DOI:
10.1039/D5SU00021A
(Critical Review)
RSC Sustainability, 2025,
3, 1652-1671
Incorporating hyperaccumulating plants in phytomining, remediation and resource recovery: recent trends in the African region – a review
Received
11th January 2025
, Accepted 11th February 2025
First published on 24th February 2025
Abstract
Phytomining, the extraction of valuable metals from soil or waste substrates using plants, has gained increasing attention as a sustainable and economically viable alternative to conventional mining practices. Central to this approach is the use of hyperaccumulating plants, which possess the remarkable ability to uptake and concentrate metals in their biomass. The recognition and use of hyperaccumulating plants have become essential components of phytomining at numerous mine sites around the globe. Metal hyperaccumulators however, suffer setbacks such as low biomass production and limited survival in harsh environments like minefields. These limitations restrict their practical application in real-world settings. This review thus explores the biological, agronomic, and environmental aspects of incorporating hyperaccumulators in phytomining. We discuss key hyperaccumulating species, factors influencing phytomining efficiency, and recent advancements in enhancing biomass yield and metal accumulation in minefields. Additionally, we evaluate the economic and ecological implications of phytomining as a green technology for resource recovery.
Sustainability spotlight
Phytomining of precious metals offers an innovative and sustainable method for resource extraction, utilising hyperaccumulating plants to harvest valuable metals from low-grade ores, such as mine tailings, and contaminated soils. This eco-friendly technology minimizes the ecological impact of traditional mining, aids in land restoration, and supports circular economy practices by recovering resources from unconventional sources. By combining principles of green chemistry with sustainable agricultural techniques, phytomining provides a promising route toward more resilient and ethical precious metal supply chains. This review highlights the significance of incorporating hyperaccumulators in the resource recovery of these high value metals and the potential of phytotechnology as a crucial advancement in sustainable resource management.
|
1. Introduction
The fourth industrial revolution is driving a rapidly growing demand for metals as global economies work to lessen their reliance on fossil fuels. This has in turn increased the depletion rate of the fixed ore source, potentially leading to metal resource scarcity and a looming resource crisis.1,2 Modern industry's increasing need for precious and industrially important/high value metals such as gold (Au), platinum (Pt), palladium (Pd), nickel (Ni), cobalt (Co), silver (Ag), manganese (Mn) among others has led to an increase in mining operations, which has consequently released these metals into the environment. In addition to their vast application in jewellery and ornamental purposes, precious metals as well as high-value metals otherwise identified as critical minerals are essential components for microchips, electronics, computers, cell phones, electric vehicles, solar panels, wind turbines, defence systems and various advanced climate mitigation technologies – clean energy.3,4 Mining and mineral processing produce significant amounts of wastes, as only a small portion of the ore is valuable. As a result, up to 99% of the material ends up as waste, primarily in the form of tailings.5,6 While the exact amount of mine waste is uncertain, it is estimated that global tailings production reached approximately 223 billion metric tons between 1771 and 2019 with the amount growing at a rate of 5–14 billion tons per year via new production.7–10 Recent estimates indicate that global metal mines produce 282.5 billion tons of waste annually, containing valuable metals such as copper (Cu), Au, iron (Fe), lead or zinc (Pb/Zn), Ni and others.11 In addition, it is estimated that South Africa has 17.7 million tons of tailings from gold mining alone.12
A region where mining is the dominant sector, employing nearly half a million people, is Africa.13,14 Notably, South Africa stands out in this regard. South Africa holds the world's largest reserves of platinum, gold, chromium, and vanadium, which are mainly extracted as primary ores from mines across several provinces, including Limpopo, Mpumalanga, and Northwest.15,16 Gold, palladium, platinum, and base metal mining account for over 40% of the nation's economy. However, this industry has also left a legacy of mine tailings that affects various aspects of African society.17 Recent findings indicate that around 239 million hectares of the world's unutilised lands are contaminated.18 The Department of Agriculture and Rural Development in Gauteng, South Africa, reports that toxic mine residue covers an area of 124 square miles.19 These mine tailing dumps are largely contaminated with a range of toxic and valuable metals such as Pb, arsenic (As), thallium (Tl), Ag, cadmium (Cd), mercury (Hg), Ni, Au, Pd, Pt and are strongly acidic, ultimately degrading soil quality.20–23
To address this growing issue, government officials in a number of nations have made the decision to create cutting-edge recycling technologies. One way to capture and recover these metals for economic prospects while revegetating and stabilizing waste areas is through phytomining.24–27 Phytomining is suitable for treating large areas of soil where the metal concentration is too low to be economically viable for recovery through traditional mining methods. The phytomining technology relies on the natural strength of plants to absorb, transport and accumulate metals from low-grade ores, wastes and contaminated soil substrates into their aerial organs for economic benefits.22,28,29 The full potential of phytomining has yet to be realised; achieving this will necessitate integrated, multidisciplinary research that combines soil biochemistry, plant science, microbiology, agricultural management, genetic engineering, environmental science and engineering (Fig. 1).
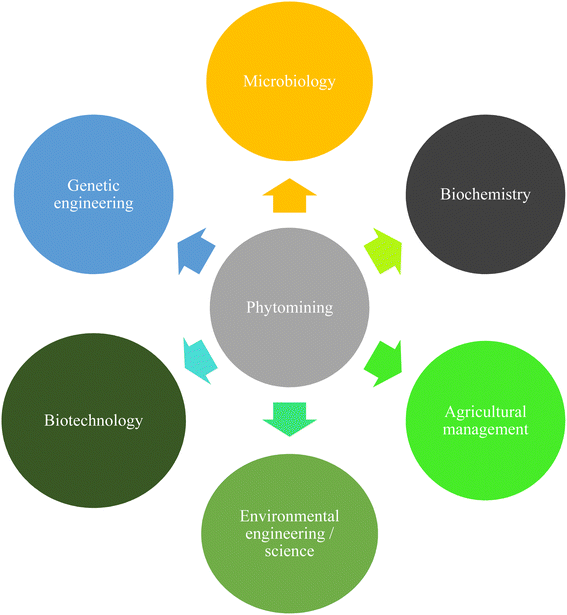 |
| Fig. 1 Potential phytomining research areas. | |
While phytomining is not a substitute for conventional mining, it could serve as a method to harness natural resources that would otherwise remain unutilised.15 The innovative nature and claimed environmental advantages of phytomining have drawn considerable scientific attention. Nonetheless, a primary challenge of using hyperaccumulators for phytoextraction lies in the limited availability of plants with a strong capacity to accumulate the desired metal.6 Some plants however possess remarkable ability to accumulate metals from metal-enriched soils, often ultramafic (serpentine), whether essential or non-essential at concentrations far above the usual levels compared with other plants in the same habitat.30–33 These plants with special traits are otherwise described as hyperaccumulators and the process is termed hyperaccumulation.34,35 Many studies to date have concentrated on laboratory or greenhouse experiments to assess plant performance in phytomining. However, field trials, which more accurately simulate real-world conditions and provide more relevant results for evaluating effectiveness in practical applications, have been sparingly demonstrated. This may be due to the harsh conditions in the mine areas, such as high metal levels and low nutrient availability, which necessitate plants with high biomass, rapid growth, and survival abilities.
In the pursuit of field-applicable plants for phytomining valuable metals, understanding metal uptake and tolerance in plants is crucial. Utilising metal hyperaccumulators could significantly improve the metal mining cycle and make the minerals sector more sustainable. More so, hyperaccumulators have been documented and experimentally verified for elements such as nickel, zinc, cadmium, manganese, arsenic, and selenium (Se). However, the natural hyperaccumulation of gold, palladium, platinum, ruthenium, and silver remains largely unverified. This paper therefore aims to review the previous and current scenario of field and laboratory studies on phytomining. The review provides a comprehensive analysis of the emerging role of hyperaccumulating plants in phytomining, environmental remediation, and resource recovery within the African region—an area that remains underexplored despite its vast metalliferous landscapes. Unlike previous studies that focus primarily on global trends, this manuscript highlights region-specific advancements, challenges, and opportunities in utilising phytotechnologies for sustainable metal extraction and ecosystem restoration. By synthesizing recent developments, it offers new insights into species selection, the ecological implications of phytomining as a green technology, and practical applications tailored to Africa's unique environmental and socio-economic conditions, bridging the gap between research and large-scale implementation. Finally, it discusses recent innovative technologies aimed at enhancing phytomining and examines future prospects.
2. Metal hyperaccumulators
The term “hyperaccumulator” was first introduced in 1976 to describe a plant species capable of naturally accumulating exceptionally high-level concentrations of certain elements—hundreds or even thousands of times higher than those typically observed in plants growing on most common soils.36,37
While hyperaccumulation has been demonstrated in hydroponic systems by cultivating plants in water media with artificially high concentrations of metal salts or other media amended with large amounts of metal salts, these conditions do not fully replicate the complexity of natural soil environments. Such experiments can provide insights into certain aspects of metal uptake behaviour but do not accurately reflect the natural evolution of hyperaccumulation.35 Thus, the criteria for classifying a species as a hyperaccumulator have been defined and refined over time. As such, the widely accepted definition of hyperaccumulators refers to plants that, when growing in their natural environment (as opposed to artificially-amended metal media), contain elemental concentrations exceeding the following thresholds in the dry weight of shoot tissue: 100 μg g−1 for Cd, Tl, or Se; 300 μg g−1 for Co or Cu; 1000 μg g−1 for Ni, As, or Rare Earth Elements (REEs); 3000 μg g−1 for zinc (Zn); 1 μg g−1 for Au and 10
000 μg g−1 for Mn.31,32,35 Alternatively, certain plant species can be induced to accumulate metals through the application of chemicals to the soil (induced hyperaccumulation).38
Hyperaccumulators are required to demonstrate a bioconcentration factor (BF) and translocation factor (TF) greater than 1 (eqn (i) and (ii)).39,40 The BF indicates a plant's ability to extract metals from its growing medium and store them, while the TF reflects its capacity to transport metals from the roots to the shoots.41 While BF values are often determined based on total metal concentrations in soil, research, including the work of Broadley et al.,42 has revealed that plants can only utilise the bioavailable fractions of these metals. The criteria for classifying a plant as a hyperaccumulator typically include the following: (i) a deep and widespread root system, (ii) rapid growth and large biomass production, (iii) ease of harvest, and (iv) the capacity to accumulate considerable amounts of metals in their shoots.6,24,43
Generally, Brassica juncea (Indian mustard) and Berkheya coddii (Asteraceae) are frequently utilised in the phytomining of precious metals (Table 2). The bioaccumulation factor and translocation factor of these plants exceed one, demonstrating their capacity to accumulate and transport precious and high value metals.10,44
| BF = concentration of metal in the plant/bio-available metal concentration in the substrate45,46 | (i) |
| TF = concentration of metal in the shoot/concentration of metal in the root45 | (ii) |
So far, over 800 hyperaccumulating plant species (http://hyperaccumulators.smi.uq.edu.au/collection/) have been identified globally across a wide range of taxonomic groups within the plant kingdom, most of which are members of the families Brassicaceae, Asteraceae, Amaranthaceae, Cyperaceae, Fabaceae, Lamiaceae, Poaceae, and Euphorbiaceae.34,47–49 The majority of these hyperaccumulators (>70%) primarily accumulate Ni and are found in ultramafic soils that are naturally rich in Ni, Co, and sometimes Mn.6,35,50,51 For instance, ultramafic soils in Albania have been utilised in a successful phytomining field study involving Alyssum murale.52 In this study, A. murale was cultivated in its native soil environment, with agronomic practices optimised to enhance yield, ultimately producing a bio-ore for further processing.53,54 Similarly, research in Indonesia has focused on reclaiming stripped land from mining operations by re-cultivating it with native Ni-hyperaccumulating species.55
The second largest group of hyperaccumulators, after those for Ni, is found in the Cu- and Co-rich soils of Central Africa, mainly in the Copper Hills of the Democratic Republic of Congo.34,56 Beyond base metal phytomining, other studies have explored gold recovery from mine tailings using cyanogenic plants,57,58 and the extraction of catalytically active nanoparticles from plants.59,60
Although hyperaccumulating plants hold significant potential, many are unsuitable for field phytomining demonstrations because of their low biomass production, suboptimal agronomic traits, and inability to thrive under unfavourable conditions found in mine field environments.61 Strategies such as optimising planting density or boosting nutrient availability through fertilisation may however enhance the biomass production of hyperaccumulators.62–64 The absorption and accumulation of metals in plants primarily depend on their bioavailability the soil substrate. Consequently, metal availability is significantly affected by factors such as pH, oxygen levels, nutrient balance, and the presence of coexisting inorganic and organic compounds.65 However, limited studies have been conducted with natural hyperaccumulator species for Au, Pb, Pd Pt and Hg, while a myriad of hyperaccumulators have been documented for As, Cd, Co, Ni, Zn, Cu, Mn, Se and Tl.66–68
2.1. Nickel hyperaccumulators in phytomining
According to the European Innovation Partnership (EIP), nickel is an element of significant economic value, with a relatively high market price (15.77 US $ per kilogram) compared to other metals in the same class.69,70 Moreover, Ni was recognised as a critical metal in 2022, owing to its broad applications in the metal industry, particularly in clean energy technologies designed to support climate mitigation efforts.71 Since the 1930s, there has been a steady rise in demand for Ni.72,73 Currently, about two-thirds of the nickel mined globally is used in the production of stainless steel. Additionally, nickel is extensively employed in the creation of non-ferrous alloys and alloy steels for specialised industrial, military, and aerospace applications.74 These trends are anticipated to persist or rather increase, partly because of the world's expanding infrastructure and Ni's crucial role in the manufacturing of batteries for electric vehicles.75 Ideally, mining and recycling this metal would balance this demand. Conversely, the mining and refining of Ni generate greenhouse gas emissions (CO2), contribute to environmental degradation (including soil contamination with potentially toxic metals and the acidification of nearby wetlands), and pose significant health risks to workers in Ni mining and refining facilities.76–78 These adverse impacts of Ni mining (and other resource extraction) create a conflict of interest for societies that are increasingly committed to addressing climate change, protecting the environment and promoting sustainable development through global supply chains.79
Then as well, some countries including United States (US), Australia, New Caledonia, Canada, Italy, Russia, Brazil, Indonesia and Turkey, have extensive regions of low-grade Ni ore that are mostly abandoned.80,81 The serpentinites (ultramafic soils) of the Barberton Greenstone Belt in South Africa covering an area of approximately 4000 km2, and home to over 2210 plant species are no exception.82–85 In South Africa, serpentine soils are primarily concentrated in Mpumalanga province, where the Barberton Schist Belt and the ultramafic formations of the Bushveld Igneous Complex are located.84 Moreover, there are over 6000 “derelict and ownerless” mines on the list kept by South Africa's Department of Mineral Resources (DMR), which has become a concern for the government over time as the previous owners are nowhere to be found.19 These contaminated areas are often suitable for Ni phytomining (an alternative method of primary Ni production that can complement traditional mining). Over the years, nickel phytomining has garnered significant attention due to high commercial demand, the vast expanse of nickel-rich natural soils, and the successful field testing of hypernickelophore plants.86
