DOI:
10.1039/D4SC07995G
(Edge Article)
Chem. Sci., 2025,
16, 3092-3098
Rapid access to functionalized nanographenes through a palladium-catalyzed multi-annulation sequence†
Received
25th November 2024
, Accepted 4th January 2025
First published on 21st January 2025
Abstract
Nanographenes and polycyclic aromatic hydrocarbons exhibit many intriguing physical properties and have potential applications across a range of scientific fields, including electronics, catalysis, and biomedicine. To accelerate the development of such applications, efficient and reliable methods for accessing functionalized analogs are required. Herein, we report the efficient synthesis of functionalized small nanographenes from readily available iodobiaryl and diarylacetylene derivatives via a one-pot, multi-annulation sequence catalyzed by a single palladium catalyst. This method enables the preparation of small nanographenes bearing various polar functional groups, such as hydroxy, amino, and pyridinic nitrogen atoms, which are otherwise difficult to incorporate. These functional groups provide valuable sites for further derivatization, allowing the modulation of small nanographenes' solubility, optoelectronic properties, and photochromic and vapochromic behaviors. Our new method thus provides a platform for facile access to novel carbon-based materials.
Introduction
Nanographenes and polycyclic aromatic hydrocarbons (PAHs) are attractive compounds due to their unique optoelectronic properties, supramolecular behaviors, and potential applications across various fields.1 Since their intrinsic properties are strictly defined by their shapes, sizes, and edge structures, the chemical synthesis of nanographenes and PAHs with atomic precision has attracted significant attention from the synthetic community over the past two decades.2 These extensive investigations have led to the emergence of new synthetic techniques, such as annulative π-extension (APEX) reactions3,4 and on-surface syntheses,5 thereby enabling rapid access to structurally diverse nanographenes and PAHs.
In addition to significant progress in synthesizing fused aromatic hydrocarbons, their functionalized analogs are also attracting increasing interest (Fig. 1A).6 Incorporating functional groups into nanographenes expands their range of applications in materials science and chemical biology by enabling the fine-tuning of photoelectronic properties, enhancing solubility, and allowing for the manipulation of supramolecular interactions. For instance, Aida and Fukushima reported that hexa-peri-hexabenzocoronene derivatives bearing long aliphatic and tetramethylene glycol chains form supramolecular nanotube architectures, resulting in distinct photoconductivity and semiconducting properties.7 Takimiya reported that a tetramethyl-thiolated pyrene exhibits ultrahigh charge mobility as a hole-transporting material in the crystal state.8 Our group also found that a warped nanographene with tetraethylene glycol chains exhibited increased water solubility and induced cell death upon light irradiation.9 Recently, Wilson reported that pyrene tethered to an osmium complex can be utilized for programmable surface modification of polymersomes.10
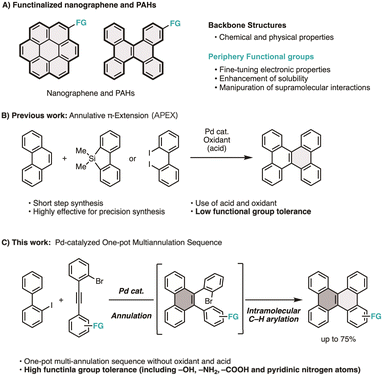 |
| Fig. 1 Functionalized nanographenes and PAHs: (A) applications of functionalized nanographenes and PAHs. (B) Annulative π-extension reactions. (C) This work: Pd-catalyzed one-pot multi-annulation sequence. FG = functional group. | |
Although the significance of functional groups is clear, the synthesis of functionalized nanographene is not straightforward. General synthetic protocols used to access nanographenes and PAHs typically involve oxidative and/or acidic conditions, which typically result in low yields or no reaction when polar functionalities are incorporated into the substrates. Thus, available substituents are generally limited to nonpolar alkyl groups. Although the late-stage functionalization of nanographenes through halogenation,11 iridium-catalyzed C–H borylation,12 and APEX reactions3,4 provide workable protocols, issues such as substrate-dependent regioselectivity and overreaction limit the scope of these approaches to specific examples (Fig. 1B). Consequently, efficient and reliable methods for synthesizing functionalized nanographenes are in high demand.
