DOI:
10.1039/D4SM01524J
(Paper)
Soft Matter, 2025,
21, 2548-2557
Quantitative rationalization of the unexpectedly moderate water wettability of poly(vinyl alcohol) surfaces: thermodynamic evaluation and prediction of surface hydrogen bonding†
Received
24th December 2024
, Accepted 24th February 2025
First published on 25th February 2025
Abstract
In this work, a series of poly(vinyl alcohol) (PVA) films with defined but varied water wettability was prepared by curing as-prepared PVA films at systematically adjusted temperatures. The polar components of surface energy (γs,p) of the resulting PVA films were calculated and correlated with the molecular configurations of their surface OH groups—free OH (OHf), trans-hydrogen bonded OH (OHt), and gauche-hydrogen bonded OH groups (OHg)—with the aid of attenuated total reflectance Fourier transform infrared spectroscopy. By decomposing the γs,p values of the PVA films as a sum of the contributions from OHf, OHt, and OHg groups, the intrinsic γs,p components of
and
were calculated to be 8.0 mN m−1 and 9.8 mN m−1, respectively, which were substantially smaller than that of
. This provided a thermodynamic foundation not only to rationalize the unexpectedly moderated surface hydrophilicity of PVA films but also to quantitatively predict the fHB component of hydrogen-bonded OH groups on their surfaces according to their water wettability.
1 Introduction
Polymers are widely utilized as surface coating materials to bestow chemical diversity and biomimetic potential onto disparate solid surfaces.1–7 In pursuit of eco-friendly industrial and household cleaning, hydrophilic polymers are increasingly favoured for crafting self-cleaning surfaces that are capable of cleaning themselves upon contact with water.8–13 However, hydrophilic polymer surfaces struggle to fully unfold their built-in hydration potential in practical applications as a consequence of surface reconstruction (inward orientation) of the polar groups on their surfaces upon being exposed to the air environment and hydrogen bonding between surface polar groups (such as hydroxyl, amine, and carboxylic acid).14–16 This significantly reduces the polar interaction components (γs,p) of surface energy and their contributions to the total surface energy (γs) of hydrophilic polymer coatings, resulting in moderate water wettability. To date, extensive experimental and theoretical efforts have been devoted to studying the impact of surface reconstruction on the water wettability of hydrophilic polymer surfaces.17–19 However, there has been limited rational analysis of how hydrogen bonding between surface polar groups affects surface hydrophilicity. In this context, the present work aimed to develop a thermodynamic framework to quantitatively rationalize the impact of hydrogen bonding of surface polar groups on the water wettability of hydrophilic polymer thin films with the objective of describing their γs,p as a sum of the intrinsic contributions from free polar groups
and hydrogen-bonded ones
on their surfaces.
Poly(vinyl alcohol) (PVA) is known for its excellent water solubility and film-forming ability on both hydrophilic and hydrophobic substrates and biocompatibility.20–22 Owing to its abundant OH side groups, PVA chains are capable of effectively forming hydrogen bonds, either with themselves (for instance, through repetitive freeze/thaw methods) or with other molecules, such as glutaraldehyde and tannic acid,23–25 leading to the formation of excellent hydrogels that have been widely applied in many fields, such as biomedicine, soft electronics, seawater desalination, and oil/water separation.26–32 However, due to this very hydrogen bonding, PVA thin films generally exhibit relatively large water contact angles in air (θw/a of 60°–72°).33–36 Despite this, there are few studies on how this unexpectedly moderate water wettability is correlated with the hydrogen bonding configuration of surface OH groups. To address this issue, herein, model PVA thin films were prepared by casting from their aqueous solutions, whose θw/a were systematically altered by varying the drying temperatures. By meticulously differentiating the surface fractions of free OH groups (OHf), trans-hydrogen bonded OH groups (OHt), and gauche-hydrogen bonded OH groups (OHg) – ff, ft and fg – with the help of X-ray photoelectron spectroscopy (XPS) and attenuated total reflection Fourier transform infrared spectroscopy (ATR-FTIR), we managed to decompose the γs,p of the model PVA thin films into the intrinsic contributions of the surface OHf, OHt, and OHg groups –
– as described in eqn (1):
|  | (1) |
As such, the

and

were calculated to be 8.0 mN m
−1 and 9.8 mN m
−1, respectively, which were substantially smaller than that of

(50 mN m
−1) and served as the rationale behind the unexpected water wettability of the cast PVA thin films (
θw/a of approx. 68°). The validity and generality of the resulting

and

were endorsed by their success in estimating the
fHB component of hydrogen-bonded OH groups on different types of PVA surfaces, according to the variant of
eqn (1)–(13), in excellent agreement with spectroscopic analysis by means of ATR-FITR. In this scenario, therefore, the present work offers a thermodynamic framework to quantitatively rationalize and describe the impact of hydrogen bonding of surface polar groups on the water wettability of hydrophilic polymer surfaces.
2 Materials and methods
2.1 Materials
Three types of PVA with low, medium and high molecular weight were used to create thin films. PVA with a Mw of 13
000–23
000 and an alcoholysis degree of 98% (L-PVA) was purchased from Sigma-Aldrich Co., Ltd (Shanghai, China). PVA with a Mw of approx. 77
000 and alcoholysis degree of 98% (M-PVA) was obtained from China National Pharmaceutical Group Co., Ltd (Beijing, China). Finally, PVA with a Mw of 85
000–124
000 and alcoholysis degree of 98–99% (H-PVA) was purchased from Shanghai Chemical Co., Ltd (Shanghai, China). Hexadecane was purchased from Aladdin Bio-Chem Technology Co., Ltd (Shanghai, China). Water with a resistivity of 18 MΩ cm−1 was prepared using a Millipore Milli-Q system, and used in all experiments.
2.2 Methods
2.2.1 Preparation of the PVA surfaces.
Aqueous PVA solution was prepared by dissolving a given amount of PVA powder in deionized water at 90 °C under magnetic stirring at 900 rpm for more than 2 h. After the PVA powder was completely dissolved, the resulting PVA solutions were cooled down to room temperature and filtered to remove any insoluble impurities, and their concentration was adjusted to be either 2 wt% or 7 wt%.
