DOI:
10.1039/D4RA08380F
(Paper)
RSC Adv., 2025,
15, 1776-1781
Recyclable and air-stable colloidal manganese nanoparticles catalyzed hydrosilylation of alkenes with tertiary silane†
Received
27th November 2024
, Accepted 12th January 2025
First published on 20th January 2025
Abstract
We synthesized N,N-dimethylformamide (DMF)-stabilized manganese nanoparticles (Mn NPs) in a one-step process under air using manganese(II) chloride as the precursor. The Mn NPs were characterized in terms of particle size, oxidation state, and local structure using annular dark-field scanning transmission electron microscopy (ADF-STEM), X-ray photoelectron spectroscopy (XPS), and X-ray absorption spectroscopy (XAS). The results indicate that Mn NPs are divalent nanosized particles with Mn–O bonds. The Mn NPs exhibited high catalytic activity, achieving a turnover number (TON) of 15
800, surpassing previous manganese catalysts in alkene hydrosilylation. Furthermore, the Mn NPs maintained their catalytic activity after the reaction, enabling multiple recycling.
Introduction
Hydrosilylation is essential for the synthesis of organosilicon compounds, which are used in numerous fields including organic synthesis, medicine, and industry.1 In general, precious-metal catalysts such as platinum complexes (Speier's,2 Karstedt's,3 and Markó's4 catalysts) are used for this reaction. However, these metals are rare, toxic, and costly, necessitating the development of alternative catalysts (Fig. 1).5
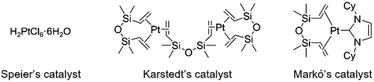 |
| Fig. 1 Platinum complex catalyst for hydrosilylation. | |
In recent years, the utility of earth-abundant first-row-transition-metal and non-noble-metal catalysts has attracted widespread research attention in the chemical sciences community. These investigations have primarily focused on metals such as iron,6 cobalt,7 and nickel8 for hydrosilylation. Manganese is the third most abundant transition metal in Earth's crust and is considered non-toxic and environmentally friendly.9 Manganese is an attractive candidate as a catalyst for a wide range of organic reactions10 and is useful as a catalyst for alkene hydrosilylation.11 In one early study, Faltynek successfully catalyzed hydrosilylation using a triphenylsilyl manganese complex. The reaction involved heptamethylcyclotetrasiloxane (H-HMCTS) and 1-pentene as substrates and was carried out by heating at 180 °C or with UV irradiation at 350 nm (Scheme 1a).12 Later, Thomas's group reported that the combination of a bis(imino)pyridine manganese complex and sodium tert-butoxide exhibited high catalytic activity for hydrosilylation of alkenes with tertiary silanes (Scheme 1b).13 Sodium tert-butoxide is an additive with good catalytic activity, but it is known to react with alkoxides and alkoxysilanes to form flammable SiH4.14 Therefore, challenges remain in the selection of practical additives.
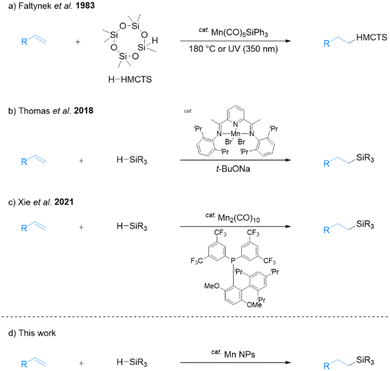 |
| Scheme 1 Manganese-catalyzed hydosilylation. | |
Recently, Xie and co-workers reported that Mn2(CO)10 tuned by the electron-deficient JackiePhos ligand catalyzes the hydrosilylation of alkenes (Scheme 1c).15 In their study, the addition of 2,2,6,6-tetramethylpiperidin-1-oxyl (TEMPO) free radicals almost completely inhibited the reaction, suggesting that a radical mechanism is involved. Specifically, the thermal dissociation of ligand-assisted Mn2(CO)10 generates a manganese-centered radical that subsequently generates a silyl radical that adds to the alkene. Finally, hydrogen atom transfer occurs from the manganese hydride produces the hydrosilylated product. This reaction also showed high catalytic activity for dehydrosilylation upon modifying the ligand. However, these homogeneous catalysts are difficult to recover from the hydrosilylated products, necessitating the development of recyclable heterogeneous catalysts with low environmental impact. Accordingly, the present study focuses on exploring the potential of transition-metal nanoparticles (NPs) as an alternative.
