DOI:
10.1039/D5SU00013K
(Perspective)
RSC Sustainability, 2025,
3, 1672-1684
Chemical bio-manufacture from diverse C-rich waste polymeric feedstocks using engineered microorganisms
Received
8th January 2025
, Accepted 19th February 2025
First published on 20th February 2025
Abstract
Sustainability targets are driving the chemicals industry away from reliance upon finite fossil fuel resources for chemical synthesis. Biotechnology holds huge promise in this area and methods to convert renewable feedstocks, such as glucose, into a myriad of value-added chemicals are well-known. Metabolic engineering and synthetic biology have been transformational in enabling microbial cells to perform non-native chemistry, increasing product yields and the scope of chemical space accessible through bio-based approaches. While the development of the bioeconomy using virgin renewable feedstocks (e.g., glucose) has been a significant milestone, we propose that the next major breakthrough towards a sustainable future lies in utilizing waste feedstocks through engineered microbes. In particular, C-rich polymeric materials such as lignocellulosic and plastic waste hold vast untapped potential for the circular bioeconomy. This mitigates land-use conflicts with the food industry and aligns with principles of the circular economy. This Perspective highlights progress and challenges in this emerging field of using biotic and abiotic polymers as a feedstock for chemical biomanufacture.
Sustainability spotlight
The chemical industry is a significant driver of fossil fuel consumption and has the largest energy usage of all industrial sectors. Sustainability targets therefore necessitate alternative and sustainable methodologies for chemical production. Simultaneously, polymeric waste, including lignocellulosic materials, non-lignocellulosic food waste, and plastics is increasing annually and current recycling technologies are insufficient to mitigate an environmental pollution. Synthetic biology can address these challenges by facilitating waste-to-chemical conversion through microbial metabolic engineering. This approach supports the development of a circular economy while aligning with United Nations Sustainable Development Goal 11 (Sustainable Cities and Communities), 12 (Responsible Consumption and Production) and 13 (Climate Action). Developing and embracing these novel technologies will enable the chemical industry to realise a step-change towards net-zero.
|
1 Introduction
Synthetic chemicals are deeply integrated into modern day society and form the cornerstone of the pharmaceuticals, plastics, agrochemicals, flavours, fragrances and cosmetics industries. Cumulatively, the chemicals industry was valued at approximately $5.7 ton in 2022,1 with 93% of this representing the petrochemicals industry, which uses finite fossil fuel resources (e.g. crude oil and natural gas) as its primary feedstock (Fig. 1a). Petrochemical synthesis accounts for 14% and 8% of all oil and gas consumption globally, with the remainder used for fuel for the transport and energy sectors.2 This reliance upon finite feedstocks to support a vast and growing array of chemical supply chains is not sustainable and has prompted a shift towards alternative raw materials.
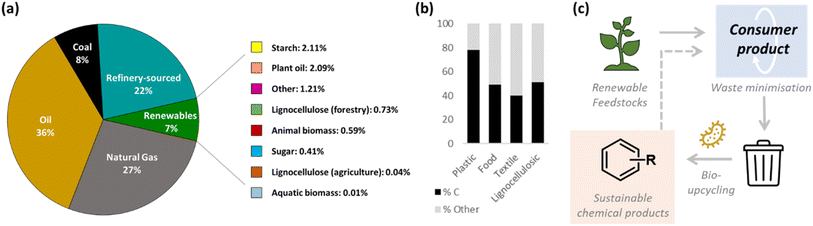 |
| Fig. 1 (a) Current feedstocks for the chemicals industry. (b) Percentage carbon content by mass of four major waste streams. (c) Proposed future materials flow for a circular chemicals economy based on renewable and waste inputs. | |
As such, the remaining 7% of the global market comprises renewable chemicals, or those wholly or partially derived from materials of biological origin, such as plants, animals and microorganisms (Fig. 1a).2,3 These renewable feedstocks may be processed via chemical or biological methods to produce the synthetic chemical of interest with life-cycle analysis studies to date often showing a reduction in greenhouse gas (GHG) emissions and energy usage for many biobased routes.4
Beyond use of virgin renewable feedstocks, there is an emerging field of valorising underutilized C-rich by-products and waste streams from industrial and municipal settings as a feedstock for chemical production. This trend is further incentivized by high feedstock costs, land-use conflicts with the food industry5,6 and circular economy policy drivers. In particular, polymeric waste streams are increasingly accessible for valorisation due to significant advances in depolymerisation technologies. In this Perspective, we will refer to these materials collectively as ‘C-rich waste feedstocks’. In contrast to virgin renewable feedstocks, waste feedstocks are typically low-cost and therefore hold potential for significant value to be added via processing into second generation chemical products. These ‘upcycling’ strategies are anticipated to generate new value chains for industry. Fig. 1b shows an estimation of the carbon content of four major waste streams which contain high proportions of polymeric content (plastic, food, textiles and non-food lignocellulosic materials). Whilst the majority of this waste is currently sent to landfill or used for energy recovery, a growing body of evidence suggests that this carbon could be diverted instead towards ‘upcycling’ processes that would yield second generation chemical products.
1.1 Biotechnological approaches to polymer upcycling
Whilst chemical approaches to polymeric waste upcycling is acknowledged as an important and active field of research,7,8 bio-based upcycling technologies hold particular advantages. Biological ‘funnelling’ of variable and mixed waste feedstocks into single chemical products presents a unique advantage over chemical processes and ‘smart’ bioprocesses could respond in real-time to feedstock variability.9 Bio-processes are also inherently suited to mesophilic and aqueous conditions, with LCA studies consistently showing sustainability advantages over chemical routes.4,10–12 In addition, enabling technologies in synthetic biology allow augmentation, adaptation or ‘re-wiring’ of microbial metabolism, including in situ depolymerisation of the polymeric feedstock into fermentable monomers.13 The result is an impressive array of engineered microbial cell factories capable of new-to-Nature enzymatic cascades14–17 and increased product titres.16,18 This will enable a paradigm shift in the synthetic chemicals industry, which transitions away from finite, petrochemical feedstocks. Rather, raw materials could be sourced from renewable, biological origin and converted into a consumer product. Aligning with the principles of the circular economy, C-rich materials would be kept in circulation for as long as possible and waste minimised.19 Unavoidable waste would then serve as the primary feedstock for bio-based upcycling processes using engineered microbial metabolism to convert industrial waste products into synthetic chemicals (Fig. 1c). This framework holds synergistic benefits of sustainable chemical production and waste valorisation which adds new value streams to established industrial processes. This Perspective highlights illustrative examples of progress in this emerging field and provides a critical evaluation of outstanding challenges. We focus on the use of C-rich polymeric feedstocks for the production of platform and fine chemicals using engineered microbial metabolism.
Transition towards a circular economy is vital to achieving a net-zero society and alignment with the UN Sustainable Development Goals (SDGs), in particular SDG 11 (sustainable cities and communities), 12 (responsible consumption and production) and 13 (climate action).20 This is driven by a staggering volume of waste materials being generated from industrial and domestic settings, including ∼2 billion tonnes of municipal solid waste produced globally pa, which is predicted to increase to ∼3.4 billion tonnes by 2050.21 An increasing proportion of this (13%–274
800 tonnes in 2020)22 is currently used for ‘waste-to-energy’ incineration, however this ultimately releases waste-embedded carbon into the atmosphere as greenhouse gasses and substantial sustainability advances could be made through instead of diverting the flow of carbon into second generation chemical products, whilst investing in ‘clean’ energy solutions in the long term.23
Two approaches have been taken to polymer waste bio-upcycling. The first is direct fermentation of waste or depolymerisation products, where these serve as the sole or primary carbon source for microbial growth and metabolism, from which the target chemical is over-produced. The second involves use of whole-cell biocatalysis (WCB) to convert a waste feedstock into the chemical of interest. This usually employs microbial cells as catalysts which metabolise a renewable feedstock (e.g. glucose) as the primary C-source for growth and heterologous enzyme and/or pathway expression. Whilst WCB can afford higher product titres, input costs tend to be higher due to the increased number of steps and nutrient broth requirements. In both cases, feedstocks typically require pre-processing into fermentable monomers prior to upcycling. The following section discusses examples of both strategies in the context of upcycling polymeric waste feedstocks into industrially important chemicals, and examples are summarised in Table 1.