2.2.1.1 Preparation of the model L-PVA thin films.
Model L-PVA thin films were prepared according to the protocol reported in the literature.33 Then, 420 μL of the resulting aqueous solution of L-PVA (2 wt%) was dropwise cast into a thin film on a glass slide (2.5 × 2.5 cm2), followed by 1 h drying at 20 °C, 50 °C, 80 °C, 110 °C, 140 °C, and 180 °C, respectively, and 24 h drying at room temperature in a desiccator. The drying duration was deliberately set to be 1 h in order to minimize the potential risk of heating-induced surface reconstruction, especially in high temperatures. The resulting model PVA films were denoted as L_PVAT2, where the superscript “T” highlights the temperature of the first drying stage and the subscript “2” highlights the concentration of the PVA aqueous solutions used for casting.
Prior to the surface wetting test, the resulting L_PVAT2 films were abraded using sandpaper to reveal the uniformity of their structural and chemical features across the bulk. The films were placed on 800 grit sandpaper and then pressed with a 100 g weight, which was pushed to move it along a ruler by 10 cm at a speed of 0.5 cm s−1, then rotated 90°, and moved back in the same way. The above-mentioned process was defined as 1 abrasion cycle.
2.2.1.2 Preparation of H-PVA thin films.
H-PVA thin films were prepared according to the protocol reported in the literature.34 The resulting aqueous solution of H-PVA (2 wt%) with 420 μL was dropwise cast onto a glass slide (2.5 × 2.5 cm2), followed by successively drying at 30 °C for 1 d, at 80 °C for 1 h, and at 40 °C under vacuum for 24 h. The resulting H-PVA films were denoted as H_PVA802.
2.2.1.3 Preparation of the L-PVA thin films.
L-PVA thin films were prepared according to the protocol reported in the literature.37 Then, 420 μL of the resulting aqueous solution of L-PVA (7 wt%) was dropwise cast onto a glass slide (2.5 × 2.5 cm2), followed by 24 h drying under ambient conditions. The resulting L-PVA films were denoted as L_PVART7.
2.2.1.4 Preparation of the dry PVA gel films.
The PVA hydrogel was first prepared using the repeated freeze–thaw method.38 Typically, 4 g of M-PVA powder was dissolved into a binary solvent consisting of 16 mL of DMSO and 4 mL of deionized water at 95 °C under magnetic stirring until a transparent and viscous solution was obtained. The resulting solution was then poured into a custom-made mold and subjected to 10 freeze–thaw cycles to form a hydrogel. After the hydrogel was demolded and thoroughly washed with deionized water to remove residual DMSO, it was thoroughly dried under ambient conditions for several days prior to surface wettability analysis. The resulting dried PVA gel films were denoted as M_PVARTG.
2.2.2 Surface wettability analysis.
Contact angle measurement was implemented on a drop shape analyser – DSA100 system (Krüss GmbH, Germany) at ambient temperature. Prior to the contact angle test, the as-prepared PVA films were stored in a desiccator for 24 h at 25 °C under a relative humidity of 25–30% to ensure measurement consistency. The static θw/a values of the as-prepared PVA films were measured by placing a water droplet of 2 μL volume. The θw/a value of a given film surface was obtained as an average of three values was collected on different positions on the surface after the 2 μL droplets of water were placed on top and equilibrated for 10 s.
Calculations of γs, γs,p and γs,d were carried out on the basis of the Fowkes model for a surface wetted by water in air,39 as described in eqn (2):
|  | (2) |
where
γw is 72.8 mN m
−1,
γdw is 21.8 mN m
−1 and
γpw is 51.0 mN m
−1.
2.3 Surface spectroscopic analysis
2.3.1 X-ray photoelectron spectroscopy (XPS).
XPS was carried out on a Kratos Axis X-ray Ultra photoelectron spectrometer, in which monochromatic Al Kα (1486.6 eV) X-ray with power of 225 W was applied at an incidence angle of 90° to achieve a depth of detection of approx. 15 nm from the surface of the samples.
2.3.2 Attenuated total reflection Fourier transform infrared spectroscopy (ATR-FTIR).
ATR-FTIR was carried out on a Nicolet Aratar 370 attenuated total reflection Fourier transform spectrometer, in which zinc selenide crystals were used as internal reflecting elements at an incidence angle of 45°, and the spectral data for the experimental part were all cumulative averages obtained from 64 scans at a resolution of 4 cm−1.
2.3.3 Step profiler.
The thicknesses of the L_PVA202 and L_PVA1802 films were measured by step profiler (KLA, D500), with three independent measurements performed to ensure statistical reliability.
3 Results and discussion
3.1 The water wettability of the model L-PVA thin films
PVA is capable of forming uniform thin films on both hydrophilic and hydrophobic substrates by means of simple casting of their aqueous solution.20 A key feature of the resulting PVA thin films is that the OH side groups of PVA tend to effectively and extensively form hydrogen bonds with each other.40 As suggested in the literature,41–43 on the other hand, the gyration radius (Rg) of the PVA chains in an aqueous solution and the distance between their OH groups can be regulated by temperature, thus enabling adjustment of the fraction of hydrogen-bonded OH (OHHB) groups on the cast PVA thin films. To study the impact of hydrogen bonding of the surface OH groups on the water wettability on the PVA surfaces, here 6 different model surfaces (L_PVAT2) were prepared by casting the aqueous solution of L-PVA into thin films, followed by drying at 20 °C, 50 °C, 80 °C, 110 °C, 140 °C, and 180 °C, respectively, as described in 2.2.1.1. UV-vis spectroscopic analysis demonstrates the excellent transparency of the as-prepared L_PVAT2 thin films (Fig. 1a), and atomic force microscopic (AFM) imaging reveals their excellent surface smoothness with a root mean square (RMS) roughness in the range of 1.67 nm to 1.82 nm (Fig. 1b). The thicknesses of the L_PVA202 and L_PVA1802 thin films were 7.0 μm and 7.3 μm by the step profiler, respectively. These results rule out the impact of the surface structural features of the L_PVAT2 thin films on their surface hydrophilicity. To ensure measurement consistency, the as-prepared L_PVAT2 thin films were subjected to 24 h incubation in a desiccator under controlled conditions (25 °C, relative humidity 25–30%) prior to the contact angle test, although they showed negligible change in θw/a during storage under ambient conditions. Fig. 1c shows that the θw/a of the L_PVAT2 thin films decreases from 68.3° to 48.3° with the drying temperature increasing from 20 °C to 180 °C. The revealed moderate water wettability reflects that surface OH groups on the L_PVAT2 thin films tend to form hydrogen bonds not with water but with each other, which can be notably disrupted when the cast thin film is dried at elevated temperatures. However, a further increase in the temperature from 140 °C to 180 °C causes little change in θw/a. This is because the negative impact of surface reconstruction becomes negligible when the cast PVA thin films are dried at such high temperature even for a short period of time. As shown in Fig. 1d, the pronounced negative impact of hydrogen bonding of the surface OH groups is endorsed by the unexpected small γs,p values of the L_PVAT2 thin films – the γs,p of the L_PVA202 thin films being 12.8 mN m−1 – especially taking into account the γs,p maximum theoretically expected for surface OH groups (
of 50 mN m−1).17 The elevation of the drying temperature from 20 °C and 140 °C can drive the γs,p of the resulting L_PVAT2 thin films by about 2 times due to disruption of the OHHB groups into OHf groups on their surfaces, yet the γs,p of the L_PVA1402 thin film (25.8 mN m−1) remains about 2 times smaller than the
of the surface OH groups (50 mN m−1). On the other hand, it is worth noting that the γs,d of all as-prepared L_PVAT2 thin films is around 27.1 mN m−1. As suggested in the literature,17 this indicates that the resulting PVA thin films are composed of densely packed PVA chains, thus ensuring stable θw/a values.