Transition-metal NPs exhibit high catalytic activities, even at low catalyst loadings, owing to their larger surface areas compared with those of the bulk metals.16 NPs exhibit catalytic activities that are different from those of conventional molecular-complex and heterogeneous catalysts, and their physical properties and application to numerous catalytic reactions are an active area of study. Although there are various methods for synthesizing metal NPs, we have focused on the liquid-phase synthesis method. Several methods for synthesizing Mn NPs by liquid-phase synthesis have been reported, but they have not yet been applied catalytically.17
Our group reported that various metal NPs synthesized by a one-step liquid-phase synthesis using N,N-dimethylformamide (DMF)18 exhibited high catalytic activity in organic reactions.19 Specifically, DMF-stabilized Fe2O3 NPs exhibit high catalytic activity for the hydrosilylation of alkenes with primary and secondary silanes, resulting in the formation of hydrosilylated products without additives.20 However, DMF-stabilized Fe2O3 NPs are not effective for hydrosilylation with tertiary silanes. To address this limitation, we reported that a DMF-stabilized combination of Fe2O3 NPs with Pt NPs can catalyze the anti-Markovnikov selective hydrosilylation of alkenes with tertiary silanes.21 While this combination represents a significant improvement of our methodology, it requires the use of Pt. Accordingly, we synthesized manganese NPs using DMF reduction and explored their potential as an effective Pt-free catalyst for hydrosilylation with tertiary silanes (Scheme 1d). The use of DMF-stabilized Mn NPs presents a more cost-effective and sustainable method for catalyzing hydrosilylation reactions, and they can be easily recycled multiple times following a simple extraction process. The present report includes details of the structural characterization of Mn NPs and their application to the hydrosilylation of alkenes with tertiary silanes.
Results and discussion
We synthesized DMF-stabilized Mn NPs from aqueous manganese chloride solution by heating and stirring at 140 °C with a reflux condenser in DMF (Scheme 2 and see ESI† for details). Annular dark-field scanning transmission electron microscopy (ADF-STEM; Fig. 2) and energy dispersive X-ray spectroscopy (EDX; Fig. S8†) show that the average particle size of the Mn NPs before and after hydrosilylation reaction are 2.9 and 7.6 nm, respectively. Thus, the Mn NPs exhibit a significant size increase over the course of hydrosilylation reaction, yet they remain within the nanoscale size range. The coordination state of the DMF layer surrounding the DMF-stabilized Mn NPs was investigated by Fourier transform infrared spectroscopy (FT-IR; Fig. S9†). The C
O and O–C–N vibrational modes of DMF in the Mn NPs were observed to shift relative to the corresponding peaks of DMF molecules. In particular, the strong absorption peak at 1675 cm−1, attributed to the C
O vibration of DMF molecules, shifted to lower wavenumbers of 1672 cm−1 for Mn NPs_br and 1664 cm−1 for Mn NPs_ar indicating an interaction between the C
O groups in DMF and the Mn NPs. Similarly, the O–C–N band of DMF, initially at 658 cm−1, shifted to 660 cm−1 for Mn NPs_br and to 662 cm−1 for Mn NPs_ar. These shifts suggest that the amide groups of DMF interact with the Mn NPs. In addition, Mn NPs_ar showed a peak in the range of 700–900 cm−1, which was not present in Mn NPs_br. These peaks indicate the presence of silane compounds in Mn NPs_ar. The incorporation of these compounds is probably related to the increased particle size of Mn NPs after the hydrosilylation reaction. X-ray diffraction (XRD) analysis could not be performed due to the ultra-small size of the sample (Fig. S10†).
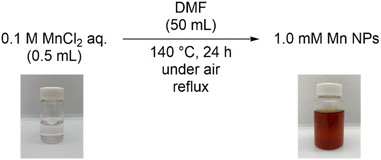 |
| Scheme 2 Synthesis of DMF-stabilized manganese nanoparticles. | |
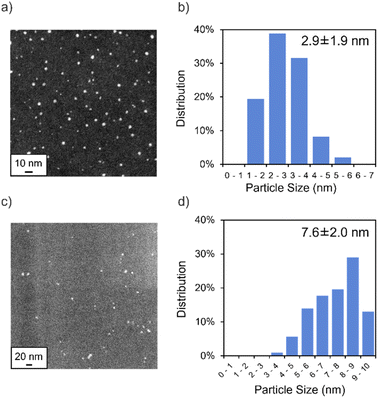 |
| Fig. 2 (a) ADF-STEM image of the Mn NPs before reaction (scale bar = 10 nm) (b) size distribution of the Mn NPs before reaction (c) ADF-STEM image of the Mn NPs after reaction (scale bar = 20 nm) (d) size distribution of the Mn NPs after reaction. | |
The oxidation state of the Mn NPs was analyzed by X-ray photoelectron spectroscopy (XPS) and X-ray absorption spectroscopy (XAS). In the Mn 2p XPS spectrum of the DMF-stabilized Mn NPs, the Mn 2p3/2 peak appears at 640.9 eV (Fig. 3a). The Mn2+ peak for the Mn 2p3/2 region has been reported to occur at 640.8–641.6 eV.17,22 Furthermore, satellite peaks (shake-up) for MnO (Mn2+) are observed. These suggest that the DMF-stabilized Mn NPs are divalent.
