DOI:
10.1039/D4RE00334A
(Minireview)
React. Chem. Eng., 2025,
10, 10-21
Bifunctional catalysts for the coupling processes of CO2 capture and conversion: a minireview
Received
9th July 2024
, Accepted 3rd October 2024
First published on 17th October 2024
Abstract
The integrated CO2 capture and utilization (ICCU) process can simultaneously achieve the reduction of CO2 emissions and the production of high-value products. Bifunctional catalysts with coupled catalytic and adsorption functions show great potential in the realization of ICCU. The aim of this study is to comprehensively review the recent research progress in the development of bifunctional catalysts. We first review the bifunctional catalysts used in the integrated CO2 capture and hydrogenation reaction including the RWGS and methanation reaction (ICCU-RWGS and ICCU-methanation). The effects of the adsorbent and catalyst components of bifunctional catalysts on the performance of ICCU-RWGS and ICCU-methanation including adsorption efficiency and catalytic activity are summarized. Then, we present the bifunctional catalysts applied in the integrated CO2 capture and dry reforming reaction (ICCU-DR). The optimization of bifunctional catalysts to improve their cyclic stability is reviewed. Finally, the applications of bifunctional catalysts that were developed for the integrated ICCU and sorption-enhanced steam reforming (SESR) reaction are demonstrated.
1. Introduction
As one of the most serious problems facing modern society today, global warming has been caused by the continuous increase of CO2 content in the atmosphere over the past century and a half.1 The excessive CO2 contributes to serious environmental problems such as rising sea levels, extreme weather, and ocean acidification.2 Meanwhile, recent studies have confirmed that the rising CO2 concentration threatens seafood production and degrades the nutritional value of rice.3,4 In this context, many researchers have been working on CO2 conversion and utilization processes for the past few decades to achieve the anthropogenic carbon cycle.5,6 Among them, the catalytic conversion of CO2 in the thermochemical process has received extensive attention as it not only can reduce CO2 emission but also directly produces a variety of valuable chemicals and fuels.7 Unfortunately, these processes often require purified CO2 as a reactant, where multiple steps including CO2 capture, sequestration and transportation are needed,8 which inevitably increases the costs and complicates system configuration.
In comparison, the integration of CO2 capture and utilization (ICCU) into a single process offers an alternative more promising strategy for the direct conversion of CO2 into valuable products, which saves the significant energy, facilities, and expense of the conventional catalytic conversion with separated process units.8 Therefore, there is increasing interest in the ICCU technology. To date, various ICCU processes have been developed through a combination of different CO2 conversion and CO2 capture processes, like the integrated hydrogenation reaction and CO2 capture9,10 and the integrated dry reforming reaction and CO2 capture.11 Different sources of CO2, like simulated flue gas conditions,12 air,13 and the sorption-enhanced steam reforming reaction (SESR),14 have also been studied in ICCU processes. On the other hand, ICCU processes can be coupled with chemical looping reforming reactions to improve energy efficiency.15,16
In general, ICCU processes need a sorbent to capture CO2 and a catalyst to subsequently convert the captured CO2. The sorbent and catalyst particles can be physically mixed in a single bed or packed separately in distinct beds.17 This strategy of physical mixing has the advantages of easy realization and low complexity,18 as the optimization of the sorbent and catalyst particles can be carried out independently. In addition, ICCU can also be performed using bifunctional catalysts that incorporate both catalytic and adsorption capabilities into one material.19,20 The intimate contact between different functional sites in a bifunctional catalyst can enhance mass and heat transfer of the conversion process, so it shows unique performance advantages in terms of ICCU technology. Moreover, the bifunctional catalyst can also reduce the volume of the reactor required, which is more attractive for ICCU process intensification.
Bifunctional catalysts typically consist of alkaline earth or alkali metals (K2O, Na2O, Li2O, MgO, and CaO) for capturing CO2 and transition metal catalysts (Ni, Ru, Pt, and Rh) for CO2 conversion. This minireview focuses on an overview of bifunctional catalysts applied to various ICCU processes, including the integrated CO2 capture and methanation reaction (ICCU-methanation), reverse water–gas shift reaction (ICCU-RWGS), and dry reforming of methane (ICCU-DRM) and other alkanes. On the other hand, this review also discusses the application of bifunctional catalysts for the integrated process of CO2 captured from the SESR reaction for ICCU.
2. Bifunctional catalysts for the integrated CO2 capture and hydrogenation reaction
Reducing gases (H2, CH4, or other alkanes) are usually required to convert captured CO2 and simultaneously regenerate the bifunctional catalyst in the ICCU process. Among them, hydrogen is the most commonly used reducing agent,21,22 especially green hydrogen obtained from water electrolysis technology using renewable energy sources such as solar, biomass, and wind. The integrated CO2 capture and hydrogenation reaction (ICCH, Fig. 1) can produce CO and CH4 through the RWGS reaction and methanation reaction, respectively. | 4H2 + CO2 → CH4 + 2H2O ΔH298K = −165 kJ mol−1 | (1) |
| CO2 + H2 → CO + H2O ΔH298K = 41.2 kJ mol−1 | (2) |
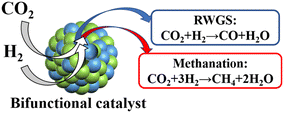 |
| Fig. 1 Conceptual diagram of ICCH processes. | |
2.1. Bifunctional catalysts for ICCU-RWGS
The ICCU-RWGS process is usually more feasible to achieve at high temperatures (>500 °C) because of the endothermic nature of the RWGS reaction. To meet the requirements of high temperature conversion, the adsorptive component of bifunctional catalysts is usually CaO, which is a high temperature CO2 adsorbent.9,23,24 A few studies have also reported Na salts and K salts as adsorptive components for bifunctional catalysts.25,26 On the other hand, although the ICCU-RWGS process often operates with bifunctional catalysts, it can be also performed using transition-metal-free bifunctional catalysts. Removing the active metal component from the bifunctional catalyst can reduce the material costs. On the other hand, it also avoids the deactivation problem of sintering or coking faced by transition metal-based bifunctional catalysts.27
2.1.1. Transition-metal-free bifunctional catalysts for ICCU-RWGS.
Kosaka et al. have reported that Na/γ-Al2O3 can be used as a bifunctional catalyst for the ICCU-RWGS process at 450–500 °C, and 90% conversion of CO2 with 95% selectivity to CO was obtained from 400 ppm CO2.28 However, Na/γ-Al2O3 has low capture capacity of CO2, which needs to be further improved. Compared with alkali metals, CaO may be a better choice because of its high CO2 adsorption capacity. Wu et al. reported that CaO-alone as both an effective adsorbent and catalyst can efficiently achieve CO2 capture and in situ conversion in the ICCU-RWGS process.27 75% conversion of the captured CO2 with a 4.15 mmol gCaO−1 yield of CO was achieved at 700 °C. Meanwhile, no CH4 was detected during the entire conversion process, and the captured CO2 could be completely converted to CO. Recently, they have successfully applied natural marble in the ICCU-RWGS process, and a high CO2 capture capacity of 9.4 mmol g−1 with 85% conversion of the captured CO2 and almost 100% CO selectivity was reported at 650 °C,29 which improves the cost-effectiveness of ICCU technology by using cheap and robust materials. Li et al. have further studied the ICCU-RWGS performance of limestone under iso-thermal conditions using micro-fluidized bed thermogravimetric analysis coupled with mass spectrometry (MFB-TGA-MS, Fig. 2).30 They showed that the CO2 conversion and CO selectivity can reach 79.1% and ∼100% at 710 °C, respectively. Moreover, the generated CaO has self-catalytic activity, resulting in a 5 times higher decomposition rate of limestone in H2 than in Ar.