Herein, we report a one-pot multi-annulation sequence for the synthesis of functionalized dibenzo[g,p]chrysene (DBC), a small nanographene motif, from 2-iodobiphenyls and diarylacetylenes using a single palladium catalyst (Fig. 1C). This simple yet powerful reaction enables the rapid synthesis of DBC derivatives bearing various polar functionalities, such as hydroxy groups, amino groups, and pyridinic nitrogen atoms. Additionally, we demonstrate how these functional groups can be utilized to modulate the solubility and photophysical properties of the functionalized DBCs through further derivatization.
Results and discussion
Optimization of reaction conditions
Initially, we speculated that avoiding acidic or oxidative conditions would improve substrate tolerance when forming fused aromatic ring systems with polar functionalities. To establish a new efficient π-extension method with high functional group tolerance, we considered Larock's report, describing the annulation of 2-iodobiphenyl with internal alkynes to furnish 9,10-diarylphenanthrene using Pd(OAc)2 and NaOAc under nearly neutral conditions.13 Given that many palladium complexes are catalysts for constructing biaryl products,14,15 we envisioned expanding palladium catalysis to a new π-extension reaction via a one-pot multi-annulation sequence of 2-iodobiaryls with appropriately designed diarylacetylenes, followed by intramolecular C–H arylation, to finally construct DBC frameworks.
We first identified the optimal conditions for forming DBC (3aa) using 2-iodobiphenyl (1a) and 1-bromo-2-(phenylethynyl)benzene (2a) as model substrates (Table 1). After extensive screening of reaction parameters, we discovered that 3aa was efficiently formed in a 74% NMR yield and a 67% isolated yield when 1a and 2a were treated with Pd(OAc)2, PtBu2Me·HBF4, 1,8-diazabicyclo(5.4.0)undec-7-ene (DBU), and KOAc in N,N-dimethylformamide (DMF) at 130 °C for 16 h (entry 1). During ligand screening, we observed that while the ligand did not influence the annulation step, it played a crucial role in the intramolecular C–H arylation. Without a ligand, the reaction halted at the first step, resulting in the mono-annulated product INT (entry 2). However, when electron-rich alkyl phosphine ligands such as PCy3·HBF4 and PtBu3·HBF4 were employed, the reaction proceeded to yield the desired product (entries 3 and 4). Conversely, reactions with triphenylphosphine and 2,2′-bipyridyl resulted in low yields (entries 5 and 6). In addition to the ligands, the choice of additives was found to be crucial. When the reaction was performed without KOAc, no product 3aa was observed, and a significant reduction in the amount of the intermediate (INT) was noted (entry 7). Similarly, using K2CO3 instead of KOAc resulted in no formation of 3aa and a reduced yield of INT (entry 8). Comparable yields were obtained when KOPiv or NaOAc were used instead of KOAc (entries 9 and 10), indicating that carboxylate anions are essential for C–H activation and annulation. The addition of DBU slightly enhanced the yield of 3aa, whereas its absence led to a reduction in yield (entry 11). Other organic bases, such as triethylamine (Et3N) and pyridine, did not influence the yield (entries 12 and 13). We postulate that DBU contributes to the efficient generation of active Pd species from the precatalyst or neutralizes the acetic acid generated during the C–H activation steps.
Table 1 Screening of reaction conditions for the one-pot multi-annulation sequence to synthesize DBC (3aa)a
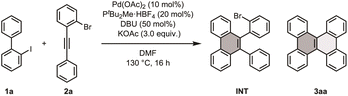
|
Entry |
Deviation from the optimum conditions |
Yieldb (%) |
INT |
3aa
|
Conditions: 1a (0.20 mmol), 2a (1.5 equiv.), Pd(OAc)2 (10 mol%), PtBu2Me·HBF4 (20 mol%), DBU(50 mol%), KOAc (3.0 equiv.), DMF (2.0 mL), 130 °C, 16 h.
NMR yield. CH2Br2 was used as an internal standard.
Isolated yield.
