Mechanically processed Sardinian wool promotes C–C bond synthesis under solvent-free conditions†
Received
21st August 2024
, Accepted 6th November 2024
First published on 7th November 2024
Abstract
The tangible environmental concerns of the last few decades are prompting science to a new sustainable paradigm for chemical reactions and a circular economy. In the present manuscript, complying with this dichotomy, Sardinian wool is presented as a promising material for organic synthesis. Aldol and nitro-aldol additions, and Knoevenagel reactions were demonstrated with high efficiency under wool-promoted and solvent-free conditions, paving the way to novel approaches for converting wool biomass waste into value-added products for C–C bond formation.
Sustainability spotlight
Our research on Sardinian wool aligns with the goal of a carbon-neutral society by repurposing an undervalued natural resource into sustainable materials. Wool, as a biodegradable and renewable resource, offers an eco-friendly alternative to synthetic fibers and energy-intensive materials. By focusing on the development of low-impact applications, such as barely solvent-free pre-treatments and solvent-less reactions, we aim to reduce the reliance of organic chemistry on toxic, expensive and/or volatile catalysts and chemicals. This approach not only supports waste reduction but also leverages local resources, contributing to regional economic sustainability. Our work addresses, directly and indirectly, some UN sustainable development goals: industry, innovation, and infrastructure (SDG 9), responsible consumption and production (SDG 12), and climate action (SDG 13).
|
Introduction
The increasing need for environmental preservation has highlighted the importance of sustainable practices in chemistry, leading to the development of green chemistry.1 This field, governed by 12 principles,2 emphasizes the use of renewable feedstocks to reduce reliance on non-renewable resources.3 Green chemistry aims to design chemical products and processes that minimize the use and generation of hazardous substances.4 The valorisation of plant- and animal-based waste, then, has gained significant attention as a means to enhance recyclability and achieve long-term sustainability.5
According to the Global Waste Management Outlook (GWMO), the total municipal solid waste generated globally in 2023 was approximately 2.1 billion tons, originating primarily from household activities, agricultural operations, and industrial and construction activities.6 Converting this waste into value-added products is crucial for addressing environmental concerns and promoting sustainability.7 Effective waste reduction strategies, improved recycling technologies, and robust policies are essential to promote sustainable consumption and production practices. Within this context, reusing waste within a circular economy framework, such as that of the European Union, is key to extending the life cycle of materials and products through practices like sharing, leasing, reusing, repairing, refurbishing, and recycling.8
Wool, a natural polymer derived from sheep, is valued for its unique mechanical and physical properties.9 It primarily consists of keratin, a protein rich in amino acids like cystine, which imparts resilience and desirable physical and mechanical properties.10 The disposal of wool waste, regulated by EU directives, poses significant challenges,11 especially in regions like Sardinia, which has a high sheep population and generates substantial wool waste.12
Recent research has explored using natural fibres as a support for catalysis in various reactions.13 With regards to wool, its amino acid composition and isoelectric point make it suitable for binding metal cations, facilitating its use in metal-based catalytic processes.14 For instance, metal catalysts based on Pd, Fe, Cu, and Mn supported on wool have been successfully utilized in C–C/C–N cross-coupling, desulfuration coupling, and oxidation reactions.15 However, these methodologies often relied on chemical treatment of the fibres and on toxic solvents, such as DMSO and TBAB, which limit their alignment with green chemistry principles.16 Addressing these limitations is crucial for developing truly sustainable catalytic processes.
Inspired by the catalytic activity of amino acids and proteins in aldol and nitroaldol reactions,17 we envisaged amino acid-containing fibers as potent promoters of organic transformations.
In this study, we report using Sardinian wool powder as a sustainable and efficient promoter for aldol, nitroaldol, and Knoevenagel reactions. By employing a ball milling device, a consolidated enabling technology for green chemistry applications,18 to enhance surface area and catalytic activity, our approach aligns with the principles of green chemistry by operating under solvent-free conditions during the reaction. This approach valorizes wool waste and contributes to the circular economy of Sardinia, transforming waste into a valuable material for catalysis.