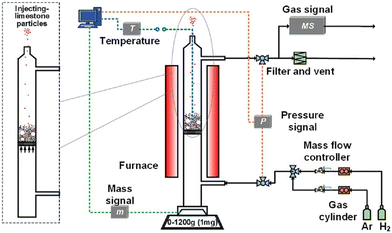 |
| Fig. 2 Schematic diagram of MFB-TGA-MS.30 | |
Although high reaction temperature is beneficial for CO generation, the CO2 conversion will decrease with the increase of reaction temperature due to the enhanced CO2 desorption kinetics.27 Typically, the inevitable decomposition of CaCO3 limits the CO2 conversion to no more than 80% during the utilization stage.31 Recently, Wu et al. proposed a novel strategy to improve the CO2 conversion of the ICCU-RWGS process by promoting direct hydrogenation of carbonates and hindering the decomposition process of CaCO3.31 They reported potassium-promoted CaO (K-CaO, Fig. 3) as a bifunctional catalyst with a CO2 conversion of >95% and a CO selectivity of nearly 100% during 50 hydrogenation cycles at 650 °C. The result showed that K species participated in the carbonation process of CaO to form stable K2Ca(CO3)2 with septal K2CO3 and CaCO3 layers. And the formation of K2Ca(CO3)2 facilitated the direct gas–solid hydrogenation of carbonates and highly suppressed the CaCO3 decomposition reaction. This work provides a simple and efficient design strategy for bifunctional catalysts used in ICCU-RWGS.
 |
| Fig. 3 (a) Schematic diagram and key reactions of ICCU. (b) The real time and (c) cyclic ICCU performance with 20 mol% K–CaO as a bifunctional catalyst at 650 °C.31 | |
2.1.2. Transition metal-containing bifunctional catalysts for ICCU-RWGS.
The transition-metal-free bifunctional catalysts have a cost advantage in the ICCU-RWGS process, but the limited kinetics of CO formation should not be underestimated due to the absence of catalytic sites. On the other hand, bifunctional catalysts containing active catalytic sites also provide more possibilities for optimizing ICCU-RWGS processes by lowering the reaction temperature. Urakawa et al. developed an FeCrCu/K/MgO–Al2O3 bifunctional catalyst and showed that the ICCU-RWGS reaction could be conducted at 450–550 °C.32 Recently, they found that a temperature of 350 °C is required on a K-promoted Cu/γ-Al2O3 catalyst to activate the dissociation of H2 and generate the active site to capture CO2.25 However, CH4 is often detected in these studies because of the exothermic nature of CO2 methanation. Shimizu et al. reported that Na-promoted Pt nanoparticles supported by Al2O3 (Pt–Na/Al2O3) could act as an effective bifunctional catalyst and found that Na species could inhibit the adsorption of the formed CO on the surface of Pt nanoparticles, suppressing the successive reduction of CO to CH4, thus leading to high selectivity to CO at a mild reaction temperature of 350 °C.26 In general, bifunctional catalysts with alkali metals as sorbent components used in the ICCU-RWGS process can operate at relatively low temperatures (350–550 °C), but face the problem of CH4 generation and low capacity of CO2 capture.
CaO-based sorbents are the more commonly used adsorption components of bifunctional catalysts in ICCU-RWGS reactions due to their low price, high theoretical CO2 uptake capacity, and wide availability.33 Additionally, the high decomposition temperature of CaCO3 matches the endothermic nature of the RWGS reaction.9 Jo et al. showed that a Ni–CaO bifunctional catalyst had a CO2 adsorption capacity of up to 14.8 mmol CO2/g and showed very good ICCU-RWGS performance with a CO yield of 11.0 mmol CO/g at 700 °C.34 Zhao et al. prepared a Ni–CaO bifunctional catalyst derived from carbide slag, and it exhibited a CO2 capture capacity of 13.28 mmol g−1 and a CO productivity of 5.12 mmol g−1 at 650 °C.21 Currently, the main challenge faced by Ni–CaO bifunctional catalysts in ICCU-RWGS is that the CO2 adsorption capacity and CO yield decrease rapidly with the number of cycles at high temperatures (>650 °C). This is because the operating temperature of the conversion stage (>650 °C) is higher than the Tammann temperature of CaCO3 (530 °C), which easily leads to the significant sintering-induced deactivation of CaO and thus the decease of CO2 adsorption capacity.35 On the other hand, the Ni species also suffer from obvious particle agglomeration, leading to the loss of active sites.33
2.1.3. Highly stable bifunctional catalysts for ICCU-RWGS.
Doping inactive stabilizers is deemed as the effective strategy to inhibit the deactivation of Ni–CaO bifunctional catalysts.23,24,33 Wu et al. investigated the influence of different stabilizers (Al2O3, CeO2, TiO2, and ZrO2) on Ni–CaO.23 The ICCU-RWGS performance of these samples showed the following order: Ni/Al2O3–CaO < Ni/ZrO2–CaO < Ni/TiO2–CaO < Ni/CeO2–CaO. 56.1% conversion of CO2 and nearly 100% CO selectivity with a 2.68 mmol g−1 yield of CO were achieved on Ni/CeO2–CaO at 650 °C. Furthermore, after 20 cycles, the decrease in CO and C1 yields for Ni/CeO2–CaO was less than 5%, with a CO selectivity greater than 99%. The superior ICCU-RWGS activity of Ni/CeO2–CaO can be attributed to its stronger basicity, optimal Ni dispersion and improved reducibility of NiO. It was reported that the oxygen vacancies generated on the surface of CeO2 could directly dissociate CO2, thereby improving CO2 conversion and CO selectivity.36 Furthermore, well-dispersed CeO2 can also act as a physical barrier to effectively inhibit the agglomeration of CaO and NiO species, ensuring the stability of the Ni/CeO2–CaO catalyst. Zhao et al. showed that the concentration of oxygen vacancies in the bifunctional catalyst could be further boosted by doping ZrO2–CeO2 binary metal oxides.33 The desired Ni/CaO–6ZrO2–6CeO2 promoted the activation and conversion of CO2 to produce CO. Wang et al. also showed that metal oxide (MgO, ZrO2, La2O3, and CeO2) modified Ni/CaO bifunctional catalysts significantly improved the cyclic stability compared with Ni/CaO.24 Ni/La2O3–CaO exhibited the best cyclic stability with a slight decrease of adsorption capacity from 13.4 mmol g−1 in the 1st cycle to 12.9 mmol g−1 in the 10th cycle, suggesting that La2O3 can also serve as an effective physical barrier to suppress the growth of CaO and Ni particles. Recently, Xu et al. showed that Na incorporation had a positive effect on the stability of Ni–CaO bifunctional catalysts.37
The preparation method for Ni–CaO bifunctional catalysts can also improve their stability. Zhao et al. compared three methods for the synthesis of Ni–CaO: acidification/impregnation, acidification/impregnation combined with citric acid complexation, and wet-mixing. The results showed that the Ni–CaO synthesized by the second method had stable CO2 adsorption capacity and CO yields for 17 cycles.21 They ascribed this to a more uniform dispersion of components accompanied by smaller particles and better textural properties. Wang et al. synthesized a three-dimensional (3D) Ni/CaO network composed of mesopores and macropores as a bifunctional catalyst,38 which could preserve the stabilization of the structure and inhibit the growth of CaO particles. A CO2 adsorption capacity of 9.7 mmol g−1 with 57.5% conversion of CO2 was achieved and maintained for 20 cycles on the 3D Ni/CaO.
Ca–Fe bifunctional catalysts have also been used in the ICCU-RWGS reaction by combining the Fe/Fe3O4 redox cycle and calcium looping. Unlike Ni–CaO bifunctional catalysts, Ca–Fe can produce CO during both capture and conversion stages (Fig. 4), which is ascribed to the re-oxidation of Fe by CO2 and the RWGS reaction, respectively.39 The CO yield in the conversion stage was significantly smaller than that in the capture stage (1.80 molCO kgCaO−1vs. 142.95 molCO kgFe2O3−1) at 650 °C,39 due to the high re-oxidation rate of Fe and the poor catalytic activity of Fe3O4 or Fe for the RWGS reaction. By doping Ni into the Ca–Fe material, Ni1Fe9–CaO showed a superior performance with a CO yield of 11.3 mol kg−1, a CO2 conversion of 82.5% and a CO selectivity of 99.9% at 650 °C.40 Hu et al. reported FexCoyMg10CaO as a heterojunction-redox catalyst for the ICCU-RWGS reaction, and the best sample exhibited a stable CO2 adsorption capacity of 9.0–9.2 mmol g−1, nearly 90% conversion of CO2 and almost 100% selectivity to CO.9 The sintering of CaO was suppressed by the hybrid doping of highly dispersed Co and Fe oxides and MgO. Furthermore, they demonstrated the stable scalability of the ICCU-RWGS process through scale-up studies with experiments and computer simulations.