|
1 |
None |
n.d. |
74 (67)c |
2 |
Without PtBu2Me·HBF4 |
64 |
23 |
3 |
PCy3·HBF4 instead of PtBu2Me·HBF4 |
n.d. |
66 |
4 |
PtBu3·HBF4 instead of PtBu2Me·HBF4 |
29 |
37 |
5 |
PPh3 instead of PtBu2Me·HBF4 |
43 |
25 |
6 |
2,2′-bipyridyl instead of PtBu2Me·HBF4 |
49 |
29 |
7 |
Without KOAc |
27 |
n.d. |
8 |
K2CO3 instead of KOAc |
18 |
n.d. |
9 |
KOPiv instead of KOAc |
8 |
58 |
10 |
NaOAc instead of KOAc |
24 |
53 |
11 |
Without DBU |
n.d. |
60 |
12 |
Et3N instead of DBU |
n.d. |
50 |
13 |
Pyridine instead of DBU |
n.d. |
63 |
Scope of substrates
Having established the optimal reaction conditions, we assessed the scope of the reaction with respect to π-extending agents (Fig. 2). We first evaluated π-extending agents bearing para-functionalized arenes and found that various functional groups were compatible with this one-pot multi-annulation process. The reaction with the methoxy-substituted π-extending agent 2b yielded the corresponding product 3ab in 70% yield. The acetoxy group, which is susceptible to hydrolysis under basic conditions, afforded the deacetylated product 3ac directly in 69% yield. The π-extending agent bearing a non-protected amine (2d), a challenging functional group in Pd catalysis, furnished DBC–NH2 (3ad) in 30% yield. Electron-withdrawing substituents such as Cl, CN, and CF3 groups were also tolerated, delivering the corresponding products in yields of 62–75% (3ae–ag). We then explored π-extending agents with meta-substituted arenes (2h–k). For substrates with electron-donating groups, the corresponding products 3ah–ak were afforded as mixtures of separable regioisomers, often in equal or higher yields compared to those with para-substituted π-extending agents. In contrast to the general regioselectivity observed in C–H activation via the concerted metalation-deprotonation (CMD) pathway,15 which typically favors the most acidic or sterically accessible C–H bond, the sterically hindered product was obtained as the major isomer. This trend in reactivity and regioselectivity can be attributed to the proximal guidance by the coordinating functional groups during the C–H activation process.16 In contrast, electron-withdrawing groups, such as acetyl and carboxylic acids, exhibited the opposite regioselectivity, likely due to increased steric hindrance. The compatibility of coordinative heteroaromatic rings is notable; reactions with π-extending agents bearing 4-pyridyl (2l), 3-pyridyl (2m), 5-pyrimidyl (2n), and 3-quinolinyl (2o) produced the corresponding aza-DBCs 3al–ao in good yields, in the range of 47–75%. Further investigations focused on utilizing the present method to construct larger structures and bifunctionalized derivatives. When 2′,5′-diiodo-1,1′:4′,1′′-terphenyl (1b) was employed instead of 1a, the reaction with 2a afforded the doubly extended product 3ba in 38% yield. Moreover, its functionalized analog 3bp was synthesized via the double π-extension of 1b with 2p, although in a low yield. Boc-protected substrate 1c reacted with 2m to yield bifunctional DBC analogs 3cm and 3mc′ as deprotected forms, in 17% and 19% yields, respectively. Overall, this novel π-extension method exhibits broad functional versatility and holds potential for the synthesis of a range of functionalized nanographene structures.
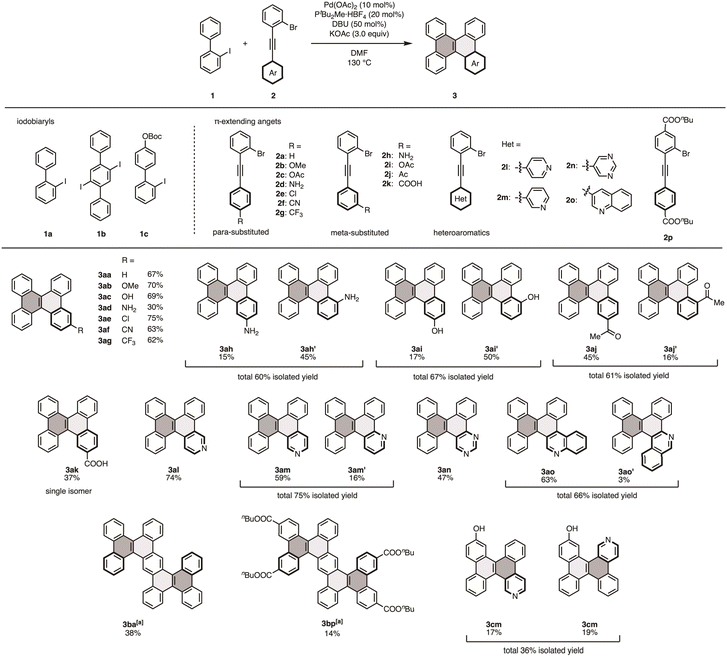 |