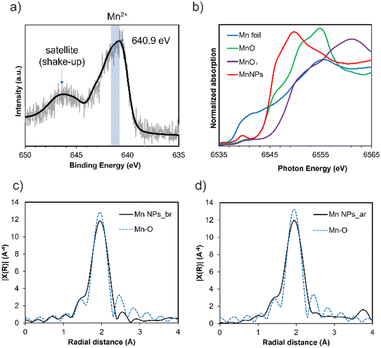 |
| Fig. 3 (a) XPS profiles for the Mn 2p3/2 region for Mn NPs and (b) Mn K-edge XANES spectra of the reference Mn compounds and Mn NPs, and (c) Fourier-transformed k3-weighted Mn K-edge EXAFS spectra of Mn NPs_before reaction (Mn NPs_br) and (d) after reaction (Mn NPs_ar). | |
X-ray absorption near edge structure (XANES) analysis of the DMF-stabilized Mn NPs was conducted, and the results were compared with those for Mn(0) foil, MnO pellets, and MnO2 pellets. The position of the absorption edge of the Mn NPs is similar to that of MnO (E0: Mn NPs = 6545.2 eV, MnO = 6545.1 eV), indicating that they are divalent (Fig. 3b). E0 was calculated by the local maximum of the first derivative of the XANES spectrum (dμ/dE). This result is consistent with the XPS results and indicates that the oxidation state of the Mn NPs is divalent. The E0 of 6545.2 eV for Mn NPs after the reaction suggests that the Mn NPs remain divalent throughout the reaction (Fig. S6†). There is a slight difference in the XANES spectra shapes of Mn NPs and MnO. This can be attributed to the liquid form of the XAS sample of Mn NPs and the presence of chloride ions in the precursor (Fig. S7†). In addition, the XPS spectrum of Mn NPs revealed a chloride peak, suggesting the presence of residual chloride species (Fig. S3†).
Next, the extended X-ray absorption fine structure (EXAFS) spectra were Fourier transformed into radial space (R-space) to analyze the local structure around the Mn NPs (Fig. 3c and d). The results show that before the reaction, the results for Mn NPs can be fitted to Mn–O bonds with a bond distance of 2.26 Å and a coordination number of 4.1 (Table S2†). After the reaction, Mn–O bonds distance of 2.25 Å and coordination number 4.0 were found to best fit the results. This suggests that Mn–O bonds are present before and after the reaction and that the coordination atom species around the Mn atom are the same in the Mn NPs before and after the reaction. This indicated that the Mn NPs would be recyclable. To summarize the XPS and XAS analysis, the XPS and XANES results indicate that the oxidation state of the Mn NPs is divalent and that residual chloride ions are present, whereas the EXAFS results confirm that the structure of the Mn NPs consists of Mn–O bonds.
We investigated the Mn NPs-catalyzed hydrosilylation of 1-dodecene (1a: 0.5 mmol) with methyldiphenylsilane (2a: 2.5 mmol) as model substrates under various conditions (Table 1). For instance, the reaction of 1a with 2a in the presence of Mn NPs (0.01 mol%) at 130 °C for 24 h gave the main product 3a in 70% yield (entry 1). GC and GC-MS analysis confirmed that no by-products such as Markovnikov or vinylsilane products were formed in this catalytic reaction. Performing the reaction without a catalyst confirmed that the catalyst is required for this reaction to proceed (entry 2). Upon lowering the temperature to 100 °C, the reaction did not proceed and no hydrosilylated product was obtained (entry 3). The use of diglyme, a high-boiling-point ether solvent, improved the yield of 3a (entry 4). This improvement is attributed to the high dispersity of the Mn NPs in the solvent. Since this Mn NPs has Mn–O bonds, MnO with these bonds were used as catalysts for the reaction. As a result, the corresponding product was not observed with MnO because the substrate was hardly converted (entry 5). A key difference between Mn NPs and MnO is their dispersibility in the reaction solution: MnO is insoluble, while Mn NPs are highly dispersed in the solution. This clear difference contributes to the catalytic activity of Mn NPs in hydrosilylation. The high dispersion of Mn NPs in the solution is thought to facilitate reactions with the substrate, leading to the generation of active species, such as silyl radicals, which subsequently lead to the formation of the desired product. When the model reaction was performed in the presence of a catalytic amount of TEMPO or 3,5-di-tert-butyl-4-hydroxytoluene (BHT) as a radical scavenger, hydrosilylation was inhibited (entry 6 and Table S6†). Moreover, the silyl radical adduct was detected by HRMS when TEMPO was added, indicating that silyl radicals are the active species in the reaction (Fig. S11, S12 and S15†). Since residual chlorine ions were observed in the Mn NPs, the reaction was carried out using HCl as the acid catalyst (Table S7,† entry 3). As a result, although the conversion was high, isomerization of alkenes and disproportionation of hydrosilanes occurred, resulting in low yields.