Until the 21st century, only high-grade nickel ores were mined, as mining low-grade ores was considered economically unfeasible. Typically, conventional mining requires an ore grade of at least 5% or more of the target metal,87 although the cutoff grade can vary depending on factors such as metal type, market price, and mining costs. For instance, nickel ores must contain at least 3% (30
000 mg kg−1) of nickel to be mined economically using traditional mining methods.88 However, few ore bodies meet this level. Phytomining thus offers a viable method to extract from orebodies with low concentration status. This in situ technology entails cultivation of hyperaccumulating plant species which can extract high amounts of Ni in their above-ground tissues, followed by harvesting, incineration of the biomass and smelting to produce high-grade bio-ores from which Ni metal/pure Ni salts are recovered.24,89,90
Phytomining can contribute to the restoration of these ultramafic (Ni-contaminated) soils, but the success of phytoextraction by hyperaccumulating plants relies on several factors, including the plants' ability to withstand metal stress, their capacity to produce substantial biomass, and the accumulation of high metal concentrations in their aerial tissues. So far, Ni phytomining trials have been conducted in Canada, France, Albania, Italy, New Zealand, Spain, and the US, utilising ultramafic soils containing 0.05–1% total Ni.89 As previously established, Ni hyperaccumulation occurs when there is an uptake and bio-accumulation of Ni on order of >1000 μg g−1 in dry leaf tissue of plant biomass.91 The level was selected to be 100–1000 times greater than what is typically observed in plants growing on non-ultramafic soil and 10–100 times higher than what is usually found in most other plants on Ni-rich ultramafic soil.32 The use of native plant species is however recommended due to their adaptation to the specific climatic and soil conditions of the area. Most phytomining efforts conducted so far have focused on Ni (Table 1), largely because of the abundance of hyperaccumulating plants and the widespread presence of ultramafic soils globally.41
Table 1 An account of Ni metal hyperaccumulation with implications for phytomining attempts
Species |
Region |
Phytomining nature |
Max conc. |
Reference |
Berkheya coddii
|
South Africa |
Field and pot trials |
100 kg ha−1 |
110
|
Alyssum Bertolonii and Berkheya coddii |
Italy |
Field trials |
13 400 and 17 000 μg g−1 |
24
|
Alyssum bertolonii
|
Italy |
In situ experimental plots |
72 kg ha−1 |
119
|
Alyssum murale
|
United States |
Greenhouse and field trials |
22 000 μg g−1 |
97
|
Alyssum murale
|
Albania |
Field experiment |
11 500 μg g−1 |
120
|
Alyssum murale
|
Albania |
Field experiment |
9129 μg g−1 |
121
|
Alyssum murale
|
Albania |
Field study |
105 kg ha−1 |
52
|
Phyllanthus rufuschaneyi
|
Malaysia |
Field and pot experiments |
>2 wt% |
122
|
Alyssum murale and Leptoplax emarginata |
Spain |
Field experiment |
4.2 and 3.0 kg Ni per ha |
64
|
Streptanthus polygaloides
|
United States |
Field trial |
100 kg ha−1 |
123 and 124 |
Berkheya coddii
|
Australia |
Green house (glasshouse) trial |
14.5 kg Ni per ha |
125
|
Alyssum murale
|
Albania |
Field experiment |
304–853 mg m−2 |
126
|
Senecio conrathii
|
South Africa |
Field sampling trial |
1695 ± 637 μg g−1 |
127
|
Manihot esculenta
|
South Africa |
Greenhouse trial |
1251 μg g−1 |
128
|
Odontarrhena plants (12) |
Greece |
Field sampling trial |
18 700 μg g−1 |
129
|
Odontarrhena chalcidica
|
Albania |
Field trial |
145 kg ha−1 |
130
|
The greatest number of reported Ni hyperaccumulators are domiciled in the genus Alyssum, many of which can achieve 30 g kg−1 Ni in dry leaf biomass.92–94 An example of this species is Odontarrhena chalcidica (also known as Alyssum murale), a perennial crop native to ultramafic soils in the arid Mediterranean region including Albania, Turkey, and Greece; however, it can grow and hyperaccumulate Ni outside its native environment.64,95 Early field experiments primarily focused on plants from the genus Odontarrhena particularly Odontarrhena muralis and Odontarrhena corsica (Alyssum corsicum).96 For instance, Chaney et al. conducted a field phytomining trial to remediate Ni-contaminated soils with the recovery of high-purity Ni metal. They utilised Alyssum murale and Alyssum corsicum to extract Ni from various Ni-rich soil types. These species are native to serpentine soils formed from ultramafic rocks in the Mediterranean region of Southern Europe.89,97,98 A one-year field experiment was conducted in a serpentine quarry in North-West Spain, to assess the performance of four Ni hyperaccumulating plant species, Bornmuellera emarginata and Odontarrhena muralis with native populations of Noccaea caerulescens and Odontarrhena serpyllifolia. Even though plants (except for O. muralis) cultivated in compost-amended plots showed lower shoot Ni contents, their higher biomass production resulted in noticeably higher Ni yields (in kg ha−1) for B. emarginata (2.9), N. caerulescens (1.9), and O. muralis (2.3). The Mediterranean species showed a greater capacity for adaptation, but all plant species were able to establish and develop in the mine soil and produce reasonable Ni yields.99
In order to maximise the bioavailability of the target metal, various factors such as application of elemental sulphur and soil acidification have been proposed to be effective.100 This could be attributed to the microbial oxidation of elemental sulphur by sulphur-oxidizing bacteria, such as Thiobacillus spp., which produces sulphuric acid (H2SO4).101,102 This process lowers the soil pH, thereby enhancing the solubility of metals like Ni, Co, Zn, and Cd, which are more bioavailable under acidic conditions. Additionally, acidification breaks down metal complexes, transforming them into plant-accessible ionic forms, e.g. Zn2+, Ni2+, and Cu2+.103,104 In a field experiment conducted at an Austrian serpentine site, Rosenkranz and co-workers evaluated the phytomining efficacy of two plant species: Odontarrhena chalcidica (Alyssum murale) and Noccaea goesingensis. For O. chalcidica, three treatments were applied: control, sulphur application (0.46 g S per kg soil), and intercropping with the legume Lotus corniculatus. For N. goesingensis, the treatments included control, high-density planting (110 plants per m2), and intercropping. Across all treatments, reports showed that O. chalcidica consistently produced higher shoot biomass, shoot Ni concentrations, and total harvested Ni compared to N. goesingensis. The highest Ni yield was achieved by O. chalcidica in the sulphur treatment, reaching 55 kg Ni per ha1. In contrast, N. goesingensis achieved its maximum yield of 36 kg Ni per ha1 under the high-density planting treatment.100
One of the primary indicators of ultramafic regions in the western North America, is the genus Streptanthus Nutt., belonging to the family of Brassicaceae Burnett (mustard family).105 This genus has attracted extensive research interest.106,107 Kruckeberg et al. previously observed that nearly all Streptanthus species are either endemic to or tolerant of ultramafic soils. However, only one species, Streptanthus polygaloides Gray, has been identified as a Ni hyperaccumulator. This annual plant can accumulate Ni at concentrations as high as 14
800 μg g−1 (1.48%) of its dry weight.108 Sánchez-Mata et al. in their field experiment, reported similar values, ranging between 0.09 and 1.18% in the dry plant biomass of Streptanthus polygaloides grown on the ultramafic soils of the Californian Sierra Nevada foothills.105
The U.S. Bureau of Mines conducted pioneering Ni phytomining trials in 1995 on a natural population of Streptanthus polygaloides A. Gray, from the Brassicaceae family, native to serpentine soils in California.108 The soil at the site contained a Ni concentration of 3340 μg g−1, consistent with typical serpentine soils. At the optimal harvest stage, S. polygaloides shoots exhibited an average Ni concentration of 5300 μg g−1 and a biomass yield of 4.8 Mg ha−1 under unfertilised conditions.109
Berkheya coddii, an herbaceous perennial plant from the Asteraceae family, is a well-documented fast-growing, high biomass Ni hyperaccumulator plant native to South Africa's Mpumalanga Province.110,111 Due to its rapid growth, large biomass, and capacity for hyperaccumulation, Berkheya coddii has gained international recognition and is regarded as a perfect candidate for phytomining metals.110 The first recorded discovery of a nickel-hyperaccumulating plant in South Africa, Berkheya coddii, was published in 1989.112 Since then, Balkwill and co-researchers have conducted extensive floristic studies on ultramafic outcrops, leading to the identification of five nickel-hyperaccumulating plant species: Berkheya coddii, Berkheya zeyheri, Berkheya nivea Senecio coronatus and S. anomalochrous.113–115 All of these species are found on the ultramafic outcrops of the Barberton Greenstone Belt. Smith et al. examined 56 out of 126 Asteraceae species known for their serpentinite tolerance in the Barberton region and identified five taxa capable of hyperaccumulating Ni.84Senecio coronatus is among the most extensively studied Ni hyperaccumulator species in South Africa. This species is widely distributed across Southern African grasslands and is also found on the ultramafic outcrops of the Barberton Greenstone Belt.116 There are two known populations of this species growing on the ultramafic outcrops: one that accumulates Ni and another that does not.117 It has been clearly demonstrated that hyperaccumulator species can serve as “metal crops” in large-scale phytomining operations, producing metal-rich biomass (bio-ore) for commercial gain, particularly for Ni.118 Some examples of Ni-hyperaccumulating plant species reported by researchers under both field and laboratory/greenhouse settings are hereby summarised in Table 1.
2.2. Hyperaccumulators of gold and silver in phytomining
Despite the prevalent application of phytomining in the extraction of Ni, emerging studies indicate that the phytomining of gold and silver also presents a financially feasible alternative.131,132 Au and Ag are widely recognised as precious metals that have captivated human interest for centuries. As reported by Thakur and colleagues, the global annual consumption of Au and Ag is 320 and 7500 tons, respectively.133 Reports of Au and Ag accumulation in plants, have been documented since the early 20th century.57,134–136 Although Au and Ag have been identified as promising candidates for phytomining, plants generally do not accumulate them naturally.137 Gold in its elemental form, Au(0), is generally not bioavailable; a precondition that is applicable to silver.138 As such, induced hyperaccumulation techniques (which entail the application of chemical amendments such as chelating agents) are often employed to solubilise these metals, and enhance their availability and uptake by plants.139 Cyanide is widely regarded as a highly effective chemical agent for solubilising Au and Ag from their ores.137,140 Its use has been shown to significantly enhance the accumulation of Au in the aerial tissues of plants,59,141 However, despite stringent regulations in many countries, improper handling of cyanide can lead to severe environmental consequences, particularly through accidental spills into waterways.142–144 For instance, numerous accidental cyanide spills into rivers and streams have caused extensive harm to aquatic ecosystems.145 These incidents are well-documented in many countries including Ghana, Kyrgyzstan, Papua New Guinea, and China. Such incidents have also been reported in Japan, Canada, and Europe.145,146
Conversely, certain plants primarily known as cyanogenic plants produce natural lixiviants that can mobilise metals in the soil.57,58,68,147 For instance, cyanides are generated through the hydrolysis of cyanogenetic glycosides, which are present in numerous plant species, including cassava (Manihot esculenta) and acacia (Acacia sieberiana). Upon hydrolysis, these glycosides produce hydrogen cyanide (HCN) which naturally complexes with the target metal and promotes the uptake of non-bioavailable metal.128 Au/Ag hyperaccumulation is characterised as accumulation beyond 1 mg kg−1 of dry matter.141,148 Nonetheless, studies on the non-induced accumulation of Au in plants are limited, likely due to the lower bioconcentration factor (BF).149 The first genuine Au phytomining experiment was not conducted until the late 1990s.141 Building on this initial discovery, efforts have intensified to enhance the level of Au accumulation in plants. Research conducted on the laboratory scale and greenhouses has demonstrated that lixiviants such as cyanide, thiocyanate, thiosulfate, peroxide, and thiourea can be used to stimulate the absorption of Au.141,150,151 The challenge lies in the use of chelating chemicals to enhance metal uptake, as this process can lead to soil and water contamination as mentioned previously. Moreover, mobilised metals may leach deeper into the soil and reach aquifers, while plants absorb only a small fraction of the mobilised gold.23
Lamb and co-workers utilised an artificial Au-bearing soil substrate with a concentration of 5 mg kg−1 to evaluate the Au uptake capacity of Brassica juncea, Berkheya coddii, and Cichorium intybus in the presence of various solubilising chemicals, including ammonium thiocyanate, ammonium thiosulfate, potassium bromide, potassium cyanide (KCN), potassium iodide, and sodium thiocyanate. The study found that hyperaccumulation induced by KCN resulted in average Au concentrations of 97 mg kg−1 in B. coddii and 326 mg kg−1 in B. juncea.152
Relevant research indicates that mine waste dumps/tailings contain considerable amounts of Au.1 Mohan reported that 33 million tonnes of residual waste contain 0.7–0.8 mg of Au per kilogram, potentially yielding up to 24 tonnes of Au over time.153 As such, the reuse of this mine waste would enable beneficial secondary applications, which encompass extracting resources or transforming waste into valuable products.59 A greenhouse study was conducted using mine tailings containing 2.35 mg kg−1 of Au ore sourced from an active mine in Mexico. The study aimed to assess the potential of Sorghum halepense for Au phytoextraction. After ten weeks of plant growth, different chemical amendments were applied including thiourea, sodium cyanide, ammonium thiosulfate, and ammonium thiocyanate to the pots two weeks before harvest. Sodium cyanide at a dose of 1 g kg−1 proved to be the most effective solubilising agent, leading to the accumulation of 23.9 mg kg−1 of Au in the aboveground dry matter of S. halepense.154
Prior investigations concerning Medicago sativa and Brassica juncea have demonstrated their exceptional capacity to accumulate metals such as Ag and Au within their tissues.132,138,141 Bali et al. highlighted the ability of Medicago sativa and Brassica juncea to hyperaccumulate Au across various concentrations and exposure durations. Their findings showed that Au uptake in Medicago sativa increased with higher concentrations of Au substrate, while Brassica juncea exhibited maximum uptake after 48 hours of exposure at elevated concentrations (100–10
000 mg kg−1). The roots of Medicago sativa accumulated a maximum of 287 mg of gold per gram of dry biomass, while Brassica juncea roots reached a maximum accumulation of 227 mg of gold per gram of dry biomass, both when exposed to a 10
000 ppm aqueous solution of KAuCl4.138
Although the concentration of Ag in plants can be as high as 1 mg kg−1, certain plants, such as Lupinus angustifolius, can accumulate up to 126 mg kg−1 through induced uptake.22 Silver is naturally accumulated by plants such as Astragalus gummifer (legume), Euphorbia macroclada (spurge), and Verbascum cheiranthifolium (Scrophulariaceae).118 Harris and co-researchers were the pioneers in suggesting the uptake of Ag metal nanoparticles by plants. The research revealed the accumulation of considerable amounts of Ag in Brassica juncea and Medicago sativa plants; in the hydroponic experiment, where Ag was supplied at concentrations ranging from 500 to 10
000 mg L−1, the highest accumulation observed was 124 g kg−1 after 48 hours.132Brassica napus (rapeseed) has been found to accumulate approximately 50
000 mg kg−1 in its roots, 30
000 mg kg−1 in its stems, and 15
000 mg kg−1 in its leaves, while the Ag concentration in mine tailings is 22.1 mg kg−1.155Table 2 highlights some of the most notable results achieved in hyperaccumulation of precious metals to date.