Results and discussion
FT-IR analysis
All the wool samples subjected to different treatments were analysed using FT-IR spectroscopy. Spectrum A (Fig. 1) corresponds to the black wool samples, whereas Spectrum B (Fig. 1) corresponds to the white wool samples. In Spectrum A, the FT-IR data for black wool fiber (BWF), black wool powder (BWP), and black wool powder treated with 10% HCl solution (BWP(HCl)) have been compared. In Spectrum A, we observed a strong band at 1657 cm−1 for C
O stretching frequency in all the black wool samples, indicating the presence of α-helix structures. Furthermore, at 1543 cm−1, peaks were observed, particularly Amide-II bands of noncyclic secondary amides arising from the combined effect of N–H bending and C–N stretching frequencies. A strong band was also observed at 1635 cm−1 corresponding to Amide-I C
O stretching frequency for β-pleated sheets. These observations indicate the presence of both α-helix and β-pleated sheets in the black wool samples. Among all the black wool samples, these peaks remain unchanged, indicating the retention of the secondary structure in the wool samples after mechanical treatment (milling in ball mills) and chemical treatment (treatment with acid). In Spectrum B, we observed similar outcomes in FT-IR analysis. Herein, the Amide-I C
O stretching frequency for α-helix structures was observed at 1540 cm−1, and the Amide-I C
O stretching frequency for β-pleated sheets was observed at 1635 cm−1. These wool samples were also resistant to changes in secondary structures upon mechanochemical and chemical treatments.
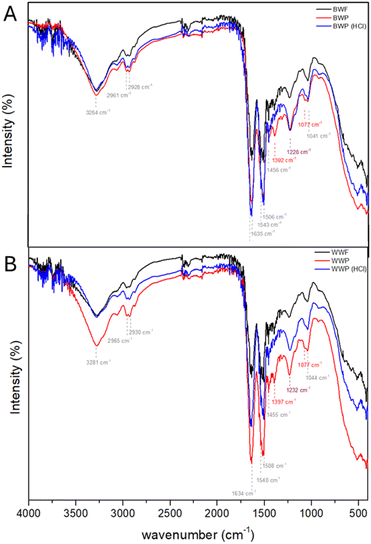 |
| Fig. 1 FT-IR spectra of wool samples. Spectrum A: black wool fiber (BWF), black wool powder (BWP), and acid-treated black wool powder (BWP(HCl)). Spectrum B: white wool fiber (WWF), white wool powder (WWP), and acid-treated white wool powder (WWP(HCl)). | |
Aldol reactions
We commenced our investigation on the evaluation of wool performances by testing a model reaction. We selected the aldol addition between cyclopentanone 1a and 4-nitro benzaldehyde 2a to obtain the aldol product 3aa in the presence of white wool powder. The best conditions were found to be as follows: 2a (0.20 mmol), 1a (1.00 mmol), water (98 μL), and 50 mg of white wool powder gently stirred at r.t. for seven days (98% yield, Table 1, entry 1). Deviations from the standard conditions, involving chicken feather powder (FP), are reported in Table 1 and discussed below.
Table 1 Optimization and screening of the keratin-catalyzed aldol reaction
Initially, we fine-tuned the best stoichiometric ratio for the aldol reactions, which we found to be good in 10-fold excess of the ketone (entry 2, Table 1). However, since no significant decrease in yield was observed by halving this amount, we opted for a 1
:
5 ratio for waste prevention purposes (entry 1, Table 1). Further reduction in the amount of ketone resulted in poor results compared to the previously mentioned ones. Notably, decreasing the amount of white wool powder from 50 mg to 25 mg resulted in a substantial decrease in conversion into 3a from 98% to a mere 47% (entry 3, Table 1), suggesting that 50 mg of WWP is the minimum amount that is needed for this kind of chemical transformation. Comparable results were obtained when white wool powder was replaced by black wool powder or chicken feather powder, further supporting the role of keratin powder in the reaction (entries 4 and 5, Table 1). However, with the use of WWP(HCl) instead of WWP, the yield of product 3a saw a remarkable drop (entry 6, Table 1), suggesting that side chains containing ionizable N-residues are crucial for the reaction to occur, further validating the mechanism via enamine catalysis. With the reduction of reaction time from 7 days to 4 days, the conversion of product 3a decreased to 61% (entry 7, Table 1). Furthermore, the reaction in the presence of WWF instead of WWP had inferior yields compared to WWP (entry 8, Table 1), suggesting the significance of the mechanical treatment of wool fibers. This can be explained by the increased active sites and surface area generated upon grinding the keratin fibres. At the same time, the primary and secondary structures of the protein are preserved, as shown in the IR spectra of the treated samples (Fig. 1). Finally, different solvent conditions and a controlled temperature of 40 °C did not provide any significant advantage (entries 9–13, Table 1), likely due to some a hydrophilic–hydrophobic balance between wool powder and reagents.