 |
| Fig. 1 (a) Plot of the transmittance values of the as-prepared L_PVAT2 thin films deposited on glass slides versus the drying temperature (T). The inset shows the corresponding UV-vis spectra in the wavelength range of 200–700 nm. (b) AFM images of the as-prepared L_PVA202 and L_PVA1802 thin films, with the corresponding RMS values marked. (c) Plot of the θw/a values of the as-prepared L_PVAT2 thin films versus the drying temperature (T), with schematics of a 2 μL droplet of water placed on the corresponding L_PVAT2 thin films and a 2 μL hexadecane droplet placed on the L_PVA202 thin films shown. (d) Histogram of the values of γs, γs,d and γs,p of the as-prepared L_PVAT2 thin films. | |
3.2 The molecular configurations of surface OH groups on model L-PVA thin films
In order to gain a better understanding of the molecular configurations of OH groups on the as-prepared L_PVAT2 thin films, both XPS and ATR-FTIR spectroscopies were applied to characterize their surfaces. As shown in Fig. 2a–c, the L_PVAT2 thin films show three distinct C 1s bands centred at 284.6, 286.2, and 289.0 eV, corresponding to C–H, C–OH, and O–C
O groups, respectively. While the presence of the O–C
O group indicates incomplete alcoholysis of the L-PVA used, the profiles and portions of the C 1s bands of the L_PVAT2 thin films are hardly dependent on the drying temperature, which rules out the disparity in the chemical composition between the L_PVAT2 thin films and their impact on their surface hydrophilicity. Fig. 2d shows the ATR-FTIR spectra of the L_PVAT2 thin films, in which a prominent band centered at 3273 cm−1 is assigned to the stretching vibrations of the OHHB groups of the PVA chains. Neither free OH side groups of the PVA chains (centred around 3600 cm−1) nor those forming hydrogen bonds with water molecules (centred around 3460 cm−1) are visible. It is worth noting that the vibrational band of the OHHB groups of the L_PVAT2 thin films becomes weaker with increasing dry temperature, indicative of the temperature-induced disruption of the hydrogen bonds of the neighboring OH groups in the bulk environment and on the surface. Fig. 2e shows that the vibrational band of the OHHB groups of the L_PVA1402 thin films and their water wettability remain largely unchanged after their surfaces are subjected to multiple cycles of abrasion via sandpaper, which is in line with the molecular configuration of the surface OH groups specifically revealed by means of sum frequency generation spectroscopy. These results highlight the uniformity of the molecular structures across the thin films. Thus, the hydrogen-bonding configurations of the OH side groups of the PVA chains, revealed in Fig. 2d, can reasonably represent the molecular configuration of the surface OH groups of the L_PVAT2 thin films. ATR-FITR spectroscopy is expected to sense thicker regions of about several μm in depth beneath their outermost surfaces,44 though.
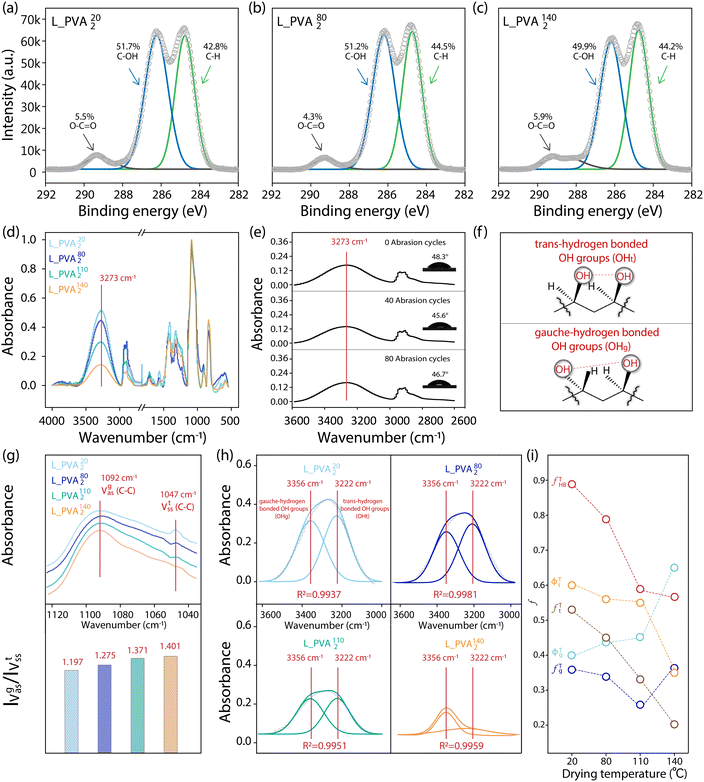 |
| Fig. 2 (a)–(c) High-resolution XPS spectra of the C 1s signals of L_PVA202 (a), L_PVA802 (b), and L_PVA1402 thin films (c). (d) ATR-FTIR spectra of the L_PVA202, L_PVA802, L_PVA1102, and L_PVA1402 thin films in the range of 4000–400 cm−1. (e) ATR-FTIR spectra of the as-prepared L_PVA1402 thin films after their surfaces were subjected to 0, 40 and 80 cycles of abrasion, with schematics of the 2 μL water droplets placed on the corresponding surfaces shown in the inset. (f) Schematic depiction of the trans- and gauche-hydrogen bonding configurations of the OH groups of the PVA chains. (g) ATR-FTIR spectra of the hydrocarbon backbones of the PVA chains of the as-prepared L_PVAT2 thin films in the range of 1130–1030 cm−1, with their corresponding IVasg/IVsst values. (h) ATR-FTIR spectra of the OH side groups of the PVA chains of as-prepared L_PVAT2 thin films in the range of 3600–3000 cm−1, with the spectra deconvoluted into the vibrational bands of the OHt and OHg groups using Gaussian curve fitting. (i) Plots of the calculated fTHB, fTt, ϕTt, fTg and ϕTg values of the as-prepared L_PVAT2 thin films versus the drying temperature (T). | |