Table 1 Optimization of Mn NPs-catalyzed hydrosilylation of 1-dodecene (1a) with methyldiphenylsilane (2a)a

|
Entry |
Reaction conditions |
Yieldb c (%) |
Reaction conditions: 1-dodecene 1a (0.5 mmol), methyldiphenylsilane 2a (2.5 mmol) and Mn NPs (0.01 mol%) at 130 °C for 24 h under Ar atmosphere. Yields were determined by GC based on 1a used (n-nonane as internal standard). nd = not detected by GC. Number in square brackets show the isolated yield. |
1 |
Standard condition |
70 |
2 |
In the absence of Mn NPs |
nd |
3 |
100 °C |
nd |
4 |
Diglyme (1 mL) as the solvent |
86 [83]d |
5 |
MnO (20 mol%) instead of Mn NPs |
nd |
6 |
TEMPO (20 mol%) |
nd |
The substrate scope of the alkene hydrosilylation was investigated with various alkenes and hydrosilanes (Table 2). Aliphatic alkenes and allylbenzenes gave the corresponding products in good yields (3a–f). Oxygen-containing functional alkenes, such as ketones, esters, epoxides, and alcohol, were well-tolerated in the reaction, yielding the corresponding products (3g–n) with good to moderate yields. In addition, in the presence of carbonyl derivatives, the reaction selectively proceeded towards the alkene hydrosilylation products (3g–i), while exhibiting no reactivity towards the C
O groups. The desired product was obtained even when cyclooctene, an internal alkene, was used (3o). The screening of other hydrosilanes, such as HSiMe2Ph, a secondary silane (H2SiPh2), and a primary silane (H3SiPh), demonstrated their amenability to the reaction system, affording the desired products in good to excellent yields (3p–r).
Table 2 Substrate scope of Mn NPs-catalyzed hydrosilylationa
Reaction conditions: alkenes 1 (0.5 mmol), hydrosilanes 2 (2.5 mmol) and Mn NPs (0.01 mol%) in 1.0 mL diglyme at 130 °C for 24 h under Ar atmosphere, unless otherwise noted. The structure was identified by NMR measurement. Yields of isolated product after purification. Reaction was proceeded at 110 °C. Reaction was proceeded at 100 °C. |
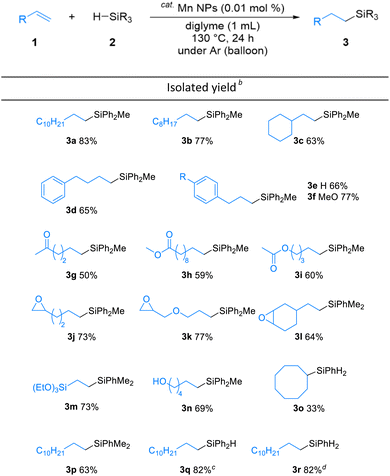 |
To further demonstrate the synthetic utility of the Mn NPs catalyst, we conducted a multi-gram scale hydrosilylation (Scheme 3). The reaction of 1a (10 mmol) and 2b (20 mmol) afforded the hydrosilylated products in a yield of 79%. By scaling up, it was possible to reduce the substrate-to-hydrosilane ratio as well as decrease the catalyst amount to 0.005% (TON = 15
800). This TON represents the highest value reported to date for manganese-catalyzed hydrosilylation of alkenes (Scheme S3†).
 |
| Scheme 3 Multi-gram scale hydrosilylation. | |
Recycling experiments were then conducted to evaluate the activity of the catalyst after the reaction. In the recycling procedure, hexane (8 mL) and DMF (2 mL) were added to the solution after the reaction, and the solution was separated into two layers (an upper hexane layer and lower DMF layer). The solution was then extracted five times with hexane (8 mL), recovering the substrate and product in the hexane layer and leaving the catalyst in the DMF layer. The recovered catalyst was recycled for subsequent hydrosilylation after DMF evaporation. The Mn NPs remained catalytically active, as the desired product was obtained in high yield even after five recycles (Fig. 4).
