:Sb surface to form a π–allyl complex intermediate. This intermediate then reacts with the activated (i.e. adsorbed) oxygen and forms propylene–oxygen complex, which dehydrates to form enal species that finally oxidizes with the adsorbed oxygen producing carbon dioxide and water, thus accelerating the adsorption of oxygen on the surface.
|
 | (7) |
So, eqn (7) suggests that the oxidation of propylene adsorbed on ZnO proceeds in two consecutive steps: the propylene–oxygen complex formation and the oxidation of the complex to CO2, and H2O.
3.2.4 The importance of dehydrogenation versus dehydration paths in solvent transformations. To investigate this mechanism/path (the propylene oxidation path) further, we treated the ZnO:Sb wires with an olefine, which is an alkene (unsaturated compound), just like propylene. Fig. 7 shows the effect of cyclohexene treatment and compares it with IPA and the case with no treatment. It is seen from the figure that cyclohexene treatment leads to an intermediate enhancement of the resistance: while its effect is weaker than that of IPA (as seen from Fig. 7A and B) demonstrates that cyclohexene treatment causes a much stronger enhancement to the photo-resistance than cyclohexane treatment This supports the IPA-transformations hypothesis, especially the dehydrogenation path, as the mechanism responsible for the strong effects of IPA treatment on the photoconductance of ZnO:Sb wires. Yet, its effect is weaker than IPA, possibly due to differences in the structures of the two olefines propylene and cyclohexene.
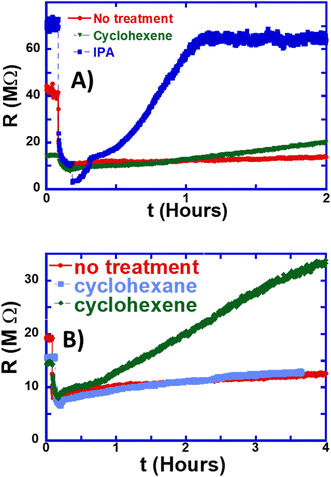 |
| Fig. 7 The effect of cyclohexene (an alkene) treatment on the photo-resistance of a ZnO:Sb wire. (A) Comparison shows that cyclohexene treatment only has a moderate effect on the photoconductance when compared to that of IPA and the untreated wire, within the first 2 hours of the treatment. (B) While cyclohexene treatment causes weaker effects than IPA, yet it does have a stronger effect on the photo-resistance than untreated or cyclohexane-treated wires, which is easily visible over a period of 5 hours. | |
3.2.5 Solvent surface treatment in the absence of light. Light exposure is not required for the IPA treatment of the wires. To rule out any possible role for light in the IPA-treatment effects, Fig. 8A shows the effect of adding an IPA drop on the resistance of a wire kept in darkness for more than 24 hours. The resistance of the wire is initially stable at about 28 MΩ. Adding an IPA drop on the ZnO:Sb wire causes an instant increase in free electrons on its surface, thus decreasing the resistance of the wire. The resistance then increases rapidly when the IPA evaporates, reaches its initial stable resistance within 10 minutes and even reaches twice the initial resistance within 20 minutes of IPA drop evaporation. The resistance finally stabilizes at the new value and never goes back down-in the absence of light, temperature, or ambient atmosphere changes. This strong effect of IPA treatment can be explained by the same IPA-transformations mechanism discussed above, thus demonstrating that light exposure is not required for this enhancement. Adding more IPA drops to increase the treatment time does not seem to have any further effect on the wire resistance, as demonstrated in Fig. 8B. Therefore, the short IPA-treatment achieved by placing one drop of IPA on the wire is sufficient and seems to give the maximum effect.