As reported in the literature,45 the OH side groups of the PVA chains can adopt two distinct hydrogen-bonding configurations: trans- and gauche-configurations (Fig. 2f). For the L_PVAT2 thin films, there are three types of OH groups present on their surfaces: free OH group (OHf), trans-hydrogen bonded OH groups (OHt), and gauche-hydrogen bonded OH groups (OHg). Fig. 2g shows that the ratio of the asymmetric C–C stretching vibration band (IVasg) of the PVA backbones to the corresponding symmetric stretching vibration band (IVsst) – IVasg/IVsst ratio – increases from 1.2 to 1.4 for the L_PVAT2 thin films with increasing drying temperature. This indicates that the temperature-induced disruption of hydrogen bonds of the OH groups for weak gauche-hydrogen bonds are expected to become more favourable than strong trans-hydrogen bonds at high temperatures.44 Furthermore, Fig. 2h reveals that the vibrational bands of the OHHB groups of the as-prepared L_PVAT2 thin films exhibit a notable dependence of their profile on the drying temperature. They can be readily decomposed into two bands centered at 3222 cm−1 and 3356 cm−1 with the assistance of Gaussian curve fitting, which can be assigned to strong trans- and weak gauche-hydrogen bonding configurations of the OH groups of PVA chains, respectively.46,47 It is clear that with the drying temperature increasing to above 140 °C, the vibrational band of OHt is more significantly reduced than that of OHg. This reflects the fact that gauche-hydrogen bonding is weaker than trans-hydrogen bonding.
On the basis of the vibrational band deconvolution of the OHHB groups from the resulting L_PVAT2 thin films, as shown in Fig. 2h, the fractions of OHt and OHg – marked as ϕTi where i represents t or g – among their hydrogen-bonded OH groups can be estimated according to the following equations:
|  | (3) |
| ϕTi = cTi/cTHB = ATi/ATHB | (4) |
where
ATi represents the integrated intensity of the corresponding vibrational bands of OH
t or OH
g,
cTi is the concentration of OH
t or OH
g groups (per volume for the entire films or per area for the film surface) and
ϕTi is their corresponding fraction. In
eqn (3),
d is the detection pathway length and
εi(
v) is the wavenumber (
v)-dependent absorption coefficient of PVA. In
eqn (4),
ATHB is the integrated intensity of the vibrational bands of the OH
HB groups and
cTHB is their concentration. Accordingly, the fractions of the OH
f and OH
HB groups, as well as the latter components OH
t and OH
g – marked as
fTi where
i represents
f, HB,
t or
g – can be described by the following equations:
| fTHB = cTHB/cTOH = ATHB/ATOH | (6) |
| fTf + fTHB = fTf + fTt + fTg = 1 | (7) |
where
ATOH is the integrated intensity of the vibrational bands of the total OH groups and
cTOH is their concentration. Since the vibrational band of the OH
f groups is hardly visible for the L_PVA
T2 thin films, we were not able to estimate the values of
ATOH (or
cTOH) and in turn, those of
fTf and
fTHB from their ATR-FITR spectra (
Fig. 2h). After, the vibrational band of the OH
HB groups from the resulting L_PVA
T2 thin films are deconvolved
via Gaussian curve fitting with coefficient of determination (
R2) above 0.99. To circumvent this issue, here are the values of
fTf and
fTHB, which were theoretically estimated according to molecular dynamics simulation data reported in the literature.
48 For a solid surface consisting of 30 PVA chains with 150 OH groups, the number of hydrogen bonds formed between these OH groups were found to be 266 instead of 300, the theoretical maximum. Bearing that in mind, the
f20HB of the resulting L_PVA
202 thin films is assumed to be 0.89 (266/300). Thus, the
fTHB of the other L_PVA
T2 thin films are estimated according to
eqn (9):
| fTHB = f20HB·(ATHB/A20HB) | (9) |
The resulting
fTHB values can be used to calculate the corresponding
fTf values according to
eqn (7). By combining
eqn (4), (8) and (9) into
eqn (10):
| fTt(g) = f20HB·(ATi/A20HB) | (10) |
The
fTt and
fTg of the L_PVA
T2 thin films can be readily derived, listed in
Table 1, from the
ATOH values measured from the vibrational band of the OH
HB groups and their
ATt and
ATg components. With the drying temperature increasing, as shown in
Fig. 2i, the
fTHB and
fTt of the resulting L_PVA
T2 thin films notably decrease and yet their
fTg remains hardly changed, which offers a quantitative testimony not only to the temperature-induced disruption of OH
HB groups, but also to the weak
gauche-hydrogen bonding configurations being more favourable than the strong
trans-hydrogen bonding configurations at high temperatures.