| Fig. 2 Scope of substrates for the Pd-catalyzed one-pot multi-annulation sequence. Conditions: (1) (0.20 mmol, 1.0 equiv.), (2) (1.5 equiv.), Pd(OAc)2 (10 mol%), PtBu2Me·HBF4 (20 mol%), DBU (50 mol%), KOAc (3.0 equiv.), DMF (2.0 mL), 130 °C, 16 h. a3.0 equiv. π-extending agent, 20 mol% Pd(OAc)2, 40 mol% PtBu2Me·HBF4, 1.0 equiv. DBU were used. | |
Further derivatization of 3ah′ and 3cm
The properties of functionalized DBCs can be further modified through additional derivatization (Fig. 3). For instance, aminated DBC 3ah′ was reacted with carboxylic acid via a general condensation procedure to yield 3ah′-amide in 82% yield. Unlike unfunctionalized DBC, 3ah′-amide remained in a liquid state at room temperature and displayed improved solubility in polar solvents. Furthermore, 3ah′ was coupled with 4-iodoaniline after nitrosation with m-chloroperbenzoic acid (mCPBA), producing 3ah′-azobenzene, which has potential applications in photoswitching materials. The Sandmeyer reaction was also used to convert 3ah′ into iodinated DBC 1d with a yield of 62%. This iodinated DBC was then used in a multi-annulation sequence with 2l, resulting in 3dl in 10% yield; 3dl is a heterocyclic analog of hexabenzo[a,c,fg,j,l,op]tetracene (HBT), known for its excellent hole-transporting properties in OLEDs.17 In addition, 3am was functionalized with various alkyl electrophiles to form ionic DBCs. Reaction with mPEG4Br produced 3am-PEG4+Br− as a gel-like yellow semisolid in quantitative yield. Treatment with 1,6-dibromohexane resulted in the formation of the ionic dimer 3a in 74% yield. A similar protocol was applied for bifunctional DBC 3cm, yielding the zwitterionic species 3cm-Me. Among these ionic DBC derivatives, 3am-PEG4+Br− displayed excellent solubility in various organic solvents and aqueous media. This dramatic enhancement in solubility can be attributed to the ionic nature of the pyridinium moiety and the hydrophilic triethylene glycol chain.
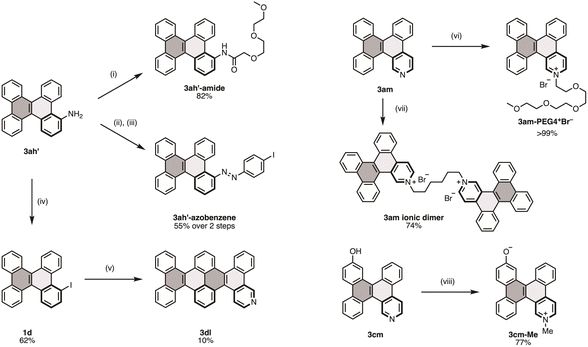 |
| Fig. 3 Further derivatization of 3ah′ and 3am. (i) [2-(2-methoxyethoxy)ethoxy]acetic acid (3.0 equiv.), HCTU (3.0 equiv.), Et3N (5.7 equiv.), DMF, rt, 26 h, 82% yield; (ii) mCPBA (3.1 equiv.), toluene, rt, 0.5 h, 81% yield; (iii) 4-iodoaniline (3.0 equiv.), CH2Cl2/AcOH, 60 °C, 24 h, 68% yield; (iv) NaNO2 (1.7 equiv.), conc. HCl, 0 °C, 2 h, then KI (10 equiv.), rt, 12 h, 62% yield; (v) 2l (1.7 equiv.), Pd2(dba)3·CHCl3 (5 mol%), KOAc (3.0 equiv.), DMF, 130 °C, 12 h, then additional Pd2(dab)3·CHCl3 (5 mol%), PtBu2Me·HBF4 (40 mol%), DBU (1.0 equiv.), 150 °C, 3.5 h, 10% yield; (vi) mPEG4Br (3.9 equiv.), DMF, 90 °C, 20 h, >99% yield; (vii) 1,6-dibromohexane (0.5 equiv.), DMF, 90 °C, 12 h, 74% yield (viii) MeI (1.1 equiv.), DMF, 90 °C, 2.5 h, then NBu4OH (1.0 equiv.), MeOH/DMSO, 5 min, 77% yield. HCTU = 2-(6-chloro-1H-benzotriazole-1-yl)-1,1,3,3-tetramethylammonium hexafluorophosphate; dba = dibenzylideneacetone; mPEG4Br = triethylene glycol 2-bromoethyl methyl ether. | |
Photophysical properties of functionalized DBCs