Table 2 An account of precious metal hyperaccumulationsa
Element |
Species |
Region |
Phytomining nature |
Max conc. |
Reference |
IH – induced hyperaccumulation N – natural hyperaccumulation; dw – dry weight.
|
Au |
Brassica juncea
|
New Zealand |
Laboratory and greenhouse trial – IH |
57 mg kg−1 |
141
|
Au |
Five crops (carrot, red beet, onion, and 2 cultivars of radish) |
New Zealand |
Greenhouse trial – IH |
Carrot roots −48.3 μg g−1 dw), and roots of two radish cultivars 113 and 102 μg g−1 dw |
150
|
Au |
Brassica juncea and Zea mays |
Brazil |
Field demonstration – IH |
39mg kg−1 |
137
|
Au |
Australian native plant species and exotic agricultural species |
Australia |
Pot/laboratory trial – IH |
Trifolium repens cv. Prestige – 27 g ton−1 dw |
139
|
Au |
Medicago sativa and Brassica juncea |
Australia |
Hydroponic experiment |
M. sativa: 287 mg g−1; B. juncea: 227 mg g−1 |
138
|
Au |
Helianthus annuus and Kalanchoe serrata |
Mexico |
Field and laboratory research – IH |
– H. annuus: 15 mg kg−1 in leaves, 16 mg kg−1 in roots and, 21 mg kg−1 in plant stems; K. serrata: >9 mg kg−1 in the dry matter of aerial tissues |
134
|
Au and Ag |
Tobacco |
Indonesia |
Field experiment – IH |
– Au: 1.2 mg kg−1 |
164
|
– Ag: 54.3 mg kg−1 |
Au and Ag |
Brassica napus
|
Mexico |
Field trial – IH |
– Au: stems – 1.5 mg kg−1; roots: 10 mg kg−1 |
155
|
– Ag: ≥50 000, 30 000, and 15 000 mg kg−1 in roots, stems, and leaves respectively |
Au |
Manihot esculenta (cassava) |
Australia |
Pot trial and hydroponics experiment |
Fibrous roots: 18.99 mg kg−1 Au |
68
|
Pt, Pd, Au |
Berkheya coddii
|
South Africa |
Greenhouse experiment (mine tailing and artificial substrate) |
– Pt: 183 μg kg−1 |
161
|
– Pd: 7677 μg kg−1 |
– Au: 1580 μg kg−1 |
Pd and Pt |
Berkheya coddii
|
South Africa |
Field experiment |
Leaf: 0.22 mg kg−1 Pt and 0.71 mg kg−1 Pd |
44
|
Root: 0.14 mg kg−1 Pt and 0.18 mg kg−1 Pd |
Pd |
Chrysopogon zizanioides (Vetiver grass) |
South Africa |
Hydroponic experiment |
Root: 0.4 mg g−1 dw |
165
|
Pd |
Mustard, miscanthus, and 16 willow species and cultivars (Arabidopsis thaliana) |
United Kingdom |
Greenhouse/glasshouse trial and hydroponic experiment – (both synthetic and mine-sourced tailings) IH |
Dried plant biomass contains: between 12 and 18 g kg−1 Pd |
60
|
2.3. Platinum group metal hyperaccumulators in phytomining
The platinum group metals (PGMs) consisting of platinum (Pt), palladium (Pd), rhodium (Rh), ruthenium (Ru), iridium (Ir) and osmium (Os) are essential to numerous modern technologies, including catalytic converters in automobiles (auto-catalysts), chemical process catalysts critical for oil refining, jewellery, electronics, hydrogen fuel cells, and specialized medical alloys. South Africa remains the leading producer and supplier of these essential mineral resources globally (Fig. 2), sourcing them entirely from the Bushveld Complex. It is followed by Russia, with smaller yet valuable contributions from Zimbabwe, Canada, and the United States.15,156
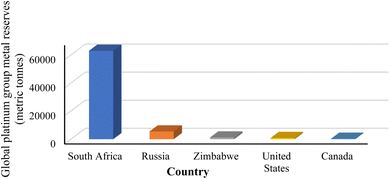 |
| Fig. 2 World reserves of PGMs.157,158 | |
PGM interactions with plants are poorly understood, and few hyperaccumulators have been identified for them to date. A few studies investigating PGM bioaccumulation were conducted in the laboratory or greenhouse and hydroponic environments. In most of these reports, the robust hyperaccumulation was stored in the roots with minimal translocation to the aerial organ of the plant. Lesniewska et al. reported the bioaccumulation of Pt, Pd, and Rh by Lolium multiflorum (ryegrass) under hydroponic conditions using nutrient solutions containing these ions at elevated (38.7 mg L−1 Pt, 21.7 mg L−1 Pd, and 7.1 mg L−1 Rh) and medium (3.6 mg L−1 Pt, 4.4 mg L−1 Pd, and 0.5 mg L−1 Rh) concentrations. The highest bioaccumulation factors were observed for Pd and Rh in the roots and for Pt in the leaves. The findings indicated that the majority of the metals studied were retained in the roots, with only a small portion being translocated to the leaves.159
Although there are no officially recognized palladium hyperaccumulators, it is expected that the threshold for PGM accumulation would be around 1 mg per kg, given their typically low concentrations in plants.160 Potassium cyanide (KCN) has been used to enhance palladium uptake in plants. Walton applied KCN at a concentration of 10 g L−1 to promote palladium accumulation in B. coddii grown on mine tailings containing 315 μg kg−1 palladium. The study revealed that the plants accumulated palladium at concentrations reaching up to 7677 μg kg−1.161 Diehl and Gagnon similarly detected Pt concentrations of 14.6 mg kg−1 in Daucus carota plants collected from a location near a heavily trafficked motorway.162
Cassava had previously shown significant potential for minefield decontamination; despite being cultivated on an Au mine site with the aim of bioaccumulating the metal, it instead accumulated zinc and lead at levels exceeding the recommended limits for daily consumption.163 Dwelling on this fact, our research group demonstrated the hypothesis of utilising the cassava plant (Manihot esculenta) to naturally accumulate Pd and Pt in an artificially contaminated potting mix. Following harvest, the cassava roots were found to contain metal concentrations of 78 ± 0.047 μg g−1 for Pd and 1276 ± 0.036 μg g−1 for Pt.67
3. Incorporating hyperaccumulating plants in mine fields and tailing dumps
The conventional methods for mining and recovering precious metals are expensive due to high energy consumption and the extensive use of chemicals for processes which often result in environmental pollution. To reduce costs and maintain profitability, industries typically rely on high-grade ores as raw materials.22,166 However, mining operations face growing challenges due to the vast depletion of local high-grade ore deposits. As a result, recycling precious metals from waste streams via phytomining (Fig. 3) offers a viable solution to address the imbalance between supply and demand.167
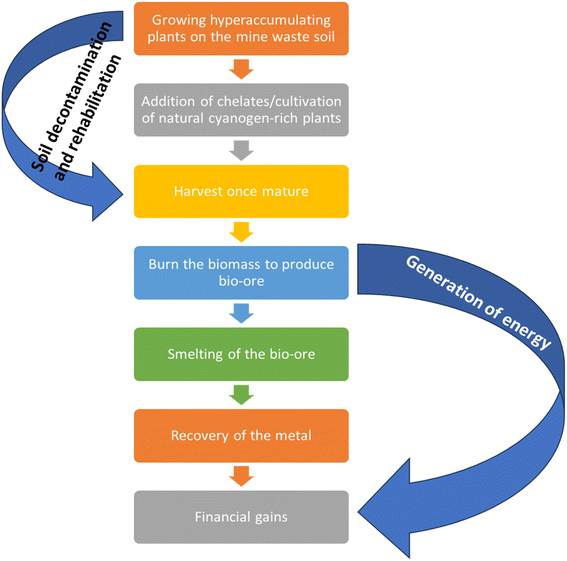 |
| Fig. 3 Process of phytomining metals. | |
PGM deposits are predominantly found in South Africa's Bushveld Igneous Complex, with significant concentrations in the Limpopo and Northwest provinces while gold mining activities primarily take place in the Witwatersrand Basin, situated in the Free State and Gauteng provinces.44,168 Base metals, on the other hand, represent a valuable secondary product of PGM extraction. In 2013, mining companies generated 562
000 times more waste than Au, according to the South African Chamber of Mines. This figure was more than double the ratio from a decade earlier, which stood at 212
000 to 1. The increase in mine waste is attributed to the depletion of South Africa's gold reserves, with the remaining deposits located several miles underground, making extraction more challenging.19 These tailing sites and waste dumps pose a significant environmental threat to the ecosystem. Phytomining offers a cost-efficient method for extracting these high value metals from mine tailings and low-grade ores,153 and these mine tailings provide ideal locations for implementing phytomining practices, which offer dual benefits: generating revenue and creating employment opportunities. Additionally, such practices contribute to the restoration of the area's ecosystem. Typically, there is a linear correlation between the metal content in plant tissues and the mineral concentration in the soil. Eucalyptus species serve as outstanding metal indicators and could be utilised to detect deep gold deposits (>10 m).169 Reports have also indicated that conifers in regions with gold ores in Canada can accumulate up to 0.02 mg of gold per kilogram, which is 100 times higher than the typical background levels found in plants.6
As previously noted, mine sites and tailings often exhibit characteristics detrimental to plant growth, such as elevated levels of toxic metals and low nutrient and organic content. Under such challenging conditions, hyperaccumulating plants may struggle to accumulate significant amounts of target metals but must still be able to survive and grow. The ideal hyperaccumulators should be fast-growing plants with high biomass production, extensive root systems, strong adaptability to high metal concentrations, capacity to thrive beyond their native collection regions and possess a significant TF. However, identifying plant species that exhibit all these characteristics is extremely challenging. For example, Alyssum bertolonii and Thlaspi caerulescens can absorb and translocate specific metals at high concentrations but have low biomass production.28,170 This has prompted researchers to explore the use of non-hyperaccumulating plant species, such as willow and miscanthus, which are valued for their rapid growth, deep root systems, and high biomass yield.171–174 Notably, willow, for instance, can tolerate a wide range of toxic and high value metals and flourish in adverse environments. Additionally, the substantial biomass produced by non-hyperaccumulators makes them suitable for dual purposes in phytomining, including soil remediation and bio-energy production (Fig. 3) which can be solid (charcoal), liquid (bioethanol and biodiesel), or gaseous (methane).18,175,176
The high costs involved in processing mine waste dumps and mine tailings using conventional methods call for a reconsideration of alternative, innovative plant-based technologies to detoxify and recapture the minerals deposited in the soil. Numerous hyperaccumulator species have been documented to extract metals in this habitat.177 However, improving the bioavailability of these minerals requires agronomic practices, including cultivation, soil management, and breeding initiatives.65 Phytomining, although still a ‘relatively new’ and underexplored concept, has the potential to offer economic and environmental advantages to soil end-users and managers working with metal-contaminated soils. By cultivating high-yield hyperaccumulator plants, it may transform yield shortages of certain commercial crops into opportunities, leveraging the metal-rich biomass for industrial purposes.160
3.1. Incorporation of food crops in phytomining
The concept of utilising food crop species for metal accumulation in phytomining requires thorough consideration. Crops like Indian mustard (Brassica juncea) and sunflower (Helianthus annuus) are frequently used in phytomining research due to their classification as “hyperaccumulators” and their rapid growth rates, which enable them to remediate larger quantities of metal efficiently. Conversely, these plant species are neither highly tolerant nor well-suited for practical field applications and are often damaged or destroyed during the phytoextraction process.178 Additionally, many non-food biomass crops significantly surpass these species in yield, achieving comparable or higher metal concentrations in their tissues without posing a risk of contaminating the human food; however, by incorporating food crops, farmers could gain income from both the harvested metals and the sale of food products, potentially offsetting the costs of phytomining operations. Growing food crops in metal-contaminated areas could help rehabilitate degraded soils while contributing to local food security, provided that metal accumulation in edible parts remains within safe limits for consumption.