Table 1 Summary of key examples of solid waste upcycling into industrially important molecules using engineered microorganisms
Feedstock |
Process |
Chassis |
Chemical |
Scale |
Time |
Titre |
Reference |
Lignocellulosic waste
|
Pine lignin |
Fed-batch fermentation |
Pseudomonas putida KT2440 |
Muconic acid |
50 mL |
54 h |
13 g L−1 |
40
|
Corn stover lignin |
Batch co-fermentation |
Rhodococcus opacus PD630, Rhodococcus jostii RHA1 VanA− |
Fatty acids (C13–C24) |
100 mL |
120 h |
0.29 g g−1 CDW (cell dry weight) |
48
|
Corn stover-derived lignin-related aromatic compounds |
Fed-batch fermentation |
Pseudomonas putida KT2440 |
β-Ketoadipic acid |
150 mL |
48 h |
25 g L−1 |
49
|
Mixed waste office paper |
Fed-batch fermentation |
E. coli KJ122 |
Succinic acid |
2.5 L |
54 h |
51.38 g L−1 |
46
|
Wheat bran, cotton seed hulls |
Batch fermentation |
Aspergillus niger N593 |
Xylitol |
50 mL |
48 h |
0.22 g L−1 and 0.26 g L−1 |
45
|
Waste orange peel |
Batch fermentation |
Saccharomyces cerevisiae BY4741 |
Meso-galactaric acid |
50 mL |
80 h |
8 g L−1 |
50
|
Waste orange peel |
Anaerobic batch fermentation |
Saccharomyces cerevisiae
|
Ethanol |
200 mL |
48 h |
7.53 g L−1 |
51
|
Non-lignocellulosic food waste
|
Bread waste |
Batch fermentation |
Euglena gracilis
|
Paramylon |
2.5 L |
72 h |
5.79 g L−1 |
52
|
Plastic waste
|
PET |
Whole cell biocatalysis |
Escherichia coli MG1655 RARE |
Vanillin |
40 mL |
24 h |
0.01 g L−1 |
53
|
PET |
Whole cell biocatalysis |
Escherichia coli BL21(DE3) |
Terephthalic acid |
3 mL |
24 h |
0.11 g L−1 |
54
|
PET |
Whole cell biocatalysis |
Escherichia coli XL1-blue, Escherichia coli MG1655(DE3) |
Gallic acid |
4–20 mL |
24 h |
0.34 g L−1 |
55
|
Pyrogallol |
24 h |
0.07 g L−1 |
Muconic acid |
6 h |
0.38 g L−1 |
Vanillic acid |
48 h |
0.23 g L−1 |
Gluconobacter oxydans KCCM 40109 |
Glycolic acid |
12 h |
∼0.76 g L−1 |
PET |
Division of labour fed-batch |
Pseudomonas putida EM42, Pseudomonas putida M31 |
Medium chain length polyhydroxyalkanoates |
50 mL |
96 h |
0.64 g L−1 |
56
|
Division of labour batch |
Muconic acid |
4.73 g L−1 |
PHB |
Batch fermentation |
Escherichia coli BL21 (DE3) |
Acetone |
5 mL |
24 h |
7 g L−1 |
13
|
PS |
Catalytic oxidative cleavage, then batch fermentation |
Aspergillus nidulans FGSC A4 (LO10050) |
Ergothioneine, pleuromutilin, mutilin |
30 mL |
144 h |
0.17 g L−1, ∼0.02 g L−1, ∼0.4 g L−1 |
57
|
PE |
Aerobic catalytic digestion, then batch fermentation |
Aspergillus nidulans FGSC A4 |
Asperbenzaldehyde |
10 mL |
144 h–72 h |
4.23 g L−1 |
58
|
Mixed plastics (PS, HDPE, PET) |
Oxidative cleavage, then batch fermentation |
Pseudomonas putida KT2440 strains |
β-Ketoadipate, polyhydroxyalkanoates (PHA) |
50 mL |
9 h |
75.5% molar yield |
59
|
1.2 Lignocellulosic waste
Lignocellulosic waste is produced at an estimated volume of 140 gigatons per year24 from agricultural and forestry residues, the food industry and municipal solid waste.25,26 It primarily comprises cellulose, hemicellulose and lignin, the proportions of which vary according to the biomass type.27,28 Despite the chemical potential of these materials as a rich and abundant source of fixed carbon, widespread application is limited by slow and energy intensive degradation into fermentable small molecules (e.g. glucose) due to the high crystallinity of cellulose and chemical stability of high-molecular weight lignin.29 Lignin also exhibits significant interspecies and intraspecies structural variability between different growth stages,30 tissues,31,32 and environmental conditions.33,34 This feedstock variability poses challenges for widespread valorisation.35,36
Lignocellulosic waste bio-valorisation efforts therefore normally employ two-step processes comprising (1) generation of fermentable small molecules through lignin degradation37,38 and (2) bio-conversion of degradation products into chemical targets of interest via engineered metabolism.39 Exemplar chemical transformations demonstrated through this approach are shown in Fig. 2 and briefly described below.
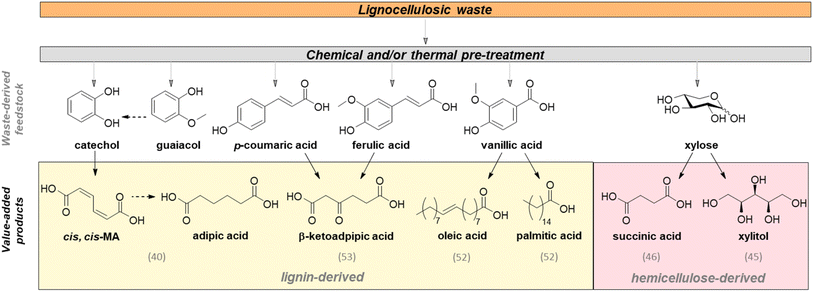 |
| Fig. 2 Chemicals accessed from lignin-rich waste feedstocks using engineered microbial metabolism. Grey arrows denote pre-processing steps; black arrows denote chemical transformations mediated by an engineered microorganism or microbial consortium; references shown in parentheses. | |
Utilisation of phenol derivatives in lignin hydrolysates has been well described. Kohlstedt et al. developed a metabolic pathway to convert pine lignin-derived catechol into cis–cis muconic acid (ccMA), a nylon precursor. A de novo metabolic pathway was constructed to convert catechol into ccMA using two native catechol 1,2-dioxygenases CatA and CatA2, and the heterologous expression of phenol hydroxylase in P. putida KT2440. A catechol and phenol-rich lignin hydrolysate from a softwood hydrothermal pre-treatment was supplemented with glucose as the carbon source for central metabolism of the engineered strain, which accumulated 13 g L−1 ccMA in a fed-batch process. The authors demonstrated production of the ccMA by chemically reducing the isolated product to adipic acid (AA) and polymerising into nylon-6,6.40 Reduction of ccMA into AA can also be achieved enzymatically using the enolate reductase from Bacillus coagulans (BcER),41 as demonstrated in E. coli BL21 (DE3) in the context of lignin-derived guaiacol upcycling to AA, giving 61% conversion to AA via co-expression of chaperone proteins.42,43 In comparison to the current industrial chemical route and assuming 100% efficiency of all steps, the guaiacol to AA route has 83% atom economy (vs. 77% for the chemical route) and crucially eliminates the production of the potent greenhouse gas N2O as a major by-product.
The hemicellulose fraction of lignocellulosic biomass contains 5 and 6-carbon monosaccharide units (e.g. arabinose, rhamnose and xylose),44 which can be fermented by engineered yeast and fungi for chemical production. For example, Meng et al. engineered an Aspergillus niger strain to convert lignocellulosic waste into xylitol, a sweetener ubiquitous in the food industry, currently produced industrially by chemical hydrogenation of xylose.45 Deletion of ladA, xdhA, and sdhA, which encode key enzymes in the pentose catabolic pathway, resulted in biomass-derived xylose degradation through batch fermentation and enabled up to 0.26 g L−1 of xylitol from cotton seed hulls.
Waste paper also represents a rich source of lignocellulosic waste and has been valorised for chemical production. For example, succinic acid (SA) is currently synthesised industrially from oil-derived benzene or n-butane at 70
000 tonne scale.46 Using engineered microorganisms, a recent bioprocess achieved >51 g L−1 SA from waste paper using E. coli KJ122 in a fed-batch system with simultaneous saccharification and fermentation.47 This process has an estimated atom economy of 95%, process mass intensity (PMI) of 20.5 and E-factor of 19.7 (calculated from paper-derived glucose and xylose to SA), which whilst higher than competing petrochemical processes (which normally fall in the PMI range of 1–5 for bulk chemicals), is an encouraging demonstration that early stage bio-processes could be competitive with their petrochemical alternatives upon further optimisation and scale-up.