Table 1 Summary of the values of fTf, fTHB, ATi, ϕTi, and fTi of the as-prepared L_PVAT2 thin films and their corresponding γs,p and γs,pHB(T) values, where i represents t and g
L_PVAT2 |
f
T
f
|
f
T
HB
|
A
T
t
|
ϕ
T
t
|
f
T
t
|
A
T
g
|
ϕ
T
g
|
f
T
g
|
γ
s,p (mN m−1) |
γ
s,pHB(T) (mN m−1) |
L_PVA202 |
0.11 |
0.89 |
59.5 |
0.60 |
0.53 |
41.7 |
0.40 |
0.36 |
12.8 |
7.3 |
L_PVA802 |
0.21 |
0.79 |
50.5 |
0.56 |
0.45 |
39.6 |
0.44 |
0.34 |
18.7 |
8.2 |
L_PVA1102 |
0.41 |
0.59 |
37.1 |
0.55 |
0.33 |
30.0 |
0.45 |
0.26 |
21.9 |
1.4 |
L_PVA1402 |
0.44 |
0.56 |
22.7 |
0.35 |
0.20 |
42.5 |
0.65 |
0.36 |
25.9 |
3.9 |
3.3 Quantitative differentiation of the contributions of hydrogen-bonded OH groups to the surface energy polar components of the model L-PVA thin films
As mentioned above, the molecular configurations of the OHHB groups of the as-prepared L_PVAT2 thin films, revealed by means of ATR-FITR spectroscopic analysis, are expected not only in the bulk phase, but also on the surface thanks to the structural and chemical uniformity across the films. With that in mind, the γs,p values of the L_PVAT2 thin films, marked as γs,p(T) to specify the drying temperature and summarized in Fig. 1d, are plotted versus their fTHB, fTt, and fTg values, which reveals that the increase in γs,p is a reflection of more OHHB groups being broken into OHf groups with the increasing drying temperature (Fig. 3a). The contribution of OHHB groups to the γs,p(T) of the L_PVAT2 thin films, specifically marked as γs,pHB(T) can be readily calculated according to eqn (11): |  | (11) |
The L_PVAT2 thin films exhibit fairly small γs,pHB(T) values in the range of 1.4–8.2 mN m−1 (listed in Table 1), which show random correlations with either the fTHB (or fTf) values or their components, fTt (or ϕTt) and fTg (or ϕTg) (Fig. 3b). By assuming that surface OHt and OHg groups independently contribute to surface energy, γs,pHB(T) can be decomposed into the intrinsic contributions of OHt and OHg groups –
and
– according to eqn (12): |  | (12) |
In order to implement the computational determination of parameters
and
, we substituted
of 50 mN m−1 into eqn (1) and transformed it into eqn (13): |  | (13) |
Thus, eqn (13) is a first-order three-variable equation in the form of Ax1 + Bx2 − y = 0, where ft, fg and (γs,p − 50) are the three variables of x1, x1 and y, respectively, and
and
are the A and B constants, respectively. By utilizing all four sets of data listed in Table 1, eqn (13) can be well fitted according to a multiple linear regression model with R2 of 0.9924, yielding
of 8.0 mN m−1 with a standard deviation (SD) of 0.67 mN m−1 and 95% confidence interval (CI) of 7.5 ± 1.5 mN m−1 and
of 9.8 mN m−1 with SD of 0.53 mN m−1 and CI of 9.1 ± 1.2 mN m−1. For the as-prepared L_PVAT2 thin films, their γs,p can thus be written as a sum of the intrinsic contributions of the surface OHf, OHt, and OHg groups –
,
, and
– according to eqn (1) (Fig. 3c). In the trans configuration, the relative orientation of two adjacent carbon atoms within the molecule is linear, resulting in a spatial arrangement that is both more stable and symmetrical. This configuration typically corresponds to a lower energy state. Conversely, in the gauche configuration, the relative orientation angle between the adjacent carbon atoms is 60 degrees. This arrangement is spatially more flexible, and may lead to localized increases in energy. Meanwhile, molecular dynamics simulations suggest that the rotation of molecular chains is enhanced with the increasing temperature, so the gauche conformation, with its greater degree of rotational freedom, emerges more frequently.49 It should be noted that the resulting
and
values are more than 5 times smaller than the
of the surface OHf groups (50 mN m−1), and fairly comparable to the γs,p* values of the weakly polar groups such as Br and pyrrole.17 Since the majority of OH groups are present on their hydrogen-bonded configurations (for instance, 89% for the L_PVA202 thin films), these surprisingly weak interactions of the surface OHHB groups with water molecules provide a sensible rationale behind the moderate water wettability of the as-prepared PVA thin films.
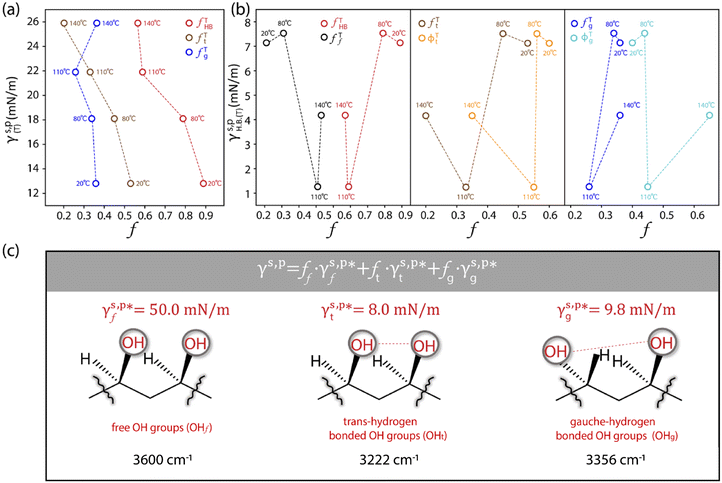 |
| Fig. 3 (a) Plots of the γs,p(T) values of the as-prepared L_PVAT2 thin films versus their fTHB, fTt, and fTg values. (b) Plots of the γs,pHB(T) values of the as-prepared L_PVAT2 thin films versus their fTHB (fTf) (left panel), fTt (ϕTt) (middle panel), and fTg (ϕTg) values (right panel). (c) Schematic summarizing the molecular configurations of the surface OHf, OHg, and OHt groups, with the corresponding , , and values marked. | |
3.4 Extension of the developed thermodynamic framework to other PVA thin films
It is important to note that the
and
, obtained in Section 3.3, are the intrinsic contributions of the surface OHt and OHg groups to the γs,p of the PVA thin films, which should be independent of the methods used to fabricate the PVA thin films. In this scenario, the developed eqn (1) should serve as a thermodynamic framework to rationalize and describe the water wettability of the PVA thin films according to their molecular configurations of surface OH groups, namely, fTHB and its fTt (ϕTt) and fTg (ϕTg) components. To assess the validity and generality of eqn (1) and
and
established above, here we prepared an additional three types of PVA thin film surfaces by using the methods reported in the literature,34,37,38 which were notably different from that used to form L_PVAT2 thin films (details described in Section 2.2.1). Compared to the model L_PVAT2 thin films, the resulting H_PVA802, L_PVART7, and M_PVARTG films show fairly similar surface morphology and transparency revealed by means of scanning electron microscopy (SEM) imaging (Fig. 4a) and UV-Vis spectrometric analysis (Fig. 4b). Fig. 4c shows that all these PVA films exhibit moderate surface hydrophilicity with fairly large θw/a and small γs,p values. It should be noted that the present θw/a values are fairly comparable to those reported in the literature,34,37,38 highlights the reproducibility of the methods reported therein.