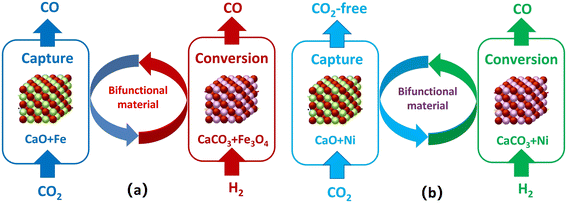 |
| Fig. 4 Process diagrams of (a) Ca–Fe and (b) Ca–Ni bifunctional catalysts in the ICCU-RWGS reaction.39 | |
2.2. Bifunctional catalysts for ICCU-methanation
The CO2 methanation reaction is thermodynamically favorable at low temperatures due to its exothermic nature.41,42 However, low reaction temperatures will lead to severe kinetic limitations.8 On the other hand, if the temperature of the methanation reaction is high (>400 °C), the RWGS will occur, so the selectivity to CH4 at high temperatures may face challenges. Therefore, the ICCU-methanation reaction is often operated at intermediate temperatures (200–400 °C). Noble metals (Rh and Ru) and Ni have been extensively employed as catalytic components in the ICCU-methanation due to their high CO2 methanation activity.43,44 As for adsorbents, alkaline earth (Mg, Ba, and Ca) or alkali (K, Na, and Li) metals are usually used due to their capture temperature of 300 to 400 °C.45–47 Additionally, the high specific surface area of the support (γ-Al2O3) is needed to maximize adsorption sites and disperse catalyst particles.48
2.2.1. Alkali metal-based bifunctional catalysts for ICCU-methanation.
Ru–Na2CO3/γ-Al2O3 is one of the most widely studied bifunctional catalysts for ICCU-methanation. Duyar and co-workers investigated the isothermal ICCU-methanation process by using a 5%Ru–10%Na2CO3/γ-Al2O3 bifunctional catalyst at 320 °C, and a methanation capacity of 1.05 mol kg−1 was achieved.49 Later on, Farrauto et al. studied the effect of cyclic aging on the ICCU-methanation with 5%Ru–6.1%Na2O/γ-Al2O3 (“Na2O” derived from hydrogenated Na2CO3) at 320 °C.50 The result showed the stable performance of ICCU-methanation for over 50 cycles (equivalent to 80 h of operation). Cobo et al. conducted a mechanism study of ICCU-methanation on 5%Ru–6.1%Na2O/γ-Al2O3 by in situ diffuse reflectance infrared Fourier transform spectroscopy (DRIFTS).51 CO2 was first adsorbed on the Al–O−–Na+ species to form bidentate carbonates, which then overflowed to the Ru–support interface for methanation with formates as intermediaries. In the work of González-Velasco,52 Na2O and NaOH were identified as storage sites for CO2, with Na2O being more active in CO2 adsorption. To achieve high methanation activity, the content of Ru in bifunctional catalysts is usually high (>5%). A promising way to reduce the Ru content while maintaining the high catalytic activity of bifunctional catalysts is to replace some of the Ru with Ni.53–55 González-Velasco et al. showed that 1%Ru/10Ni%–16%Na2CO3/γ-Al2O3 presented a selectivity of more than 95% for CH4 in the temperature range of 320 °C to 400 °C, and a CH4 yield of 137 μmol g−1 with a fast formation rate was achieved at 360 °C.53 Jeong-Potter et al. further explored the effect of Ni loading (1%, 5%, 10%, 20%) on Ru–Na2O/γ-Al2O3.54 Among them, 0.5%Ru/5Ni%–6.1%Na2O/γ-Al2O3 had the highest CO2 adsorption capacity while 0.5%Ru/20Ni%–6.1%Na2O/γ-Al2O3 was the most stable. Ni–Na2O/γ-Al2O3 has also been used in the ICCU-methanation reaction.55,56 González-Velasco et al. showed that the highest CH4 productivity of 177 μmol g−1 and 90% selectivity to CH4 were achieved on 10%Ni–10%Na2CO3/γ-Al2O3.56 The Ni-alone bifunctional catalyst is often used in an O2-free environment, and is not suitable for O2-containing applications (flue gas or air) as it oxidizes and cannot be reactivated under methanation conditions (300–400 °C).54,55,57 In contrast, Ru-based bifunctional catalysts exhibit good stability in an O2-containing environment because of the high reducibility of RuOx species (Fig. 5).
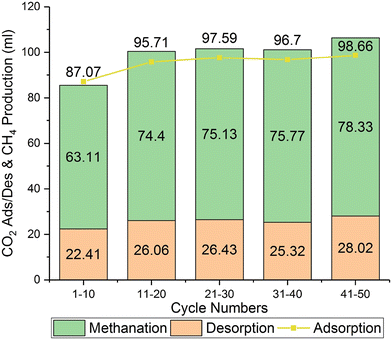 |
| Fig. 5 Cyclic aging study on 5%Ru–6.1%Na2O/γ-Al2O3.50 | |
Other alkali metals (K and Li) are also used in ICCU-methanation as sorbent components of bifunctional catalysts. Farrauto et al. demonstrated that a CO2 sorbent capacity of 496.4 mmol kg−1 and a CH4 yield of 466.9 mmol kg−1 were achieved at 320 °C on 5%Ru–7.01%K2O/γ-Al2O3.58 Cimino et al. reported a 0.50 mmol g−1 yield of CH4 with a CH4 selectivity of 99.9% on 0.89%Ru–5%Li/γ-Al2O3 at 280 °C.59 The formed Li-aluminate captures and stores CO2 at moderate temperatures (230–350 °C). They further studied the effect of alkali metals (K, Na, Li) on the ICCU-methanation performance of 1%Ru/γ-Al2O3.46 The CO2 capture capacity was in the order Ru–Li/γ-Al2O3 > Ru–Na/γ-Al2O3 ≥ Ru–K/γ-Al2O3. Meanwhile, Li–Ru/γ-Al2O3 displayed the highest methanation activity. However, in another study, it was found that the Ru–Li/γ-Al2O3 bifunctional catalyst just showed a similar performance to Ru–Li/γ-Al2O3, which was worse than Ru–K/γ-Al2O3 and Ru–Na/γ-Al2O3.47
2.2.2. MgO-based bifunctional catalysts for ICCU-methanation.
MgO or MgO-based materials are good CO2 adsorbent components of bifunctional catalysts in ICCU-methanation processes since the reversible transformation of MgO–MgCO3 occurs at 300 °C–400 °C.60 Sun et al. reported that nearly 100% capture of CO2 with the successful production of CH4 was achieved in prolonged cycles at low temperatures (≤250 °C) using 2D-layered Ni–MgO–Al2O3 nanosheets as a bifunctional catalyst.61 However, the CO2 adsorption capacity and CH4 yield of MgO-based bifunctional catalysts are usually limited due to the high lattice enthalpy of MgO.62 The introduction of alkaline metal salts (AMS) has been conducted to appreciably improve the slow adsorption rate and cycle stability of MgO-based bifunctional catalysts.62–64 Zhao et al. showed that NaNO3-promoted Ni/MgO exhibited good ICCU-methanation performance with a high CO2 adsorption capacity (6.46 mmol g−1) and CH4 yield of 0.85 mmol g−1 at 300 °C.62 Gilliard-AbdulAziz et al. constructed a Ru/K2CO3–MgO bifunctional catalyst, and it achieved a stable CH4 yield of 1.07–1.19 mmol g−1 and 100% selectivity to CH4 at the carbonation temperature of 150 °C and methanation temperature of 320 °C.63 In addition, they found that the Ru crystallite size in Ru/K2CO3–MgO was smaller than that in Ru/MgO due to the presence of the K2RuO3 phase. Hu et al. developed a NixCoy-AMS/CaMgO bifunctional catalyst by loading alkaline metal salts (AMS) on porous CaMgO composites, and the AMS were composed of KNO3 (0.28 mmol), LiNO3 (0.22 mmol), Na2CO3 (0.125 mmol), and K2CO3 (0.125 mmol).64 NixCoy-AMS/CaMgO had excellent ICCU-methanation performance with 93.4% CO2 conversion and 88% CH4 selectivity at 350 °C.