The primary concern with using food crops in phytomining is the potential for harmful levels of metals to accumulate in the edible portions, posing health risks. Careful selection of crop species and cultivars with limited translocation of metals to edible parts is essential. Certain crops, such as leafy vegetables, cereals, and root crops, may vary in their ability to tolerate and accumulate metals.179 Breeding or genetic modification to enhance metal exclusion from grains, fruits, or leaves could also be adopted. Research is needed to identify crops that can effectively accumulate metals in non-edible tissues, such as roots or stems, while keeping edible parts safe. Optimising agronomic practices, such as soil amendments, irrigation, and fertilisation, can improve metal bioavailability and plant growth.180 However, it is essential to prevent the accumulated metals from contaminating the food chain or causing health risks. Regulatory frameworks must ensure that food crops grown on metal-rich soils meet safety standards. Ethical concerns regarding the consumption of such crops must also be addressed through transparent communication and risk assessments. Further research is needed to develop genetically modified or selectively bred food crops that can thrive in metal-rich soils and effectively sequester metals in non-edible parts. Additionally, studies on the long-term effects of cultivating food crops in phytomining systems on soil health, crop yields, and food safety are critical.
4. Enhancing phytomining technology
Metals serve diverse roles in all living organisms, including plants, as they form the active centres of many enzymes, catalyse essential biochemical reactions, and help maintain protein structure.181 Recent advances in genetic engineering and plant breeding offer promising avenues for improving the efficiency of phytomining.182–184 Genetic modification of plants involves transferring a foreign gene from an organism, such as another plant species, bacteria, or animals, into the genome of a target plant. Following DNA recombination, the foreign gene is passed down, imparting specific traits to the plants.185 Genetic modifications can target key transporters responsible for metal uptake in plants, such as ZIP (ZRT-IRT-like Proteins) family transporters, which play a crucial role in zinc, iron, and nickel uptake; Heavy Metal ATPases (HMA) which facilitate the movement of metals like cadmium, copper, and zinc across cellular membranes; and NRAMP (Natural Resistance-Associated Macrophage Protein) transporters, which enhance iron and manganese uptake and transport.186,187 Overexpression of these transporters can significantly improve the bioaccumulation of targeted metals.
Unlike traditional breeding, genetic engineering offers the advantage of altering plants with desirable traits for phytomining in a much shorter time frame. Fast growing, high-biomass plants are frequently engineered to exhibit the primary characteristics of hyperaccumulators.185 Transgenic approaches, such as overexpression of metal transporters and chelating proteins, have also shown potential in enhancing metal uptake and tolerance. Plants often sequester heavy metals in vacuoles to mitigate toxicity. Genetic engineering can enhance the expression of metal-binding proteins, for example, metallothioneins, small cysteine-rich proteins that bind and detoxify heavy metals; phytochelatins – peptides that chelate metals and enhance metal tolerance and ferritins – proteins that store iron and mitigate oxidative stress induced by heavy metal accumulation.188 Genetic modifications can also focus on enhancing plant growth. Strategies like overexpression of growth-promoting genes such as those involved in hormone synthesis (e.g., auxins and cytokinins) and incorporation of drought and stress-tolerant genes to improve plant resilience in metal-contaminated soils can also be adopted to improve plant biomass production.
Additionally, microbial-assisted phytomining, leveraging plant-associated microorganisms to mobilise metals, represents an emerging field of study.85 This involves the use of bacteria and fungi that interact with plant roots to enhance metal solubility, uptake, and tolerance. These microorganisms can release enzymes and chelating agents into the rhizosphere, resulting in the formation of metal-chelate complexes, which in turn enhance metal uptake and translocation. In this way, microbial community in the rhizosphere can directly stimulate root growth, which in turn promotes plant development, enhances metal tolerance, and improves overall plant stability and suitability.189 Certain rhizosphere bacteria can convert metals into more bioavailable forms. For example, sulphur-oxidizing bacteria (Thiobacillus spp.) produce sulphuric acid which enhances metal solubility and phosphate-solubilizing bacteria (Bacillus and Pseudomonas spp.) that release organic acids that increase metal availability.102,190
Although Fadzil et al. highlighted that introducing non-native, invasive species may pose a threat to biodiversity,48 this has yet to be demonstrated in practice. Nonetheless, we believe that utilising or genetically enhancing endemic or native plants adapted to metal-enriched soils could be an effective strategy to improve phytomining efficiency and increase revenue.
Furthermore, the effectiveness of metal extraction relies on the metal concentration in the soil and the substrate's pH level. Soil pH can be lowered using various substances, such as ammonia-based fertilizers, acids, and zero-valent sulphur as amendments. However, the extent to which pH can be reduced is limited, as most plant species can only grow within a specific pH range. Typically, the lowest pH tolerable for many plants is around 4.5.65
5. Economic and environmental implications
Phytomining offers dual benefits of resource recovery and land rehabilitation, particularly in areas with low-grade ores or metal-contaminated soils. By rehabilitating degraded lands, phytomining can restore habitats and promote biodiversity. However, careful management is needed to avoid introducing invasive plant species that may threaten local ecosystems. The economic feasibility depends on factors such as the market value of the target metal, operational costs, and biomass processing methods. While phytomining is less invasive than conventional mining, it may still pose environmental risks, such as unintended metal leaching. A careful assessment of these risks is crucial to ensure sustainable implementation.48
For phytomining to be economically viable, extensive mineralised areas are necessary to produce a high biomass of the desired plant species, enabling the recovery of significant amounts of the target metal. This large biomass would require a centralised incineration facility, generating revenue from both metal sales and energy production. Successful implementation of this technology requires experienced project designers who can carefully select the appropriate plant species and cultivars for specific metals (or metal combinations) and regions, while effectively managing the system to optimise metal recovery.22 Additionally, soil amendments can increase the overall cost of phytomining operations. The global phytoextraction market, valued at 34–54 billion USD, is growing in developed countries, presenting a promising opportunity for this green technology.
To estimate the potential revenue from metals extracted by hyperaccumulating plants per hectare (Table 3), we analysed current metal prices alongside data on metal concentrations and biomass yields. For instance, in the case of nickel, hyperaccumulating plants can typically store up to 4% Ni in their leaves. Field trials indicate that species such as Alyssum murale can yield between 5 and 10 metric tonnes of dry biomass per hectare each year. With a 4% nickel concentration, this corresponds to roughly 200 to 400 kilograms of Ni per hectare (ha) annually. Given the current Ni price of $7.1373 per pound, or approximately $15.73 per kilogram, this implies that cultivating nickel hyperaccumulators could generate annual revenues ranging from $3146 to $6292 per hectare, depending on plant species, growth conditions, and market fluctuations.92,191 Nevertheless, to assess the feasibility of the phytomining process, it is crucial to carefully evaluate practical challenges such as plant selection, cultivation methods, metal extraction efficiency, and economic considerations.
Table 3 Current metal prices based on reported metal concentrations and biomass yields of hyperaccumulators
|
Metal |
Metal concentration |
Biomass yield (ha per year) |
Metal yield (kg per ha per year) |
Current metal price (USD per kg) |
Estimated revenue (USD per ha per year) |
References |
1 |
Ni |
40 g kg−1 |
Alyssum murale: 5–10 metric tonnes |
200–400 |
7.1373–15.73 |
3146–6292 |
191
|
2 |
Co |
600 μg g−1 |
Berkheya coddii: 16.5 kg |
16.5 |
21.55 |
355.58 |
192 and 193 |
3 |
Pt |
8.7 g kg−1 |
Sinapis alba: 5 metric tonnes |
8.7 |
31 816.36 |
276 809.33 |
194
|
4 |
Pd |
0.4 mg g−1 |
Chrysopogon zizanioides: 20 metric tonnes |
1.6 |
31 252.13 |
50 003.41 |
165 and 195 |
5 |
Au |
1 mg kg−1 |
10 metric tonnes |
0.01 |
92 025 |
920.25 |
196
|
6 |
Tl |
5.88 kg |
Silene latifolia
|
5.88 |
7400 |
43 512 |
197
|
6. Recent developments and prospects
In recent years, Africa has made notable progress in using hyperaccumulating plants for phytomining valuable metals, environmental cleanup, and resource recovery. These advancements are especially relevant due to the continent's large-scale mining operations and related environmental concerns. Efforts to catalogue native African plant species with hyperaccumulation capabilities have increased significantly. In South Africa, for example, Berkheya zeyheri, a species native to serpentine soils, has shown an exceptional ability to absorb high levels of nickel, making it a promising option for phytoremediation and phytomining projects.198
Various initiatives have been introduced to utilise the phytoremediation/phytomining capabilities of native plants. In the Zambian Copperbelt, projects are exploring the combination of phytomining with ethanol production.199 This strategy aims to cleanse heavy metal-contaminated soils while producing biofuels, offering economic benefits alongside environmental restoration.200,201 Across Africa, governments and research institutions are increasingly acknowledging the importance of phytomining. In South Africa, for instance, environmental policies have been introduced to encourage research and the application of phytoextraction technologies. This support has facilitated the discovery of numerous plant species suitable for ecological restoration, such as Eichhornia crassipes (water hyacinth), which is used for nutrient removal from water systems.202
Despite these advancements, several challenges persist that undermine this progress. The lack of prioritisation for phytoextraction funding by many African governments has led to dependence on individuals with limited expertise, often resulting in ineffective project execution. Conversely, selecting appropriate hyperaccumulator plants demands specialised expertise. However, the limited number of professionals in this field often results in inadequate plant selection for Africa's diverse soil conditions.203 The absence of research centres and adequate laboratory equipments limits academics' ability to effectively communicate phytomining concepts to the public, resulting in low confidence in the technology.
Looking ahead, incorporating hyperaccumulating plants into phytomining and remediation in Africa presents significant potential. The concept offers a dual benefit of environmental cleanup and economic gain. For example, studies have explored the feasibility of using hyperaccumulating plants to recover precious metals from mine tailings, presenting a sustainable approach to resource recovery. In addition, equipping local communities with knowledge about the advantages and techniques of this phytotechnology can increase involvement in environmental restoration projects. Educational programs can encourage community-driven efforts to rehabilitate polluted lands through the use of native hyperaccumulating plants. With continued research, policy support, and community engagement, these green technologies have the potential to address environmental pollution and contribute to sustainable development across the continent.
7. Conclusion
The incorporation of hyperaccumulating plants in phytomining represents a promising strategy for sustainable metal recovery and environmental management. Despite its potential, phytomining faces challenges such as low biomass yields, limited metal recovery rates, and long cultivation cycles. Future research should prioritise the development of high-yielding, fast-growing hyperaccumulator species; integration of phytomining with other remediation and agricultural practices; exploration of underutilised hyperaccumulating species and novel ecosystems; genetic modification of genes associated with metal uptake, translocation, sequestration, and tolerance to enhance metal accumulation or tolerance in plants. Additionally, natural cyanogenic plants that release lixiviants and chelating agents, along with beneficial microorganisms, can be utilised to increase metal bioavailability, thereby facilitating metal accumulation in plants. These approaches can also improve soil health, further promoting plant growth and fitness. Economic modelling to optimise profitability and scalability should also be explored. Likewise, the discovery of new hyperaccumulator plants has the potential to significantly advance phytomining as a green technology. Targeted research in underexplored regions and soils, combined with advances in plant screening and genetic engineering, could uncover species with enhanced metal uptake capabilities. For instance, the Barberton Greenstone Belt, in South Africa is known for its ultramafic soils; this region has already yielded hypernickelophore plants. Additional undiscovered species may also be present. While significant progress has been made in understanding and optimising this technology, continued interdisciplinary research is needed to overcome existing limitations and fully realise its potential. By bridging gaps between plant science, agricultural management, biotechnology, and environmental engineering, phytomining can contribute to a greener and more resource-efficient future.
Data availability
There are no supporting data for this work elsewhere.
Author contributions
Babatunde Joseph Akinbile: writing the original draft, review, editing, and validation. Charles Mbohwa: supervision and validation.
Conflicts of interest
The authors declare no conflict of interest.
Acknowledgements
The authors would like to appreciate the University of South Africa for support in carrying out this research.
References
- P. Dang and C. Li, A Mini-Review of Phytomining, Int. J. Environ. Sci. Technol., 2022, 19(12), 12825–12838, DOI:10.1007/s13762-021-03807-z.
-
A. J. Hunt, Element Recovery and Sustainability, in RSC Green Chemistry Series, ed. A. Hunt, Royal Society of green Chemistry, Cambridge, 2013 Search PubMed.
-
United Nations, The UN Secretary-General’s Panel on Critical Energy Transition Minerals, United Nations, 2024, https://www.un.org/en/climatechange/critical-minerals, accessed 2024-12-24 Search PubMed.
- A. N. Erkmen, R. Ulber, T. Jüstel and M. Altendorfner, Towards Sustainable Recycling of Critical Metals from E-Waste: Bioleaching and Phytomining, Resour., Conserv. Recycl., 2025, 215, 108057, DOI:10.1016/j.resconrec.2024.108057.
- M. Edraki, T. Baumgartl, E. Manlapig, D. Bradshaw, D. M. Franks and C. J. Moran, Designing Mine Tailings for Better Environmental, Social and Economic Outcomes: A Review of Alternative Approaches, J. Cleaner Prod., 2014, 84, 411–420, DOI:10.1016/j.jclepro.2014.04.079.
- A. Corzo Remigio, R. L. Chaney, A. J. M. Baker, M. Edraki, P. D. Erskine, G. Echevarria and A. van der Ent, Phytoextraction of High Value Elements and Contaminants from Mining and Mineral Wastes: Opportunities and Limitations, Plant Soil, 2020, 449(1), 11–37, DOI:10.1007/S11104-020-04487-3.
- Z. Bao, C. J. Ptacek and D. W. Blowes, Extracting Resources from Abandoned Mines, Science, 2023, 381(6659), 731–732, DOI:10.1126/SCIENCE.ABN5962/ASSET/E258CC0E-D7F6-4DAF-8E4C-7E0C346610F8/ASSETS/GRAPHIC/SCIENCE.ABN5962-F1.SVG.
- C. Wang, D. Harbottle, Q. Liu and Z. Xu, Current State of Fine Mineral Tailings Treatment: A Critical Review on Theory and Practice, Miner. Eng., 2014, 58, 113–131, DOI:10.1016/J.MINENG.2014.01.018.
- E. Schoenberger, Environmentally Sustainable Mining: The Case of Tailings Storage Facilities, Resour. Policy, 2016, 49, 119–128, DOI:10.1016/J.RESOURPOL.2016.04.009.