Based on the standard optimized conditions, we decided to test the reaction against differently substituted benzaldehydes. Compounds with electron-withdrawing groups and heterocyclic aldehyde, as in the case of 2a–c and 2m, reacted smoothly to provide excellent yields (85–98%, Scheme 1A). Halogen-bearing benzaldehydes like 2d, 2e, and 2f provided the corresponding products in yields ranging from acceptable to good (30–73%, Scheme 1A). Additionally, the effect of the position of the electron-withdrawing group on the benzaldehyde ring from para to meta positions yielded comparable yields. However, in the case of aldehyde 2e, we observed a significant drop in yield compared to its para-substituted counterpart with 2d. Notably, benzaldehydes substituted with electron-donating residues, namely 2g and 2h, provided the aldol product in acceptable yields (43% and 40%, respectively, Scheme 1A). Lastly, ketones 1b and 1c reacted with 4-nitrobenzaldehyde 2a to obtain the corresponding products 3ba and 3ca in excellent yields (96% and 83%, respectively, Scheme 1A). Comparing the reactions performed on black wool, white wool, and feather powders, we achieved almost similar yields and diastereomeric ratio outcomes. Notably, in the case of benzaldehydes 2a and 2i, an inverted diastereomeric ratio syn/anti = 1
:
0.6 was obtained when using black wool powder instead of white wool powder. Lastly, the present reaction was optimized for gram scale synthesis, and the recyclability of wool was assessed, showing that the material can be reused for up to 5 complete cycles despite a progressive loss in yield and material, likely due to material poisoning.
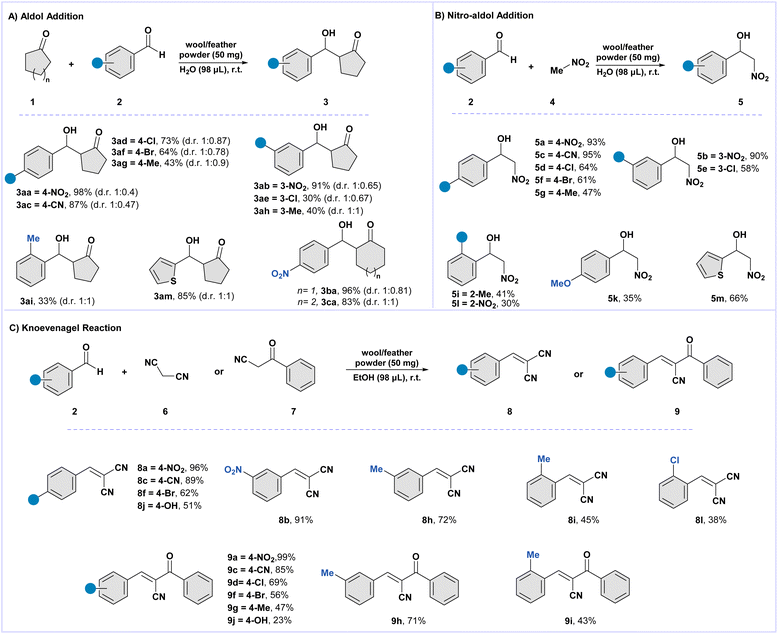 |
| Scheme 1 Reaction scope for wool-catalyzed organic reaction models. Section 1A: the aldol reaction scope. Section 1B: the nitro-aldol reaction scope. Section 1C: the Knoevenagel reaction scope. | |
Nitro-aldol reactions
Based on our investigation regarding the aldol reaction, we investigated the nitro-aldol response using the same 4-nitro benzaldehyde 2a and nitromethane 4 as the model substrates. Through the initial screening, we found that the reaction gave the best results with a 10-fold excess of nitromethane compared to benzaldehyde to achieve the nitro–aldol product after 24 h. A library of compounds (5a–5m, 30–95%) was synthesized under the optimized conditions (Scheme 1B). The reactions provided excellent yields in the presence of electron-withdrawing groups on the benzaldehyde ring (2a–f). With the substitution at the meta position, we obtained only a slight decrease in yields compared to the para-substituted benzaldehydes. Black wool and chicken feathers were also used to assess the products, which showed comparable outcomes. The kinetic trend of this reaction was obtained by GC-MS, providing the kinetic curve in Fig. 2 below.
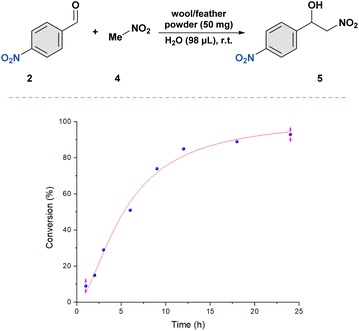 |
| Fig. 2 Reaction rate followed for the nitro-aldol reaction under wool-catalyzed conditions. GC-MS was used to calculate the conversion (%). | |
Knoevenagel reaction
Under our previously optimized conditions, we decided to focus on the Knoevenagel reaction using 4-nitro benzaldehyde 2a and dicyanomalonate 6 in the presence of WWP. Notably, the response was investigated using a stoichiometric ratio of the two reagents to avoid any purification process involving column chromatography or crystallization of the reaction mixture. This entailed extending the reaction time up to 14 days for completion, however remarkably improving the waste and toxicity prevention. Since the aldol adduct intermediate must undergo a dehydration process to form the π bond, a different solvent in place of H2O has been investigated. The solvent screening is reported below in Table 2. We found a good compromise between efficiency and greenness with EtOH (entry 5, Table 1), so all the reactions were carried out in the presence of 98 μL of EtOH. Generally, polar solvents work better than apolar ones; specifically, polar protic solvents lead to higher product yields of 8a.