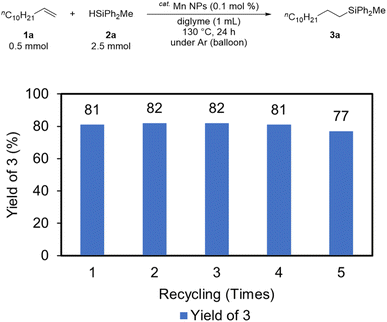 |
| Fig. 4 Recycling experiment result for Mn NPs-catalyzed hydrosilylation of 1a with 2a. | |
Conclusions
In summary, we synthesized DMF-stabilized Mn NPs using a DMF-reduction method and characterized them as divalent Mn NPs with Mn–O bonds (1–5 nm) by annular dark-field scanning transmission electron microscope, energy dispersive X-ray, X-ray photoelectron spectroscopy, X-ray adsorption near-edge structure, and extended X-ray absorption fine structure analyses. The Mn NPs catalyzed hydrosilylation reactions in high yields and high product selectivities at low catalyst loading, and they were also found to be applicable to a wide variety of substrates and to be recyclable multiple times.
Data availability
The data supporting this article have been included as part of the ESI.†
Author contributions
N. K. conducted the experiments, performed the analysis, and wrote the manuscript. Y. O. supervised the work and edited the manuscript. T. N. and M. Y. contributed to the experimental work. N. K., K. T., T. N., T. T., Y. J., Z. M., K. S., and T. W. performed the XAS measurements and analyses. T. S. conducted the TEM and ICP analysis. The manuscript was written through contribution of all authors. All authors have given approval to the final version of the manuscript.
Conflicts of interest
There are no conflicts to declare.
Acknowledgements
This work was supported by the “Development of Innovative Catalytic Processes for Organosilicon Functional Materials” project (PL: Kazuhiko Sato) from the New Energy and Industrial Technology Development Organization (NEDO), the Joint Usage/Research Center for Catalysis (Proposal 23DS0173), and JST SPRING, Grant Number JPMJSP2150. We thank Mr Yosuke Murakami of the Comprehensive Analysis Center, SANKEN (ISIR), Osaka University, for his assistance with TEM analysis. The synchrotron radiation experiments were conducted at beamline BL14B2 of SPring-8 with the approval of the Japan Synchrotron Radiation Research Institute (JASRI) (Proposal No. 2021A1615, 2022B1952).
References
-
(a) N. Katayama and Y. Obora, Chem. Lett., 2024, 53, upae134 CrossRef;
(b) A. Roy and M. Oestreich, Angew. Chem., Int. Ed., 2021, 60, 4408–4410 CrossRef CAS PubMed;
(c) E. Rémond, C. Martin, J. Martinez and F. Cavelier, Chem. Rev., 2016, 116, 11654–11684 CrossRef PubMed;
(d) S. E. Denmark and A. Ambrosi, Org. Process Res. Dev., 2015, 19, 982–994 CrossRef CAS PubMed;
(e) A. K. Franz and S. O. Wilson, J. Med. Chem., 2013, 56, 388–405 CrossRef CAS PubMed;
(f) H. F. Sore, W. R. J. D. Galloway and D. R. Spring, Chem. Soc. Rev., 2012, 41, 1845–1866 RSC;
(g) Y. Nakao and T. Hiyama, Chem. Soc. Rev., 2011, 40, 4893–4901 RSC;
(h) D. Troegel and J. Stohrer, Coord. Chem. Rev., 2011, 255, 1440–1459 CrossRef CAS;
(i) C. Sanchez, P. Belleville, M. Popall and L. Nicole, Chem. Soc. Rev., 2011, 40, 696–753 RSC;
(j) B. Marciniec, Coord. Chem. Rev., 2005, 249, 2374–2390 CrossRef CAS;
(k) G. A. Showell and J. S. Mills, Drug Discovery Today, 2003, 8, 551–556 CrossRef CAS PubMed.
- J. L. Speier, J. A. Webster and G. H. Barnes, J. Am. Chem. Soc., 1957, 79, 974–979 CrossRef CAS.
- B. D. Karstedt, General Electric Company, US3775452A, 1973.