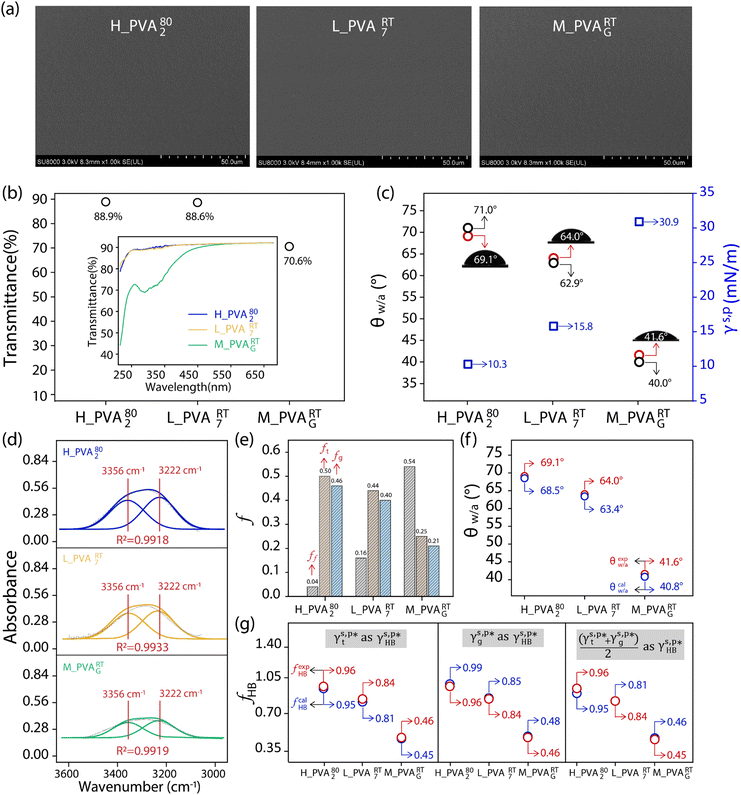 |
| Fig. 4 (a) SEM image of the as-prepared H_PVA802, L_PVART7, and M_PVARTG films. (b) Summary of the transmittance values of the resulting H_PVA802, L_PVART7, and M_PVARTG films, with the corresponding UV-Vis spectra in the wavelength range of 200–700 nm shown in the inset. (c) Summary of the θw/a values of the as-prepared H_PVA802, L_PVART7, and M_PVARTG films (red circles), the γs,p values derived from them (blue squares), and the θw/a values of the corresponding films reported in the literature (black circles), with schematics of the 2 μL droplets of water placed on the corresponding surfaces shown in the inset. (d) and (e) ATR-FTIR spectra of the OH side groups of the PVA chains of the as-prepared H_PVA802, L_PVART7, and M_PVARTG films in the range of 3600–3000 cm−1 (d), with the spectra deconvoluted into the vibrational bands of the OHt and OHg groups using Gaussian curve fitting, and a summary of their ff, ft and fg values determined from them included (e). (f) Comparison of the θcalw/a values (blue circles) of the as-prepared H_PVA802, L_PVART7, and M_PVARTG films, derived from their fHB, ft, fg values according to eqn (1) and (2), with the corresponding experimentally measured θw/a values (red circles) shown in panel c. (g) Summary of the fcalHB values (blue circles) of the as-prepared H_PVA802, L_PVART7, and M_PVARTG films, derived from their θw/a values using , , and as , according to eqn (2) and (13), with the corresponding experimentally measured fHB values shown in panels d and e (red circles). | |
The deconvolution of the ATR-FTIR spectra of these PVA thin films via Gaussian curve fitting (R2 of 0.99) reveals that their surface OH groups tend to form hydrogen bonds with each other (Fig. 4d). In the case of the H_PVA802 thin films, as shown in Fig. 4e, nearly all their surface OH groups are hydrogen bonded together. The fHB is as high as 0.96 with ft of 0.50 and fg of 0.46, which are responsible for their γs,p of 10.3 mN m−1. This value is surprisingly low for hydrophilic polymer thin films. In the case of the thin films made of L-PVA, the slight difference in θw/a and γs,p between the L_PVA202 and L_PVART7 thin films can be reasonably assigned to the subtle variation in their fHB, ft, and fg values. For the M_PVARTG thin films, their γs,p is approximately 30.9 mN m−1 – 61.8% of the
of the surface OHf groups – and their fHB is approx. 0.46 with ft of 0.25 and fg of 0.21. This highlights that even for the PVA hydrogel films, drying in air can drive a noticeable amount of the OH groups of the PVA chains to form hydrogen bonds together in order to significantly lower the γs,p of the dried gel films. These results highlight the non-negligible sensitivity of the surface hydrophilicity of PVA thin films on the polymer molecular weight, and the protocols of fabrication or post-treatments of the thin films.
As suggested above, the H_PVA802, L_PVART7, and M_PVARTG films are expected to possess γs,d similar to that of the L_PVAT2 thin films (27.1 mN m−1), since it was hardly altered by the experimental conditions. With that in mind, here are the γs,p values of the H_PVA802, L_PVART7, and M_PVARTG films were derived from their fHB, ft, fg values by using
of 8.0 mN m−1 and
of 9.8 mN m−1 according to eqn (1), which, subsequently, were utilized to calculate their θw/a values – denoted as θcalw/a – according to eqn (2). Fig. 4f shows that the θcalw/a values of the H_PVA802, L_PVART7, and M_PVARTG films are fairly comparable to those experimentally measured with a deviation of less than 2%. In reverse, the use of
and
may also enable us to derive the fHB, ft, and fg values of the H_PVA802, L_PVART7, and M_PVARTG films from their θw/a values (their γs,p values, narrowly speaking) according to eqn (1). For a specific type of PVA thin films, unfortunately, the θw/a alone was not sufficient to solve the first-order three-variable function of eqn (1). Instead of determining the ft and fg components, here the fHB values of the H_PVA802, L_PVART7, and M_PVARTG films – donated as fcalHB – were calculated by utilizing
,
or their average
to serve as the hypothetically intrinsic γs,pHB of surface OHOH groups
according to the variant of eqn (1) or (11):
|  | (14) |
Fig. 4g shows that the
fcalHB values derived from the
θw/a values of the H_PVA
802, L_PVA
RT7, and M_PVA
RTG films are fairly close to those values, experimentally determined by means of ATR-FTIR spectroscopic analysis (
Fig. 4e), and the deviation of the
fcalHB values obtained by using either

or

as

from their corresponding experimental values can be as small as 3%. These results confirm the validity and generality of

(8.0 mN m
−1),

(9.8 mN m
−1), and
eqn (1) for the rationalization and description of water wettability on PVA surfaces.