2.2.3. CaO-based bifunctional catalysts for ICCU-methanation.
As the pioneer of ICCU-methanation, Farrauto's group reported one of the earliest CaO-based bifunctional catalysts.65 They constructed Ru–CaO/γ-Al2O3 bifunctional catalysts by dispersing CaO and Ru on porous γ-Al2O3 and studied the effect of different weight loadings of the CaO adsorbent (1–10 wt%) and Ru catalyst (1.1–10.6 wt%) on the ICCU-methanation performance. The results indicated that 5%Ru–10%CaO/γ-Al2O3 as the optimum composition realized a CH4 yield of 0.50 mol kg−1 at 320 °C. They further showed that a higher CH4 yield of 0.70 mol kg−1 was achieved on 5Rh–10%CaO/γ-Al2O3, suggesting that Rh–CaO-based bifunctional catalysts were more active than those containing Ru and CaO.49 However, the high cost of Rh limited their use. González-Velasco et al. thought that high reaction temperatures were required for CaO-based bifunctional catalysts because of the formation of highly stable carbonates on CaO.52 They conducted the ICCU-methanation reaction at a temperature of 400 °C on 4%Ru–15%CaO/γ-Al2O3 and achieved a maximum production of 414 μmol g−1 for CH4. At high operating temperatures, the support in bifunctional catalysts can be eliminated, thus increasing the CaO content and making full use of the CaO sorbent capacity. Kim et al. reported an excellent CO2 uptake (8.96 mmol g−1) and CH4 yield (8.34 mmol g−1) with 96% CH4 selectivity at 500 °C using 10%Ni–CaO.66 Later on, they studied the effect of H2 concentration and reaction temperature on ICCU-methanation over 10Ni–CaO.34 The intermediate temperature (500–600 °C) and high H2 concentration were conducive to the formation of CH4. Wu et al. studied the effect of Ni loading on the ICCU-methanation performance of Ni–CaO.22 They found that 1%Ni–CaO at 550 °C exhibited a low CO2 conversion of 38% and 58% selectivity for CH4. However, when the Ni loading was increased to 10%, the CO2 conversion and CH4 selectivity improved to 45% and 69%, respectively. The lower methanation activity in the former case was attributed to volume expansion caused by the carbonation process, which led to Ni active sites being covered by formed CaCO3. Zhou et al. investigated the effect of doping a series of metal oxides (MgO, MnO2, La2O3, Y2O3, Al2O3, ZrO2, and CeO2) on 10Ni–CaO.18 Thanks to the good thermal stability at 600 °C, 10Ni–ZrO2/CaO showed the best ICCU-methanation performance in 20 cycles. They proposed a two-step mechanism (Fig. 6) in which CO2 was first released from CaCO3 decomposition, followed by its conversion to CO at CaO sites and to CH4 at Ni sites.
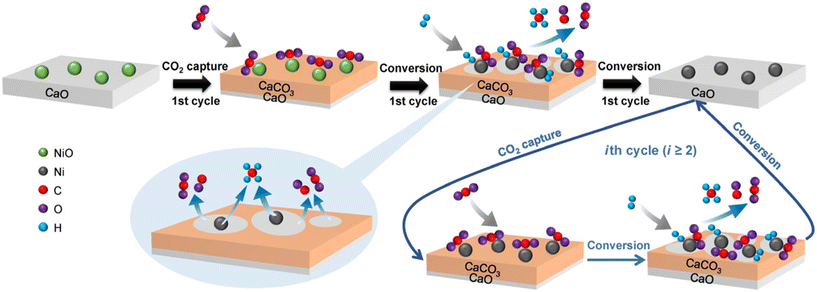 |
| Fig. 6 Illustration of the ICCU-methanation over Ni–CaO bifunctional catalysts.18 | |
3. Bifunctional catalysts for integrated CO2 capture and dry reforming reactions
Dry reforming of CH4 simultaneously utilizes two major greenhouse gases to produce syngas, thus receiving wide attention. The reaction can combine with CO2 capture to realize the ICCU-DRM. Other light alkanes (C2H6 and C3H8) have also been used as reduction gases in the ICCU process to achieve the integrated CO2 capture and dry reforming of alkane (ICCU-DRA). In addition to the production of syngas, the ICCU-DRA process can also produce light olefins (C2H4 and C3H6),67,68 which is appealing to chemical industry. The ICCU-DR reaction process requires high temperatures (>600 °C) due to the highly endothermic nature of DR reactions.14,69 CaO or CaO-based materials as high-temperature adsorbents have been used in bifunctional catalysts for the ICCU-DR reaction. On the other hand, other high-temperature adsorbents (sodium zirconate or lithium silicate) have also been studied.70 | CH4 + CO2 → 2H2 + 2CO ΔH298K = 247 kJ mol−1 | (3) |
| 2CO2 + C2H6 → 4CO + 2H2 ΔH298K = 429 kJ mol−1 | (4) |
| CO2 + C2H6 → CO + C2H4 + H2O ΔH298K = 178 kJ mol−1 | (5) |
| 3CO2 + C3H8 → 6CO + 4H2 ΔH298K = 620 kJ mol−1 | (6) |
| CO2 + C3H8 → CO + C3H6 + H2O ΔH298K = 164 kJ mol−1 | (7) |
3.1. Bifunctional catalysts for ICCU-DRM
Heriberto Pfeiffer et al. studied the decomposition of carbonated samples in the CH4 atmosphere on a NiO–CaO bifunctional catalyst and reported that syngas was produced by the CH4 reforming process between 400 and 600 °C.71 Unfortunately, they only showed the H2 production. Tian et al. constructed Ni–CaO bifunctional catalysts by the Pechini sol–gel method and conducted the ICCU-DRM reaction at a CO2 capture temperature of 600 °C and a dry reforming temperature of 800 °C.16 An average cyclic utilization efficiency of 65% for CO2 was obtained on the optimized Ni–CaO bifunctional catalyst with 86% average cyclic conversion for CH4 (Fig. 7). Meanwhile, a maximum syngas yield of 10.4 mmol g−1 for a H2/CO molar ratio of 1.1 was reported. More importantly, they indicated that the ICCU-DRM process consumed 22% less energy than the conventional DRM reaction. Huang et al. investigated the synergistic effect of CaO and Ni at the interface (Fig. 8) and proposed that the Ni–CaO interface provided another pathway for CO2 activation and reduced the energy barrier for CHO formation, thus ensuring long-term stability and high efficiency of the catalyst.11 Hu et al. suggested that controlling the adsorptive/catalytic interface by balancing the size and loading of Ni nanoparticles on porous CaO was key to achieving ultra-high CH4 and CO2 conversions of 96.0% and 96.5%, respectively, at 650 °C.72
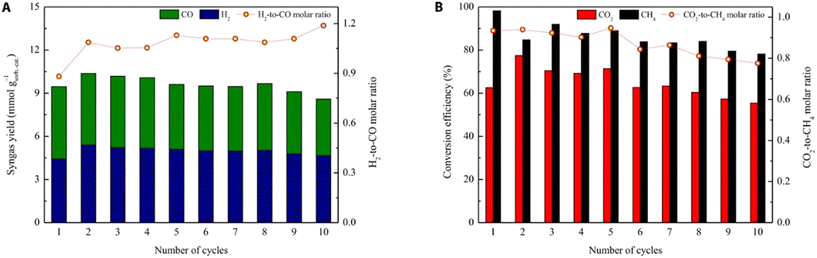 |
| Fig. 7 The performance of the ICCU-DRM reaction for the Ni–CaO bifunctional catalyst. (A) Syngas yield and the molar ratio of H2 to CO and (B) CO2 and CH4 conversion with the molar ratio of their consumption.16 | |
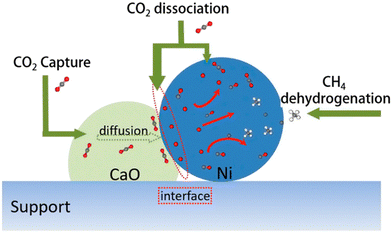 |
| Fig. 8 Schematic diagram of the reaction mechanism of ICCU-DRM on the Ni–CaO catalyst.11 | |
Sintering of active metals and carbon deposition at high temperature often lead to the deactivation of bifunctional catalysts, which limits the application of the ICCU-DRM process. Kawi et al. synthesized a porous CeO2-modified CaO microsphere loaded with Ni as a bifunctional catalyst.73 The optimized 5Ni–CeO2/CaO with an 85
:
15 molar ratio of Ca/Ce showed constant CO2 uptake while retaining ∼80–90% of its initial syngas yield over 9 cycles at 650 °C. The introduction of CeO2 as a promoter enhanced the CaO affinity to CO2 and the reducibility of Ni, thereby significantly improving the low-temperature activity for DRM at 650 °C. Additionally, CeO2 can also be used as a structure stabilizer to improve the sintering resistance of Ni and CaO particles, thus maintaining the stability of the ICCU-DRM reaction. Later on, they prepared a novel Ni–CaO-based bifunctional catalyst by co-loading CeO2 and Ni particles onto ZrO2-coated CaCO3.74 The ZrO2 layer could stabilize Ni and CaO particles to maintain catalytic activity and CO2 capture capacity. The addition of CeO2 can eliminate carbon deposition and activate CH4 and CO2. Recently, they synthesized a mesoporous Ni–CaO bifunctional catalyst by pre-doping trace amounts of Ni into the CaO matrix, which realized the tandem distribution of Ni in the CaO framework.75 The ICCU-DRM performance indicated that the obtained Ni/NiCa had 38% higher CO2 adsorption capacity and 35% higher CO2 and CH4 conversions than Ni/Ca. Meanwhile, higher stability was also observed on the Ni/NiCa bifunctional catalyst.