Selected UV-vis absorption spectra of the functionalized DBCs in dry DMF solution (6.87–7.50 × 10−5 M) are shown in Fig. 4. Compounds 3am and 3cm displayed intense absorption bands with the longest absorption maxima at 352 and 355 nm, respectively (Fig. 4 and ESI). These absorption profiles closely resemble those of the parent DBC in dichloromethane solution,18 indicating that nitrogen-doping and hydroxylation have minimal impact on the DBC structure's absorption characteristics. In contrast, 3am-PEG4+Br− exhibited a red-shifted absorption band, with the longest wavelength absorption maximum at 421 nm. DFT calculations at the B3LYP/6-31+G(d) level estimated the HOMO and LUMO energy levels of 3am to be −5.761 eV and −1.94 eV, respectively, while for 3am-PEG4+Br−, the values were −8.722 eV and −5.791 eV (see ESI†). The red-shifted absorption is likely due to a significant decrease in the LUMO energy level relative to the HOMO upon N-alkylation, leading to a narrow HOMO–LUMO energy gap. In addition, 3cm-Me showed a further red-shifted absorption band with a weak broad absorption between 480 and 700 nm, attributed to intramolecular charge transfer.19 This weak absorption band was attenuated when a mixture of dry DMF and H2O (99
:
1, v/v) was used as the solvent, and was completely diminished in a DMF/AcOH (99
:
1, v/v) solution (Fig. 5A). Sensitivity to water and acid was even more pronounced in the solid state (Fig. 5B). The solid form of 3cm-Me changed color from dark brown to orange when exposed to atmospheric moisture and reverted to dark brown after 30 min under vacuum. Upon exposure to AcOH vapor, the orange solid of 3cm-Me irreversibly turned yellow, corresponding to the protonated state. This vapochromic behavior in response to humidity and acid vapor demonstrates the potential of 3cm-Me as a vapor-sensing material.20
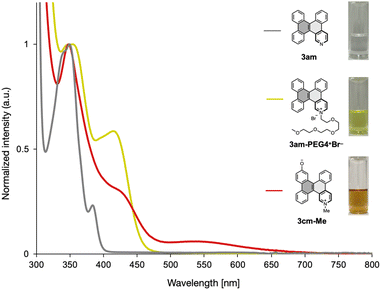 |
| Fig. 4 UV-vis absorption spectra of 3am, 3am-PEG4+Br−, and 3cm-Me in dry DMF solution. | |
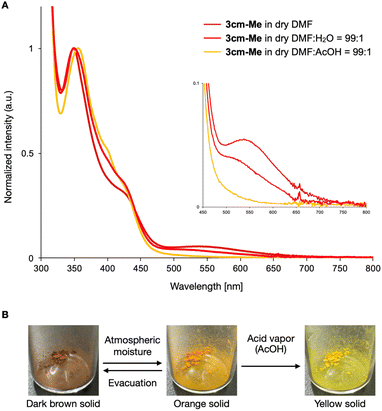 |
| Fig. 5 Water and acid response behavior of 3cm-Me. (A) Water and acid responsive absorption changes in solution. (B) Moisture and acid vapor responsive color changes in the solid state. | |
Conclusions
In summary, we established an efficient approach for functionalizing nanographenes and PAHs via a Pd-catalyzed one-pot, multi-annulation sequence. The combination of Pd(OAc)2/PtBu2Me·HBF4/KOAc effectively promotes both the annulation of 2-iodobiphenyls with diarylacetylenes and subsequent intramolecular C–H arylation, leading to the formation of the DBC framework. The high functional group tolerance of this one-pot sequence allows rapid access to a range of functionalized DBCs bearing amino, hydroxy, and carboxylic acid groups, as well as pyridinic nitrogens—compounds that are challenging to synthesize using previous π-extension methods. We also demonstrated that these functional groups serve as reliable reactive sites for further derivatization, yielding azobenzene, azananographene, and ionic DBCs. UV-vis absorption spectra and DFT calculations of the ionic DBCs indicate that N-alkylation significantly affects their photophysical properties. Additionally, we revealed the unique vapochromic properties of the zwitterionic DBC. Overall, the method developed in this study serves as a reliable platform for facile access to novel carbon-based materials.
Data availability
The data supporting the findings of this study are available in the ESI material.†
Author contributions
T. M. and K. I. conceived and designed the research. T. M. performed experiments and collected the data. T. M. and K. I. wrote the manuscript.
