Evaluation of the alkyl chain length and photocatalytic antibacterial performance of cation g-C3N4†
Received
23rd May 2024
, Accepted 1st November 2024
First published on 4th November 2024
Abstract
Several cation graphite carbon nitrides (g-C3N4-(CH2)n-ImI+) were synthesized by chemically attaching imidazolium appended alkane chains with different lengths (n = 2, 4, 8, 12 and 16) to g-C3N4. The introduction of a cation segment potentially improved the interaction between the carbon material and Gram negative (MDR-A. baumannii) and Gram positive (S. aureus) bacteria as characterized by ζ potential measurement. Short alkane chain (carbon numbers of 2, 4 and 8) carbon materials displayed relatively stronger bacterial interactions compared to long alkane chain bearing ones (n = 12 and 16). In addition, short chain carbon materials (g-C3N4-(CH2)4-ImI+) displayed relatively higher photocatalytic reactive oxygen species (1O2, ˙O2− and ˙OH) production efficiency. Bacterial interaction and ROS production efficiency synergistically contribute to photocatalytic antibacterial performance. The current data revealed that g-C3N4 with short flexible cations attached exhibited bacterial interaction and ROS production. Among these synthesized materials, g-C3N4-(CH2)4-ImI+ exhibited the most pronounced photocatalytic antibacterial efficiency (>99%).
1. Introduction
Bacterial infection remains one of the most critical and human health threating issues all over the world.1,2 Despite exhibiting promising outcomes in antibacterial applications, chemo-drugs can lead to the development of resistance mechanisms in bacteria upon long term use.3,4 Thus, inappropriate application of antibiotics causes generation of multi-drug resistant bacteria, which can eventually lead to a loss of drug effectiveness.5–7 In addition to chemotherapy, photodynamic, photocatalytic and photothermal methods are regarded as potential strategies against drug resistance,8–13 as the active components (reactive oxygen species (ROS) and heat) would possibly not trigger corresponding resistance mechanisms. Owing to this advantage, plenty of photoactive materials for bacterial killing have been fabricated with promising outcomes.14–21
One challenge of photothermal antibacterial materials is to better control the generated heat, which could reduce neighboring tissue injury during the treatment. In contrast, photodynamic and photocatalytic materials were considered to be relatively safer in this aspect.22 Photodynamic antibacterial materials typically transform triplet oxygen molecules (3O2) to singlet oxygen molecules (1O2) under light irradiation. The 1O2 subsequently damages the bacterial membrane and bioactive molecules, which could result in the death of the bacteria.23 Currently, photoactive organic molecules, metal–organic complexes and conjugated polymers are the main photodynamic materials.12 The syntheses are relatively complicated and also these materials displayed certain biocompatibility concerns.11,24,25 The mechanism for photocatalytic antibacterial materials is photo-assisted generation of super oxide anions (˙O2−), hydroxyl free radicals (˙OH) and another species. Graphite carbon nitride (g-C3N4) is one of classical photocatalytic antibacterials with the merits of a facile synthesis procedure and acceptable biocompatibility.26–29 Intrinsic g-C3N4 displayed limited ROS generation capability and short wavelength absorbance. Accordingly, chemical doping with metal and nonmetal elements in the triazine skeleton of g-C3N4 with the purpose of tuning ROS efficiency and absorption profile is one of the main research focuses, and the antibacterial efficiency indeed increased.30–39 Yet, most of these materials lack sufficient interaction between bacteria due to the hydrophobic nature of g-C3N4. This was suspected to be critical in bacterial killing as well, because intense interaction could facilitate enhanced usage of short life time ROS.40 Thus, ionic g-C3N4 and the related antibacterial performance remain to be explored.