Mendoza-Nieto et al. applied alkaline zirconates (Li2ZrO3 and Na2ZrO3) as bifunctional catalysts to the ICCU-DRM process.76 They found that Na2ZrO3 had the higher CO2 capture. H2 was produced via the DRM reaction at 450–750 °C and the partial oxidation of methane (POM) above 750 °C. In another report, they further studied the NiO doping on Na2ZrO3 for the ICCU-DRM reaction.70 It was shown that the addition of NiO increased the H2 production. Moreover, a sharp decrease in reaction temperatures was observed for the NiO-doped Na2ZrO3. Although sodium zirconate or lithium silicate materials can be used as bifunctional catalysts in ICCU-DRM, their poor adsorption capacity will limit their application compared to high capacity CaO-based materials.
3.2. Bifunctional catalysts for ICCU-DRA
Ethane (C2H6) and propane (C3H8) are also used as reactants in the ICCU-DR process. Rezaei et al. prepared γ-Al2O3 supported Ni-impregnated CaO and MgO as bifunctional catalysts and successfully applied them in integrated CO2 capture and DR of ethane (ICCU-DRE) to produce syngas.77 The performance results indicated that the adsorbed CO2 could be released completely. And the best performing sample exhibited 100% conversion of C2H6 and 65% conversion of CO2 at 650 °C. Meanwhile, the H2/CO ratio of the obtained syngas was 1.30, meeting the requirement for major chemical production processes. They further synthesized K–Ca and Cr-impregnated H-ZSM-5 bifunctional catalysts for the production of C2H4 by the ICCU-DRE process.78 The performance experiments were conducted under adsorption conditions of 600 °C and 10% CO2/Ar and reaction conditions of 700 °C and 5% C2H6/Ar. On the optimal bifunctional catalyst, the CO2 adsorption capacity was 5.2 mmol g−1, the conversion of C2H6 was 25%, and the selectivity to C2H4 was 88%, respectively. Recently, they proposed 3D-printed metal-oxide-CaO/ZSM-5 (V, Ga, Ni, and Ti) structured monoliths as bifunctional catalysts.67 V–CaO/ZSM-5 as the best sample achieved a high CO2 capture of 5.4 mmol g−1. Meanwhile, 36.5% C2H6 conversion, 65.2% CO2 conversion, and 98% C2H4 selectivity with a 35.8% yield of C2H4 were also shown.
The CO2 capture process can also be coupled with the dry reforming of propane reaction (ICCU-DRP).68,79 Hu et al. conducted the study of ICCU-DRP on Pt/ZrO2–5CaO to produce syngas.68 The result showed that the CO2 adsorption capacity of Pt/ZrO2–5CaO was 10.3 mmol g−1, which could be completely converted to CO at 600 °C. Meanwhile, they found that 77% of C3H6 was reformed with CO2, and 23% of C3H8 underwent dehydrogenation. The high dissociation activity of Pt to C–H and C–C bonds might be the reason responsible for the low productivity of C3H6. Rezaei et al. reported that C3H6 could be produced from the ICCU-DRP process with 3D-printed CaO/M@ZSM-5 (M = Ni-, Ti-, Ga-, or V-oxide) monoliths as bifunctional catalysts.79 Among them, 3D-printed CaO/Ti@ZSM-5 achieved 76% conversion of CO2 and 39% selectivity to C3H6. Besides alkanes, Yu et al. demonstrated that an ethanol and glycerol mixture could be used as a hydrogen donor in the ICCU-DR reaction.80 Nearly 100% conversion of the ethanol and glycerol mixture and 92% syngas selectivity with a 1.2 ratio of H2/CO were obtained on the 10Ni/CaCO3 bifunctional catalyst.
4. Bifunctional catalysts for integrated SESR and CO2 conversion
Although the mixture of CO2 and N2 is often used for CO2 adsorption in ICCU processes, there is also ongoing research on the effects of O2 and H2O by studying simulated flue gas and air. The ICCU can be further combined with other CO2-producing processes, such as the sorption-enhanced steam reforming (SESR) reaction.15,81 The SESR reaction can produce high-purity hydrogen (>96%) through a single-step integration of SR, WGS and in situ CO2 capture.82,83 To continuously produce hydrogen by SESR, a decarbonation step must be performed to regenerate the adsorbent (usually CaO) or bifunctional catalyst. The decarbonation reaction typically occurs at high temperatures (>700 °C) in an inert atmosphere to decompose carbonates and release CO2, resulting in the dilution of captured CO2 by carrier gas, which poses challenges for further CO2 utilization. The integrated SESR reaction and CO2 utilization (ICCU-SESR) offers a promising solution. Yu et al. demonstrated the technical feasibility of the coupled process between SESR of glycerol (SESRG) and dry reforming of methane (ICCU-SESRG, Fig. 9) with Ni–CaO–Ca12Al14O33 as a bifunctional catalyst.14 98.53% high-purity H2 was produced during SESRG at 550 °C, and 95.3% conversion of CH4 and syngas with a H2/CO molar ratio of less than 2 were achieved during DRM at 700 °C. Meanwhile, the ICCU-SESRG process was stable over ten cycles. Later on, they reported that the temperature of DRM could be lowered to 650 °C by increasing the decomposition activity of CH4 over a Pd-promoted Ni–Ca–Al bifunctional catalyst in the ICCU-SESRG process,4 resulting in co-production of a hydrogen-enriched stream (98.3%) and syngas with a H2/CO molar ratio less than <3. Meanwhile, the sorption-enhanced effect only decreased by 25% after ten cycles due to the lower regeneration temperature. Wu et al. reported that CO2 could be simultaneously captured by CaO in the production of syngas from lignin gasification.5 And these captured CO2 can be effectively converted into CO to achieve zero CO2 emissions.
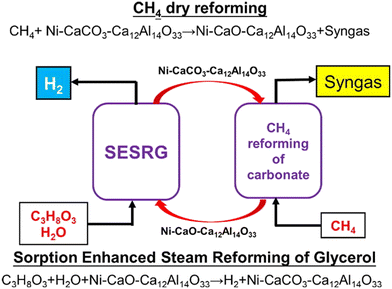 |
| Fig. 9 Schematic diagram of the ICCU-SESRG process.14 | |
5. Outlook
Bifunctional catalysts play a crucial role in ICCU processes, determining the unique performance advantages and system efficiency. Therefore, great efforts have been made to develop bifunctional catalysts. The ICCU-methanation reaction is often operated at intermediate temperatures (200–400 °C) to balance the kinetics and endothermic nature of the methanation reaction. Alkali earth metals (Na, K and Li) and MgO, as adsorbent components of bifunctional catalysts, are more suitable for the ICCU-methanation process because of the CO2 adsorption properties at low and medium temperatures. The main problems faced by these bifunctional catalysts are their low adsorption rate and cycling stability. Dispersing them on supports with high specific surface areas and porous structures is currently the main optimization method, but it is necessary to pay attention to the compromise between the carrier and the amount of adsorbent. In addition, it may be more potential to realize thin-layer bifunctional catalyst by optimizing the preparation method. CaO-based bifunctional catalysts can also be used in ICCU-methanation due to their excellent CO2 adsorption capacity. However, the high stability of CaCO3 requires that the ICCU-methanation reaction is operated at high temperatures (>550 °C) to achieve appropriate kinetics, which leads to the occurrence of RWGS, so the selectivity to CH4 is challenged. The key to the optimization of CaO-based bifunctional catalysts is to achieve fast kinetics and high CH4 selectivity of the ICCU-methanation reaction by improving the methanation performance of bifunctional catalysts at low temperatures.
The ICCU-RWGS process is usually operated at high temperatures due to the endothermic nature of the RWGS reaction, and CaO-based bifunctional catalysts are most commonly used in this process. Although the ICCU-RWGS reaction can be realized over transition-metal-free bifunctional catalysts with high selectivity to CO, the high conversion of captured CO2 is difficult to achieve because of the rapid decomposition of CaCO3 and the limited kinetics of CO formation at high temperatures (>650 °C). Transition-metal-containing bifunctional catalysts, especially Ni–CaO, provide more choices to realize high CO selectivity and CO2 conversion by lowering the reaction temperature (500–600 °C). On the other hand, the low temperature can also inhibit the deactivation caused by sintering at high temperatures, which is another key problem of CaO-based bifunctional catalysts for ICCU-RWGS. Therefore, the ICCU-RWGS process focuses on developing bifunctional catalysts that inhibit CH4 by-products at low temperatures to simultaneously achieve high CO2 conversion, CO selectivity and stability.