-
(a) S. Dierick, E. Vercruysse, G. Berthon-Gelloz and I. E. Markó, Chem.–Eur. J., 2015, 21, 17073–17078 CrossRef CAS PubMed;
(b) O. Buisine, G. Berthon-Gelloz, J.-F. Brière, S. Stérin, G. Mignani, P. Branlard, B. Tinant, J.-P. Declercq and I. E. Markó, Chem. Commun., 2005, 3856–3858 RSC;
(c) I. E. Markó, S. Stérin, O. Buisine, G. Mignani, P. Branlard, B. Tinant and J.-P. Declercq, Science, 2002, 298, 204–206 CrossRef PubMed.
-
(a) L. D. de Almeida, H. Wang, K. Junge, X. Cui and M. Beller, Angew. Chem., Int. Ed., 2021, 60, 550–565 CrossRef CAS;
(b) Y. Naganawa, K. Inomata, K. Sato and Y. Nakajima, Tetrahedron Lett., 2020, 61, 151513 CrossRef CAS;
(c) J. V. Obligacion and P. J. Chirik, Nat. Rev. Chem, 2018, 2, 15–34 CrossRef CAS PubMed;
(d) X. Du and Z. Huang, ACS Catal., 2017, 7, 1227–1243 CrossRef CAS;
(e) Y. Nakajima and S. Shimada, RSC Adv., 2015, 5, 20603–20616 RSC.
-
(a) Y. Bai, J. Liu, T. Ouyang, H. Lan, J. Peng and J. Li, Appl. Organomet. Chem., 2024, 38, e7493 CrossRef CAS;
(b) W. Sun, M.-P. Li, L.-J. Li, Q. Huang, M.-Y. Hu and S.-F. Zhu, Chem. Sci., 2022, 13, 2721–2728 RSC;
(c) P. W. Smith, Y. Dong and T. D. Tilley, Chem. Sci., 2020, 11, 7070–7075 RSC;
(d) R. Agahi, A. J. Challinor, J. Dunne, J. H. Docherty, N. B. Carter and S. P. Thomas, Chem. Sci., 2019, 10, 5079–5084 RSC;
(e) M.-Y. Hu, Q. He, S.-J. Fan, Z.-C. Wang, L.-Y. Liu, Y.-J. Mu, Q. Peng and S.-F. Zhu, Nat. Commun., 2018, 9, 221 CrossRef PubMed;
(f) Y. Toya, K. Hayasaka and H. Nakazawa, Organometallics, 2017, 36, 1727–1735 CrossRef CAS;
(g) A. J. Challinor, M. Calin, G. S. Nichol, N. B. Carter and S. P. Thomas, Adv. Synth. Catal., 2016, 358, 2404–2409 CrossRef CAS;
(h) X. Du, Y. Zhang, D. Peng and Z. Huang, Angew. Chem., Int. Ed., 2016, 55, 6671–6675 CrossRef CAS;
(i) A. M. Tondreau, C. C. H. Atienza, K. J. Weller, S. A. Nye, K. M. Lewis, J. G. P. Delis and P. J. Chirik, Science, 2012, 335, 567–570 CrossRef CAS PubMed;
(j) A. N. Nesmeyanov, R. K. Freidlina, E. C. Chukovskaya, R. G. Petrova and A. B. Belyavsky, Tetrahedron, 1962, 17, 61–68 CrossRef CAS.
-
(a) J. Jose and T. V. Mathew, ChemCatChem, 2024, 16, e202301626 CrossRef CAS;
(b) K. Kobayashi, N. Fukaya and H. Nakazawa, New J. Chem., 2023, 47, 11784–11791 RSC;
(c) S.-N. Yang, C.-H. Liu, L.-B. He, H. Zheng, C.-S. Kuai, B. Wan, D.-W. Ji and Q.-A. Chen, Org. Chem. Front., 2023, 10, 2204–2210 RSC;
(d) Y. Gao, L. Wang and L. Deng, ACS Catal., 2018, 8, 9637–9646 CrossRef CAS;
(e) B. Raya, S. Jing and T. V. RajanBabu, ACS Catal., 2017, 7, 2275–2283 CrossRef CAS;
(f) K. L. Lee, Angew. Chem., Int. Ed., 2017, 56, 3665–3669 CrossRef CAS;
(g) Y. Liu and L. Deng, J. Am. Chem. Soc., 2017, 139, 1798–1801 CrossRef CAS;
(h) C. Wang, W. J. Teo and S. Ge, ACS Catal., 2017, 7, 855–863 CrossRef CAS;
(i) J. Sun and L. Deng, ACS Catal., 2016, 6, 290–300 CrossRef CAS;
(j) A. J. Chalk and J. F. Harrod, J. Am. Chem. Soc., 1967, 89, 1640–1647 CrossRef CAS.