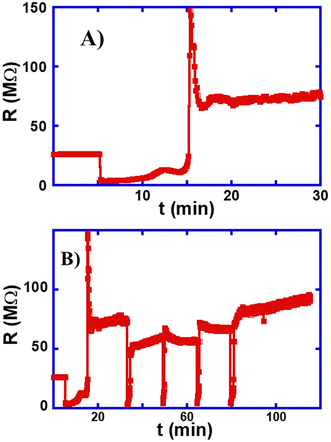 |
| Fig. 8 IPA treatment of a ZnO:Sb wire kept in the dark. (A) A significant and rapid enhancement of the resistance of the wire is observed. This demonstrates that light exposure is not needed while treating the ZnO:Sb wires by immersion in IPA. (B) Repeating the IPA treatment (thus increasing the treatment time) by adding more IPA droplets does not have a strong effect on the resistance of the wire, thus indicating that one short treatment with IPA is sufficient to cause the maximum increase in the wire resistance. | |
3.2.6 Binary oxide catalytic effects. We would like to finally point to the important role played by the Sb atoms on the surface of the wire in amplifying/catalyzing the dehydration reaction that lead to the surface transformations and the large and rapid increase in wire resistance. As L. Saad et al. found,29 the surface transformations/reactions of dehydration (as well as dehydrogenation) of isopropanol were found to be more efficient in ZnO:Sb than in ZnO for a wide range of temperatures. The binary oxide (i.e. Sb and Zn) catalysts were found to be more selective for propylene, as the predominant dehydrated product, which is agreement with our photoconductance and solvent-treatment studies. The single oxide catalyst (i.e. Zn alone in ZnO wires) was found to show higher selectivity towards acetone as the dehydrogenated product. This selectivity increased when increasing the reaction temperature. The added catalytic effect of Sb ions can be understood by considering that the incorporation of more electronegative cations (Sb3+ and Zn2+) increases the density and strength of the acid sites, thus decreasing the activation energy of β-H abstraction. As a result, the isopropanol dehydration on mixed oxide catalysts (like having both Zn and Sb on the surface) is more likely to proceed through a process called the E2 elimination mechanism making it the more likely surface transformations mechanism.32
4. Conclusion
In conclusion, we demonstrated that a short treatment of ZnO:Sb micro-wires with single IPA droplets causes two important effects on the persistent photo-resistance of the wires: the resistance rises by more than 200% and the rise in resistance takes place quite quickly. This has important implications on the applications of ZnO:Sb micro-wires as sensors, where the slow recovery of the resistance to its initial value is a limiting factor to the utilization of the wires in devices. The effects of the IPA treatment were monitored in situ while the resistance was being measured in order to gain comprehensive insight into the process. Furthermore, we found that the mechanism of resistance enhancement is the transformations of the solvent IPA on the ZnO:Sb wire surface. Sb atoms seem to play an important role in catalyzing the reactions and amplifying the treatment effect. Only IPA and cyclohexene seem to cause this effect among other solvents that we studied, including water, ethanol, acetone and cyclohexane.
Data availability
Data for this article, including text files are available at Zenodo repository at [https://zenodo.org/badge/DOI/10.5281/zenodo.13675330.svg] (https://doi.org/10.5281/zenodo.13675330).
Conflicts of interest
There are no conflicts to declare.
Acknowledgements
We would like to thank Shadi K'Aibni and Asem Mubarak from Birzeit University-Testing Laboratories Center and Department of Chemistry for their help preparing the ZnO:Sb wires and characterizing them. We would also like to thank Prof. Atef Qasrawi from Arab American University (AAU)-Physics Department for capturing the SEM images of the ZnO:Sb wires.
References
- M. D. McCluskey and S. J. Jokela, Defects in zno, J. Appl. Phys., 2009, 106(7), 10 CrossRef.
- T. P. Chhetri, L. Kerr, N. Masmali, H. Jaeger and K. F. Eid, Oxygen sensing with individual ZnO: Sb micro-wires: effects of temperature and light exposure on the sensitivity and stability, R. Soc. Open Sci., 2022, 9(1), 211243 CrossRef PubMed.
- F. Claeyssens, C. L. Freeman, N. L. Allan, Ye Sun, M. N. R. Ashfold and J. H. Harding, Growth of ZnO thin films—experiment and theory, J. Mater. Chem., 2005, 15(1), 139–148 RSC.
- P. Yang, H. Yan, S. Mao, R. Russo, J. Johnson, R. Saykally, N. Morris, J. Pham, R. He and H. -J. Choi, Controlled growth of ZnO nanowires and their optical properties, Adv. Funct. Mater., 2002, 12(5), 323–331 Search PubMed.
- M. N. R. Ashfold, R. P. Doherty, N. George Ndifor-Angwafor, D. Jason Riley and Y. Sun, The kinetics of the hydrothermal growth of ZnO nanostructures, Thin Solid Films, 2007, 515(24), 8679–8683 Search PubMed.
- A. M. Alsmadi, B. Salameh, L. L. Kerr and K. F. Eid, Influence of Antimony doping on the electronic, optical and luminescent properties of ZnO microrods, Phys. B, 2018, 545, 519–526 CrossRef CAS.
- A. M. Alsmadi, N. Masmali, H. Jia, J. Guenther, H. Abu Jeib, L. L. Kerr and K. F. Eid, Hot probe measurements of n-type conduction in Sb-doped ZnO microwires, J. Appl. Phys., 2015, 117(15), 155703 CrossRef.
- H.-S. Woo, C. W. Na, Il-D. Kim and J.-H. Lee, Highly sensitive and selective trimethylamine sensor using one-dimensional ZnO–Cr2O3 hetero-nanostructures, Nanotechnology, 2012, 23(24), 245501 CrossRef PubMed.
- D. A. Neamen, Semiconductor Physics and Devices: Basic Principles, McGraw-Hill, 4th eqn, 2011 Search PubMed.
- P. Liu, G. She, Z. Liao, Y. Wang, Z. Wang, W. Shi, X. Zhang, S.-T. Lee and D. Chen, Observation of persistent photoconductance in single ZnO nanotube, Appl. Phys. Lett., 2009, 94(6), 063120 CrossRef.
- Zinc Oxide Bulk, Thin Films and Nanostructures: Processing, Properties, and Applications, ed. C. Jagadish and S. J. Pearton, Elsevier, 2011 Search PubMed.