ICCU-DRA is a more attractive and potential process because it can produce high value-added products, especially C2H4 and C3H6. CaO-based materials are the most commonly used bifunctional catalysts in ICCU-DRA processes due to the highly endothermic nature of dry reforming reactions requiring high operating temperatures. Compared with ICCU-methanation and ICCU-RWGS, ICCU-DRA is a more complex process with side reactions, so the selectivity to the product needs to be regulated. Additionally, carbon deposits also need to be considered. The work of bifunctional catalysts for ICCU-DRA is mainly focused on the optimization of catalytic performance by adjusting the active metal components. To enable the ICCU-DRA process, three major issues of bifunctional catalysts remain to be solved: (1) high selectivity to products, especially the co-production of lower olefins; (2) high stability, including the resistance to sintering and carbon deposition; (3) isothermal operation from CO2 capture to conversion. Meanwhile, further studies can be conducted to couple different sources of CO2 such as flue gas, air and the sorption-enhanced steam reforming (SESR) reaction in the ICCU process.
Data availability
No primary research results, software or code have been included and no new data were generated or analysed as part of this review.
Conflicts of interest
There are no conflicts to declare.
Acknowledgements
This work was supported by the National Natural Science Foundation of China (No. 22078106), the Natural Science Foundation of Guangdong Province (2024B1515040016, 2024A1515030255) and the Guizhou Provincial Science and Technology Plan Project Natural Science Key Project (ZK[2022] 028).
References
- C. Dang, Y. Li, S. M. Yusuf, Y. Cao, H. Wang, H. Yu, F. Peng and F. Li, Calcium cobaltate: a phase-change catalyst for stable hydrogen production from bio-glycerol, Energy Environ. Sci., 2018, 11, 660–668 RSC.
- M. Mathis, F. Lacroix, S. Hagemann, D. M. Nielsen, T. Ilyina and C. Schrum, Enhanced CO2 uptake of the coastal ocean is dominated by biological carbon fixation, Nat. Clim. Change, 2024, 14, 373–379 CrossRef CAS.
- C. Zhu, K. Kobayashi, I. Loladze, J. Zhu, Q. Jiang, X. Xu, G. Liu, S. Seneweera, K. L. Ebi, A. Drewnowski, N. K. Fukagawa and L. H. Ziska, Carbon dioxide (CO2) levels this century will alter the protein, micronutrients, and vitamin content of rice grains with potential health consequences for the poorest rice-dependent countries, Sci. Adv., 2018, 4, eaaq1012 CrossRef.
- C. Dang, J. Long, H. Li, W. Cai and H. Yu, Pd-promoted Ni-Ca-Al bi-functional catalyst for integrated sorption-enhanced steam reforming of glycerol and methane reforming of carbonate, Chem. Eng. Sci., 2021, 230, 116226 CrossRef CAS.
- Y. Zhu, B. Li, J. Miao, S. Sun, Y. Wang, X. Zhao, B. Chen and C. Wu, Achieving zero CO2 emissions from integrated biomass gasification with CO2 capture and utilization (IGCCU), Chem. Eng. J., 2023, 474, 145767 CrossRef CAS.
- H. Mansour and E. Iglesia, Mechanistic Connections between CO2 and CO Hydrogenation on Dispersed Ruthenium Nanoparticles, J. Am. Chem. Soc., 2021, 143, 11582–11594 CrossRef CAS PubMed.
- H. Xia, C. Dang, D. Zhou and W. Cai, Lamellar cross-linking Ni/CeO2 as an efficient and durable catalyst for dry reforming of methane, Chem. Eng. J., 2024, 489, 151365 CrossRef CAS.
- B. Shao, Y. Zhang, Z. Sun, J. Li, Z. Gao, Z. Xie, J. Hu and H. Liu, CO2 capture and in-situ conversion: recent progresses and perspectives, Green, Chem. Eng., 2022, 3, 189–198 Search PubMed.
- B. Shao, G. Hu, K. A. M. Alkebsi, G. Ye, X. Lin, W. Du, J. Hu, M. Wang, H. Liu and F. Qian, Heterojunction-redox catalysts of FexCoyMg10CaO for high-temperature CO2 capture and in situ conversion in the context of green manufacturing, Energy Environ. Sci., 2021, 14, 2291–2301 RSC.
- X. Ma, H. Cui, Z. Cheng and Z. Zhou, Application of engineered natural ores to intermediate- and high-temperature CO2 capture and conversion, AIChE J., 2023, 69, e18146 CrossRef CAS.
- P. Wu, Y. Tao, H. Ling, Z. Chen, J. Ding, X. Zeng, X. Liao, C. Stampfl and J. Huang, Cooperation of Ni and CaO at Interface for CO2 Reforming of CH4: A Combined Theoretical and Experimental Study, ACS Catal., 2019, 9, 10060–10069 CrossRef CAS.
- S. Sun, Y. Zhang, C. Li, Y. Wang, C. Zhang, X. Zhao, H. Sun and C. Wu, Upgrading CO2 from simulated power plant flue gas via integrated CO2 capture and dry reforming of methane using Ni-CaO, Sep. Purif. Technol., 2023, 308, 122956 CrossRef CAS.
- C. Jeong-Potter and R. Farrauto, Feasibility Study of Combining Direct Air Capture of CO2 and Methanation at Isothermal Conditions with Dual Function Materials, Appl. Catal., B, 2021, 282, 119416 CrossRef CAS.
- C. Dang, S. Wu, Y. Cao, H. Wang, F. Peng and H. Yu, Co-production of high quality hydrogen and synthesis gas via sorption-enhanced steam reforming of glycerol coupled with methane reforming of carbonates, Chem. Eng. J., 2019, 360, 47–53 CrossRef CAS.
- B. Dou, K. Wu, H. Zhang, B. Chen, H. Chen and Y. Xu, Sorption-enhanced chemical looping steam reforming of glycerol with CO2 in-situ capture and utilization, Chem. Eng. J., 2023, 452, 139703 CrossRef CAS.
- S. Tian, F. Yan, Z. Zhang and J. Jiang, Calcium-looping reforming of methane realizes in situ CO2 utilization with improved energy efficiency, Sci. Adv., 2019, 5, eaav5077 CrossRef CAS.
- S. M. Kim, P. M. Abdala, M. Broda, D. Hosseini, C. Copéret and C. Müller, Integrated CO2 Capture and Conversion as an Efficient Process for Fuels from Greenhouse Gases, ACS Catal., 2018, 8, 2815–2823 CrossRef CAS.
- X. Ma, X. Li, H. Cui, W. Zhang, Z. Cheng and Z. Zhou, Metal oxide-doped Ni/CaO dual-function materials for integrated CO2 capture and conversion: Performance and mechanism, AIChE J., 2023, 69, e17520 CrossRef CAS.
- H. Li, C. Dang, G. Yang, Y. Cao, H. Wang, F. Peng and H. Yu, Bi-functional particles for integrated thermo-chemical processes: Catalysis and beyond, Particuology, 2021, 56, 10–32 CrossRef CAS.
- J. Zhou, C. Dang, G. Zheng and W. Cai, The capture and in-situ hydrogenation of CO2 over Ni-CaO-Ca12Al14O33 bifunctional catalyst, Sep. Purif. Technol., 2025, 354, 129375 CrossRef CAS.
- G. Wang, Y. Guo, J. Yu, F. Liu, J. Sun, X. Wang, T. Wang and C. Zhao, Ni-CaO dual function materials prepared by different synthetic modes for integrated CO2 capture and conversion, Chem. Eng. J., 2022, 428, 132110 CrossRef CAS.
- H. Sun, Y. Wang, S. Xu, A. I. Osman, G. Stenning, J. Han, S. Sun, D. Rooney, P. T. Williams, F. Wang and C. Wu, Understanding the interaction between active sites and sorbents during the integrated carbon capture and utilization process, Fuel, 2021, 286, 119308 CrossRef CAS.
- S. Sun, C. Zhang, S. Guan, S. Xu, P. T. Williams and C. Wu, Ni/support-CaO bifunctional combined materials for integrated CO2 capture and reverse water-gas shift reaction: Influence of different supports, Sep. Purif. Technol., 2022, 298, 121604 CrossRef CAS.
- Y. Hu, Q. Xu, X. Zou, X. Wang, H. Cheng, X. Zou and X. Lu, MxOy (M = Mg, Zr, La, Ce) modified Ni/CaO dual functional materials for combined CO2 capture and hydrogenation, Int. J. Hydrogen Energy, 2023, 48, 24871–24883 CrossRef CAS.
- D. Pinto, S. Minorello, Z. Zhou and A. Urakawa, Integrated CO2 capture and reduction catalysis: Role of γ-Al2O3 support, unique state of potassium and synergy with copper, J. Environ. Sci., 2024, 140, 113–122 CrossRef CAS.
- L. Li, S. Miyazaki, S. Yasumura, K. W. Ting, T. Toyao, Z. Maeno and K.-I. Shimizu, Continuous CO2 Capture and Selective Hydrogenation to CO over Na-Promoted Pt Nanoparticles on Al2O3, ACS Catal., 2022, 12, 2639–2650 CrossRef CAS.