Microbial co-cultures have also been explored to mitigate high metabolic burden placed on cells through overexpression of large heterologous pathways.60–63 For example, a co-culture of a wild type and engineered Rhodococcus strain showed higher lipid biosynthesis from corn stover, a residual product of corn harvest, compared to monocultures. An engineered strain of R. jostii modified lignin-derived feedstocks through the native β-ketoadipate pathway and accumulated vanillic acid, which was used as a sole carbon source for growth and production of lipids by R. opacus PD630. The co-fermentation approach enabled ∼40% lignin degradation and accumulation of 0.29 g lipid per g of cell dry weight (CDW) after 5 days, compared to 34% and 21% degradation in monoculture experiments.48 Alkaline pre-treated corn stover has also been valorised for β-ketoadipic acid production using engineered P. putida KT2440 in a fed-batch fermentation. Overexpression of vanillate O-demethylase (VanAB), replacement of endogenous p-hydroxybenzoate hydroxylase (PobA) with heterologous PraIJJ-1b from Paenibacillus sp. JJ-1b and deletion of global regulator Crc, allowed for the conversion of p-cumarate and ferulate from lignin into β-ketoadipic acid, yielding 25 g L−1 in 48 hours at 30 °C from glucose-supplemented media.49
An inherent challenge to the valorisation of polymeric waste is the requisite depolymerisation to release fermentable sugars. Synthetic biology offers a unique opportunity for simultaneous feedstock depolymerisation and upcycling through surface-display or secretion of degradative enzymes. For example, Yang et al. extracellularly expressed three heterologous cellulases (endo-1,4-β-glucanase, exo-1,4-β-glucanase, and β-xylanase) via genomic integration in S. cerevisiae.51 This enabled the production of glucose from orange-peel waste, a prolific by-product of the juice industry produced at >20 million tonne scale every year (Fig. 3).64 Bioprocessing under anaerobic conditions gave 7.53 g L−1 ethanol, an important biofuel.51 Other studies have demonstrated the use of citrus peel waste for chemical bioproduction under aerobic fermentation conditions, such as production of meso-galactaric acid from industrial orange peel waste. Here, S. cerevisiae was engineered to co-utilize peel waste-derived D-galacturonic acid and D-glucose, producing 8 g L−1 product from an 80 h batch-fermentation.50
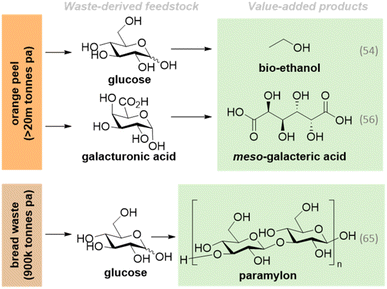 |
| Fig. 3 Examples of chemicals accessed from lignocellulosic and non-lignocellulosic food waste feedstocks using engineered microbial metabolism. The figures quoted represent the approximate volume of each waste feedstock generated each year. References shown in parentheses. | |
The potential for these preliminary studies to yield bioprocesses with genuine sustainability advantages over current petrochemical routes remains a critical area of investigation. Whilst a limited number of LCA studies have been reported, there is clear evidence of potential for genuine environmental benefits. In one study, bio-based adipic acid production was estimated to reduce greenhouse gas (GHG) emissions by 62–78% compared to petrochemical processes, which emit nitrous oxide (N2O), a greenhouse gas with 273-fold the global warming potential of CO2. However, this work also identified lignin pre-treatment energy demands and base inputs as major environmental burdens.10 A broader review of LCA studies on lignin valorisation gave a more nuanced picture.11 While lignin bioprocessing often resulted in reduced GHG emissions, fossil fuel depletion, and ecotoxicity relative to petrochemical methods, environmental burdens could increase across several impact categories and were primarily attributed to solvent and energy-intensive lignin pre-treatment and depolymerisation steps.11,65 Further, lack of standardisation in LCA methodology can hinder direct comparison between studies. This underscores the ongoing need for development of standardised LCA methodologies, and innovation to improve the release of fermentable monomers from lignin at mesophilic conditions, for example via bio- or abiotic catalysis.66
1.3 Non-lignocellulosic food waste
Food and garden waste account for more than 50% of global municipal solid waste production, presenting a significant opportunity for the circular bioeconomy which aligns with SDG 12 (food waste and losses).20,23 Biotechnology is well established in this field, with anaerobic digestion (AD) widely demonstrated as a cost-effective method for treatment of agricultural, industrial and food waste for the production of methane,67 biofuels68 and other useful products.69 Coupling AD systems with production of high-value chemical products could have additional cost benefits whilst providing new sustainable chemical manufacturing routes.70,71
For example, bread waste is an abundant source of polymeric glucose, with bread being the second most wasted food item in the UK.72 In a recent study, bread waste was used as a feedstock for paramylon and syngas production, using biological and chemical approaches, respectively. Bread was enzymatically hydrolysed to yield glucose, which fuelled the synthesis of paramylon by fermentation of the algae Euglena gracilis, while H2 was generated via catalytic pyrolysis of the solid hydrolysis residue using CO2 and Ni/SiO2 catalysis.52 This demonstrates the promising application of two complementary technologies to valorise all fractions of a processed waste stream.
1.4 Plastic waste
Plastic production currently exceeds 400 million tonnes of plastic each year, of which only 9% is recycled, 57% sent to landfill and 29% incinerated.73–75 Although traditionally considered as recalcitrant, a growing body of evidence shows that many plastics can be degraded under biologically relevant conditions into small molecules which can support microbial metabolism.76–82 These degradation products can further be ‘upcycled’ into higher-value, second generation products through engineering microbial metabolism to favour C-flux towards the product of interest. Many studies have reported innovative methods to upcycle single-use, post-consumer plastic waste, as summarised in Fig. 4. For example, poly(ethylene terephthalate) (PET)-derived terephthalic acid (TA) was upcycled into the industrially valuable small molecule vanillin through the introduction of a novel pathway in engineered Escherichia coli for whole-cell biocatalysis, giving 79% conversion of terephthalic acid into vanillin, which was sequestered via in situ product removal to mitigate product toxicity.53 Whilst we note that the theoretical (assuming 100% conversion at each step) atom efficiency of the TA to vanillin route is the same as the industrial chemical guiacol to vanillin process, a full LCA will be required to determine whether it holds tangible sustainability benefits in other categories. In another study, TA from PET was upcycled into adipic acid (115 mg L−1), a key precursor to nylon, by optimization of a six-enzyme de novo biosynthetic pathway using calcified alginate bead-encapsulated E. coli.54 To valorise both PET monomers, Kim et al. demonstrated biological valorisation of both TA and ethylene glycol (EG). Gallic acid, pyrogallol, catechol, muconic acid, and vanillic acid were obtained from whole-cell biocatalysis using plastic-derived TA as feedstock, while glycolic acid was obtained from EG conversion using a Gluconobacter oxydans strain.55 Simultaneous TA and EG consumption were also demonstrated by Bao et al., who highlighted the advantages of a division of labour approach to fully upcycling PET hydrolysate. Two strains of P. putida were designed for the respective degradation of TA and EG from a PET hydrolysate and upcycled into medium chain length PHA and cis,cis-muconic acid (ccMA) with titres of 0.64 g L−1 and 4.73 g L−1 respectively.56 Collectively, these examples offer considerable evidence that post-consumer PET offers a promising C-feedstock for chemical biomanufacture.
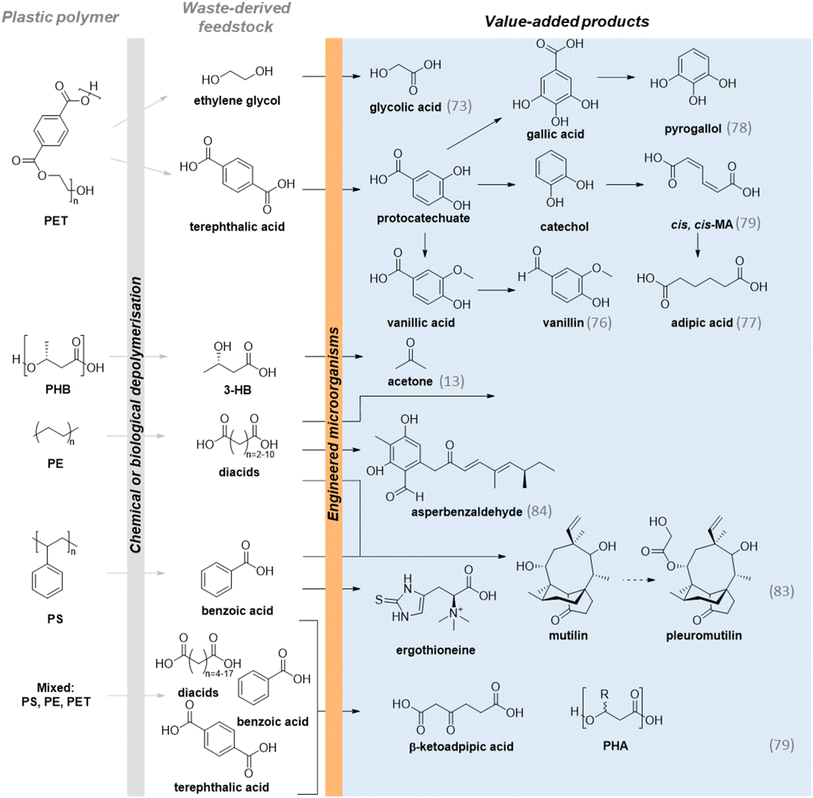 |
| Fig. 4 Chemicals accessed from plastic waste feedstocks using engineered microbial metabolism. Grey arrows denote depolymerisation steps (chemical or biological); black arrows denote chemical transformations mediated by an engineered microorganism or microbial consortium; references shown in parentheses. | |
However, many ‘real-world’ waste streams comprise complex mixtures of polymeric materials. This so called ‘mixed plastic waste’ is a significant challenge for upcycling and chemical approaches can result in complex product mixtures of low value.83,84 However, the plasticity of microbial metabolism has been demonstrated to be a powerful tool for ‘funnelling’ these mixed feedstocks into a target value-added product. A seminal example of this is from Sullivan et al. who reported a tandem chemical oxidation and bioconversion of mixed waste models comprising PET, high-density polystyrene (HDPS) and polystyrene (PS) into β-ketoadipate and polyhydroxyalkanoates (PHA).59 Mixed plastics were first depolymerised via metal-catalysed autoxidation using O2, Co(II), Mn(II) and N-hydroxyphthalimide in acetic acid at elevated temperatures to generate a mixture of dicarboxylic acids, benzoic acid and terephthalate. This mixture was then funnelled into the TCA cycle of P. putida, which was engineered for production of the target molecules. This approach enabled a 75.5% molar yield of β-ketoadipate from mixed PET, PS and HDPS.