-
(a) A. S.-M. Chang, K. E. Kawamura, H. S. Henness, V. M. Salpino, J. C. Greene, L. N. Zakharov and A. K. Cook, ACS Catal., 2022, 12, 11002–11014 CrossRef CAS;
(b) D. Bai, F. Wu, L. Chang, M. Wang, H. Wu and J. Chang, Angew. Chem., Int. Ed., 2022, 61, e202114918 CrossRef CAS PubMed;
(c) T. Hashimoto, K. Shiota, T. Ishimaru and Y. Yamaguchi, Eur. J. Org Chem., 2021, 2021, 5449–5452 CrossRef CAS;
(d) X. Wu, G. Ding, W. Lu, L. Yang, J. Wang, Y. Zhang, X. Xie and Z. Zhang, Org. Lett., 2021, 23, 1434–1439 CrossRef CAS;
(e) J. Yang, V. Postils, M. I. Lipschutz, M. Fasulo, C. Raynaud, E. Clot, O. Eisenstein and T. D. Tilley, Chem. Sci., 2020, 11, 5043–5051 RSC;
(f) G. Vijaykumar, A. Pariyar, J. Ahmed, B. K. Shaw, D. Adhikari and S. K. Mandal, Chem. Sci., 2018, 9, 2817–2825 RSC;
(g) Y. Nakajima, K. Sato and S. Shimada, Chem. Rec., 2016, 16, 2379–2387 CrossRef CAS PubMed;
(h) I. Pappas, S. Treacy and P. J. Chirik, ACS Catal., 2016, 6, 4105–4109 CrossRef CAS;
(i) I. Buslov, J. Becouse, S. Mazza, M. Montandon-Clerc and X. Hu, Angew. Chem., Int. Ed., 2015, 54, 14523–14526 CrossRef CAS PubMed;
(j) H. Maciejewski, B. Marciniec and I. Kownacki, J. Organomet. Chem., 2000, 597, 175–181 CrossRef CAS.
- D. A. Valyaev, G. Lavigne and N. Lugan, Coord. Chem. Rev., 2016, 308, 191–235 CrossRef CAS.
-
(a) A. Torres-Calis and J. J. García, ACS Omega, 2022, 7, 37008–37038 CrossRef CAS PubMed;
(b) S. Weber and K. Kirchner, Acc. Chem. Res., 2022, 55, 2740–2751 CrossRef CAS PubMed;
(c) K. Das, S. Waiba, A. Jana and B. Maji, Chem. Soc. Rev., 2022, 51, 4386–4464 RSC;
(d) E. S. Gulyaeva, E. S. Osipova, R. Buhaibeh, Y. Canac, J.-B. Sortais and D. A. Valyaev, Coord. Chem. Rev., 2022, 458, 214421 CrossRef CAS.
-
(a) I. K. Goncharova, S. A. Filatov, A. P. Drozdov, A. A. Tereshchenko, P. A. Knyazev, A. A. Guda, I. P. Beletskaya and A. V. Arzumanyan, J. Catal., 2024, 429, 115269 CrossRef CAS;
(b) N. Katayama and Y. Obora, Tetrahedron Lett., 2023, 132, 154798 CrossRef CAS;
(c) A. Vivien, L. Veyre, R. Mirgalet, C. Camp and C. Thieuleux, Green Chem., 2023, 25, 7721–7728 RSC;
(d) A. Vivien, L. Veyre, R. Mirgalet, C. Camp and C. Thieuleux, Chem. Commun., 2022, 58, 4091–4094 RSC;
(e) X. Yang and C. Wang, Chin. J. Chem., 2018, 36, 1047–1051 CrossRef CAS;
(f) T. K. Mukhopadhyay, M. Flores, T. L. Groy and R. J. Trovitch, Chem. Sci., 2018, 9, 7673–7680 RSC;
(g) C. Obradors, R. M. Martinez and R. A. Shenvi, J. Am. Chem. Soc., 2016, 138, 4962–4971 CrossRef CAS PubMed;
(h) W. Jondi, A. Zyoud, W. Mansour, A. Q. Hussein and H. S. Hilal, React. Chem. Eng., 2016, 1, 194–203 RSC;
(i) H. S. Hilal, M. A. Suleiman, W. J. Jondi, S. Khalaf and M. M. Masoud, J. Mol. Catal. A:Chem., 1999, 144, 47–59 CrossRef CAS;
(j) H. S. Hilal, M. Abu-Eid, M. Al-Subu and S. Khalaf, J. Mol. Catal., 1987, 39, 1–11 CrossRef CAS.