Conflicts of interest
The authors declare no conflict of interest.
Acknowledgements
We thank Mr Jia-Wei She and Dr Hsiao-Hua Yu (Academia Sinica) for their support with the UV-vis absorption measurements. We also thank Mr Daiki Imoto and Associate Prof. Akiko Yagi (Nagoya University) for their support with the DFT calculations. This study was supported by the Ministry of Science and Technology of Taiwan and Academia Sinica (grant number AS-GC-111-M05). Computational studies were conducted at the Research Center for Computational Science in Okazaki, Japan (24-IMS-C123). Mass spectrometry analyses were performed by Mass Spectrometry facility of the Institute of Chemistry, Academia Sinica, Taiwan.
Notes and references
- For selected reviews on the application of nanographenes and graphene nanoribbons, see:
(a) V. Georgakilas, J. A. Perman, J. Tucek and R. Zboril, Chem. Rev., 2015, 115, 4744 CrossRef CAS;
(b) N. Panwar, A. M. Soehartono, K. K. Chan, S. Zeng, G. Xu, J. Qu, P. Coquet, K.-T. Yong and X. Chen, Chem. Rev., 2019, 119, 9559 CrossRef CAS PubMed;
(c) Y. Gu, Z. Qiu and K. Müllen, J. Am. Chem. Soc., 2022, 144, 11499 CrossRef CAS PubMed.
-
(a) Y. Segawa, H. Ito and K. Itami, Nat. Rev. Mater., 2016, 1, 15002 CrossRef CAS;
(b) Y. Segawa, A. Yagi, K. Matsui and K. Itami, Angew. Chem., Int. Ed., 2016, 55, 5136 CrossRef CAS;
(c) K. Itami and T. Maekawa, Nano Lett., 2020, 20, 4718 CrossRef CAS;
(d) I. A. Stepek, M. Nagase, A. Yagi and K. Itami, Tetrahedron, 2022, 123, 132907 CrossRef CAS.
- For reviews on APEX reactions, see:
(a) H. Ito, K. Ozaki and K. Itami, Angew. Chem., Int. Ed., 2017, 56, 11144 CrossRef CAS PubMed;
(b) H. Ito, Y. Segawa, K. Murakami and K. Itami, J. Am. Chem. Soc., 2019, 141, 3 CrossRef CAS PubMed.
-
(a) K. Mochida, K. Kawasumi, Y. Segawa and K. Itami, J. Am. Chem. Soc., 2011, 133, 10716 CrossRef CAS;
(b) K. Ozaki, K. Kawasumi, M. Shibata, H. Ito and K. Itami, Nat. Commun., 2015, 6, 6251 CrossRef CAS;
(c) K. Ozaki, K. Murai, W. Matsuoka, K. Kawasumi, H. Ito and K. Itami, Angew. Chem., Int. Ed., 2017, 56, 1361 CrossRef CAS PubMed;
(d) W. Matsuoka, H. Ito and K. Itami, Angew. Chem., Int. Ed., 2017, 56, 12224 CrossRef CAS;
(e) Y. Koga, T. Kaneda, Y. Saito, K. Murakami and K. Itami, Science, 2018, 359, 435 CrossRef CAS;
(f) S. Matsubara, Y. Koga, Y. Segawa, K. Murakami and K. Itami, Nat. Catal., 2020, 3, 710 CrossRef CAS;
(g) K. P. Kawahara, W. Matsuoka, H. Ito and K. Itami, Angew. Chem., Int. Ed., 2020, 59, 6383 CrossRef CAS;
(h) W. Matsuoka, H. Ito, D. Sarlah and K. Itami, Nat. Commun., 2021, 12, 3940 CrossRef CAS;
(i) W. Matsuoka, K. P. Kawahara, H. Ito, D. Sarlah and K. Itami, J. Am. Chem. Soc., 2023, 145, 658 CrossRef CAS.