A further challenge in plastic upcycling is valorisation of non-hydrolysable plastics, typically comprising C–C or C–O–C linked polymer backbones, yet here again engineered microbial metabolism has demonstrated potential. For example, Rabot et al. developed a hybrid chemical and biological approach to convert post-consumer PS into high value fungal secondary metabolites. PS was oxidatively depolymerised using metal catalysis, generating benzoic acid (BA) that served as sole carbon source for three engineered Aspergillus nidulans strains. A six-day fermentation produced ergothioneine, a natural anti-oxidant; mutilin, an antibiotic precursor; and its active derivative pleuromutilin.57Aspergillus nidulans has also been used for polyethylene (PE) upcycling. In this application, catalytic digestion in anaerobic conditions allowed for depolymerization of PE into a mixture of characterized carboxylic diacids that served as sole carbon source for the growth of engineered A. nidulans strains. Background strain engineering coupled with overexpression of the biosynthetic cluster genes afoG, afoE and afoC, provided 4.23 g L−1 asperbenzaldehyde in a batch fermentation. In a second system, heterologous expression of genes from A. terreus var. aureus and Clitopilus passeckerianus enabled the production of mutilin and citreoviridin, respectively.58
Whilst most plastic upcycling studies to date have focussed on petrochemical-derived polymers, bio-based and biodegradable plastics such as poly(hydroxyalkanoates) (PHAs) and poly(lactic acid) (PLA) are gaining popularity for single-use applications. Whilst widely marketed as biodegradable, there are growing concerns over ‘pollution swapping’ due to unknown effects of degradation products and microplastics on the environment, and greenhouse gas emissions through their biodegradation.85–89 To address this, our laboratory has recently demonstrated the potential of waste next-generation plastics as a feedstock for the circular bioeconomy through conversion of PHB waste into acetone, a solvent widely used in the chemical industry and cosmetics which is currently produced at 8 million tonne scale annually from oil via the cumene process. In this one-pot approach, a single strain of E. coli BL21 (DE3) was engineered to simultaneously secrete a PHB hydrolase for feedstock depolymerisation into 3-hydroxybutyrate (3-HB) whilst intracellularly expressing 3-HB dehydrogenase and acetoacetate decarboxylase for conversion of 3-HB into acetone. This strategy enabled acetone titres of up to 7 g L−1 from 24 h fermentation at 30 °C.13
Compared to lignocellulosic waste streams, the field of plastic bio-upcycling is relatively new and as such, detailed LCA studies to determine sustainability advantages are lacking. However, based on LCA studies on closed-loop plastic (bio)degradation and recycling, it is anticipated that feedstock depolymerisation will be a driver of environmental burden. We therefore reiterate that methods to depolymerise these materials at low temperature under biocompatible conditions to enable direct interfacing with biological upcycling processes will be critical in enabling ultimate success of novel upcycling technologies.90–92 Whilst plastic bio-upcycling cannot solve the vast and complex issue of plastics pollution alone, we propose that it will be an important part of a suite of technologies and policies that embed circularity into the lifecycle of unavoidable plastic waste of the future.93
2 Outlook and future perspectives
Waste bio-upcycling using engineered microorganisms holds vast potential for sustainable chemical production, and indeed preliminary life-cycle assessments in this field indicate sustainability advantages over existing petrochemical routes.91,92,94 Additionally, ambitious policy drivers are further motivating industry to both de-fossilise product supply chains, and seek opportunities for waste valorisation to generate additional revenue and meet sustainability targets. This is driving end-user engagement with engineering biology technologies to bridge waste generation and chemical bioproduction.
Whilst feedstock pre-treatment and depolymerisation has been discussed above, further outstanding challenges remain.95 First, successful process scale-up to technology readiness level (TRL) > 4 is also crucial to navigating the infamous ‘valley of death’. This is non-trivial as key process indicators (e.g. dissolved oxygen, pH, growth rate, feedstock consumption and product distribution) often do not scale linearly with fermentation volume.96,97 Earlier integration of LCA and TEA into bioprocess development may help to mitigate this, such that strains are engineered from the outset for scale up. For example, this could include greater focus on genomic integration of pathway genes to decrease costs associated with antibiotic usage, and use of constitutive or self-inducible promoter systems to decrease process costs. Additionally, transparent and comprehensive waste-mapping by region, incentivised by policy changes, could vastly accelerate transition to a more sustainable circular chemicals industry. This would enable rapid identification of potential feedstocks and matching by the academic and industrial biotechnology community to suitable chemical targets.13 Finally, mixed and variable feedstocks remain a central challenge. Costs of their pre-treatment to release fermentable substrates and removal of potential microbial inhibitors. A range of technologies are emerging for this, including polymeric resins98 and solvent extraction,99,100 however solutions are likely to be developed and scaled on a case-by-case basis as appropriate to the specific chemistry of the system.
Despite sustainability advantages inherent to a biological waste upcycling process, some metrics of sustainability such as PMI E-factor (e.g. ∼8535 and 8533, respectively, for biological upcycling of PET into vanillin based on 79% conversion of 1 mM TA) remain high for very early proof-of-concept bio-upcycling studies performed at low substrate loading and high cell density. Development of workflows to rapidly optimise these bioprocesses towards higher product titres with lower process inputs (e.g. lower cell densities, higher feedstock loading and improved product recovery) in a single fermentative step is therefore a research priority to improve their sustainability profile and bring these technologies closer to commercialisation.
The scope of molecules accessible via engineered microbial metabolism is inherently limited to chemical transformations known to nature, or which can be catalysed by new-to-nature, engineered or evolved enzyme.101–104 This precludes bioproduction of a multitude of industrially important molecules, prompting the emerging field of biocompatible chemistry, which directly interfaces abiotic chemistry with microbial metabolism to leverage the ‘best of both worlds”.105–108 We propose that biocompatible chemistry will be an important addition to the biotechnology toolbox for waste upcycling for sustainable chemical production. An early example of this has recently been reported by Valenzuela-Ortega et al. in the conversion of PET-derived terephthalic acid into the nylon 6,6-precursor adipic acid. The bottleneck double reduction of ccMA to AA using BcER was alleviated through addition of a H2-producing strain of E. coli, in combination with a Pd catalyst for chemical reduction of TA-derived ccMA to the target molecule adipic acid with 80% conversion.54 As this field continues to expand over the coming decade we anticipate these hybrid chemo-biological processes becoming increasingly prevalent in the field of waste upcycling.
This Perspective has focussed on waste streams for which upcycling via engineered microbial metabolism has been reported. However, other abundant C-rich solid waste streams also hold promise for sustainable chemical manufacture, including flower waste,109 textiles110,111 and potato waste,112–115 although opportunities to merge these with engineered microbial metabolism remain under-exploited.
3 Conclusions
Microbial metabolism has been generating functional molecules from diverse C-rich feedstocks for millions of years in a staggering range of environments. This remarkable ability for microbes to perform complex chemistry from basic building blocks continues to inspire the discovery, characterisation and application of biological machinery for the production of synthetic chemicals. In this Perspective, we have demonstrated how engineering biology can be applied to engineer microbial metabolism to enable valorisation of C-rich waste feedstocks from industry and municipal solid waste. We propose that this approach holds vast untapped potential to address sustainability issues inherent to the petrochemicals industry. In particular, lignocellulosic by-products from industry, plastic waste and food waste have all been demonstrated to be promising alternatives to virgin renewable feedstocks for the industrial biotechnology sector. We highlight the current need for future work in this field to focus on low-cost polymer depolymerisation technologies, bio-process scale up and identification and valorisation of novel C-rich waste polymeric feedstocks to realise the vast potential this technology holds for the future of a sustainable chemicals industry.
Data availability
No primary research results, software or code have been included and no new data were generated or analysed as part of this review.
Conflicts of interest
The authors declare no conflicts of interest.
Acknowledgements
JCS and MFP acknowledge a PhD studentship from IBioIC (Scottish Funding Council H19001).
References
-
Statista, Global Chemical Industry Revenue 2021|Statista, Statista, https://www.statista.com/statistics/302081/revenue-of-global-chemical-industry/, accessed 2024-07-15 Search PubMed.
- P. G. Levi and J. M. Cullen, Mapping Global Flows of Chemicals: From Fossil Fuel Feedstocks to Chemical Products, Environ. Sci. Technol., 2018, 52(4), 1725–1734, DOI:10.1021/ACS.EST.7B04573.
- V. Sturm, M. van Leeuwen, A. Gonzalez-Martinez, D. Verhoog, N. Hark and N. de Beus, Providing Insights into the Markets for Bio-Based Materials with BioMAT, Sustainability, 2023, 15(4), 3064, DOI:10.3390/su15043064.