Table 2 Optimization and screening of the keratin-catalyzed nitro-aldol reaction
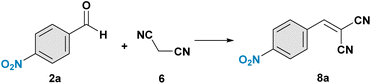
|
Entry |
Solvent |
8a (NMR yield)a |
Reaction conditions: 2a (0.20 mmol), 6 (0.2 mmol), solvent (98 μL) and 50 mg of white wool powder were gently stirred at r.t. for 14 days. NMR yield was calculated by using trimethoxybenzene as an internal standard.
|
1 |
Hexane |
23% |
2 |
Toluene |
20% |
3 |
Distilled H2O |
8% |
4 |
AcOEt |
73% |
5 |
MeOH |
96% |
6 |
Absolute EtOH |
98% |
7 |
2-MeTHF |
58% |
Based upon the optimized conditions, the reaction scope was extended to differently substituted benzaldehydes. Compounds bearing electron-withdrawing groups, as in the case of 2a–c, provided excellent yields of products 8a–c (87–98%, Scheme 1C). Benzaldehydes having halogens, like 2f and 2l, provided the corresponding products 8f and 8l in excellent and acceptable yields (62% and 38%, respectively, Scheme 1C). Instead, benzaldehydes bearing electron-donating residues, namely 2h–j, provided the aldol product in good yields (45–51%, Scheme 1C). A similar reaction trend was observed when benzoyl acetonitrile seven was used instead of malononitrile. A broad library of compounds was obtained in yields ranging from excellent to poor (9a–j, 23–99%, Scheme 1C). The reactions with meta-substituted benzaldehydes (2b and 2h) resulted in yields comparable to those of their para-substitution counterpart. In the case of ortho-substituted benzaldehyde (2i and 2l), we observed a significant decrease in the formation of the desired products.
Experimental
Materials and methods
All components of the wool samples were collected from the Sardinian province of Italy. White wool fibers were collected from the city of Ittiri in the northern region of Sardinia, while black wool fibers were obtained from Guspini, a town in the Sardinian inland. The wool was procured from the Sechi farm, ensuring full compliance with animal welfare standards. Chicken feathers were obtained from the Concimi-Bio company. All the wool fibers and chicken feathers were obtained as raw materials and then treated in our laboratory. All reagents and solvents were purchased from Sigma-Aldrich, Fluorochem, and TCI. Dimethyl sulfoxide-d6 (99.9% D) and chloroform-d (99.9% D) were also purchased from Sigma-Aldrich and TCI. All purchased chemicals were used without further purification.
Wool and feather sample preparation
The wool samples were prepared using the following procedure: the raw material was thoroughly rinsed and washed with tap water and soap to eliminate hydrophilic impurities. Afterward, the fibers were rinsed with heptane and then with acetone to remove the hydrophobic impurities. After several washes, the fibers were dried overnight inside an oven. To prepare the acidic wool samples, 10 g of washed wool fibers were placed inside a beaker containing 500 mL of HCl solution (10%) and left untouched for 72 hours. Afterward, the treated fibers were washed with regular tap water and dried overnight in an oven. The dried long fibers have been finely cut into 1 cm pieces to obtain the desired wool fiber (WF) size. The cut fibers were ground with ball mills using zirconia jars and balls. The best results in terms of finer powder were achieved using a Fritsch P7 Premium planetary ball mill (3.00 g of cut fiber inside a 20 mL zirconia jar with 35 balls with diameter Φ = 5 mm and mtot = 13.75 g). After grinding, black wool powder (BWP) and white wool powder (WWP) have been obtained. Wool powders were also obtained from the hydrochloric acid-treated wool fibers (BWP(HCl) and WWP(HCl)) using the same ball milling methodology.
General procedure for synthesis of nitro-aldol compounds
Inside a 4 mL glass vial equipped with a magnetic stirrer, aromatic aldehyde (0.20 mmol), nitromethane (10 equiv.), and 50 mg of wool powder were placed in 98 μL of distilled water. When the reaction was completed, mixture was gently stirred, and the reaction progress was monitored using TLC (Heptane/AcOEt 1
:
1). The product was recovered by implementing simple filtration using AcOEt with a paper filter. After that, the solvent was evaporated under reduced pressure to obtain the pure products.