- For selected reviews on on-surface synthesis, see:
(a) S. Clair and D. G. De Oteyza, Chem. Rev., 2019, 119, 4717 CrossRef CAS PubMed;
(b) L. Grill and S. Hecht, Nat. Chem., 2020, 12, 115 CrossRef CAS PubMed , For selected examples on on-surface synthesis, see: ;
(c) J. Cai, P. Ruffieux, R. Jaafar, M. Bieri, T. Braun, S. Blankenburg, M. Muoth, A. P. Seitsonen, M. Saleh, X. Feng, K. Müllen and R. Fasel, Nature, 2010, 466, 470 CrossRef CAS PubMed;
(d) H. Zhang, H. Lin, K. Sun, L. Chen, Y. Zagranyarski, N. Aghdassi, S. Duhm, Q. Li, D. Zhong, Y. Li, K. Müllen, H. Fuchs and L. Chi, J. Am. Chem. Soc., 2015, 137, 4022 Search PubMed;
(e) S. Kawai, S. Saito, S. Osumi, S. Yamaguchi, A. S. Foster, P. Spijker and E. Meyer, Nat. Commun., 2015, 6, 8098 CrossRef CAS PubMed;
(f) P. Ruffieux, S. Wang, B. Yang, C. Sánchez-Sánchez, J. Liu, T. Dienel, L. Talirz, P. Shinde, C. A. Pignedoli, D. Passerone, T. Dumslaff, X. Feng, K. Müllen and R. Fasel, Nature, 2016, 531, 489 CrossRef CAS PubMed;
(g) C. Moreno, M. Vilas-Varela, B. Kretz, A. Garcia-Lekue, M. V. Costache, M. Paradinas, M. Panighel, G. Ceballos, S. O. Valenzuela, D. Peña and A. Mugarza, Science, 2018, 360, 199 CrossRef CAS PubMed;
(h) S. Mishra, T. G. Lohr, C. A. Pignedoli, J. Liu, R. Berger, J. I. Urgel, K. Müllen, X. Feng, P. Ruffieux and R. Fasel, ACS Nano, 2018, 12, 11917 Search PubMed;
(i) J. Li, S. Sanz, N. Merino-Díez, M. Vilas-Varela, A. Garcia-Lekue, M. Corso, D. G. De Oteyza, T. Frederiksen, D. Peña and J. I. Pascual, Nat. Commun., 2021, 12, 5538 CrossRef.
-
(a) F. Würthner, C. R. Saha-Möller, B. Fimmel, S. Ogi, P. Leowanawat and D. Schmidt, Chem. Rev., 2016, 116, 962 CrossRef;
(b) A. Borissov, Y. K. Maurya, L. Moshniaha, W.-S. Wong, M. Żyła-Karwowska and M. Stępień, Chem. Rev., 2022, 122, 565 CrossRef;
(c) Q. Li, Y. Zhang, Z. Xie, Y. Zhen, W. Hu and H. Dong, J. Mater. Chem. C, 2022, 10, 2411 RSC.
-
(a) J. P. Hill, W. Jin, A. Kosaka, T. Fukushima, H. Ichihara, T. Shimomura, K. Ito, T. Hashizume, N. Ishii and T. Aida, Science, 2004, 304, 1481 CrossRef PubMed;
(b) Y. Yamamoto, T. Fukushima, Y. Suna, N. Ishii, A. Saeki, S. Seki, S. Tagawa, M. Taniguchi, T. Kawai and T. Aida, Science, 2006, 314, 1761 CrossRef PubMed;
(c) W. Zhang, W. Jin, T. Fukushima, A. Saeki, S. Seki and T. Aida, Science, 2011, 334, 340 CrossRef PubMed.
- K. Takimiya, K. Bulgarevich, M. Abbas, S. Horiuchi, T. Ogaki, K. Kawabata and A. Ablat, Adv. Mater., 2021, 33, 2102914 CrossRef PubMed.
- H.-A. Lin, Y. Sato, Y. Segawa, T. Nishihara, N. Sugimoto, L. T. Scott, T. Higashiyama and K. Itami, Angew. Chem., Int. Ed., 2018, 57, 2874 CrossRef PubMed.
- S. Zhang, W. Li, J. Luan, A. Srivastava, V. Carnevale, M. L. Klein, J. Sun, D. Wang, S. P. Teora, S. J. Rijpkema, J. D. Meeldijk and D. A. Wilson, Nat. Chem., 2023, 15, 240 CrossRef PubMed.
-
(a) Y.-Z. Tan, B. Yang, K. Parvez, A. Narita, S. Osella, D. Beljonne, X. Feng and K. Müllen, Nat. Commun., 2013, 4, 2646 CrossRef PubMed;
(b) Y.-M. Liu, H. Hou, Y.-Z. Zhou, X.-J. Zhao, C. Tang, Y.-Z. Tan and K. Müllen, Nat. Commun., 2018, 9, 1901 CrossRef PubMed.