- M. Montazeri, G. G. Zaimes, V. Khanna and M. J. Eckelman, Meta-Analysis of Life Cycle Energy and Greenhouse Gas Emissions for Priority Biobased Chemicals, ACS Sustain. Chem. Eng., 2016, 4(12), 6443–6454, DOI:10.1021/ACSSUSCHEMENG.6B01217.
- G. R. Timilsina, J. C. Beghin, D. van der Mensbrugghe and S. Mevel, The Impacts of Biofuels Targets on Land-Use Change and Food Supply: A Global CGE Assessment, Agric. Econ., 2012, 43(3), 315–332, DOI:10.1111/j.1574-0862.2012.00585.x.
- Y. Maksymiv, V. Yakubiv, N. Pylypiv, I. Hryhoruk, I. Piatnychuk and N. Popadynets, Strategic Challenges for Sustainable Governance of the Bioeconomy: Preventing Conflict between SDGs, Sustainability, 2021, 13(15), 8303, DOI:10.3390/su13158308.
- L. D. Ellis, N. A. Rorrer, K. P. Sullivan, M. Otto, J. E. McGeehan, Y. Román-Leshkov, N. Wierckx and G. T. Beckham, Chemical and Biological Catalysis for Plastics Recycling and Upcycling, Nat. Catal., 2021, 4(7), 539–556, DOI:10.1038/s41929-021-00648-4.
- K. Faust, P. Denifl and M. Hapke, Recent Advances in Catalytic Chemical Recycling of Polyolefins, ChemCatChem, 2023, 15(13), e202300310, DOI:10.1002/CCTC.202300310.
- C. L. Gargalo, I. Udugama, K. Pontius, P. C. Lopez, R. F. Nielsen, A. Hasanzadeh, S. S. Mansouri, C. Bayer, H. Junicke and K. V. Gernaey, Towards Smart Biomanufacturing: A Perspective on Recent Developments in Industrial Measurement and Monitoring Technologies for Bio-Based Production Processes, J. Ind. Microbiol. Biotechnol., 2020, 47(11), 947–964, DOI:10.1007/S10295-020-02308-1.
- A. Corona, M. J. Biddy, D. R. Vardon, M. Birkved, M. Z. Hauschild and G. T. Beckham, Life Cycle Assessment of Adipic Acid Production from Lignin, Green Chem., 2018, 20(16), 3857–3866, 10.1039/C8GC00868J.
- A. Kylili, M. Koutinas, P. Z. Georgali and P. A. Fokaides, Lignin Valorisation: Life Cycle Assessment (LCA) Considerations for Enabling Circular Bioeconomy, Int. J. Sustainable Energy, 2023, 42(1), 1008–1027, DOI:10.1080/14786451.2023.2250869.
- X. Zhao, Y. Zhang, Y. Cheng, H. Sun, S. Bai and C. Li, Identifying Environmental Hotspots and Improvement Strategies of Vanillin Production with Life Cycle Assessment, Sci. Total Environ., 2021, 769, 144771, DOI:10.1016/J.SCITOTENV.2020.144771.
- B. O. Armijo-Galdames and J. C. Sadler, One-Pot Biosynthesis of Acetone from Waste Poly(Hydroxybutyrate), ACS Sustain. Chem. Eng., 2024, 12(20), 7748–7756, DOI:10.1021/acssuschemeng.4c00357.
- S. Y. Lee, H. U. Kim, T. U. Chae, J. S. Cho, J. W. Kim, J. H. Shin, D. I. Kim, Y.-S. Ko, W. D. Jang and Y.-S. Jang, A Comprehensive Metabolic Map for Production of Bio-Based Chemicals, Nat. Catal., 2019, 2(1), 18–33, DOI:10.1038/s41929-018-0212-4.
- F. G. Calvo-Flores and F. J. Martin-Martinez, Biorefineries: Achievements and Challenges for a Bio-Based Economy, Front. Chem., 2022, 10(November), 1–23, DOI:10.3389/fchem.2022.973417.
- Y. S. Ko, J. W. Kim, J. A. Lee, T. Han, G. B. Kim, J. E. Park and S. Y. Lee, Tools and Strategies of Systems Metabolic Engineering for the Development of Microbial Cell Factories for Chemical Production, Chem. Soc. Rev., 2020, 49(14), 4615–4636, 10.1039/d0cs00155d.
- W. Jiang, D. Hernández Villamor, H. Peng, J. Chen, L. Liu, V. Haritos and R. Ledesma-Amaro, Metabolic Engineering Strategies to Enable Microbial Utilization of C1 Feedstocks, Nat. Chem. Biol., 2021, 17(8), 845–855, DOI:10.1038/s41589-021-00836-0.
- M. Cao, M. Gao, M. Suástegui, Y. Mei and Z. Shao, Building Microbial Factories for the Production of Aromatic Amino Acid Pathway Derivatives: From Commodity Chemicals to Plant-Sourced Natural Products, Metab. Eng., 2020, 58, 94–132, DOI:10.1016/j.ymben.2019.08.008.
-
European Commission, Circular Economy Action Plan for a Cleaner and More Competitive Europe, Publications Office of the European Union, 2020 Search PubMed.
-
United Nations Department of Economic and Social Affairs, The {Sustainable} {Development} {Goals} {Report} 2023: {Special} {Edition}; the {Sustainable} {Development} {Goals} {Report}, United Nations, 2023, doi: DOI:10.18356/9789210024914.
-
World Bank, What a Waste 2.0: A Global Snapshot of Waste Management to 2050, Trends in Solid Waste Management, The World Bank 2022 Search PubMed.
-
United Nations Environment Programme, Global Waste Management Outlook 2024 - beyond an Age of Waste: Turning Rubbish into a Resource, United Nations Environment Programme, 2024. doi: DOI:10.59117/20.500.11822/44939.
-
IEA, Clean Energy Transitions Programme 2022, 2023, doi: DOI:10.1787/dfc77c9e-en.
-
K. B. Devi, R. Malakar, A. Kumar, N. Sarma and D. K. Jha, Ecofriendly Utilization of Lignocellulosic Wastes: Mushroom Cultivation and Value Addition, in Value-Addition in Agri-Food Industry Waste through Enzyme Technology, Elsevier, 2023, pp. 237–254, doi: DOI:10.1016/B978-0-323-89928-4.00016-X.
- H. Y. Leong, C. K. Chang, K. S. Khoo, K. W. Chew, S. R. Chia, J. W. Lim, J. S. Chang and P. L. Show, Waste Biorefinery towards a Sustainable Circular Bioeconomy: A Solution to Global Issues, Biotechnol. Biofuels, 2021, 14(1), 87, DOI:10.1186/s13068-021-01939-5.
- A. Adewuyi, Underutilized Lignocellulosic Waste as Sources of Feedstock for Biofuel Production in Developing Countries, Front. Energy Res., 2022, 10, 741570, DOI:10.3389/fenrg.2022.741570.
-
A. Yousuf, D. Pirozzi and F. Sannino, Fundamentals of Lignocellulosic Biomass, in Lignocellulosic Biomass to Liquid Biofuels, Elsevier, 2019, pp. 1–15, DOI:10.1016/B978-0-12-815936-1.00001-0.
- Z. Gao, K. Alshehri, Y. Li, H. Qian, D. Sapsford, P. Cleall and M. Harbottle, Advances in Biological Techniques for Sustainable Lignocellulosic Waste Utilization in Biogas Production, Renewable Sustainable Energy Rev., 2022, 170, 112995, DOI:10.1016/j.rser.2022.112995.
- R. Liguori and V. Faraco, Biological Processes for Advancing Lignocellulosic Waste Biorefinery by Advocating Circular Economy, Bioresour. Technol., 2016, 215, 13–20, DOI:10.1016/j.biortech.2016.04.054.
- C. Zhang, X. Shen, M. Liu, J. Wen and T. Q. Yuan, Uncovering the Structure of Lignin from Moso Bamboo with Different Tissues and Growing Ages for Efficient Ambient-Pressure Lignin Depolymerization, ACS Sustain. Chem. Eng., 2023, 11(37), 13778–13786, DOI:10.1021/acssuschemeng.3c04206.
- A. Lourenço, J. Rencoret, C. Chemetova, J. Gominho, A. Gutiérrez, J. C. Del Río and H. Pereira, Lignin Composition and Structure Differs between Xylem, Phloem and Phellem in Quercus Suber L, Front. Plant Sci., 2016, 7, 1612, DOI:10.3389/fpls.2016.01612.
- L. A. Donaldson, Lignification and Lignin Topochemistry - An Ultrastructural View, Phytochemistry, 2001, 57(6), 859–873, DOI:10.1016/S0031-9422(01)00049-8.
- F. Takahashi, T. Kuromori, K. Urano, K. Yamaguchi-Shinozaki and K. Shinozaki, Drought Stress Responses and Resistance in Plants: From Cellular Responses to
Long-Distance Intercellular Communication, Front. Plant Sci., 2020, 11, 556972, DOI:10.3389/fpls.2020.556972.