- Q. A. Drmosh, I. Olanrewaju Alade, M. Qamar and A. Sheikh, Zinc Oxide-Based Acetone Gas Sensors for Breath Analysis: A Review, Chem.–Asian J., 2021, 16(12), 1519–1538 CrossRef CAS PubMed.
- X. Chen, D. Bagnall and N. Nasiri, Capillary-Driven Self-Assembled Microclusters for Highly Performing UV Photodetectors, Adv. Funct. Mater., 2023, 2302808 Search PubMed.
- S.-J. Chang, T.-J. Hsueh, I.-C. Chen, S.-F. Hsieh, S.-P. Chang, C.-L. Hsu, Y.-R. Lin and B.-R. Huang, Highly sensitive ZnO nanowire acetone vapor sensor with Au adsorption, IEEE Trans. Nanotechnol., 2008, 7(6), 754–759 Search PubMed.
- S.-P. Chang, R.-H. Yang and C.-H. Lin, Development of indium titanium zinc oxide thin films used as sensing layer in gas sensor applications, Coatings, 2021, 11(7), 807 CrossRef CAS.
- L. Wang, S. Wang, M. Xu, X. Hu, H. Zhang, Y. Wang and W. Huang, A Au-functionalized ZnO nanowire gas sensor for detection of benzene and toluene, Phys. Chem. Chem. Phys., 2013, 15(40), 17179–17186 RSC.
- W. Park, G. Jo, W.-K. Hong, J. Yoon, M. Choe, S. Lee and Y. Ji, et al., Enhancement in the photodetection of ZnO nanowires by introducing surface-roughness-induced traps, Nanotechnology, 2011, 22(20), 205204 CrossRef PubMed.
- A. Kushwaha, and M. Aslam, Roughness enhanced surface defects and photoconductivity of acid etched ZnO nanowires, in 2012 International Conference on Emerging Electronics, IEEE, 2012, pp. 1–4 Search PubMed.
- L. Guo and L. L. Kerr, Growth of antimony doped zno fiber and its application in dye sensitized solar cells, Funct. Mater. Lett., 2010, 3(4), 279–283 CrossRef CAS.
- J. Cui, G. Pan, X. Yang, M. Zhu, C. Huang and J. Qi, Enhanced acetone sensing performance of CeO2-ZnO at low temperature and its photo-excitation effect, Mater. Sci. Semicond. Process., 2020, 118, 105221 CrossRef CAS.
- J. C. Moore and C. V. Thompson, A phenomenological model for the photocurrent transient relaxation observed in ZnO-based photodetector devices, Sensors, 2013, 13(8), 9921–9940 CrossRef CAS PubMed.
- X. Zhang, X. Han, J. Su, Z. Qi and Y. Gao, Well vertically aligned ZnO nanowire arrays with an ultra-fast recovery time for UV photodetector, Appl. Phys. A, 2012, 107(2), 255–260 CrossRef CAS.
- J. Bao, I. Shalish, Z. Su, R. Gurwitz, F. Capasso, X. Wang and Z. Ren, Photoinduced oxygen release and persistent photoconductivity in ZnO nanowires, Nanoscale Res. Lett., 2011, 6(1), 1–7 CrossRef PubMed.
- A. Dey, Semiconductor metal oxide gas sensors: A review, Mater. Sci. Eng. B, 2018, 229, 206–217 CrossRef CAS.
- V. S. Bhati, M. Hojamberdiev and M. Kumar, Enhanced sensing performance of ZnO nanostructures-based gas sensors: A review, Energy Rep., 2020, 6, 46–62 CrossRef.
- B. Yuliarto, S. Julia, S. Wulan, N. Luh, M. Iqbal and M. F. Ramadhani, The Effect of Tin Addition to ZnO Nanosheet Thin Films for Ethanol and Isopropyl Alcohol Sensor Applications, J. Eng. Technol. Sci., 2015, 47(1), 76–91 CrossRef CAS.
- J. Gao and A. V. Teplyakov, Chemical transformations of acetone on ZnO powder, J. Catal., 2014, 319, 136–141 CrossRef CAS.
- J. M. Vohs and A. B. Mark, Structure sensitivity, selectivity, and adsorbed intermediates in the reactions of acetone and 2-propanol on the polar surfaces of zinc oxide, J. Phys. Chem., 1991, 95(1), 297–302 CrossRef CAS.
- L. Saad and R. Mary, Characterization of various zinc oxide catalysts and their activity in the dehydration-dehydrogenation of isobutanol, J. Serb. Chem. Soc., 2008, 73(10), 997–1009 CrossRef CAS.
- D. Kulkarni and I. E. Wachs, Isopropanol oxidation by pure metal oxide catalysts: number of active surface sites and turnover frequencies, Appl. Catal., A, 2002, 237(1–2), 121–137 CrossRef CAS.
- Y. Osafune, Y. Jin, T. Hirao, M. Tobisu and T. Amaya, Oxovanadium (V)-catalyzed oxidative cross-coupling of enolates using O2 as a terminal oxidant, Chem. Commun., 2020, 56(78), 11697–11700 RSC.
|
This journal is © The Royal Society of Chemistry 2025 |
Click here to see how this site uses Cookies. View our privacy policy here.