General procedure for the synthesis of aldol compounds
Inside a 4 mL glass vial with a magnetic stirrer, aldehyde (0.20 mmol), ketone (5 equiv.), and 50 mg of wool powder were placed in 98 μL of distilled water. The mixture was gently stirred, and the reaction progress was monitored using TLC (Heptane/AcOEt 5
:
1) until completion. The product was recovered by implementing simple filtration using AcOEt with a paper filter. After that, the solvent was evaporated under reduced pressure to obtain the pure products.
General procedure for the synthesis of Knoevenagel compounds
Inside a 4 mL glass vial equipped with a magnetic stirrer, aldehyde (0.20 mmol), malononitrile or benzoyl acetonitrile (0.20 mmol), and 50 mg of wool powder were placed in 98 μL of absolute ethanol. The mixture was gently stirred, and the reaction progress was monitored using TLC (Heptane/AcOEt 5
:
1) until completion. The product was recovered by implementing simple filtration using AcOEt with a paper filter. After that, the solvent was evaporated under reduced pressure to obtain the pure products.
Conclusions
In conclusion, this investigation aimed to test the potential of Sardinian wool as a renewable and sustainable catalyst for various organic reactions. Derived from its keratin content and functional groups, wool's features confer a unique advantage in heterogeneous catalysis. Our findings have shown that wool-based catalysts effectively promote aldol and Knoevenagel reactions, highlighting the versatility and efficiency of wool in catalytic applications. The optimization of the reaction conditions revealed that the stoichiometry, the composition of the catalytic support, and mechanical processing significantly impact the catalytic performances. FT-IR analyses confirmed that the structural integrity of wool is preserved across different treatments, thus maintaining the necessary activity for catalytic purposes. Comparative tests with other keratinous materials, such as chicken feathers, suggested a broader applicability of keratin-based catalysts. Moreover, the use of locally sourced wool not only promotes the valorization of what is traditionally considered agricultural waste but also contributes economically and environmentally to local communities in Sardinia, embodying the ideals of the circular economy.
Data availability
The data supporting this article have been included as part of the ESI.†
Author contributions
F. C. wrote the first draft of the article. F. C. and A. P. conceptualized the idea and revised the manuscript's drafts. F. C. and So. B. performed the syntheses and collected the data. F. C., F. B., and St. B. analysed the data. A. P. supervised the work. All the authors contributed to the draft's correction and finalization.
Conflicts of interest
There are no conflicts to declare.
Acknowledgements
This study was carried out within the RETURN Extended Partnership and received funding from the European Union Next-GenerationEU (National Recovery and Resilience Plan – NRRP, Mission 4, Component 2, Investment 1.3 – D.D. 1243 2/8/2022, PE0000005). MASE also provided financial support for the project having grant number F33C23000680001 and MUR with PNRR PE2 – NEST – Network 4 Energy Sustainable Transition – (MUR no. PE0000021, CUP no. F53C22000770007). We acknowledge Prof. Angelo Frongia for having shared his expertise in aldol reactions and the CeSAR (Centro Servizi Ricerca d’Ateneo) core facility of the University of Cagliari, Dr Sandrina Lampis for assistance with the generation of NMR data and Dr. Marco Marceddu for assistance with the generation of SEM images.
Notes and references
-
(a) R. Ratti, SN Appl. Sci., 2020, 2, 1–7 CrossRef
;
(b) I. Eilks and F. Rauch, Chem. Educ. Res. Pract., 2012, 13, 57–58 RSC
;
(c) P. Dunn, Chem. Soc. Rev., 2012, 41, 1452–1461 RSC
;
(d) M. Kirchhoff, Resour., Conserv. Recycl., 2005, 44, 237–243 CrossRef
;
(e) R. Cioc, E. Ruijter and R. Orru, Green Chem., 2014, 16, 2958–2975 RSC
;
(f) M. Kidwai and R. Thakur, J. Indian Chem. Soc., 2005, 82, 1103–1111 CAS
;
(g)
R. Lankey and P. Anastasin Advancing Sustainability through Green Chemistry and Engineering, ed. R. Lankey and P. Anastas, ACS Symposium Series, 2002, vol. 823 CrossRef
;
(h) M. Karpudewan, Z. Ismail and W.-M. Roth, Chem. Educ. Res. Pract., 2012, 13, 120–127 RSC
;
(i) P. Anastas and N. Eghbali, Chem. Soc. Rev., 2010, 39, 301–312 RSC
.