- S. J. Choi, Z. Lee, S. Kim, E. Jeong and J. S. Shim, Modulation of Lignin Biosynthesis for Drought Tolerance in Plants, Front. Plant Sci., 2023, 14, 1116426, DOI:10.3389/fpls.2023.1116426.
- C. C. Polo, L. Pereira, P. Mazzafera, D. N. A. Flores-Borges, J. L. S. Mayer, M. Guizar-Sicairos, M. Holler, M. Barsi-Andreeta, H. Westfahl and F. Meneau, Correlations between Lignin Content and Structural Robustness in Plants Revealed by X-Ray Ptychography, Sci. Rep., 2020, 10(1), 6023, DOI:10.1038/s41598-020-63093-6.
- M. Li, Y. Pu and A. J. Ragauskas, Current Understanding of the Correlation of Lignin Structure with Biomass Recalcitrance, Front. Chem., 2016, 4, 45, DOI:10.3389/fchem.2016.00045.
- B. Segers, P. Nimmegeers, M. Spiller, G. Tofani, E. Jasiukaityte-Grojzdek, E. Dace, T. Kikas, J. M. Marchetti, M. Rajic, G. Yildiz and P. Billen, Lignocellulosic Biomass Valorisation: A Review of Feedstocks, Processes and Potential Value Chains and Their Implications for the Decision-Making Process, RSC Sustainability, 2024, 2(12), 3730–3749, 10.1039/D4SU00342J.
- C. Xu, R. A. D. Arancon, J. Labidi and R. Luque, Lignin Depolymerisation Strategies: Towards Valuable Chemicals and Fuels, Chem. Soc. Rev., 2014, 43(22), 7485–7500, 10.1039/C4CS00235K.
- J. Becker and C. Wittmann, A Field of Dreams: Lignin Valorization into Chemicals, Materials, Fuels, and Health-Care Products, Biotechnol. Adv., 2019, 37(6), 107360, DOI:10.1016/J.BIOTECHADV.2019.02.016.
- M. Kohlstedt, S. Starck, N. Barton, J. Stolzenberger, M. Selzer, K. Mehlmann, R. Schneider, D. Pleissner, J. Rinkel, J. S. Dickschat, J. Venus, J. B. J. H. van Duuren and C. Wittmann, From Lignin to Nylon: Cascaded Chemical and Biochemical Conversion Using Metabolically Engineered Pseudomonas Putida, Metab. Eng., 2018, 47, 279–293, DOI:10.1016/j.ymben.2018.03.003.
- J. C. Joo, A. N. Khusnutdinova, R. Flick, T. Kim, U. T. Bornscheuer, A. F. Yakunin and R. Mahadevan, Alkene Hydrogenation Activity of Enoate Reductases for an Environmentally Benign Biosynthesis of Adipic Acid, Chem. Sci., 2017, 8(2), 1406–1413, 10.1039/c6sc02842j.
- J. T. Suitor, S. Varzandeh and S. Wallace, One-{Pot} {Synthesis} of {Adipic} {Acid} from {Guaiacol} in {Escherichia} Coli, ACS Synth. Biol., 2020, 9(9), 2472–2476, DOI:10.1021/acssynbio.0c00254.
- J. F. C. Steele and S. Wallace, Deciding the Future of Adipic Acid, Nat. Chem., 2024, 16(5), 838, DOI:10.1038/s41557-024-01496-y.
- F. H. Isikgor and C. R. Becer, Lignocellulosic Biomass: A Sustainable Platform for the Production of Bio-Based Chemicals and Polymers, Polym. Chem., 2015, 6(25), 4497–4559, 10.1039/c5py00263j.
- J. Meng, T. Chroumpi, M. R. Mäkelä and R. P. de Vries, Xylitol Production from Plant Biomass by Aspergillus Niger through Metabolic Engineering, Bioresour. Technol., 2022, 344, 126199, DOI:10.1016/j.biortech.2021.126199.
-
R. K. Saxena, S. Saran, J. Isar and R. Kaushik, Production and Applications of Succinic Acid, in Current Developments in Biotechnology and Bioengineering: Production, Isolation and Purification of Industrial Products, ed. Pandey, A., Negi, S. and Soccol, C. R., Elsevier, 2016, pp. 601–630, DOI:10.1016/B978-0-444-63662-1.00027-0.
- C. Kalia, W. Congthai, C. Phosriran, S. Chou, K. Onsanoi, C. Gosalawit, K.-C. Cheng and K. Jantama, Exploiting Mixed Waste Office Paper Containing Lignocellulosic Fibers for Alternatively Producing High-Value Succinic Acid by Metabolically Engineered
Escherichia Coli KJ122, Int. J. Mol. Sci., 2025, 26(3), 982, DOI:10.3390/IJMS26030982.
- Y. He, X. Li, H. Ben, X. Xue and B. Yang, Lipid Production from Dilute Alkali Corn Stover Lignin by Rhodococcus Strains, ACS Sustain. Chem. Eng., 2017, 5(3), 2302–2311, DOI:10.1021/acssuschemeng.6b02627.
- A. Z. Werner, W. T. Cordell, C. W. Lahive, B. C. Klein, C. A. Singer, E. C. D. Tan, M. A. Ingraham, K. J. Ramirez, D. H. Kim, J. N. Pedersen, C. W. Johnson, B. F. Pfleger, G. T. Beckham and D. Salvachúa, Lignin Conversion to β-Ketoadipic Acid by Pseudomonas Putida via Metabolic Engineering and Bioprocess Development, Sci. Adv., 2023, 9(36), eadj0053, DOI:10.1126/sciadv.adj0053.
- R. J. Protzko, L. N. Latimer, Z. Martinho, E. de Reus, T. Seibert, J. P. Benz and J. E. Dueber, Engineering Saccharomyces Cerevisiae for Co-Utilization of d-Galacturonic Acid and d-Glucose from Citrus Peel Waste, Nat. Commun., 2018, 9(1), 5059, DOI:10.1038/s41467-018-07589-w.
- P. Yang, Y. Wu, Z. Zheng, L. Cao, X. Zhu, D. Mu and S. Jiang, CRISPR-Cas9 Approach Constructing Cellulase Sestc-Engineered Saccharomyces Cerevisiae for the Production of Orange Peel Ethanol, Front. Microbiol., 2018, 9, 2436, DOI:10.3389/fmicb.2018.02436.
- J. M. Jung, J. Y. Kim, J. H. Kim, S. M. Kim, S. Jung, H. Song, E. E. Kwon and Y. E. Choi, Zero-Waste Strategy by Means of Valorization of Bread Waste, J. Cleaner Prod., 2022, 365, 132795, DOI:10.1016/j.jclepro.2022.132795.
- J. C. Sadler and S. Wallace, Microbial Synthesis of Vanillin from Waste Poly(Ethylene Terephthalate), Green Chem., 2021, 23(13), 4665–4672, 10.1039/d1gc00931a.
- M. Valenzuela-Ortega, J. T. Suitor, M. F. M. White, T. Hinchcliffe and S. Wallace, Microbial Upcycling of Waste PET to Adipic Acid, ACS Cent. Sci., 2023, 9(11), 2057–2063, DOI:10.1021/acscentsci.3c00414.
- H. T. Kim, J. K. Kim, H. G. Cha, M. J. Kang, H. S. Lee, T. U. Khang, E. J. Yun, D. H. Lee, B. K. Song, S. J. Park, J. C. Joo and K. H. Kim, Biological Valorization of Poly(Ethylene Terephthalate) Monomers for Upcycling Waste PET, ACS Sustain. Chem. Eng., 2019, 7(24), 19396–19406, DOI:10.1021/acssuschemeng.9b03908.
- T. Bao, Y. Qian, Y. Xin, J. J. Collins and T. Lu, Engineering Microbial Division of Labor for Plastic Upcycling, Nat. Commun., 2023, 14(1), 5712, DOI:10.1038/s41467-023-40777-x.
- C. Rabot, Y. Chen, S. Y. Lin, B. Miller, Y. M. Chiang, C. E. Oakley, B. R. Oakley, C. C. C. Wang and T. J. Williams, Polystyrene Upcycling into Fungal Natural Products and a Biocontrol Agent, J. Am. Chem. Soc., 2023, 145(9), 5222–5230, DOI:10.1021/jacs.2c12285.
- C. Rabot, Y. Chen, S. Bijlani, Y. M. Chiang, C. E. Oakley, B. R. Oakley, T. J. Williams and C. C. C. Wang, Conversion of Polyethylenes into Fungal Secondary Metabolites, Angew. Chem., Int. Ed., 2023, 62(4), e202214609, DOI:10.1002/anie.202214609.
- K. P. Sullivan, A. Z. Werner, K. J. Ramirez, L. D. Ellis, J. R. Bussard, B. A. Black, D. G. Brandner, F. Bratti, B. L. Buss, X. Dong, S. J. Haugen, M. A. Ingraham, M. O. Konev, W. E. Michener, J. Miscall, I. Pardo, S. P. Woodworth, A. M. Guss, Y. Román-Leshkov, S. S. Stahl and G. T. Beckham, Mixed Plastics Waste Valorization through Tandem Chemical Oxidation and Biological Funneling, Science, 2022, 378(6616), 207–211, DOI:10.1126/science.abo4626.