In this study, we attached imidazolium segments into alkane-chains with different lengths bearing g-C3N4 to yield ionic carbon nitride (g-C3N4-(CH2)n-ImI+). The choice of imidazolium was based on the report that imidazolium bearing materials could influence the bacterial membrane and exhibit intense antimicrobial activity.41–43 We found that the introduction of a cation segment obviously increased the antibacterial efficiency in both Gram negative (MDR-A. baumannii) and Gram positive (S. aureus) bacteria strains compared to intrinsic g-C3N4. Furthermore, the results revealed that the antibacterial performance was dependent on the alkane chain length. The middle length alkane chain (n = 8) facilitated maximum bacterial interaction. Middle and short alkane chains (n = 2, 4 and 8) resulted in better light-assisted antibacterial killing efficiency (>95%) compared to intrinsic g-C3N4 (∼75%) and long alkane chains (n = 12 (∼89%) and 16 (∼86%)). The most potent antibacterial performance (>99%) was obtained with g-C3N4-(CH2)4-ImI+. Photoelectrochemical measurements suggest that g-C3N4-(CH2)4-ImI+ featured better ROS production efficiency. We hope that these results could serve as hints for future g-C3N4 based antibacterial material design.
2. Experimental section
2.1 Materials and instruments
Melamine was purchased from China National Pharmaceutical Group Corporation. Chemicals for g-C3N4 derivation including 1,2-dibromomethane, 1,4-dibromobutane, 1,8-dibromooctane, 1,12-dibromododecane, 1,16-dibromocetane and N-methylimidazole were purchased from Shanghai Aladdin Biochemical Technology Co., Ltd and used without further purification. ROS indicators and reference compound including 9,10-anthracenediyl-bis(methylene)dimalonic acid (ABDA), 5,5-dimethyl-1-pyrroline N-oxide, rose bengal (RB), benzoic acid and nitroblue tetrazolium were purchased from Macklin Inc. The clinical A. baumannii strain AB43 was isolated from the affiliated hospital of Yangzhou University in Jiangsu, China. The species was identified using Gram stain, 16S RNA sequencing, and the VITEK-2 system (bioMerieux, Marcy l’Etoile, France) in the clinical microbiology laboratory. S. aureus was purchased from ATCC with strain no. 29213. Chemicals for bacteria culture were purchased from China National Pharmaceutical Group Corporation. UV-vis spectra were recorded on a PerkinElmer Lambada 650 spectrometer. Fluorescent spectra were recorded on a Hitachi F-2700 spectrometer. ˙OH quantitative analysis was achieved using a Shimadzu LC-20A HPLC equipped with a UV-vis detector. SEM images were taken on a Carl Zeiss Gemini SEM 300 microscope. Raman spectra were recorded on a Renishaw inVia spectrometer. XRD spectra were recorded on a Bruker D8 Advance instrument. ζ Potentials were measured using a Malvern ZEN 3690 instrument. Transient photocurrent measurements, electrochemical impedance spectroscopy test and Mott–Schottky measurements were achieved on a CHI 660B workstation. Glassy carbon was used as a working electrode, Pt was used as a counter electrode, saturated calomel was used as a reference electrode and 0.5 M Na2SO4 solution was used as the electrolyte. Solid 13C NMR spectra were recorded using an Agilent DD2 spectrometer. The CEL-HXF 300 Xeon light source with a filter cutter of 400 nm from China Education Au-Light Co. Ltd. was used as the light source for antibacterial experiments. The light intensity was measured using a CEL-NP 2000 optical power meter.
2.2 Synthesis of the g-C3N4 precursor
The precursor was synthesized according to a standard protocol.44,45 10.00 g of melamine was calcined at 550 °C for 4 hours with a temperature increase rate of 5 °C min−1 to yield a light yellow solid.
2.3 Synthesis of g-C3N4-(CH2)n-Br
The resultant precursor was ground into fine powder and mixed with 100 mM of dibromo alkane (1,2-dibromoethane, 1,4-dibromobutane, 1,8-dibromooctane, 1,12-dibromododecane and 1,16-dibromocetane) and 100 mM of K2CO3 in 20 mL of DMSO. The mixture was subsequently heated at 90 °C for 12 hours followed by quenching and washing with 200 mL of petroleum ether to yield a yellow solid.