-
(a) H. C. Erythropel, J. Zimmerman, T. Winter, L. Petitjean, F. Melnikov, C. Lam, A. Lounsbury, K. Mellor, N. Z. Janković, Q. Tu, L. N. Pincus, M. Falinski, W. Shi, P. Coish, D. Plata and P. Anastas, Green Chem., 2018, 20, 1929–1961 RSC
;
(b) A. Ivanković, A. Dronjić, A. Bevanda and S. Talić, Int. J. Lit. Arts, 2017, 6, 39 Search PubMed
;
(c) P. Jessop, S. Trakhtenberg and J. Warner, ACS Symp. Ser., 2009, 1000, 401–436 CrossRef CAS
;
(d) F. Casti, F. Basoccu, R. Mocci, L. De Luca, A. Porcheddu and F. Cuccu, Molecules, 2022, 27, 1988 CrossRef CAS PubMed
.
-
(a) J. Clark, V. Budarin, F. Deswarte, J. J. E. Hardy, F. M. Kerton, A. J. Hunt, R. Luque, D. Macquarrie, K. Milkowski, A. Rodríguez, O. Samuel, S. Tavener, R. J. White and A. J. Wilson, Green Chem., 2006, 8, 853–860 RSC
;
(b) P. Gallezot, Green Chem., 2007, 9, 295–302 RSC
;
(c) R. Höfer and J. Bigorra, Green Chem. Lett. Rev., 2008, 1, 79–97 CrossRef
;
(d) V. Gude and E. Martínez-Guerra, Environ. Chem. Lett., 2018, 16, 327–341 CrossRef CAS
;
(e) N. Dimitratos, J. Lopez-Sanchez and G. Hutchings, Top. Catal., 2009, 52, 258–268 CrossRef CAS
.
-
(a) T. Wale, S. Suryavanshi, V. Wavare, P. Phalke and M. N. Sharmale, J. Drug Deliv. Ther., 2023, 13, 1–10 Search PubMed
;
(b) A. Erdem Yayayürük and O. Yayayürük, Curr. Anal. Chem., 2019, 15, 745–758 CrossRef
;
(c) R. Varma, Green Chem. Microwave Energy, 2013, 115–156 Search PubMed
;
(d) A. Das, A. Sadhukhan, S. Chakraborty, S. Bhattacharya, A. Roy and A. Bhattacharjee, Int. J. Res. Appl. Sci. Eng. Technol., 2022, 10, 1234–1245 CrossRef
.
-
(a) D. Hidalgo, F. Corona, J. Martín-Marroquín, M. Gomez, A. Aguado and G. Antolín, Environ. Eng. Manag. J., 2014, 13, 2467–2475 CrossRef
;
(b) J. Sadhukhan and E. Martinez-Hernández, Bioresour. Technol., 2017, 243, 135–146 CrossRef CAS PubMed
;
(c) R. Kothari, A. Singh, A. Pandey, V. Tyagi, D. Egamberdieva, S. Bellingrath-Kimura and N. Arora, Environ. Sustain., 2021, 4, 199–200 CrossRef
;
(d) C. Caldeira, A. Vlysidis, G. Fiore, V. De Laurentiis, G. Vignali and S. Sala, Bioresour. Technol., 2020, 312, 123575 CrossRef CAS
;
(e) Q. Jin, L. Yang, N. E. Poe and H. Huang, Trends Food Sci. Technol., 2018, 74, 119–131 CrossRef CAS
;
(f) T. H. Kwan, Y. Hu and C. Lin, J. Clean. Prod., 2018, 181, 72–87 CrossRef CAS
;
(g) T. Vandermeersch, R. Alvarenga, P. Ragaert and J. Dewulf, Resour. Conserv. Recycl., 2014, 87, 57–64 CrossRef
;
(h) S. Marcelino, P. D. Gaspar and A. Paço, Sustainability, 2023, 15, 13333 CrossRef
;
(i) W.-T. Tsai and Y.-Q. Lin, Fermentation, 2021, 7, 51 CrossRef
;
(j) S. Ben-Othman, I. Jõudu and R. Bhat, Molecules, 2020, 25, 510 CrossRef CAS PubMed
.
-
https://www.unep.org/ietc/resources/report/global-waste-management-outlook-2024, Last access to the website: 25/07/2024.
-
(a) R. Sindhu, E. Gnansounou, S. Rebello, P. Binod, S. Varjani, I. Thakur, R. B. Nair and A. Pandey, J. Environ. Manag., 2019, 241, 619–630 CrossRef
;
(b) G. Albor, A. Mirkouei and E. Struhs, J. Manuf. Sci. Eng., 2022, 27, 1–11 Search PubMed
;
(c) C. R. Chilakamarry, A. M. Sakinah, A. Zularisam, R. Sirohi, I. A. Khilji, N. Ahmad and A. Pandey, Bioresour. Technol., 2021, 343, 126065 CrossRef PubMed
.