- T. Dinh, Z. Dobo and H. Kovacs, Phytomining of Noble Metals – A Review, Chemosphere, 2022, 286, 131805, DOI:10.1016/J.CHEMOSPHERE.2021.131805.
-
N. LePan, All of the World's Mine Tailings, in One Visualization, https://elements.visualcapitalist.com/visualizing-the-size-of-mine-tailings/, accessed 2025-01-04 Search PubMed.
- P. H. M. Kinnunen and A. H. Kaksonen, Towards Circular Economy in Mining: Opportunities and Bottlenecks for Tailings Valorization, J. Cleaner Prod., 2019, 228, 153–160, DOI:10.1016/j.jclepro.2019.04.171.
- K. Venkateswarlu, R. Nirola, S. Kuppusamy, P. Thavamani, R. Naidu and M. Megharaj, Abandoned Metalliferous Mines: Ecological Impacts and Potential Approaches for Reclamation, Rev. Environ. Sci. Bio/Technol., 2016, 15(2), 327–354, DOI:10.1007/S11157-016-9398-6.
-
South Africa: Mining Employment 2011-2022, Statista, https://www.statista.com/statistics/1312267/south-africa-mining-employment/, accessed 2024-11-23 Search PubMed.
- B. J. Akinbile, B. C. E. Makhubela and A. A. Ambushe, Phytomining of Valuable Metals: Status and Prospective-a Review, Int. J. Environ. Anal. Chem., 2023, 103(16), 3913–3933, DOI:10.1080/03067319.2021.1917557.
-
Mineral Maps-Council for Geoscience, https://www.geoscience.org.za/. https://www.geoscience.org.za/cgs/systems/publications/downloadable-material/, accessed 2025-02-07 Search PubMed.
- C. K. Bempah and A. Ewusi, Heavy Metals Contamination and Human Health Risk Assessment around Obuasi Gold Mine in Ghana, Environ. Monit. Assess., 2016, 188(5), 261, DOI:10.1007/S10661-016-5241-3.
- P. Laval-Gilly, S. Henry, M. Mazziotti, A. Bonnefoy, A. Comel and J. Falla, Miscanthus x Giganteus Composition in Metals and Potassium After Culture on Polluted Soil and Its Use as Biofuel, Bioenergy Res., 2017, 10(3), 846–852, DOI:10.1007/S12155-017-9846-3/FIGURES/2.
-
M. Olalde, The Haunting Legacy of South Africa’s Gold Mines, Yale Environment 360, 2015 Search PubMed.
- K. Naicker, E. Cukrowska and T. S. McCarthy, Acid Mine Drainage Arising from Gold Mining Activity in Johannesburg, South Africa and Environs, Environ. Pollut., 2003, 122(1), 29–40, DOI:10.1016/S0269-7491(02)00281-6.
-
G. Okereafor, M. Mamookho, M. Lukhanyo and M. Vuyo, Evaluation of Trace Elemental Levels as Pollution Indicators in an Abandoned Gold Mine Dump in Ekurhuleni Area, South Africa, in Trace Metals in the Environment: New Approaches and Recent Advances, ed. M.-T. Mario Alfonso, S.-N. Hugo and S. Agnieszka, IntechOpen, London, UK, 2019 Search PubMed.
- V. Sheoran, A. S. Sheoran and P. Poonia, Phytomining: A Review, Miner. Eng., 2009, 22(12), 1007–1019, DOI:10.1016/j.mineng.2009.04.001.
- V. Sheoran, A. S. Sheoran and P. Poonia, Phytomining of Gold: A Review, J. Geochem. Explor., 2013, 128, 42–50, DOI:10.1016/j.gexplo.2013.01.008.
- C. W. N. Anderson, R. R. Brooks, A. Chiarucci, C. J. Lacoste, M. Leblanc, B. H. Robinson, R. Simcock and R. B. Stewart, Phytomining for Nickel, Thallium and Gold, J. Geochem. Explor., 1999, 67(1–3), 407–415, DOI:10.1016/S0375-6742(99)00055-2.
- R. R. Brooks, M. F. Chambers, L. J. Nicks and B. H. Robinson, Phytomining, Trends Plant Sci., 1998, 3(9), 359–362, DOI:10.1016/S1360-1385(98)01283-7.
- E. L. Rylott and N. C. Bruce, Plants to Mine Metals and Remediate Land, Science, 2022, 377(6613), 1380–1381, DOI:10.1126/SCIENCE.ABN6337/ASSET/1C163D7B-33FF-470B-B1C9-292199AE4FA5/ASSETS/GRAPHIC/SCIENCE.ABN6337-F1.SVG.
- H. W. Tan, Y. L. Pang, S. Lim and W. C. Chong, A State-of-the-Art of Phytoremediation Approach for Sustainable Management of Heavy Metals Recovery, Environ. Technol. Innovation, 2023, 30, 103043, DOI:10.1016/J.ETI.2023.103043.
- M. Rabbani, M. Taqi Rabbani, F. Muthoni, Y. Sun and E. Vahidi, Advancing Phytomining: Harnessing Plant Potential for Sustainable Rare Earth Element Extraction, Bioresour. Technol., 2024, 401, 130751, DOI:10.1016/J.BIORTECH.2024.130751.
-
M. N. V. Prasad, Technologies for Recovery of Metals (Including Precious) from Waste, in Environmental Materials and Waste, Elsevier, 2024, pp. 445–497, DOI:10.1016/B978-0-443-22069-2.00012-7.
- R. S. Boyd, Plant Defense Using Toxic Inorganic Ions: Conceptual Models of the Defensive Enhancement and Joint Effects Hypotheses, Plant Sci., 2012, 195, 88–95, DOI:10.1016/J.PLANTSCI.2012.06.012.
- R. D. Reeves, Tropical Hyperaccumulators of Metals and Their Potential for Phytoextraction, Plant Soil, 2003, 249(1), 57–65, DOI:10.1023/A:1022572517197.
- A. van der Ent, A. J. M. Baker, R. D. Reeves, A. J. Pollard and H. Schat, Hyperaccumulators of Metal and Metalloid Trace Elements: Facts and Fiction, Plant Soil, 2013, 362(1–2), 319–334, DOI:10.1007/s11104-012-1287-3.
- R. Kama, S. Li, F. Nabi, M. Aidara, P. Huang, Z. Li, S. Diatta, C. Ma and H. Li, Hyperaccumulators' Diversity Enhances Cd-Contaminated Soil Restoration and Reduces Rice Cd Uptake under an Intercropping System, ACS Omega, 2024, 9(26), 28784–28790, DOI:10.1021/ACSOMEGA.4C03107.
- R. D. Reeves, A. J. M. Baker, T. Jaffré, P. D. Erskine, G. Echevarria and A. van der Ent, A Global Database for Plants That Hyperaccumulate Metal and Metalloid Trace Elements, New Phytol., 2018, 218(2), 407–411, DOI:10.1111/NPH.14907.
- R. D. Reeves, The Discovery and Global Distribution of Hyperaccumulator Plants: A Personal Account, Ecol. Res., 2024, 39(4), 416–436, DOI:10.1111/1440-1703.12444.
- T. Jaffré, R. R. Brooks, J. Lee and R. D. Reeves, Sebertia Acuminata: A Hyperaccumulator of Nickel from New Caledonia, Science, 1976, 193(4253), 579–580, DOI:10.1126/science.193.4253.579.
-
Z. A. Ergenekon, Hyperaccumulator Plants and Their Use in Phytoremediation, in Biotechnology in Action: Unveiling Nature’s Potential, 2024, vol. 2, p. 157 Search PubMed.
- B. Krasnodębska-Ostręga, M. Sadowska, E. Biaduń, R. Mazur and J. Kowalska, Sinapis Alba as a Useful Plant in Bioremediation – Studies of Defense Mechanisms and Accumulation of As, Tl and PGEs, Int. J. Phytorem., 2022, 24(14), 1475–1490, DOI:10.1080/15226514.2022.2036098.
- M. R. Macnair, The Hyperaccumulation of Metals by Plants, Adv. Bot. Res., 2003, 40, 63–105, DOI:10.1016/S0065-2296(05)40002-6.
- I. Purwadi, P. D. Erskine, L. W. Casey and A. van der Ent, Recognition of Trace Element Hyperaccumulation Based on Empirical Datasets Derived from XRF Scanning of Herbarium Specimens, Plant Soil, 2023, 492(1–2), 429–438, DOI:10.1007/S11104-023-06185-2/FIGURES/3.
-
L. A. B. Novo, P. M. L. Castro, P. Alvarenga and E. F. da SilvaPhytomining of Rare and Valuable Metals, in Phytoremediation: Management of Environmental Contaminants, Volume 5, ed. A. Ansari, S. Gill, R. Gill, G. Lanza and L. Newman, Springer International Publishing, 2017, pp. 469–486, DOI:10.1007/978-3-319-52381-1_18.
- M. R. Broadley, P. J. White, J. P. Hammond, I. Zelko and A. Lux, Zinc in Plants, New Phytol., 2007, 173(4), 677–702, DOI:10.1111/j.1469-8137.2007.01996.x.
- M. Gavrilescu, Enhancing Phytoremediation of Soils Polluted with Heavy Metals, Curr. Opin. Biotechnol., 2022, 74, 21–31, DOI:10.1016/J.COPBIO.2021.10.024.
- T. Nemutandani, D. Dutertre, L. Chimuka, E. Cukrowska and H. Tutu, The Potential of Berkheya Coddii for Phytoextraction of Nickel, Platinum, and Palladium Contaminated Sites, Toxicol. Environ. Chem., 2006, 88(2), 175–185, DOI:10.1080/02772240600585842.
-
T. Mishra and V. C. Pandey, Phytoremediation of Red Mud Deposits Through Natural Succession, in Phytomanagement of Polluted Sites, Elsevier, 2019, pp. 409–424, DOI:10.1016/B978-0-12-813912-7.00016-8.
- H. L. Medina-Díaz, F. J. López-Bellido, J. Alonso-Azcárate, F. J. Fernández-Morales and L. Rodríguez, A New Hyperaccumulator Plant (Spergularia Rubra) for the Decontamination of Mine Tailings through Electrokinetic-Assisted Phytoextraction, Sci. Total Environ., 2024, 912, 169543, DOI:10.1016/j.scitotenv.2023.169543.
- A. L. D. Paul, V. Gei, S. Isnard, B. Fogliani, G. Echevarria, P. D. Erskine, T. Jaffré, J. Munzinger and A. van der Ent, Nickel Hyperaccumulation in New Caledonian Hybanthus (Violaceae) and Occurrence of Nickel-Rich Phloem in Hybanthus Austrocaledonicus, Ann. Bot., 2020, 126(5), 905–914, DOI:10.1093/AOB/MCAA112.
- F. N. M. Fadzil, M. A. N. Mohamad, R. Repin and Z. A. S. Harumain, Metal Uptake and Tolerance in Hyperaccumulator Plants: Advancing Phytomining Strategies, Rhizosphere, 2024, 29, 100836, DOI:10.1016/J.RHISPH.2023.100836.
- B. Nedjimi, Phytoremediation: A Sustainable Environmental Technology for Heavy Metals Decontamination, SN Appl. Sci., 2021, 3(3), 1–19, DOI:10.1007/S42452-021-04301-4.
-
R. Reeves and A. Baker, Metal Accumulating Plants, in Phytoremediation of Toxic Metals Using Plants to Clean up the Environment, ed. J. Raskin and B. Ensley, John Wiley and Sons, Inc., New York, 2000, pp. 193–230, DOI:10.12691/aees-1-5-4.
- R. D. Reeves, A. J. M. Baker, T. Jaffré, P. D. Erskine, G. Echevarria and A. van der Ent, A Global Database for Plants That Hyperaccumulate Metal and Metalloid Trace Elements, New Phytol., 2018, 218(2), 407–411, DOI:10.1111/NPH.14907.
- A. Bani, G. Echevarria, S. Sulçe and J. L. Morel, Improving the Agronomy of Alyssum Murale for Extensive Phytomining: A Five-Year Field Study, Int. J. Phytorem., 2015, 17(2), 117–127, DOI:10.1080/15226514.2013.862204.
- R. Barbaroux, E. Plasari, G. Mercier, M. O. Simonnot, J. L. Morel and J. F. Blais, A New Process for Nickel Ammonium
Disulfate Production from Ash of the Hyperaccumulating Plant Alyssum Murale, Sci. Total Environ., 2012, 423, 111–119, DOI:10.1016/J.SCITOTENV.2012.01.063.
- X. Zhang, B. Laubie, V. Houzelot, E. Plasari, G. Echevarria and M. O. Simonnot, Increasing Purity of Ammonium Nickel Sulfate Hexahydrate and Production Sustainability in a Nickel Phytomining Process, Chem. Eng. Res. Des., 2016, 106, 26–32, DOI:10.1016/J.CHERD.2015.12.009.
- A. Van der Ent, A. J. M. Baker, M. M. J. van Balgooy and A. Tjoa, Ultramafic Nickel Laterites in Indonesia (Sulawesi, Halmahera): Mining, Nickel Hyperaccumulators and Opportunities for Phytomining, J. Geochem. Explor., 2013, 128, 72–79, DOI:10.1016/j.gexplo.2013.01.009.
- R. Brooks, R. Reeves, R. Morrison and F. Malaisse, Hyperaccumulation of Copper and Cobalt: A Review, Bull. Soc. R. Bot. Belg., 1980, 13, 166–172 Search PubMed.
- C. A. Girling and P. J. Peterson, Gold in Plants, Gold Bull., 1980, 13(4), 151–157, DOI:10.1007/BF03215461.
- H. T. Shacklette, H. W. Lakin, A. E. Hubert and E. Al, Absorption of Gold by Plants, Bull U.S. Geol. Surv., 1970, 1314B, 1–23 Search PubMed.
- V. Wilson-Corral, C. W. N. Anderson and M. Rodriguez-Lopez, Gold Phytomining. A Review of the Relevance of This Technology to Mineral Extraction in the 21st Century, J. Environ. Manage., 2012, 111, 249–257, DOI:10.1016/J.JENVMAN.2012.07.037.
- Z. A. S. Harumain, H. L. Parker, A. Muñoz García, M. J. Austin, C. R. McElroy, A. J. Hunt, J. H. Clark, J. A. Meech, C. W. N. Anderson, L. Ciacci, T. E. Graedel, N. C. Bruce and E. L. Rylott, Toward Financially Viable Phytoextraction and Production of Plant-Based Palladium Catalysts, Environ. Sci. Technol., 2017, 51(5), 2992–3000, DOI:10.1021/acs.est.6b04821.