- G. Wu, Q. Yan, J. A. Jones, Y. J. Tang, S. S. Fong and M. A. G. Koffas, Metabolic Burden: Cornerstones in Synthetic Biology and Metabolic Engineering Applications, Trends Biotechnol., 2016, 34(8), 652–664, DOI:10.1016/J.TIBTECH.2016.02.010.
- G. W. Roell, J. Zha, R. R. Carr, M. A. Koffas, S. S. Fong and Y. J. Tang, Engineering Microbial Consortia by Division of Labor, Microb. Cell Fact., 2019, 18(1), 1–11, DOI:10.1186/S12934-019-1083-3.
- J. A. Jones and X. Wang, Use of Bacterial Co-Cultures for the Efficient Production of Chemicals, Curr. Opin. Biotechnol., 2018, 53, 33–38, DOI:10.1016/j.copbio.2017.11.012.
- R. Wang, S. Zhao, Z. Wang and M. A. Koffas, Recent Advances in Modular Co-Culture Engineering for Synthesis of Natural Products, Curr. Opin. Biotechnol., 2020, 62, 65–71, DOI:10.1016/j.copbio.2019.09.004.
-
A. Selvam, P. M. K. Ilamathi, M. Udayakumar, K. Murugesan, J. R. Banu, Y. Khanna and J. Wong, Food Waste Properties, in Current Developments in Biotechnology and Bioengineering: Sustainable Food Waste Management: Resource Recovery and Treatment, ed. Wong, J., Kaur, G., Taherzadeh, M., Pandey, A. and Lasaridi, K., Elsevier, 2020, pp. 11–41, DOI:10.1016/B978-0-12-819148-4.00002-6.
- C. Moretti, B. Corona, R. Hoefnagels, I. Vural-Gürsel, R. Gosselink and M. Junginger, Review of Life Cycle Assessments of Lignin and Derived Products: Lessons Learned, Sci. Total Environ., 2021, 770, 144656, DOI:10.1016/J.SCITOTENV.2020.144656.
- Z. Sun, B. Fridrich, A. De Santi, S. Elangovan and K. Barta, Bright Side of Lignin Depolymerization: Toward New Platform Chemicals, Chem. Rev., 2018, 118(2), 614–678, DOI:10.1021/ACS.CHEMREV.7B00588.
- D. Krishna and A. S. Kalamdhad, Pre-Treatment and Anaerobic Digestion of Food Waste for High Rate Methane Production - A Review, J. Environ. Chem. Eng., 2014, 2(3), 1821–1830, DOI:10.1016/j.jece.2014.07.024.
- L. M. G. Saye, T. A. Navaratna, J. P. J. Chong, M. A. O’malley, M. K. Theodorou and M. Reilly, The Anaerobic Fungi: Challenges and Opportunities for Industrial Lignocellulosic Biofuel Production, Microorganisms, 2021, 9(4), 694, DOI:10.3390/microorganisms9040694.
-
K. Laçın, B. Çaloğlu and B. Binay, Anaerobic Digestion Methods for the Production of Fuels, in Bioenergy Engineering: Fundamentals, Methods, Modelling, and Applications, ed. Shadangi, K. P., Sarangi, P. K., Mohanty, K., Deniz, I. and Kiran Gollakota, A. R., Woodhead Publishing, 2023, pp. 201–235, DOI:10.1016/B978-0-323-98363-1.00004-1.
- Y. Ren, M. Yu, C. Wu, Q. Wang, M. Gao, Q. Huang and Y. Liu, A Comprehensive Review on Food Waste Anaerobic Digestion: Research Updates and Tendencies, Bioresour. Technol., 2018, 247, 1069–1076, DOI:10.1016/j.biortech.2017.09.109.
- F. Xu, Y. Li, X. Ge, L. Yang and Y. Li, Anaerobic Digestion of Food Waste – Challenges and Opportunities, Bioresour. Technol., 2018, 247, 1047–1058, DOI:10.1016/j.biortech.2017.09.020.
- V. Narisetty, R. Cox, N. Willoughby, E. Aktas, B. Tiwari, A. S. Matharu, K. Salonitis and V. Kumar, Recycling Bread Waste into Chemical Building Blocks Using a Circular Biorefining Approach, Sustainable Energy Fuels, 2021, 5(19), 4842–4849, 10.1039/d1se00575h.
-
R. Geyer, Production, Use, and Fate of Synthetic Polymers, Elsevier Inc., 2020, DOI:10.1016/b978-0-12-817880-5.00002-5.
- R. Geyer, J. R. Jambeck and K. L. Law, Production, Use, and Fate of All Plastics Ever Made, Sci. Adv., 2017, 3(7), e1700782, DOI:10.1126/sciadv.1700782.
-
OECD iLibrary, Global Plastics Outlook, https://www.oecd-ilibrary.org/environment/data/global-plastic-outlook_c0821f81-en, accessed 2024-07-23 Search PubMed.
- N. Taghavi, I. A. Udugama, W. Q. Zhuang and S. Baroutian, Challenges in Biodegradation of Non-Degradable Thermoplastic Waste: From Environmental Impact to Operational Readiness, Biotechnol. Adv., 2021, 49, 107731, DOI:10.1016/j.biotechadv.2021.107731.
- E. Nikolaivits, B. Pantelic, M. Azeem, G. Taxeidis, R. Babu, E. Topakas, M. Brennan Fournet and J. Nikodinovic-Runic, Progressing Plastics Circularity: A Review of Mechano-Biocatalytic Approaches for Waste Plastic (Re)Valorization, Front. Bioeng. Biotechnol., 2021, 9, 696040, DOI:10.3389/fbioe.2021.696040.
- R. Balu, N. K. Dutta and N. Roy Choudhury, Plastic Waste Upcycling: A Sustainable Solution for Waste Management, Product Development, and Circular Economy, Polymers, 2022, 14(22), 4788, DOI:10.3390/polym14224788.
- X. E. Crystal Thew, S. C. Lo, R. N. Ramanan, B. T. Tey, N. D. Huy and O. Chien Wei, Enhancing Plastic Biodegradation Process: Strategies and Opportunities, Crit. Rev. Biotechnol., 2024, 44(3), 477–494, DOI:10.1080/07388551.2023.2170861.
- H. Ballerstedt, T. Tiso, N. Wierckx, R. Wei, L. Averous, U. Bornscheuer, K. O'Connor, T. Floehr, A. Jupke, J. Klankermayer, L. Liu, V. de Lorenzo, T. Narancic, J. Nogales, R. Perrin, E. Pollet, A. Prieto, W. Casey, T. Haarmann, A. Sarbu, U. Schwaneberg, F. Xin, W. Dong, J. Xing, G. Q. Chen, T. Tan, M. Jiang and L. M. Blank, MIXed Plastics Biodegradation and UPcycling Using Microbial Communities: EU Horizon 2020 Project MIX-UP Started January 2020, Environ. Sci. Eur., 2021, 33(1), 99, DOI:10.1186/s12302-021-00536-5.
-
R. Andler, T. Tiso, L. Blank, C. Andreeßen, J. Zampolli, V. D'Afonseca, C. Guajardo and A. Díaz-Barrera, Current Progress on the Biodegradation of Synthetic Plastics: From Fundamentals to Biotechnological Applications, Reviews in Environmental Science and Biotechnology, Springer, 2022, pp. 829–850, doi: DOI:10.1007/s11157-022-09631-2.
- T. Tiso, B. Winter, R. Wei, J. Hee, J. de Witt, N. Wierckx, P. Quicker, U. T. Bornscheuer, A. Bardow, J. Nogales and L. M. Blank, The Metabolic Potential of Plastics as Biotechnological Carbon Sources – Review and Targets for the Future, Metab. Eng., 2022, 71, 77–98, DOI:10.1016/j.ymben.2021.12.006.
- M. L. Lepage and J. E. Wulff, Mixed Plastics Upcycled Dynamically, Nature, 2023, 616(7958), 663–664, DOI:10.1038/d41586-023-01352-y.
- K. Ragaert, L. Delva and K. Van Geem, Mechanical and Chemical Recycling of Solid Plastic Waste, Waste Manage., 2017, 69, 24–58, DOI:10.1016/j.wasman.2017.07.044.
- W. Purahong, S. F. M. Wahdan, D. Heinz, K. Jariyavidyanont, C. Sungkapreecha, B. Tanunchai, C. Sansupa, D. Sadubsarn, R. Alaneed, A. Heintz-Buschart, M. Schädler, A. Geissler, J. Kressler and F. Buscot, Back to the Future: Decomposability of a Biobased and Biodegradable Plastic in Field Soil Environments and Its Microbiome under Ambient and Future Climates, Environ. Sci. Technol., 2021, 55(18), 12337–12351, DOI:10.1021/ACS.EST.1C02695.
- C. Gioia, G. Giacobazzi, M. Vannini, G. Totaro, L. Sisti, M. Colonna, P. Marchese and A. Celli, End of Life of Biodegradable Plastics: Composting versus Re/Upcycling, ChemSusChem, 2021, 14(19), 4167–4175, DOI:10.1002/cssc.202101226.