- S. L. Pratt and R. A. Faltynek, J. Organomet. Chem., 1983, 258, C5–C8 CrossRef CAS.
- J. R. Carney, B. R. Dillon, L. Campbell and S. P. Thomas, Angew. Chem., Int. Ed., 2018, 57, 10620–10624 CrossRef CAS PubMed.
- J. H. Docherty, J. Peng, A. P. Dominey and S. P. Thomas, Nat. Chem., 2017, 9, 595–600 CrossRef CAS PubMed.
- J. Dong, X.-A. Yuan, Z. Yan, L. Mu, J. Ma, C. Zhu and J. Xie, Nat. Chem., 2021, 13, 182–190 CrossRef CAS PubMed.
-
(a) J. M. Asensio, D. Bouzouita, P. W. N. M. van Leeuwen and B. Chaudret, Chem. Rev., 2020, 120, 1042–1084 CrossRef CAS PubMed;
(b) L. Liu and A. Corma, Chem. Rev., 2018, 118, 4981–5079 CrossRef CAS PubMed;
(c) Q. Zhang, W. Deng and Y. Wang, Chem. Commun., 2011, 47, 9275–9292 RSC.
-
(a) M. Mauri, V. Collico, L. Morelli, P. Das, I. García, J. Penaranda Avila, M. Bellini, R. Rotem, M. Truffi, F. Corsi, R. Simonutti, L. M. Liz-Marzán, M. Colombo and D. Prosperi, ACS Appl. Nano Mater., 2020, 3, 3787–3797 CrossRef CAS;
(b) V. Hoseinpour and N. Ghaemi, J. Photochem. Photobiol., B, 2018, 189, 234–243 CrossRef CAS PubMed;
(c) J. F. Bondi, K. D. Oyler, X. Ke, P. Schiffer and R. E. Schaak, J. Am. Chem. Soc., 2009, 131, 9144–9145 CrossRef CAS PubMed.
-
(a) T. S. Rodrigues, M. Zhao, T.-H. Yang, K. D. Gilroy, A. G. M. da Silva, P. H. C. Camargo and Y. Xia, Chem.–Eur. J., 2018, 24, 16944–16963 CrossRef CAS PubMed;
(b) I. Pastoriza-Santos and L. M. Liz-Marzán, Adv. Funct. Mater., 2009, 19, 679–688 CrossRef CAS;
(c) X. Liu, C. Li, J. Xu, J. Lv, M. Zhu, Y. Guo, S. Cui, H. Liu, S. Wang and Y. Li, J. Phys. Chem. C, 2008, 112, 10778–10783 CrossRef CAS.
-
(a) H. Iguchi, N. Katayama, T. Suzuki, T. Fujihara, Y. Jing, T. Toyao, Z. Maeno, K.-I. Shimizu and Y. Obora, Chem. Commun., 2022, 58, 11851–11854 RSC;
(b) T. Nagata, K. Okada, R. Kondo, T. Toyao, K.-I. Shimizu, T. Suzuki and Y. Obora, RSC Adv., 2022, 12, 16599–16603 RSC;
(c) T. Nagata and Y. Obora, ACS Omega, 2020, 5, 98–103 CrossRef CAS PubMed;
(d) M. Hyotanishi, Y. Isomura, H. Yamamoto, H. Kawasaki and Y. Obora, Chem. Commun., 2011, 47, 5750–5752 RSC.
- R. Azuma, S. Nakamichi, J. Kimura, H. Yano, H. Kawasaki, T. Suzuki, R. Kondo, Y. Kanda, K.-I. Shimizu, K. Kato and Y. Obora, ChemCatChem, 2018, 10, 2378–2382 CrossRef CAS.
- T. Nagata, T. Tanaka, X. Lin, R. Kondo, T. Suzuki, Y. Kanda, T. Toyao, K.-I. Shimizu and Y. Obora, ChemCatChem, 2022, 14, e202101672 CrossRef CAS.
-
(a) Y. Kita, M. Kuwabara, K. Kamata and M. Hara, ACS Catal., 2022, 12, 11767–11775 CrossRef CAS;
(b) B. Eren and A. R. Head, J. Phys. Chem. C, 2020, 124, 3557–3563 CrossRef CAS.
|
This journal is © The Royal Society of Chemistry 2025 |
Click here to see how this site uses Cookies. View our privacy policy here.