- A. J. Hunt, C. W. N. Anderson, N. Bruce, A. M. García, T. E. Graedel, M. Hodson, J. A. Meech, N. T. Nassar, H. L. Parker, E. L. Rylott, K. Sotiriou, Q. Zhang and J. H. Clark, Phytoextraction as a Tool for Green Chemistry, Green Process. Synth., 2014, 3(1), 3–22, DOI:10.1515/GPS-2013-0103.
- V. Álvarez-López, Prieto-Fernández, M. I. Cabello-Conejo and P. S. Kidd, Organic Amendments for Improving Biomass Production and Metal Yield of Ni-Hyperaccumulating Plants, Sci. Total Environ., 2016, 548–549, 370–379, DOI:10.1016/J.SCITOTENV.2015.12.147.
- Y. M. Li, R. Chaney, E. Brewer, R. Roseberg, J. S. Angle, A. Baker, R. Reeves and J. Nelkin, Development of a Technology for Commercial Phytoextraction of Nickel: Economic and Technical Considerations, Plant Soil, 2003, 249(1), 107–115, DOI:10.1023/A:1022527330401/METRICS.
- T. Pardo, B. Rodríguez-Garrido, R. F. Saad, J. L. Soto-Vázquez, M. Loureiro-Viñas, Á. Prieto-Fernández, G. Echevarria, E. Benizri and P. S. Kidd, Assessing the Agromining Potential of Mediterranean Nickel-Hyperaccumulating Plant Species at Field-Scale in Ultramafic Soils under Humid-Temperate Climate, Sci. Total Environ., 2018, 630, 275–286, DOI:10.1016/j.scitotenv.2018.02.229.
- V. Sheoran, A. S. Sheoran and P. Poonia, Factors Affecting Phytoextraction: A Review, Pedosphere, 2016, 26(2), 148–166, DOI:10.1016/S1002-0160(15)60032-7.
-
R. D. Reeves, A. van der Ent, G. Echevarria, S. Isnard and A. J. M. Baker, Global Distribution and Ecology of Hyperaccumulator Plants, in Agromining: Farming for Metals. Mineral Resource Reviews, ed. A. van der Ent, A. Baker, G. Echevarria, M. Simonnot and J. Morel, Springer, Cham, 2021, pp. 133–154, DOI:10.1007/978-3-030-58904-2_7.
- B. J. Akinbile, L. C. Matsinha, B. C. E. Makhubela and A. A. Ambushe, Towards Sustainability Pathway with Bio-Derived Platinum and Palladium Catalyst for Furfural Hydrogenation—A Novel Greener Approach in Catalysis, Minerals, 2021, 11(8), 895, DOI:10.3390/MIN11080895.
- H. J. P. Alcantara, A. I. Doronila and S. D. Kolev, Phytoextraction Potential of Manihot Esculenta Crantz. (Cassava) Grown in Mercury- and Gold-Containing Biosolids and Mine Tailings, Miner. Eng., 2017, 114, 57–63, DOI:10.1016/j.mineng.2017.09.010.
-
European Union, European Innovation Partnership on Raw Materials Raw Materials Scoreboard, Publications Office of the European Union, Luxembourg, 2016, DOI:10.2873/069791.
-
Daily Metal Price: Nickel Price (USD/Troy Ounce) Chart for the Last Week, https://www.dailymetalprice.com/metalpricecharts, accessed 2024-12-11 Search PubMed.
- P. Dilshara, B. Abeysinghe, R. Premasiri, N. Dushyantha, N. Ratnayake, S. Senarath, A. Sandaruwan Ratnayake and N. Batapola, The Role of Nickel (Ni) as a Critical Metal in Clean Energy Transition: Applications, Global Distribution and Occurrences, Production-Demand and Phytomining, J. Asian Earth Sci., 2024, 259, 105912, DOI:10.1016/j.jseaes.2023.105912.
- G. M. Mudd, Global Trends and Environmental Issues in Nickel Mining: Sulfides versus Laterites, Ore Geol. Rev., 2010, 38(1–2), 9–26, DOI:10.1016/J.OREGEOREV.2010.05.003.
- R. A. Smoak and J. L. Schnoor, Nickel Hyperaccumulator Biochar as a Ni-Adsorbent and Enhanced Bio-Ore, ACS Environ. Au, 2022, 2(1), 65–73, DOI:10.1021/acsenvironau.1c00018.
- P. Dilshara, B. Abeysinghe, R. Premasiri, N. Dushyantha, N. Ratnayake, S. Senarath, A. Sandaruwan Ratnayake and N. Batapola, The Role of Nickel (Ni) as a Critical Metal in Clean Energy Transition: Applications, Global Distribution and Occurrences, Production-Demand and Phytomining, J. Asian Earth Sci., 2024, 259, 105912, DOI:10.1016/J.JSEAES.2023.105912.
- R. T. Nguyen, R. G. Eggert, M. H. Severson and C. G. Anderson, Global Electrification of Vehicles and Intertwined Material Supply Chains of Cobalt, Copper and Nickel, Resour., Conserv. Recycl., 2021, 167, 105198, DOI:10.1016/J.RESCONREC.2020.105198.
- S. M. Jowitt, G. M. Mudd and J. F. H. Thompson, Future Availability of Non-Renewable Metal Resources and the Influence of Environmental, Social, and Governance Conflicts on Metal Production, Commun. Earth Environ., 2020, 1(1), 1–8, DOI:10.1038/s43247-020-0011-0.
- P. Smith, Soil Carbon Sequestration and Biochar as Negative Emission Technologies, Global Change Biol., 2016, 22(3), 1315–1324, DOI:10.1111/gcb.13178.
- M. Mistry, J. Gediga and S. Boonzaier, Life Cycle Assessment of Nickel Products, Int. J. Life Cycle Assess., 2016, 21(11), 1559–1572, DOI:10.1007/s11367-016-1085-x.
- G. M. Mudd, Sustainable/Responsible Mining and Ethical Issues Related to the Sustainable Development Goals, Geol. Soc. London, Spec. Publ., 2021, 508(1), 187–199, DOI:10.1144/SP508-2020-113.
- A. Doroshenko, V. Budarin, R. McElroy, A. J. Hunt, E. Rylott, C. Anderson, M. Waterland and J. Clark, Using: In Vivo Nickel to Direct the Pyrolysis of Hyperaccumulator Plant Biomass, Green Chem., 2019, 21(6), 1236–1240, 10.1039/c8gc03015d.
- A. van der Ent, A. J. M. Baker, M. M. J. van Balgooy and A. Tjoa, Ultramafic Nickel Laterites in Indonesia (Sulawesi, Halmahera): Mining, Nickel Hyperaccumulators and Opportunities for Phytomining, J. Geochem. Explor., 2013, 128, 72–79, DOI:10.1016/j.gexplo.2013.01.009.
- S. J. Siebert, N. C. Schutte, S. P. Bester, D. M. Komape, N. Rajakaruna and N. E. Br. Senecio Conrathii, (Asteraceae), a New Hyperaccumulator of Nickel from Serpentinite Outcrops of the Barberton Greenstone Belt, South Africa, Ecol. Res., 2018, 33(3), 651–658, DOI:10.1007/S11284-017-1541-5/FIGURES/3.
- J. C. Hughes and A. D. Noble, Extraction of Chromium, Nickel and Iron and the Availability of Chromium and Nickel to Plants from Some Serpentinite-derived Soils from the Eastern Transvaal as Revealed by Various Single and Sequential Extraction Techniques, Commun. Soil Sci. Plant Anal., 1991, 22(17–18), 1753–1766, DOI:10.1080/00103629109368533.
- S. Smith, K. Balkwill and S. Williamson, Compositae on Serpentine in the Barberton Greenstone Belt, South Africa, S. Afr. J. Sci., 2001, 97(11–12), 518–520 Search PubMed.
- J. Mesjasz-Przybyłowicz and W. J. Przybyłowicz, Ecophysiology of Nickel Hyperaccumulating Plants from South Africa – from Ultramafic Soil and Mycorrhiza to Plants and Insects, Metallomics, 2020, 12(7), 1018–1035, 10.1039/C9MT00282K.
-
R. L. Chaney and I. A. Baklanov, Phytoremediation and Phytomining: Status and Promise, Elsevier Ltd, 2017, vol. 83, DOI:10.1016/bs.abr.2016.12.006.
- R. Nkuna, G. N. Ijoma, T. S. Matambo and N. Chimwani, Accessing Metals from Low-Grade Ores and the Environmental Impact Considerations: A Review of the Perspectives of Conventional versus Bioleaching Strategies, Minerals, 2022, 12(5), 506, DOI:10.3390/MIN12050506.
-
L. A. B. Novo, P. M. L. Castro, P. Alvarenga and E. F. da Silva, Phytomining of Rare and Valuable Metals, Phytoremediation: Management of Environmental Contaminants, 2017, vol. 5, pp. 469–486, DOI:10.1007/978-3-319-52381-1_18.
- P. N. Nkrumah, A. J. M. Baker, R. L. Chaney, P. D. Erskine, G. Echevarria, J. L. Morel and A. van der Ent, Current Status and Challenges in Developing Nickel Phytomining: An Agronomic Perspective, Plant Soil, 2016, 406(1–2), 55–69, DOI:10.1007/s11104-016-2859-4.
- Y. M. Li, R. Chaney, E. Brewer, R. Roseberg, J. S. Angle, A. Baker, R. Reeves and J. Nelkin, Development of a Technology for Commercial Phytoextraction of Nickel: Economic and Technical Considerations, Plant Soil, 2003, 249(1), 107–115, DOI:10.1023/A:1022527330401.
- R. R. Brooks, J. Lee, R. D. Reeves and T. Jaffre, Detection of Nickeliferous Rocks by Analysis of Herbarium Specimens of Indicator Plants, J. Geochem. Explor., 1977, 7(C), 49–57, DOI:10.1016/0375-6742(77)90074-7.
- A. Van Der Ent, A. J. M. Baker, R. D. Reeves, R. L. Chaney, C. W. N. Anderson, J. A. Meech, P. D. Erskine, M. O. Simonnot, J. Vaughan, J. L. Morel, G. Echevarria, B. Fogliani, Q. Rongliang and D. R. Mulligan, Agromining: Farming for Metals in the Future?, Environ. Sci. Technol., 2015, 49(8), 4773–4780, DOI:10.1021/es506031u.
- R. D. Reeves and N. Adigüzel, The Nickel Hyperaccumulating Plants of the Serpentines of Turkey and Adjacent Areas: A Review with New Data, Turk. J. Biol., 2008, 32(3), 143–153 CAS.
- C. L. Broadhurst and R. L. Chaney, Growth and Metal Accumulation of an Alyssum Murale Nickel Hyperaccumulator Ecotype Co-Cropped with Alyssum Montanum and Perennial Ryegrass in Serpentine Soil, Front. Plant Sci., 2016, 7, 1–9, DOI:10.3389/fpls.2016.00451.
- L. Cecchi, I. Bettarini, I. Colzi, A. Coppi, G. Echevarria, L. Pazzagli, A. Bani, C. Gonnelli and F. Selvi, The Genus Odontarrhena (Brassicaceae) in Albania: Taxonomy and Nickel Accumulation in a Critical Group of Metallophytes from a Major Serpentine Hot-Spot, Phytotaxa, 2018, 351(1), 1, DOI:10.11646/phytotaxa.351.1.1.
- Y. M. Li, R. L. Chaney, E. P. Brewer, J. S. Angle and J. Nelkin, Phytoextraction of Nickel and Cobalt by Hyperaccumulator Alyssum Species Grown on Nickel-Contaminated Soils, Environ. Sci. Technol., 2003, 37(7), 1463–1468, DOI:10.1021/ES0208963.
- Y. M. Li, R. L. Chaney, E. P. Brewer, J. S. Angle and J. Nelkin, Phytoextraction of Nickel and Cobalt by Hyperaccumulator Alyssum Species Grown on Nickel-Contaminated Soils, Environ. Sci. Technol., 2003, 37(7), 1463–1468, DOI:10.1021/es0208963.
- R. L. Chaney, Phytoextraction and Phytomining of Soil Nickel, Nickel Soils Plants, 2018, 341–374, DOI:10.1201/9781315154664-15.
- A. Cerdeira-Pérez, C. Monterroso, B. Rodríguez-Garrido, G. Machinet, G. Echevarria, Á. Prieto-Fernández and P. S. Kidd, Implementing Nickel Phytomining in a Serpentine Quarry in NW Spain, J. Geochem. Explor., 2019, 197, 1–13, DOI:10.1016/j.gexplo.2018.11.001.
- T. Rosenkranz, C. Hipfinger, C. Ridard and M. Puschenreiter, A Nickel Phytomining Field Trial Using Odontarrhena Chalcidica and Noccaea Goesingensis on an Austrian Serpentine Soil, J. Environ. Manage., 2019, 522–528, DOI:10.1016/j.jenvman.2019.04.073.
- K. V. Usharani, D. Naik and R. L. Manjunatha, Sulphur Oxidizing Bacteria: Oxidation, Mechanism and Uses-A Review, Trends Biosci., 2019, 12(3), 203–213 Search PubMed.
-
B. Saha, S. Saha, D. Padhan, A. Das, P. Poddar, S. Pati and G. C. Hazra, Sulfur Cycle in Agricultural Soils: Microbiological Aspects, Biofertilizers and Biopesticides in Sustainable Agriculture, 2019, pp. 279–298, DOI:10.1201/9780429059384-14.
- L. M. Zhang, L. L. Long, Q. R. Zhu, C. Chen, M. Xu, J. Wu and G. Yang, Mechanism and Ecological Environmental Risk Assessment of Peroxymonosulfate for the Treatment of Heavy Metals in Soil, Sci. Total Environ., 2024, 926, 171717, DOI:10.1016/J.SCITOTENV.2024.171717.
- M. Zaib, M. Aryan, A. Khaliq, K. Haider, S. Ahmad, A. Zeeshan, E. U. Haq, U. Ahmad, H. Akbar and H. Zubair, Essential Insights for Effective Environmental Management and Human Well-Being: Strategies for Remediation in Soil-Plant- Environment Systems, J. Asian Dev. Studies, 2023, 12(3), 1453–1469 Search PubMed.