4 Conclusions
In summary, we have managed to quantitatively assess and differentiate the impact of hydrogen bonding configurations of surface OH groups on the surface hydrophilicity of PVA thin films, especially with the assistance of ATR-FTIR spectroscopic analysis. In turn, we can then describe the γs,p of PVA thin films as a sum of the
,
, and
of the surface OHf, OHt and OHg groups, weighted by the corresponding ff, ft, and fg (eqn (1)). This enabled us to predict the surface hydrophilicity (θw/a) of the PVA thin films from the molecular hydrogen bonding configurations of their surface OH groups; namely, fHB and its ft and fg components or vice versa with an accuracy of >97%.
The
and
of the PVA thin films are determined to be 8.0 mN m−1 and 9.8 mN m−1, which are more than 5 times smaller than the
of the surface OHf groups (50 mN m−1) and comparable to that of weak polar groups, thus highlighting the surprisingly weak interactions of the OHHB groups with water molecules. This impact of hydrogen bonding on hydration may be generally expected for polar groups, such as OH, NH2 and COOH groups bearing both proton acceptors and donors. On the one hand, it is unfavourable for hydrophilic coatings to achieve peculiar surface functionality such as anti-fog, where effective surface hydration is essential to promote aggressive water wetting.50 On the other hand, it is favourable for hydrogels, where robust hydrogen bonding is necessary to enhance the mechanical strength.51 Taken together, the present work may offer a technical framework to thermodynamically differentiate the hydrogen bonding of polar groups with themselves from that with water molecules, which will serve as a quantitative guidance to describe and design not only the surface hydration efficiency and water wettability of hydrophilic polymer surfaces, but also the self-organization behaviour of hydrophilic polymers in water.
Author contributions
Z. G.: writing – original draft, methodology, investigation. Z. M.: writing – review & editing, investigation, formal analysis, supervision. D. W.: writing – review & editing, supervision, project administration, funding acquisition, formal analysis, conceptualization. All authors have approved the final version of the manuscript.
Data availability
The data supporting this article, including the original SFG spectra, UV-vis spectra, ATR-FTIR spectra, and XPS spectra, original AFM images, and original SEM images, have been included in the ESI.†
Conflicts of interest
There are no conflicts to declare.
Acknowledgements
This research is funded by the National Natural Science Foundation of China (grant no. 21932003 and 22161132009).
References
- Y. Q. Xia, V. Adibnia, R. Huang, F. Murschel, J. Faivre, G. J. Xie, M. Olszewski, G. De Crescenzo, W. Qi, Z. M. He, R. X. Su, K. Matyjaszewski and X. Banquy, Angew. Chem., Int. Ed., 2019, 131, 1322–1328 CrossRef.
- A. B. Asha, Y. J. Chen and R. Narain, Chem. Rev., 2021, 50, 11668–11683 CAS.
- D. Hetemi and J. Pinson, Chem. Rev., 2017, 46, 5701–5713 CAS.
- S. F. Zhao and J. H. Ahn, Mater. Sci. Eng., R, 2022, 148, 100672 CrossRef.
- A. Abbas, C. Zhang, M. Asad, A. Waqas, A. Khatoon, S. Hussain and S. H. Mir, Polymers, 2022, 14, 238 CrossRef CAS PubMed.
- K. Chaudhuri and J. T. Pham, Soft Matter, 2022, 18, 3698–3704 RSC.
- M. S. Ganewatta, Z. Wang and C. Tang, Nat. Rev. Chem., 2021, 5, 753–772 CrossRef CAS PubMed.
- Q. Tao, S. Huang, X. Li, X. F. Chu, X. L. Lu and D. Y. Wang, Angew. Chem., Int. Ed., 2020, 59, 14466 CrossRef CAS PubMed.
- R. Wang, C. L. Cheng, H. Y. Wang, Q. Tao and D. Y. Wang, Adv. Funct. Mater., 2023, 33, 2301085 CrossRef CAS.
- Y. X. Zhu, G. R. Guo, J. C. Lu, C. S. Ye, Y. Q. Xie, Y. H. Lu and S. Tu, Chem. Eng. J., 2024, 496, 153773 CrossRef CAS.
- S. Huang and D. Y. Wang, Angew. Chem., Int. Ed., 2017, 56, 1–6 CrossRef.
- X. Su, D. Z. Hao, Z. N. Li, X. L. Guo and L. Jiang, J. Mater. Chem. B, 2019, 7, 1322–1332 RSC.
- S. Chernyy, M. Järn, K. Shimizu, A. Swerin, S. U. Pedersen, K. Daasbjerg, L. Makkonen, P. Claesson and J. Iruthayaraj, ACS Appl. Mater. Interfaces, 2014, 6, 6487 CrossRef CAS PubMed.
- S. H. Lee and E. Ruckenstein, J. Colloid Interface Sci., 1987, 120, 529 CrossRef.
- P. Guo, Y. S. Tu, J. R. Yang, C. L. Wang, N. Sheng and H. P. Fang, Phys. Rev. Lett., 2015, 115, 186101 CrossRef PubMed.
- T. Sun, G. Wang, L. Feng, B. Liu, Y. Ma, L. Jiang and D. Zhu, Angew. Chem., Int. Ed., 2004, 43, 357–360 CrossRef CAS PubMed.
- Z. Y. Ma and D. Y. Wang, Colloids Surf., A, 2023, 675, 132031 CrossRef CAS.
- X. K. Liu, C. L. Leng, L. Yu, K. He, L. J. Brown, Z. Chen, J. Cho and D. Y. Wang, Angew. Chem., Int. Ed., 2015, 54, 4851–4856 CrossRef CAS PubMed.
- Z. Y. Ma, C. L. Cheng, Q. H. Cheng, R. Wang, Z. H. Guo and D. Y. Wang, Colloids Surf., A, 2024, 694, 134108 CrossRef CAS.