- S. Sun, Z. Lv, Y. Qiao, C. Qin, S. Xu and C. Wu, Integrated CO2 capture and utilization with CaO-alone for high purity syngas production, Carbon Capture Sci. Technol., 2021, 1, 100001 CrossRef CAS.
- T. Sasayama, F. Kosaka, Y. Liu, T. Yamaguchi, S.-Y. Chen, T. Mochizuki, A. Urakawa and K. Kuramoto, Integrated CO2 capture and selective conversion to syngas using transition-metal-free Na/Al2O3 dual-function material, J. CO2 Util., 2022, 60, 102049 CrossRef CAS.
- S. Sun, Y. Wang, X. Zhao, C. Zhang and C. Wu, One step upcycling CO2 from flue gas into CO using natural stone in an integrated CO2 capture and utilisation system, Carbon Capture Sci. Technol., 2022, 5, 100078 CrossRef CAS.
- I. Wang, D. Li, S. Wang, Y. Wang, G. Lin, B. Yan and Z. Li, Limestone hydrogenation combined with reverse water-gas shift reaction under fluidized and iso-thermal conditions using MFB-TGA-MS, Chem. Eng. J., 2023, 472, 144822 CrossRef CAS.
- S. Sun, Z. Chen, Y. Xu, Y. Wang, Y. Zhang, C. Dejoie, S. Xu, X. Xu and C. Wu, Potassium-Promoted Limestone for Preferential Direct Hydrogenation of Carbonates in Integrated CO2 Capture and Utilization, JACS Au, 2024, 4, 72–79 CrossRef CAS.
- L. F. Bobadilla, J. M. Riesco-García, G. Penelás-Pérez and A. Urakawa, Enabling continuous capture and catalytic conversion of flue gas CO2 to syngas in one process, J. CO2 Util., 2016, 14, 106–111 CrossRef CAS.
- Y. Guo, G. Wang, J. Yu, P. Huang, J. Sun, R. Wang, T. Wang and C. Zhao, Tailoring the performance of Ni-CaO dual function materials for integrated CO2 capture and conversion by doping transition metal oxides, Sep. Purif. Technol., 2023, 305, 122455 CrossRef CAS.
- S. B. Jo, J. H. Woo, J. H. Lee, T. Y. Kim, H. I. Kang, S. C. Lee and J. C. Kim, CO2 green technologies in CO2 capture and direct utilization processes: methanation, reverse water-gas shift, and dry reforming of methane, Sustainable Energy Fuels, 2020, 4, 5543–5549 RSC.
- C. Dang, L. Liu, G. Yang, W. Cai, J. Long and H. Yu, Mg-promoted Ni-CaO microsphere as bi-functional catalyst for hydrogen production from sorption-enhanced steam reforming of glycerol, Chem. Eng. J., 2020, 383, 123204 CrossRef CAS.
- H. Sun, J. Wang, J. Zhao, B. Shen, J. Shi, J. Huang and C. Wu, Dual functional catalytic materials of Ni over Ce-modified CaO sorbents for integrated CO2 capture and conversion, Appl. Catal., B, 2019, 244, 63–75 CrossRef CAS.
- Y. Hu, Q. Xu, Y. Sheng, X. G. Wang, H. W. Cheng, X. L. Zou and X. G. Lu, The Effect of Alkali Metals (Li, Na, and K) on Ni/CaO Dual-Functional Materials for Integrated CO2 Capture and Hydrogenation, Materials, 2023, 16, 5430 CrossRef CAS PubMed.
- Y. Hu, Q. Xu, Y. Sheng, X. Wang, H. Cheng, X. Zou and X. Lu, Catalytic Synthesis of CO by Combining CO2 Capture and Hydrogenation over Three-Dimensional Ni/CaO Networks, Energy Fuels, 2023, 37, 7871–7880 CrossRef CAS.
- B. Jin, T. Ouyang, Z. Zhang, Y. Zhao, H. Zhang, W. Yao, G. Huang and Z. Liang, Prussian blue derived Ca-Fe bifunctional materials for chemical looping CO2 capture and in-situ conversion, Sep. Purif. Technol., 2023, 320, 123975 CrossRef CAS.
- S. Sun, S. He and C. Wu, Ni promoted Fe-CaO dual functional materials for calcium chemical dual looping, Chem. Eng. J., 2022, 441, 135752 CrossRef CAS.
- C. Dang, J. Zhou, H. Xia and W. Cai, A hierarchical hollow Ni/γ-Al2O3 catalyst derived from flower-like Ni-Al layered double hydroxide with stable catalytic performance for CO2 methanation, J. Mater. Chem. A, 2024, 12, 8281–8290 RSC.
- D. Zhang, H. Li, G. Yang, H.-F. Wang, Y. Cao, H. Wang and H. Yu, Hydrogen-driven routes to steel from siderite with low CO2 emissions: A modeling study, Chem. Eng. Sci., 2024, 287, 119702 CrossRef CAS.
- X. G. Zhang, A. Buthiyappan, J. Jewaratnam, H. S. C. Metselaar and A. A. A. Raman, Bifunctional materials for integrated CO2 capture and conversion:
review on adsorbent and catalyst types, recent advances, and challenges, J. Environ. Chem. Eng., 2024, 12, 111799 CrossRef CAS.
- I. S. Omodolor, H. O. Otor, J. A. Andonegui, B. J. Allen and A. C. Alba-Rubio, Dual-Function Materials for CO2 Capture and Conversion: A Review, Ind. Eng. Chem. Res., 2020, 59, 17612–17631 CrossRef CAS.
- E. García-Bordejé, A. Belén Dongil, J. M. Conesa, A. Guerrero-Ruiz and I. Rodríguez-Ramos, Dual functional materials based on Ni and different alkaline metals on alumina for the cyclic stepwise CO2 capture and methanation, Chem. Eng. J., 2023, 472, 144953 CrossRef.
- S. Cimino, F. Boccia and L. Lisi, Effect of alkali promoters (Li, Na, K) on the performance of Ru/Al2O3 catalysts for CO2 capture and hydrogenation to methane, J. CO2 Util., 2020, 37, 195–203 CrossRef CAS.
- A. Porta, R. Matarrese, C. G. Visconti, L. Castoldi and L. Lietti, Storage Material Effects on the Performance of Ru-Based CO2 Capture and Methanation Dual Functioning Materials, Ind. Eng. Chem. Res., 2021, 60, 6706–6718 CrossRef CAS.
- L.-P. Merkouri, T. R. Reina and M. S. Duyar, Closing the Carbon Cycle with Dual Function Materials, Energy Fuels, 2021, 35, 19859–19880 CrossRef CAS.
- M. S. Duyar, S. Wang, M. A. Arellano-Treviño and R. J. Farrauto, CO2 utilization with a novel dual function material (DFM) for capture and catalytic conversion to synthetic natural gas: An update, J. CO2 Util., 2016, 15, 65–71 CrossRef CAS.
- S. Wang, R. J. Farrauto, S. Karp, J. H. Jeon and E. T. Schrunk, Parametric, cyclic aging and characterization studies for CO2 capture from flue gas and catalytic conversion to synthetic natural gas using a dual functional material (DFM), J. CO2 Util., 2018, 27, 390–397 CrossRef CAS.
- L. Proaño, E. Tello, M. A. Arellano-Trevino, S. Wang, R. J. Farrauto and M. Cobo, In-situ DRIFTS study of two-step CO2 capture and catalytic methanation over Ru,“Na2O”/Al2O3 Dual Functional Material, Appl. Surf. Sci., 2019, 479, 25–30 CrossRef.
- A. Bermejo-López, B. Pereda-Ayo, J. A. González-Marcos and J. R. González-Velasco, Mechanism of the CO2 storage and in situ hydrogenation to CH4. Temperature and adsorbent loading effects over Ru-CaO/Al2O3 and Ru-Na2CO3/Al2O3 catalysts, Appl. Catal., B, 2019, 256, 117845 CrossRef.
- A. Bermejo-López, B. Pereda-Ayo, J. A. Onrubia-Calvo, J. A. González-Marcos and J. R. González-Velasco, Aging studies on dual function materials Ru/Ni-Na/Ca-Al2O3 for CO2 adsorption and hydrogenation to CH4, J. Environ. Chem. Eng., 2022, 10, 107951 CrossRef.
- C. Jeong-Potter, A. Zangiabadi and R. Farrauto, Extended aging of Ru-Ni, Na2O/Al2O3 dual function materials (DFM) for combined capture and subsequent catalytic methanation of CO2 from power plant flue gas, Fuel, 2022, 328, 125283 CrossRef CAS.