-
Directorate-General for Environment, EU Policy Framework on Biobased, Biodegradable and Compostable Plastics, 2022, https://environment.ec.europa.eu/publications/communication-eu-policy-framework-biobased-biodegradable-and-compostable-plastics_en Search PubMed.
- A. Mo, Y. Zhang, W. Gao, J. Jiang and D. He, Environmental Fate and Impacts of Biodegradable Plastics in Agricultural Soil Ecosystems, Appl. Soil Ecol., 2023, 181, 104667, DOI:10.1016/J.APSOIL.2022.104667.
- Y. Sun, C. Duan, N. Cao, C. Ding, Y. Huang and J. Wang, Biodegradable and Conventional Microplastics Exhibit Distinct Microbiome, Functionality, and Metabolome Changes in Soil, J. Hazard. Mater., 2022, 424, 127282, DOI:10.1016/J.JHAZMAT.2021.127282.
- A. Singh, N. A. Rorrer, S. R. Nicholson, E. Erickson, J. S. DesVeaux, A. F. T. Avelino, P. Lamers, A. Bhatt, Y. Zhang, G. Avery, L. Tao, A. R. Pickford, A. C. Carpenter, J. E. McGeehan and G. T. Beckham, Techno-Economic, Life-Cycle, and Socioeconomic Impact Analysis of Enzymatic Recycling of Poly(Ethylene Terephthalate), Joule, 2021, 5(9), 2479–2503, DOI:10.1016/j.joule.2021.06.015.
- T. Uekert, J. S. DesVeaux, A. Singh, S. R. Nicholson, P. Lamers, T. Ghosh, J. E. McGeehan, A. C. Carpenter and G. T. Beckham, Life Cycle Assessment of Enzymatic Poly(Ethylene Terephthalate) Recycling, Green Chem., 2022, 24(17), 6531–6543, 10.1039/d2gc02162e.
- A. Corona, M. J. Biddy, D. R. Vardon, M. Birkved, M. Z. Hauschild and G. T. Beckham, Life Cycle Assessment of Adipic Acid Production from Lignin, Green Chem., 2018, 20(16), 3857–3866, 10.1039/c8gc00868j.
- F. Vidal, E. R. van der Marel, R. W. F. Kerr, C. McElroy, N. Schroeder, C. Mitchell, G. Rosetto, T. T. D. Chen, R. M. Bailey, C. Hepburn, C. Redgwell and C. K. Williams, Designing a Circular Carbon and Plastics Economy for a Sustainable Future, Nature, 2024, 626(7997), 45–57, DOI:10.1038/s41586-023-06939-z.
- A. Singh, N. A. Rorrer, S. R. Nicholson, E. Erickson, J. S. DesVeaux, A. F. T. Avelino, P. Lamers, A. Bhatt, Y. Zhang, G. Avery, L. Tao, A. R. Pickford, A. C. Carpenter, J. E. McGeehan and G. T. Beckham, Techno-Economic, Life-Cycle, and Socioeconomic Impact Analysis of Enzymatic Recycling of Poly(Ethylene Terephthalate), Joule, 2021, 5(9), 2479–2503, DOI:10.1016/j.joule.2021.06.015.
- V. de Lorenzo and J. Couto, The Important versus the Exciting: Reining Contradictions in Contemporary Biotechnology, Microb. Biotechnol., 2019, 12(1), 32–34, DOI:10.1111/1751-7915.13348.
- N. Mahendrasinh Kosamia, M. Samavi, K. Piok and S. Kumar Rakshit, Perspectives for Scale up of Biorefineries Using Biochemical Conversion Pathways: Technology Status, Techno-Economic, and Sustainable Approaches, Fuel, 2022, 324, 124532, DOI:10.1016/j.fuel.2022.124532.
-
P. Neubauer and S. Junne, Scale-Up and Scale-Down Methodologies for Bioreactors, in Bioreactors, John Wiley & Sons, Ltd, 2016, pp. 323–354, DOI:10.1002/9783527683369.ch11.
- C. Huang, Y. Zheng, W. Lin, Y. Shi, G. Huang and Q. Yong, Removal of Fermentation Inhibitors from Pre-Hydrolysis Liquor Using Polystyrene Divinylbenzene Resin, Biotechnol. Biofuels, 2020, 13(1), 1–14, DOI:10.1186/S13068-020-01828-3.
- Z. Honarmandrad, K. Kucharska, M. Kaykhaii and J. Gębicki, Removal of Phenolic Inhibitor Compounds from Hydrolysates and Post-Fermentation Broths by Using a Hydrophobic Magnetic Deep Eutectic Solvent, J. Environ. Chem. Eng., 2024, 12(3), 112621, DOI:10.1016/J.JECE.2024.112621.
- K. J. Tomek, C. R. C. Saldarriaga, F. P. C. Velasquez, T. Liu, D. B. Hodge and T. A. Whitehead, Removal and Upgrading of Lignocellulosic Fermentation Inhibitors by in Situ Biocatalysis and Liquid-Liquid Extraction, Biotechnol. Bioeng., 2015, 112(3), 627–632, DOI:10.1002/BIT.25473.
- F. H. Arnold, Directed Evolution: Bringing New Chemistry to Life, Angew. Chem., Int. Ed., 2018, 57(16), 4143–4148, DOI:10.1002/anie.201708408.
- E. M. Brustad and F. H. Arnold, Optimizing Non-Natural Protein Function with Directed Evolution, Curr. Opin. Chem. Biol., 2011, 15(2), 201–210, DOI:10.1016/j.cbpa.2010.11.020.
- H. Renata, Z. J. Wang and F. H. Arnold, Expanding the Enzyme Universe: Accessing Non-Natural Reactions by Mechanism-Guided Directed Evolution, Angew. Chem., Int. Ed., 2015, 54(11), 3351–3367, DOI:10.1002/anie.201409470.
- J. C. Lewis, P. S. Coelho and F. H. Arnold, Enzymatic Functionalization of Carbon-Hydrogen Bonds, Chem. Soc. Rev., 2011, 40(4), 2003–2021, 10.1039/c0cs00067a.
- J. C. Sadler, The Bipartisan Future of Synthetic Chemistry and Synthetic Biology, ChemBioChem, 2020, 21(24), 3489–3491, DOI:10.1002/cbic.202000418.
- J. C. Sadler, J. A. Dennis, N. W. Johnson and S. Wallace, Interfacing Non-Enzymatic Catalysis with Living Microorganisms, RSC Chem. Biol., 2021, 2(4), 1073–1083, 10.1039/d1cb00072a.
- S. Wallace and E. P. Balskus, Interfacing Microbial Styrene Production with a Biocompatible Cyclopropanation Reaction, Angew. Chem., Int. Ed., 2015, 54(24), 7106–7109, DOI:10.1002/anie.201502185.
- S. Wallace and E. P. Balskus, Opportunities for Merging Chemical and Biological Synthesis, Curr. Opin. Biotechnol., 2014, 30, 1–8, DOI:10.1016/J.COPBIO.2014.03.006.
- S. Grigary and M. Umesh, Floral Waste as a Potential Feedstock for Polyhydroxyalkanoate Production Using Halotolerant Bacillus Cereus TS1: Optimization and Characterization Studies, Biomass Convers. Biorefin., 2023 DOI:10.1007/s13399-023-05003-0.
- N. Pensupa, S. Y. Leu, Y. Hu, C. Du, H. Liu, H. Jing, H. Wang and C. S. K. Lin, Recent Trends in Sustainable Textile Waste Recycling Methods: Current Situation and Future Prospects, Top. Curr. Chem., 2017, 375(5), 76, DOI:10.1007/s41061-017-0165-0.
- V. Thirumurugan, A Review Article on “Fast Fashion”, Curr. Trends Fashion Technol. Textile Eng., 2019, 5(5), 555675, DOI:10.19080/ctftte.2019.05.555675.
- E. M. Barampouti, A. Christofi, D. Malamis and S. Mai, A Sustainable Approach to Valorize Potato Peel Waste towards Biofuel Production, Biomass Convers. Biorefin., 2023, 13(9), 8197–8208, DOI:10.1007/s13399-021-01811-4.
- A. Soltaninejad, M. Jazini and K. Karimi, Sustainable Bioconversion of Potato Peel Wastes into Ethanol and Biogas Using Organosolv Pretreatment, Chemosphere, 2022, 291, 133003, DOI:10.1016/j.chemosphere.2021.133003.
- I. A. Escanciano, V. E. Santos, Á. Blanco and M. Ladero, Bioproduction of Succinic Acid from Potato Waste. Kinetic Modeling, Ind. Crops Prod., 2023, 203, 117124, DOI:10.1016/j.indcrop.2023.117124.
- M. Tian and Q. Yuan, Optimization of Phytase Production from Potato Waste Using Aspergillus Ficuum, 3 Biotech, 2016, 6(2), 256, DOI:10.1007/s13205-016-0573-9.
|
This journal is © The Royal Society of Chemistry 2025 |
Click here to see how this site uses Cookies. View our privacy policy here.