- M. Kozlov and T. J. McCarthy, Langmuir, 2004, 20, 9170–9176 CrossRef CAS PubMed.
- N. B. Halima, RSC Adv., 2016, 6, 39823–39832 RSC.
- H. K. Chang, C. W. Huang, C. C. Chiu, H. J. Wang and P. Y. Chen, Macromolecules, 2020, 53, 8809–8818 CrossRef CAS.
- Y. Liu, J. Y. Yin, Y. B. Fu, P. P. Zhao, Y. H. Zhang, B. Q. He and P. X. He, Chem. Eng. J., 2020, 382, 122925 CrossRef CAS.
- J. B. Fan, Y. Y. Song, S. T. Wang, J. X. Meng, G. Yang, X. L. Guo, L. Feng and L. Jiang, Adv. Funct. Mater., 2015, 25, 5368–5375 CrossRef CAS.
- Z. X. Bai, K. Jia, C. C. Liu, L. L. Wang, G. Lin, Y. M. Huang, S. N. Liu and X. B. Liu, Adv. Funct. Mater., 2021, 31, 2104701 CrossRef CAS.
- J. Lin, Z. Yao, M. M. Xiong, F. Hu, X. C. Wei and S. Y. Huang, Adv. Compos. Hybrid Mater., 2023, 6, 193 CrossRef CAS.
- Q. He, Y. Zhong, J. W. Li, S. M. Chai, Y. Q. Yang, S. Q. Liang, Z. Chang, G. Z. Fang and A. Q. Pan, Adv. Energy Mater., 2024, 14, 2400170 CrossRef CAS.
- Y. G. Wu, C. H. Xue, X. J. Guo, M. C. Huang, H. D. Wang, C. Q. Ma, X. Wang and Z. Y. Shao, Chem. Eng. J., 2023, 471, 144313 CrossRef CAS.
- C. Huang, M. T. Fu, L. Ma, C. K. Mao, Y. Y. Yao, X. Xu, L. Xu, J. G. Han, X. G. Xue, G. Xu, M. H. Wu, H. Y. Shao and H. J. Ma, Chem. Eng. J., 2023, 474, 145718 CrossRef CAS.
- H. Lee, M. L. Alcaraz, M. F. Rubner and R. E. Cohen, ACS Nano, 2013, 7, 2172–2185 CrossRef CAS PubMed.
- H. Q. Zou, X. T. Meng, X. Zhao and J. S. Qiu, Adv. Mater., 2023, 35, 2207262 CrossRef CAS PubMed.
- Y. J. Gu, J. H. Yang and S. X. Zhou, J. Mater. Chem. A, 2017, 5, 10866–10875 RSC.
- W. Zhang, Z. N. Zhang and X. P. Wang, J. Colloid Interface Sci., 2009, 333, 346–353 CrossRef CAS PubMed.
- W. Zhang, Y. J. Fang and X. P. Wang, J. Membr. Sci., 2007, 303, 173–182 CrossRef CAS.
- M. C. Popescu, Int. J. Biol. Macromol., 2017, 101, 783–790 CrossRef CAS PubMed.
- B. Zuo, Y. Y. Hu, X. L. Lu, S. X. Zhang, H. Fan and X. P. Wang, J. Phys. Chem. C, 2013, 117, 3396–3406 CrossRef CAS.
- R. Paneru, S. H. Ki, P. Lamichhane, L. N. Nguyen, B. C. Adhikari, I. J. Jeong, S. Mumtaz, J. Choi, J. S. Kwon and E. H. Choi, Appl. Surf. Sci., 2020, 532, 147339 CrossRef CAS.
- Y. Liu, H. Y. Hu, X. L. Yang, J. Lv, L. Zhou and Z. K. Luo, Biomed. Mater., 2019, 14, 055009 CrossRef CAS PubMed.
- M. Fowkes, J. Ind. Eng. Chem., 1960, 12, 40–52 Search PubMed.
- S. W. Wu, M. T. Hua, Y. Alsaid, Y. J. Du, Y. F. Ma, Y. S. Zhao, C. Y. Lo, C. R. Wang, D. Wu, B. W. Yao, J. Strzalka, H. Zhou, X. Y. Zhu and X. M. He, Adv. Mater., 2021, 33, 2007829 CrossRef CAS PubMed.
- R. Kurapati and U. Natarajan, Ind. Eng. Chem. Res., 2020, 59, 16099–16111 CrossRef.
- K. Masuda and F. Horii, Macromolecules, 1998, 31, 5810 CrossRef CAS.
- K. Masuda, H. Kaji and F. Horii, J. Polym. Sci., Polym. Phys. Ed., 2000, 38, 1 CrossRef CAS.
- G. M. Whitesides, P. E. Laibinis and J. P. Folkers, Langmuir, 1992, 8, 1330–1341 CrossRef.
- S. Han, Y. M. Luan, S. F. Pang and Y. H. Zhang, Spectrochim. Acta, Part A, 2015, 139, 37–42 CrossRef CAS PubMed.
- E. G. Kononova, M. N. Rodnikova, I. A. Solonina and E. V. Shirokova, Russ. J. Phys. Chem. A, 2020, 94, 2233–2237 CrossRef CAS.
- S. Hassen, H. Chebbi, M. F. Zid and Y. Arfaoui, J. Mol. Struct., 2019, 1179, 678–684 CrossRef CAS.
- Z. Z. Fu, S. J. Guo, C. X. Li, K. Wang, Q. Zhang and Q. Fu, Phys. Chem. Chem. Phys., 2022, 24, 1885–1895 RSC.
- P. Pruettiangkura, S. Ho and M. Schwartz, Spectrosc. Lett., 1979, 12, 679–685 CrossRef CAS.
- H. Lee, M. L. Alcaraz, M. F. Rubner and R. E. Cohen, ACS Nano, 2013, 3, 2172–2185 CrossRef PubMed.
- S. Wu, M. Hua, Y. Alsaid, Y. Du, Y. Ma, Y. Zhao, C. Lo, C. Wang, D. Wu, B. Yao, J. Strzalka, H. Zhou, X. Zhu and X. He, Adv. Mater., 2021, 33, 2007829 CrossRef CAS PubMed.
|
This journal is © The Royal Society of Chemistry 2025 |
Click here to see how this site uses Cookies. View our privacy policy here.