- D. Sánchez-Mata, V. De La Fuente, L. Rufo, N. Rodríguez and R. Amils, Localization of Nickel in Tissues of Streptanthus Polygaloides Gray (Cruciferae) and Endemic Nickel Hyperaccumulators from California, Biol. Trace Elem. Res., 2014, 157(1), 75–83, DOI:10.1007/s12011-013-9868-4.
- A. R. Kruckeberg, Intraspecific Variability in the Response of Certain Native Plant Species to Serpentine Soil, Am. J. Bot., 1951, 38(6), 408, DOI:10.2307/2438248.
- A. R. Kruckeberg, Soil Diversity and the Distribution of Plants, with Examples from Western North America, Madroño, 1969, 20, 137–154 Search PubMed.
- R. D. Reeves, R. R. Brooks and R. M. Macfarlane, Nickel Uptake by Californian Streptanthus and Caulanthus with Particula Reference to the Hyperaccumulator S. Polygaloides Gray (Brassicaceae), Am. J. Bot., 1981, 68(5), 708, DOI:10.2307/2442798.
- L. Nicks and M. F. Chambers, Farming for Metals, Mining Environ. Manage., 1995, 3(3), 15–18 Search PubMed.
- B. H. Robinson, R. R. Brooks, A. W. Howes, J. H. Kirkman and P. E. H. Gregg, The Potential of the High-Biomass Nickel Hyperaccumulator Berkheya Coddii for Phytoremediation and Phytomining, J. Geochem. Explor., 1997, 60(2), 115–126, DOI:10.1016/S0375-6742(97)00036-8.
- B. H. Robinson, E. Lombi, F. J. Zhao and S. P. McGrath, Uptake and Distribution of Nickel and Other Metals in the Hyperaccumulator Berkheya Coddii, New Phytol., 2003, 158(2), 279–285, DOI:10.1046/j.1469-8137.2003.00743.x.
- D. R. Morrey, K. Balkwill and M.-J. Balkwill, Studies on Serpentine Flora: Preliminary Analyses of Soils and Vegetation Associated with Serpentinite Rock Formations in the South-Eastern Transvaal, S. Afr. J. Bot., 1989, 55(2), 171–177, DOI:10.1016/S0254-6299(16)31203-0.
-
K. Balkwill, S. Williamson, C. Kidger, E. Robinson, M. Stalmans and M. Balkwill, Diversity and Conservation of Serpentine Sites in Southern Mpumalanga (Eastern Transvaal), South Africa, in The ecology of ultramafic and metalliferous areas; ORSTOM Nouméa, Documents Scienlifiques et Techniques, 1997, vol. 3, pp. 133–138 Search PubMed.
- S. D. Williamson and K. Balkwill, Plant Census and Floristic Analysis of Selected Serpentine Outcrops of the Barberton Greenstone Belt, Mpumalanga, South Africa, S. Afr. J. Bot., 2015, 97, 133–142, DOI:10.1016/J.SAJB.2014.12.004.
- S. Smith, K. Balkwill and S. Williamson, Compositae on Serpentine in the Barberton Greenstone Belt, South Africa, S. Afr. J. Sci., 2001, 97(11), 518–520, DOI:10.10520/EJC97256.
- J. Mesjasz-Przybyłowicz and W. J. Przybyłowicz, Ecophysiology of Nickel Hyperaccumulating Plants from South Africa – from Ultramafic Soil and Mycorrhiza to Plants and Insects, Metallomics, 2020, 12(7), 1018–1035, 10.1039/c9mt00282k.
- J. Mesjasz-Przybyłowicz, W. J. Przybyłowicz, V. M. Prozesky and C. A. Pineda, Quantitative Micro-PIXE Comparison of Elemental Distribution in Ni-Hyperaccumulating and Non-Accumulating Genotypes of Senecio Coronatus, Nucl. Instrum. Methods Phys. Res., Sect. B, 1997, 130(1–4), 368–373, DOI:10.1016/S0168-583X(97)00228-0.
-
S. Dhiman, A. D. Singh, J. Kour, T. Bhardwaj, R. Tikoria, D. Kumar, R. Sharma, P. Ohri and R. BhardwajPhytomining: An Innovative Technique for Sustainable Recovery of Valuable Metals/Metalloids from Agro-Industrial and Mining Wastes, in Clean Energy Production Technologies, Springer, Singapore, 2024, pp. 101–127, DOI:10.1007/978-981-97-0840-6_5.
- B. H. Robinson, A. Chiarucci, R. R. Brooks, D. Petit, J. H. Kirkman, P. E. H. Gregg and V. De Dominicis, The Nickel Hyperaccumulator Plant Alyssum Bertolonii as a Potential Agent for Phytoremediation and Phytomining of Nickel, J. Geochem. Explor., 1997, 59(2), 75–86, DOI:10.1016/S0375-6742(97)00010-1.
- A. Bani, G. Echevarria, S. Sulçe and J. L. Morel, Improving the Agronomy of Alyssum Murale for Extensive Phytomining: A Five-Year Field Study, Int. J. Phytorem., 2015, 17(2), 117–127, DOI:10.1080/15226514.2013.862204.
- A. Bani, G. Echevarria, S. Sulçe, J. L. Morel and A. Mullai, In-Situ Phytoextraction of Ni by a Native Population of Alyssum Murale on an Ultramafic Site (Albania), Plant Soil, 2007, 293(1–2), 79–89, DOI:10.1007/s11104-007-9245-1.
- P. N. Nkrumah, R. Tisserand, R. L. Chaney, A. J. M. Baker, J. L. Morel, R. Goudon, P. D. Erskine, G. Echevarria and A. van der Ent, The First Tropical ‘Metal Farm’: Some Perspectives from Field and Pot Experiments, J. Geochem. Explor., 2019, 198, 114–122, DOI:10.1016/J.GEXPLO.2018.12.003.
-
L. J. Nicks and M. F. Chambers, Pioneering Study of the Potential of Phytomining for Nickel, in Plants that Hyperaccumulate Heavy Metals: Their Role in Phytoremediation, Microbiology, Archaeology, Mineral Exploration and Phytomining, ed. R. R. Brooks, CAB International, Wallingford, Oxon, UK, New York, 1998, pp. 313–326 Search PubMed.
- L. J. Nicks and M. F. Chambers, Farming for Metals, Min. Environ. Manag., 1995, 3(3), 15–16 Search PubMed.
- S. M. Keeling, R. B. Stewart, C. W. N. Anderson and B. H. Robinson, Nickel and Cobalt Phytoextraction by the Hyperaccumulator Berkheya Coddii: Implications for Polymetallic Phytomining and Phytoremediation, Int. J. Phytorem., 2003, 5(3), 235–244, DOI:10.1080/713779223.
- M. Osmani, A. Bani and B. Hoxha, The Phytomining of Nickel from Industrial Polluted Site of Elbasan, AlbaniaThe Phytomining of Nickel from Industrial Polluted Site of Elbasan, Albania, Eur. Acad. Res., 2018, 5(10), 20–22 Search PubMed.
- S. J. Siebert, N. C. Schutte, S. P. Bester, D. M. Komape, N. Rajakaruna and N. E. Br. Senecio Conrathii, (Asteraceae), a New Hyperaccumulator of Nickel from Serpentinite Outcrops of the Barberton Greenstone Belt, South Africa, Ecol. Res., 2018, 33(3), 651–658, DOI:10.1007/s11284-017-1541-5.
- B. J. Akinbile, L. C. Matsinha, A. A. Ambushe and B. C. E. Makhubela, Catalytic Conversion of CO2 to Formate Promoted by a Biochar-Supported Nickel Catalyst Sourced from Nickel Phytoextraction Using Cyanogen-Rich Cassava, ACS Earth Space Chem., 2021, 5(10), 2846–285, DOI:10.1021/ACSEARTHSPACECHEM.1C00223.
- I. Bettarini, E. Bianchi, I. Colzi, A. Coppi, G. Echevarria, C. Gonnelli and F. Selvi, A New Species of Odontarrhena (Brassicaceae) Endemic to Greek Ultramafics: From Taxonomy to Metal Accumulation Behavior, Ecol. Res., 2024, 39(6), 822–837, DOI:10.1111/1440-1703.12491.
-
A. Bani, D. Pavlova and S. Shallari, Phytomining as a Nature-Based Solution in the Cities of Albania, in Nature-based Solutions for Circular Management of Urban Water, Springer, Cham, 2024, pp. 131–144, DOI:10.1007/978-3-031-50725-0_8.
-
M.-O. Simonnot, J. Vaughan and B. Laubie, Processing of Bio-Ore to Products, in Mineral Resource Reviews (MIRERE), ed. R. John Slack, 2018, pp. 39–51 Search PubMed.
- A. T. Harris and R. Bali, On the Formation and Extent of Uptake of Silver Nanoparticles by Live Plants, J. Nanopart. Res., 2008, 10(4), 691–695, DOI:10.1007/s11051-007-9288-5.
- P. Thakur and S. Kumar, Metallurgical Processes Unveil the Unexplored “Sleeping Mines” e- Waste: A Review, Environ. Sci. Pollut. Res., 2020, 27(26), 32359–32370, DOI:10.1007/S11356-020-09405-9/FIGURES/3.
- V. Wilson-Corral, C. Anderson, M. Rodriguez-Lopez, M. Arenas-Vargas and J. Lopez-Perez, Phytoextraction of Gold and Copper from Mine Tailings with Helianthus Annuus L. and Kalanchoe Serrata L, Miner. Eng., 2011, 24(13), 1488–1494, DOI:10.1016/j.mineng.2011.07.014.
- H. Warren and R. Delavault, Gold and Silver Content of Some Trees and Horsetails in British Columbia, Geol. Soc. Am. Bull., 1950, 61(2), 123–128 CAS.
-
J. Erdman and J. Olson, The Use of Plants in Prospecting for Precious Metals, Principally Gold: A Selected Bibliography and Topic Index, 1985 Search PubMed.
- C. Anderson, F. Moreno and J. Meech, A Field Demonstration of Gold Phytoextraction Technology, Miner. Eng., 2005, 18(4), 385–392, DOI:10.1016/j.mineng.2004.07.002.
- R. Bali, R. Siegele and A. T. Harris, Phytoextraction of Au: Uptake, Accumulation and Cellular Distribution in Medicago Sativa and Brassica Juncea, Chem. Eng. J., 2010, 156(2), 286–297, DOI:10.1016/j.cej.2009.10.019.
- R. C. R. Piccinin, S. D. Ebbs, S. M. Reichman, S. D. Kolev, I. E. Woodrow and A. J. M. Baker, A Screen of Some Native Australian Flora and Exotic Agricultural Species for Their Potential Application in Cyanide-Induced Phytoextraction of Gold, Miner. Eng., 2007, 20(14), 1327–1330, DOI:10.1016/j.mineng.2007.07.005.
- N. Kuyucak and A. Akcil, Cyanide and Removal Options from Effluents in Gold Mining and Metallurgical Processes, Miner. Eng., 2013, 50–51, 13–29, DOI:10.1016/J.MINENG.2013.05.027.
- C. W. N. Anderson, R. R. Brooks, R. B. Stewart and R. Simcock, Harvesting a Crop of Gold in Plants [4], Nature, 1998, 395(6702), 553–554, DOI:10.1038/26875.
- T. Mudder and M. Botz, Cyanide and Society: A Critical Review, Eur. J. Miner. Process. Environ. Prot., 2004, 4(1), 62–74 Search PubMed.
- E. Cordos, C. Roman, M. Ponta, T. Frentiu and R. Rautiu, Evaluation of Soil Pollution with Copper, Lead, Zinc and Cadmium in the Mining Area Baia Mare, Rev. Chim., 2007, 58(5), 470 CAS.
- C. A. Johnson, The Fate of Cyanide in Leach Wastes at Gold Mines: An Environmental Perspective, Appl. Geochem., 2015, 57, 194–205, DOI:10.1016/J.APGEOCHEM.2014.05.023.
- N. A. Amegbey and A. A. Adimado, Incidents of Cyanide Spillage in Ghana, Miner. Process. Extr. Metall., 2003, 112(2), 126–130, DOI:10.1179/037195503225002808.
- R. Eisler and S. N. Wiemeyer, Cyanide Hazards to Plants and Animals from Gold Mining and Related Water Issues, Rev. Environ. Contam. Toxicol., 2004, 183, 21–54, DOI:10.1007/978-1-4419-9100-3_2.
- C. W. N. Anderson, R. R. Brooks, R. B. Stewart and R. Simcock, Gold Uptake by Plants, Gold Bull., 1999, 32(2), 48–52, DOI:10.1007/bf03214790.
- J. Pratas, P. J. C. Favas, R. D'Souza, M. Varun and M. S. Paul, Phytoremedial Assessment of Flora Tolerant to Heavy Metals in the Contaminated Soils of an Abandoned Pb Mine in Central Portugal, Chemosphere, 2013, 90(8), 2216–2225, DOI:10.1016/j.chemosphere.2012.09.079.
- T. Dinh, H. Kovács and Z. Dobó, The Formation of Gold in Woody Biomass Combustion Ashes, Heliyon, 2024, 10(11), e32425, DOI:10.1016/j.heliyon.2024.e32425.
- F. A. Msuya, R. R. Brooks and C. W. N. Anderson, Chemically - Induced Uptake of Gold by Root Crops: Its Significance for Phytomining, Gold Bull., 2000, 33(4), 134–137, DOI:10.1007/BF03215491.
- B. D. Krisnayanti and C. Anderson, Gold Phytomining: A New Idea for Environmental Sustainability in Indonesia, Indonesian J. Geosci., 2014, 1(1), 1–7, DOI:10.17014/ijog.v1i1.171.
- A. E. Lamb, C. W. N. Anderson and R. G. Haverkamp, The Induced Accumulation of Gold In The Plants Brassica Juncea, Berkheya Coddii and Chicory, Chem. N. Z., 2001, 9, 34–36 Search PubMed.
- B. Mohan, Phytomining of Gold, Curr. Sci., 2005, 88(7), 1021–1022 Search PubMed.