- L. Proaño, M. A. Arellano-Treviño, R. J. Farrauto, M. Figueredo, C. Jeong-Potter and M. Cobo, Mechanistic assessment of dual function materials, composed of Ru-Ni, Na2O/Al2O3 and Pt-Ni, Na2O/Al2O3, for CO2 capture and methanation by in-situ DRIFTS, Appl. Surf. Sci., 2020, 533, 147469 CrossRef.
- A. Bermejo-López, B. Pereda-Ayo, J. A. González-Marcos and J. R. González-Velasco, Alternate cycles of CO2 storage and in situ hydrogenation to CH4 on Ni-Na2CO3/Al2O3: influence of promoter addition and calcination temperature, Sustainable Energy Fuels, 2021, 5, 1194–1210 RSC.
- M. A. Arellano-Treviño, N. Kanani, C. W. Jeong-Potter and R. J. Farrauto, Bimetallic catalysts for CO2 capture and hydrogenation at simulated flue gas conditions, Chem. Eng. J., 2019, 375, 121953 CrossRef.
- M. A. Arellano-Treviño, Z. He, M. C. Libby and R. J. Farrauto, Catalysts and adsorbents for CO2 capture and conversion with dual function materials: Limitations of Ni-containing DFMs for flue gas applications, J. CO2 Util., 2019, 31, 143–151 CrossRef.
- S. Cimino, R. Russo and L. Lisi, Insights into the cyclic CO2 capture and catalytic methanation over highly performing Li-Ru/Al2O3 dual function materials, Chem. Eng. J., 2022, 428, 131275 CrossRef CAS.
- W. Gao, T. Zhou, Y. Gao, B. Louis, D. O'Hare and Q. Wang, Molten salts-modified MgO-based adsorbents for intermediate-temperature CO2 capture: A review, J. Energy Chem., 2017, 26, 830–838 CrossRef.
- Z. Zhou, N. Sun, B. Wang, Z. Han, S. Cao, D. Hu, T. Zhu, Q. Shen and W. Wei, 2D-Layered Ni-MgO-Al2O3 Nanosheets for Integrated Capture and Methanation of CO2, ChemSusChem, 2020, 13, 360–368 CrossRef CAS PubMed.
- P. Huang, J. Chu, J. Fu, J. Yu, S. Li, Y. Guo, C. Zhao and J. Liu, Influence of reduction conditions on the structure-activity relationships of NaNO3-promoted Ni/MgO dual function materials for integrated CO2 capture and methanation, Chem. Eng. J., 2023, 467, 143431 CrossRef CAS.
- S. Jo, H. D. Son, T.-Y. Kim, J. H. Woo, D. Y. Ryu, J. C. Kim, S. C. Lee and K. L. Gilliard-AbdulAziz, Ru/K2CO3-MgO catalytic sorbent for integrated CO2 capture and methanation at low temperatures, Chem. Eng. J., 2023, 469, 143772 CrossRef CAS.
- Z. Sun, B. Shao, Y. Zhang, Z. Gao, M. Wang, H. Liu and J. Hu, Integrated CO2 capture and methanation from the intermediate-temperature flue gas on dual functional hybrids of AMS/CaMgO||NixCoy, Sep. Purif. Technol., 2023, 307, 122680 CrossRef CAS.
- M. S. Duyar, M. A. A. Treviño and R. J. Farrauto, Dual function materials for CO2 capture and conversion using renewable H2, Appl. Catal., B, 2015, 168-169, 370–376 CrossRef CAS.
- S. B. Jo, J. H. Woo, J. H. Lee, T. Y. Kim, H. I. Kang, S. C. Lee and J. C. Kim, A novel integrated CO2 capture and direct methanation process using Ni/CaO catal-sorbents, Sustainable Energy Fuels, 2020, 4, 4679–4687 RSC.
- S. Lawson, K. Baamran, K. Newport, F. Rezaei and A. A. Rownaghi, Formulation and processing of dual functional Adsorbent/Catalyst structured monoliths using an additively manufactured contactor for direct Capture/Conversion of CO2 with cogeneration of ethylene, Chem. Eng. J., 2022, 431, 133224 CrossRef CAS.
- J. Dong, Y. Peng, J. Li, Z.-W. Liu and R. Hu, CO2 capture and conversion to syngas via dry reforming of C3H8 over a Pt/ZrO2-CaO catalyst, Catal. Sci. Technol., 2023, 13, 1650–1665 RSC.
- H. Li, C. Dang, Y. Li, G. Yang, Y. Cao, H. Wang, F. Peng and H. Yu, Pt-calcium cobaltate enables sorption-enhanced steam reforming of glycerol coupled with chemical-looping CH4 combustion, AIChE J., 2021, 67, e17383 CrossRef CAS.
- J. A. Mendoza-Nieto, S. Tehuacanero-Cuapa, J. Arenas-Alatorre and H. Pfeiffer, Nickel-doped sodium zirconate catalysts for carbon dioxide storage and hydrogen production through dry methane reforming process, Appl. Catal., B, 2018, 224, 80–87 CrossRef CAS.
- A. Cruz-Hernández, J. A. Mendoza-Nieto and H. Pfeiffer, NiO-CaO materials as promising catalysts for hydrogen production through carbon dioxide capture and subsequent dry methane reforming, J. Energy Chem., 2017, 26, 942–947 CrossRef.
- B. Shao, Z.-Q. Wang, X.-Q. Gong, H. Liu, F. Qian, P. Hu and J. Hu, Synergistic promotions between CO2 capture and in-situ conversion on Ni-CaO composite catalyst, Nat. Commun., 2023, 14, 996 CrossRef CAS PubMed.
- J. Hu, P. Hongmanorom, P. Chirawatkul and S. Kawi, Efficient integration of CO2 capture and conversion over a Ni supported CeO2-modified CaO microsphere at moderate temperature, Chem. Eng. J., 2021, 426, 130864 CrossRef CAS.
- J. Hu, P. Hongmanorom, V. V. Galvita, Z. Li and S. Kawi, Bifunctional Ni-Ca based material for integrated CO2 capture and conversion via calcium-looping dry reforming, Appl. Catal., B, 2021, 284, 119734 CrossRef CAS.
- J. Hu, P. Hongmanorom, J. Chen, W. Wei, P. Chirawatkul, V. V. Galvita and S. Kawi, Tandem distributing Ni into CaO framework for isothermal integration of CO2 capture and conversion, Chem. Eng. J., 2023, 452, 139460 CrossRef CAS.
- J. A. Mendoza-Nieto, Y. Duan and H. Pfeiffer, Alkaline zirconates as effective materials for hydrogen production through consecutive carbon dioxide capture and conversion in methane dry reforming, Appl. Catal., B, 2018, 238, 576–585 CrossRef CAS.
- A. Al-Mamoori, A. A. Rownaghi and F. Rezaei, Combined Capture and Utilization of CO2 for Syngas Production over Dual-Function Materials, ACS Sustainable Chem. Eng., 2018, 6, 13551–13561 CrossRef CAS.
- A. Al-Mamoori, S. Lawson, A. A. Rownaghi and F. Rezaei, Oxidative dehydrogenation of ethane to ethylene in an integrated CO2 capture-utilization process, Appl. Catal., B, 2020, 278, 119329 CrossRef CAS.
- S. Lawson, K. Baamran, K. Newport, T. Alghamadi, G. Jacobs, F. Rezaei and A. A. Rownaghi, Integrated direct air capture and oxidative dehydrogenation of propane with CO2 at isothermal conditions, Appl. Catal., B, 2022, 303, 120907 CrossRef CAS.
- C. Dang, S. Wu, G. Yang, Y. Cao, H. Wang, F. Peng and H. Yu, Syngas production by dry reforming of the mixture of glycerol and ethanol with CaCO3, J. Energy Chem., 2020, 43, 90–97 CrossRef.
- J. Zhou, C. Dang and W. Cai, High-purity hydrogen production from biogas by sorption-enhanced steam reforming over Ni-MgO-CaO bifunctional catalyst with paper fiber as template, Sep. Purif. Technol., 2024, 339, 126724 CrossRef CAS.
- C. Dang, H. Yu, H. Wang, F. Peng and Y. Yang, A bi-functional Co-CaO-Ca12Al14O33 catalyst for sorption-enhanced steam reforming of glycerol to high-purity hydrogen, Chem. Eng. J., 2016, 286, 329–338 CrossRef CAS.
- C. Dang, H. Wang, H. Yu and F. Peng, Co-Cu-CaO catalysts for high-purity hydrogen from sorption-enhanced steam reforming of glycerol, Appl. Catal., B, 2017, 533, 9–16 CrossRef CAS.
|
This journal is © The Royal Society of Chemistry 2025 |
Click here to see how this site uses Cookies. View our privacy policy here.