-
(a) M. N. Eliseeva and L. T. Scott, J. Am. Chem. Soc., 2012, 134, 15169 CrossRef CAS PubMed;
(b) R. Yamaguchi, S. Hiroto and H. Shinokubo, Org. Lett., 2012, 14, 2472 CrossRef CAS PubMed;
(c) K. Kawasumi, Q. Zhang, Y. Segawa, L. T. Scott and K. Itami, Nat. Chem., 2013, 5, 739 CrossRef CAS PubMed;
(d) Y. Segawa, T. Maekawa and K. Itami, Angew. Chem., Int. Ed., 2015, 54, 66 CrossRef CAS PubMed;
(e) K. Kato, H.-A. Lin, M. Kuwayama, M. Nagase, Y. Segawa, L. T. Scott and K. Itami, Chem. Sci., 2019, 10, 9038 RSC;
(f) I. A. Stepek and K. Itami, ACS Mater. Lett., 2020, 2, 951 CrossRef CAS.
- R. C. Larock, M. J. Doty, Q. Tian and J. M. Zenner, J. Org. Chem., 1997, 62, 7536 CrossRef CAS.
- For selected reviews on biaryl synthesis by C–H arylation, see:
(a) D. Alberico, M. E. Scott and M. Lautens, Chem. Rev., 2007, 107, 174 CrossRef CAS;
(b) L.-C. Campeau, D. R. Stuart and K. Fagnou, Aldrichimica Acta, 2007, 40, 35 CAS;
(c) X. Chen, K. M. Engle, D. Wang and J.-Q. Yu, Angew. Chem., Int. Ed., 2009, 48, 5094 CrossRef CAS PubMed;
(d) L. Ackermann, R. Vicente and A. R. Kapdi, Angew. Chem., Int. Ed., 2009, 48, 9792 CrossRef CAS;
(e) J. Yamaguchi, A. D. Yamaguchi and K. Itami, Angew. Chem., Int. Ed., 2012, 51, 8960 CrossRef CAS;
(f) M. Simonetti, D. M. Cannas and I. Larrosa, Adv. Organomet. Chem., 2017, 67, 299 CrossRef CAS;
(g) W. Hagui, H. Doucet and J.-F. Soulé, Chem, 2019, 5, 2006 CrossRef CAS;
(h) J. Grover, G. Prakash, N. Goswami and D. Maiti, Nat. Commun., 2022, 13, 1085 CrossRef CAS.
-
(a) L.-C. Campeau, M. Parisien, M. Leblanc and K. Fagnou, J. Am. Chem. Soc., 2004, 126, 9186 CrossRef CAS;
(b) M. Parisien, D. Valette and K. Fagnou, J. Org. Chem., 2005, 70, 7578 CrossRef CAS;
(c) L.-C. Campeau, P. Thansandote and K. Fagnou, Org. Lett., 2005, 7, 1857 CrossRef CAS PubMed;
(d) L.-C. Campeau, M. Parisien, A. Jean and K. Fagnou, J. Am. Chem. Soc., 2006, 128, 581 CrossRef CAS PubMed;
(e) M. Lafrance, D. Lapointe and K. Fagnou, Tetrahedron, 2008, 64, 6015 CrossRef CAS;
(f) D. Lapointe and K. Fagnou, Chem. Lett., 2010, 39, 1118 CrossRef.
- C. Pinilla, V. Salamanca, A. Lledós and A. C. Albéniz, ACS Catal., 2022, 12, 14527 CrossRef CAS.
- Y. Morinaka, H. Ito, K. J. Fujimoto, T. Yanai, Y. Ono, T. Tanaka and K. Itami, Angew. Chem., Int. Ed., 2024, 63, e202409619 CrossRef CAS PubMed.
- S. Hashimoto, T. Ikuta, K. Shiren, S. Nakatsuka, J. Ni, M. Nakamura and T. Hatakeyama, Chem. Mater., 2014, 26, 6265 CrossRef CAS.
- V. Diemer, H. Chaumeil, A. Defoin, P. Jacques and C. Carré, Tetrahedron Lett., 2005, 46, 4737 CrossRef CAS.
-
(a) H. Yamagishi, S. Nakajima, J. Yoo, M. Okazaki, Y. Takeda, S. Minakata, K. Albrecht and K. Yamamoto, Commun. Chem., 2020, 3, 118 CrossRef CAS;
(b) T. Ono and Y. Hisaeda, Symmetry, 2020, 12, 1903 CrossRef CAS.
|
This journal is © The Royal Society of Chemistry 2025 |
Click here to see how this site uses Cookies. View our privacy policy here.