-
M. Rodriguez-Lopez, V. Wilson-Corral, C. Anderson and J. Lopez-Perez, Chemically Assisted Gold Phytoextraction in Sorghum Halepense, in The 5th International Conference on Gold Science, Technology and its Applications, World Gold Council, Heidelberg, 2009, p. 353 Search PubMed.
- E. González-Valdez, A. Alarcón, R. Ferrera-Cerrato, H. R. Vega-Carrillo, M. Maldonado-Vega, M. Á. Salas-Luévano and R. Argumedo-Delira, Induced Accumulation of Au, Ag and Cu in Brassica Napus Grown in a Mine Tailings with the Inoculation of Aspergillus Niger and the Application of Two Chemical Compounds, Ecotoxicol. Environ. Saf., 2018, 154, 180–186, DOI:10.1016/j.ecoenv.2018.02.055.
- G. M. Mudd, S. M. Jowitt and T. T. Werner, Science of the Total Environment Global Platinum Group Element Resources, Reserves and Mining – A Critical Assessment, Sci. Total Environ., 2018, 623, 614–625, DOI:10.1016/j.scitotenv.2017.11.350.
-
U.S. Geological Survey, Mineral Commodity Summaries 2024, 2024, DOI:10.3133/mcs2024.
- C. O’connor and T. Alexandrova, The Geological Occurrence, Mineralogy, and Processing by Flotation of Platinum Group Minerals (Pgms) in South Africa and Russia, Minerals, 2021, 11(1), 1–15, DOI:10.3390/MIN11010054.
- B. A. Lesniewska, J. Messerschmidt, N. Jakubowski and A. Hulanicki, Bioaccumulation of Platinum Group Elements and Characterization of Their Species in Lolium Multiflorum by Size-Exclusion Chromatography Coupled with ICP-MS, Sci. Total Environ., 2004, 322(1–3), 95–108, DOI:10.1016/j.scitotenv.2003.09.019.
- C. Kikis, G. Thalassinos and V. Antoniadis, Soil Phytomining: Recent Developments—A Review, Soil Syst., 2024, 8(1), 8, DOI:10.3390/SOILSYSTEMS8010008.
-
D. Walton, The Phytoextraction of Gold and Palladium from Mine Tailings, This Thesis Is Presented in Fulfilment of the Requirements for the Degree of Master of Philosophy, Massey University, 2002.
- D. B. Diehl and Z. E. Gagnon, Interactions between Essential Nutrients with Platinum Group Metals in Submerged Aquatic and Emergent Plants, Water, Air, Soil Pollut., 2007, 184(1–4), 255–267, DOI:10.1007/S11270-007-9414-0/FIGURES/8.
- M. Zango, M. Anim-Gyampo and B. Ampadu, Health Risks of Heavy Metals in Selected Food Crops Cultivated in Small-Scale Gold-Mining Areas in Wassa-Amenfi-West District of Ghana, J. Nat. Sci. Res., 2013, 3(5), 7–12 Search PubMed.
- B. Krisnayanti, C. Anderson, S. Sukartono, Y. Afandi, H. Suheri and A. Ekawanti, Phytomining for Artisanal Gold Mine Tailings Management, Minerals, 2016, 6(3), 84, DOI:10.3390/min6030084.
- F. Masinire, D. O. Adenuga, S. M. Tichapondwa and E. M. N. Chirwa, Palladium Phytoremediation and Phytomining Potential of Vetiver Grass (Chrysopogon Zizanioides), Chem. Eng. Trans., 2022, 94, 1429–1434, DOI:10.3303/CET2294238.
-
J. Marsden and I. House, The Chemistry of Gold Extraction, Society for Mining Metallurgy and Exploration, Inc, 2006 Search PubMed.
- J. He and A. Kappler, Recovery of Precious Metals from Waste Streams, Microb. Biotechnol., 2017, 10(5), 1194–1198, DOI:10.1111/1751-7915.12759.
-
Platinum Mining in South Africa, https://projectsiq.co.za/platinum-mining-in-south-africa.htm, accessed 2025-01-03 Search PubMed.
- M. Lintern, R. Anand, C. Ryan and D. Paterson, Natural Gold Particles in Eucalyptus Leaves and Their Relevance to Exploration for Buried Gold Deposits, Nat. Commun., 2013, 4(1), 1–8, DOI:10.1038/ncomms3614.
- A. Patel and D. D. Patra, Phytoextraction Capacity of Pelargonium Graveolens L’Hér. Grown on Soil Amended with Tannery Sludge – Its Effect on the Antioxidant Activity and Oil Yield, Ecol. Eng., 2015, 74, 20–27, DOI:10.1016/J.ECOLENG.2014.10.013.
- M. Greger and T. Landberg, Use of Willow in Phytoexfraction, Int. J. Phytorem., 1999, 1(2), 115–123, DOI:10.1080/15226519908500010.
- M. Šyc, M. Pohořelý, P. Kameníková, J. Habart, K. Svoboda and M. Punčochář, Willow Trees from Heavy Metals Phytoextraction as Energy Crops, Biomass Bioenergy, 2012, 37, 106–113, DOI:10.1016/j.biombioe.2011.12.025.
- M. Mleczek, M. Gąsecka, K. Drzewiecka, P. Goliński, Z. Magdziak and T. Chadzinikolau, Copper Phytoextraction with Willow (Salix Viminalis L.) under Various Ca/Mg Ratios. Part 1. Copper Accumulation and Plant Morphology Changes, Acta Physiol. Plant., 2013, 35(11), 3251–3259, DOI:10.1007/s11738-013-1360-4.
- V. Pidlisnyuk, A. Mamirova, K. Pranaw, P. Y. Shapoval, J. Trögl and A. Nurzhanova, Potential Role of Plant Growth-Promoting Bacteria in Miscanthus x Giganteus Phytotechnology Applied to the Trace Elements Contaminated Soils, Int. Biodeterior. Biodegrad., 2020, 155, 105103, DOI:10.1016/J.IBIOD.2020.105103.
- V. Pidlisnyuk, T. Stefanovska, E. E. Lewis, L. E. Erickson and L. C. Davis, Miscanthus as a Productive Biofuel Crop for Phytoremediation, CRC Crit. Rev. Plant Sci., 2014, 33(1), 1–19, DOI:10.1080/07352689.2014.847616.
- M. Mohanty, Post-Harvest Management of Phytoremediation Technology, J. Environ. Anal. Toxicol., 2016, 6(5), 398, DOI:10.4172/2161-0525.1000398.
- H. Chen, H. Chen and Z. Chen, A Review of in Situ Phytoextraction of Rare Earth Elements from Contaminated Soils, Int. J. Phytorem., 2022, 24(6), 557–566, DOI:10.1080/15226514.2021.1957770.
- A. van der Ent and E. L. Rylott, Inventing Hyperaccumulator Plants: Improving Practice in Phytoextraction Research and Terminology, Int. J. Phytorem., 2024, 26(9), 1379–1382, DOI:10.1080/15226514.2024.2322631.
- T. Abe, M. Ito, R. Takahashi, T. Honma, N. Sekiya, K. Shirao, M. Kuramata, M. Murakami and S. Ishikawa, Breeding of a Practical Rice Line ‘TJTT8’ for Phytoextraction of Cadmium Contamination in Paddy Fields, Soil Sci. Plant Nutr., 2017, 63(4), 388–395, DOI:10.1080/00380768.2017.1345598.
- B. R. Singh, S. K. Gupta, H. Azaizeh, S. Shilev, D. Sudre, W. Y. Song, E. Martinoia and M. Mench, Safety of Food Crops on Land Contaminated with Trace Elements, J. Sci. Food Agric., 2011, 91(8), 1349–1366, DOI:10.1002/JSFA.4355.
-
A. Bhargava and S. Srivastava, Phytomining: Principles and Applications, in Biotechnology: Recent Trends and Emerging Dimensions, CRC Press, 2017, pp. 141–159, DOI:10.1201/9780203711033-9.
- M. A. d. C. Gomes, R. A. Hauser-Davis, A. N. de Souza and A. P. Vitória, Metal Phytoremediation: General Strategies, Genetically Modified Plants and Applications in Metal Nanoparticle Contamination, Ecotoxicol. Environ. Saf., 2016, 134, 133–147, DOI:10.1016/j.ecoenv.2016.08.024.
- A. Bhargava, F. F. Carmona, M. Bhargava and S. Srivastava, Approaches for Enhanced Phytoextraction of Heavy Metals, J. Environ. Manage., 2012, 105, 103–120, DOI:10.1016/J.JENVMAN.2012.04.002.
- R. Balint and I. P. Boajă, Assisted Phytoextraction as a Nature-Based Solution for the Sustainable Remediation of Metal(Loid)-Contaminated Soils, Integr. Environ. Assess. Manage., 2024, 20(6), 2003–2022, DOI:10.1002/IEAM.4907.
- A. Yan, Y. Wang, S. N. Tan, M. L. Mohd Yusof, S. Ghosh and Z. Chen, Phytoremediation: A Promising Approach for Revegetation of Heavy Metal-Polluted Land, Front. Plant Sci., 2020, 11, 513099, DOI:10.3389/FPLS.2020.00359/BIBTEX.
- T. P. A. Krishna, T. Maharajan and S. A. Ceasar, The Role of Membrane Transporters in the Biofortification of Zinc and Iron in Plants, Biol. Trace Elem. Res., 2022, 201(1), 464–478, DOI:10.1007/S12011-022-03159-W.
- P. Sharma, H. H. Ngo, S. Khanal, C. Larroche, S.-H. Kim and A. Pandey, Efficiency of Transporter Genes and Proteins in Hyperaccumulator Plants for Metals Tolerance in Wastewater Treatment: Sustainable Technique for Metal Detoxification, Environ. Technol. Innovation, 2021, 23, 101725, DOI:10.1016/J.ETI.2021.101725.
- A. Shourie, S. Mazahar and A. Singh, Biotechnological Approaches for Enhancement of Heavy Metal Phytoremediation Capacity of Plants, Environ. Monit. Assess., 2024, 196(9), 1–18, DOI:10.1007/S10661-024-12940-4.
- E. Fasani, A. Manara, F. Martini, A. Furini and G. DalCorso, The Potential of Genetic Engineering of Plants for the Remediation of Soils Contaminated with Heavy Metals, Plant, Cell Environ., 2018, 41(5), 1201–1232, DOI:10.1111/PCE.12963.
- P. Rawat, S. Das, D. Shankhdhar and S. C. Shankhdhar, Phosphate-Solubilizing Microorganisms: Mechanism and Their Role in Phosphate Solubilization and Uptake, J. Soil Sci. Plant Nutr., 2020, 21(1), 49–68, DOI:10.1007/S42729-020-00342-7.
-
Daily Metal Price: Free Metal Price Tables and Charts, https://www.dailymetalprice.com/?utm, accessed 2025-02-08 Search PubMed.
- M. Rue, A. L. D. Paul, G. Echevarria, A. Van Der Ent, M. O. Simonnot and J. L. Morel, Uptake, Translocation and Accumulation of Nickel and Cobalt in Berkheya Coddii, a ‘Metal Crop’ from South Africa, Metallomics, 2020, 12(8), 1278–1289, 10.1039/D0MT00099J.
-
LME Cobalt, London Metal Exchange, https://www.lme.com/en/metals/ev/lme-cobalt?utm, accessed 2025-02-08 Search PubMed.
- M. Asztemborska, R. Steborowski, J. Kowalska and G. Bystrzejewska-Piotrowska, Accumulation of Platinum Nanoparticles by Sinapis Alba and Lepidium Sativum Plants, Water, Air, Soil Pollut., 2015, 226(4), 1–7, DOI:10.1007/s11270-015-2381-y.
-
Palladium Prices: What Is The Live Price of Palladium?, Commodity.com. https://commodity.com/precious-metals/palladium/price/,accessed 2025-02-08 Search PubMed.
-
S. Singh, Gold Phytomining in India: An Approach to Circular Economy in the 21st Century, in Environmental Management in India: Waste to Wealth, Springer International Publishing, 2022, pp. 249–257, DOI:10.1007/978-3-030-93897-0_12/FIGURES/1.
- A. Corzo Remigio, P. N. Nkrumah, F. Pošćić, M. Edraki, A. J. M. Baker and A. van der Ent, Thallium Accumulation and Distribution in Silene Latifolia (Caryophyllaceae) Grown in Hydroponics, Plant Soil, 2022, 480(1–2), 213–226, DOI:10.1007/S11104-022-05575-2/FIGURES/6.
-
University of Witwatersrand-Chemistry, Featured research, Exploring the phytoremediation potential of local South African plants, Wits University, https://www.wits.ac.za/chemistry/news/featured-research/exploring-the-phytoremediation-potential-of-local-south-african-plants.html?utm, accessed 2025-02-07 Search PubMed.
- T. Sinkala, Integrated Phytomining and Ethanol Production in the Zambian Copperbelt to Minimize Mine Decontamination Costs and Environmental and Social Impacts: A Review, J. South. Afr. Inst. Min. Metall., 2018, 118(8), 815–824, DOI:10.17159/2411-9717/2018/V118N8A4.
- M. M. Nabuyanda, P. Kelderman, J. van Bruggen and K. Irvine, Distribution of the Heavy Metals Co, Cu, and Pb in Sediments and Typha Spp. And Phragmites Mauritianus in Three Zambian Wetlands, J. Environ. Manage., 2022, 304, 114133, DOI:10.1016/J.JENVMAN.2021.114133.
- J. L. Calderon, R. B. Kaunda, T. Sinkala, C. F. Workman, M. D. Bazilian and G. Clough, Phytoremediation and Phytoextraction in Sub-Saharan Africa: Addressing Economic and Social Challenges, Ecotoxicol. Environ. Saf., 2021, 226, 112864, DOI:10.1016/J.ECOENV.2021.112864.
- E. A. Akinpelu and F. Nchu, Advancements in Phytoremediation Research in South Africa (1997–2022), Appl. Sci., 2024, 14(17), 7660, DOI:10.3390/APP14177660.
- G. K. Malunguja and M. Paschal, Evaluating Potential Phytoremediators to Combat Detrimental Impacts of Mining on Biodiversity: A Review Focused in Africa, Discov. Environ., 2024, 2(1), 1–15, DOI:10.1007/S44274-024-00133-2.
|
This journal is © The Royal Society of Chemistry 2025 |
Click here to see how this site uses Cookies. View our privacy policy here.