-
(a) C. Hepburn,
et al.
, Nature, 2019, 575, 87–97 CrossRef CAS PubMed
;
(b) Q. Zhu, C. Bai and J. Sarkis, Transp. Res. E Logist. Transp. Rev., 2022, 164, 102824 CrossRef
;
(c) Z. Huang, R. G. Grim, J. A. Schaidle and L. Tao, Energy Environ. Sci., 2021, 14, 3664–3678 RSC
;
(d) P. Kowalski and C. Legendre, Resour. Conserv. Recycl., 2021, 173, 105694 CrossRef
;
(e) K. De Kleijne,
et al.
, One Earth, 2022, 5, 168–185 CrossRef
.
-
(a) T. Bouagga, T. Harizi, F. Sakli and M. Zoccola, J. Nat. Fibers, 2020, 17, 28–40 CrossRef CAS
;
(b) K. Ballagh, Appl. Acoust., 1996, 48, 101–120 CrossRef
;
(c) D. Kaur and O. Singh, Asian J. Dairy Food Res., 2007, 26, 187–189 Search PubMed
.
-
(a) H. Rajabinejad, M. Zoccola, A. Patrucco, A. Montarsolo, G. Rovero and C. Tonin, Text. Res. J., 2018, 88, 2415–2424 CrossRef CAS
;
(b) J. M. Cardamone, J. Mol. Struct., 2010, 969, 97–105 CrossRef CAS
;
(c) A. Shavandi, T. Silva, A. A. Bekhit and A. E. Bekhit, Biomater. Sci., 2017, 5, 1699–1735 RSC
.
-
(a) A. Batričević and N. Paunović, Strani pravni život, 2017, 61(4), 107–122 CrossRef
;
(b) J. Vehlow, B. Bergfeldt, R. Visser and C. Wilén, J. Mater. Cycles Waste Manag., 2007, 9, 130–139 CrossRef CAS
;
(c) O. Väntsi and T. Kärki, J. Mater. Cycles Waste Manag., 2014, 16, 62–72 CrossRef
.
-
(a) D.-Y. Wang, Y.-T. Tang, G. Long, D. Higgitt, J. He and D. Robinson, Waste Manag., 2019, 102, 452–463 CrossRef PubMed
;
(b) E. Iacovidou, D. Ohandja and N. Voulvoulis, Sci. Total Environ., 2012, 423, 1–7 CrossRef CAS PubMed
;
(c) P. S. Bhavsar, M. Zoccola, A. Patrucco, A. Montarsolo, R. Mossotti, G. Rovero, M. Giansetti and C. Tonin, ACS Sustain. Chem. Eng., 2016, 4, 6722–6731 CrossRef CAS
;
(d) E. Vagnoni, C. Carrino, N. DiBenedetto, E. Pieragostini and B. Consenti, Small Rumin. Res., 2016, 135, 85–89 CrossRef
;
(e) A. Atzori, L. Bayer, G. Molle, P. Arca, A. Franca, M. Vannini, G. Cocco, D. Usai, P. Duce and E. Vagnoni, Integr. Environ. Assess. Manag., 2022, 18, 1187–1198 CrossRef PubMed
;
(f) R. Furesi, F. Madau and P. Pulina, Agric. Food Econ., 2013, 1, 1–11 CrossRef
.
-
(a) R. Ross, C. Fairbridge and J. R. Maccallum, Carbon, 1985, 23, 209–213 CrossRef CAS
;
(b) D. Durkin, T. Ye, E. G. Larson, L. Haverhals, K. Livi, H. Long, P. Trulove and D. Fairbrother, ACS Sustain. Chem. Eng., 2016, 4, 5511–5522 CrossRef CAS
;
(c) X.-L. Shi, H. Yang, M. Tao and W. Zhang, RSC Adv., 2013, 3, 3939–3945 RSC
.
-
(a) H. Rajabinejad, M. Zoccola, A. Patrucco, A. Montarsolo, G. Rovero and C. Tonin, Text. Res. J., 2018, 88, 2415–2424 CrossRef CAS
;
(b) J. M. Cardamone, J. Mol. Struct., 2010, 969, 97–105 CrossRef CAS
;
(c) A. Shavandi, T. Silva, A. A. Bekhit and A. E. Bekhit, Biomater. Sci., 2017, 5, 1699–1735 RSC
.
-
(a) S. McNeil, M. R. Sunderland and S. J. Leighs, Appl. Catal. A Gen., 2017, 541, 120–140 CrossRef CAS
;
(b) S. Ghadamgahi, J. H. Johnston and C. Fonseca-Paris, Nanomaterials, 2018, 8, 621 CrossRef PubMed
;
(c) M.-Y. Yin, G.-L. Yuan, M.-Y. Huang and Y.-Y. Jiang, J. Mol. Catal. A Chem., 1999, 147, 89–92 CrossRef CAS
;
(d) L. Xue, B. Jia, L. Tang, X. F. Ji, M. Y. Huang and Y. Y. Jiang, Polym. Adv. Technol., 2004, 15, 346–349 CrossRef CAS
;
(e) S. Wu, H. Ma, X. Jia, Y. Zhong and Z. Lei, Tetrahedron, 2011, 67, 250–256 CrossRef CAS
.
-
(a) M. Häring, A. Pettignano, F. Quignard, N. Tanchoux and D. Díaz Díaz, Molecules, 2016, 21, 1122 CrossRef PubMed
;
(b) Y. Arakawa and H. Wennemers, ChemSusChem, 2013, 6, 242–245 CrossRef CAS PubMed
;
(c) C. Wu, B. Hu, H. Liu, J. Jiang and J. Kim, Chem. Select., 2022, 7, e202104433 CAS
;
(d) B. Nozière and A. Córdova, J. Phys. Chem. A, 2008, 112, 2827–2837 CrossRef PubMed
.
-
(a) M. Tobiszewski and M. Bystrzanowska, Green Chem., 2020, 22, 7983–7988 RSC
;
(b) C. Chignell, S.-K. Han, A. Mouithys-Mickalad, R. Sik, K. Stadler and M. Kadiiska, Toxicol. Appl. Pharmacol., 2008, 230, 17–22 CrossRef CAS PubMed
;
(c) K. Kim and S.-E. Lee, Chemosphere, 2020, 270, 129405 CrossRef PubMed
;
(d) Q. He, X. Wang, P. Sun, Z. Wang and L.-S. Wang, Aquat. Toxicol., 2015, 164, 145–154 CrossRef CAS PubMed
;
(e) S. Iyer, N. Dhiman, S. P. Zade, S. Mukherjee, N. Singla and M. Kumar, ACS Chem. Neurosci., 2023, 10, 1785–1798 CrossRef PubMed
;
(f) J. Modrzyński, J. H. Christensen and K. Brandt, Ecotoxicol., 2019, 28, 1136–1141 CrossRef PubMed
.
-
(a) F. Basoccu, F. Cuccu, F. Casti, R. Mocci, C. Fattuoni and A. Porcheddu, Beilstein J. Org. Chem., 2022, 18, 732–737 CrossRef CAS PubMed
;
(b) C. Bolm, C. J. Adams, S. L. James, D. Braga, P. Collier, T. Friščić, F. Grepioni, K. D. M. Harris, G. Hyett, W. Jones, A. Krebs, J. Mack, L. Maini, A. G. Orpen, I. P. Parkin, W. C. Shearouse, J. W. Steed and D. C. Waddell, Chem. Soc. Rev., 2012, 41, 413–447 RSC
;
(c) R. R. A. Bolt, J. A. Leitch, A. C. Jones, W. I. Nicholson and D. L. Browne, Chem. Soc. Rev., 2022, 51, 4243–4260 RSC
;
(d) A. Porcheddu, R. Mocci, M. Brindisi, F. Cuccu, C. Fattuoni, F. Delogu, E. Colacino and M. V. D'Auria, Green Chem., 2022, 24, 4859–4869 RSC
;
(e) F. Cuccu, L. De Luca, F. Delogu, E. Colacino, N. Solin, R. Mocci and A. Porcheddu, ChemSusChem, 2022, 15, e20220036 CrossRef PubMed
;
(f) F. Cuccu, D. Browne and A. Porcheddu, ChemCatChem, 2023, 15, e202300762 CrossRef CAS
;
(g) N. Fantozzi, J.-N. Volle, A. Porcheddu and D. Virieux, Chem. Soc. Rev., 2023, 52, 6680–6714 RSC
;
(h) F. Cuccu, F. Basoccu, C. Fattuoni and A. Porcheddu, Green Chem., 2024, 26, 1927–1934 RSC
;
(i) F. Cuccu and A. Porcheddu, Green Chem., 2024, 26, 2684–2691 RSC
;
(j) F. Basoccu, F. Cuccu, P. Caboni, L. De Luca and A. Porcheddu, Molecules, 2023, 28, 2239 CrossRef CAS PubMed
.
|
This journal is © The Royal Society of Chemistry 2025 |
Click here to see how this site uses Cookies. View our privacy policy here.