2.4 Synthesis of g-C3N4-(CH2)n-ImI+
1.00 g of synthesized g-C3N4-(CH2)n-Br was mixed with 0.50 g of N-methylimidazole in 10 mL of DMSO and heated at 70 °C for 12 hours. Subsequently, the reaction mixture was quenched by the addition of 50 mL of petroleum ether. The resulting precipitate was collected by filtration as a desired product.
2.5
1O2 detection
Rose bengal (RB) and g-C3N4-(CH2)n-ImI+ were dispersed in Milli-Q water to reach a final concentration of 2.50 μM and 50 μg mL−1. 1O2 sensor 9,10-anthracenediyl-bis(methylene)dimalonic acid (ABDA) was then added to these solutions to reach a final concentration of 50 μM. The resulting dispersion was then subjected to white light irradiation (100 mW cm−2) for a total of 120 min. UV-vis spectra were recorded every 20 min, and the absorbance decrease at 378 nm was used to characterize the decomposition of ABDA by 1O2.
2.6 ˙OH and ˙O2− detection
1.00 mg of g-C3N4-(CH2)n-ImI+ was dispersed in 1 mL of freshly prepared nitrogen saturated H2O (for ˙OH) and absolute methanol (for ˙O2−). The sample was subsequently added to 0.10 mM of 5,5-dimethyl-1-pyrroline N-oxide and immediately placed on an EPR spectrometer. The measurement was conducted under light irradiation (100 mW cm−2) for 20 s at room temperature.
2.7 ˙OH and ˙O2− quantification
A water dispersion of carbon materials (1 mg mL−1) was added to a round-bottomed flask with a rubber stopper, followed by Ar gas bubbling to remove oxygen. Subsequently, benzoic acid (BA) solution (20 mL, 10.4 mmol L−1) was continuously bubbled with Ar gas for 30 min to purge the oxygen and subsequently pipetted into the round-bottomed flask in an inert atmosphere. Then a certain amount of H2O2 was pipetted into BA solution to trigger the reaction under shaking in order to make the standard curve. The g-C3N4-(CH2)n-ImI+ suspension was irradiated with white light (100 mW cm−2) for 2 hours, and the resulting reaction solution was collected and filtrated through 0.22 μm membranes. HPLC was used to determine the p-hydroxybenzoic acid (p-HBA) concentration. The mobile phase was acetonitrile/water (30/70, v
:
v) and the detection wavelength was 270 nm. It should be noted that that 5.87 ± 0.18 moles ˙OH reacting with BA produced one mole p-HBA. The cumulative ˙OH concentration is therefore approximately 5.87 times that of p-HBA concentration. 1 mol of nitroblue tetrazolium (NBT) can react with 4 mol of ˙O2−. We quantified the concentration of generated ˙O2− in the Fenton suspension by recording the residual concentration of NBT on a UV-vis spectrophotometer (maximum absorbance at 270 nm). The experimental procedure was similar to that of ˙OH determination experiments except replacing BA with NBT.
2.8 Preparation of bacterial dispersion
A single colony of MDR-A. baumannii (Gram-negative) and S. aureus (Gram-positive) was cultured in 5 mL of liquid Luria-Broth (LB) culture medium at 37 °C for 3 hours. The bacteria were harvested using a centrifuge. The collected bacteria were then washed using 1× PBS three times followed by resuspension in 1× PBS. The bacterial dispersion was adjusted to be 0.5 at 600 nm as measured using a UV-vis spectrometer.
2.9
ζ potential measurements
100 μL of MDR-A. baumannii and S. aureus dispersion (in 1× PBS, 104 CFU mL−1) was subjected to ζ potential measurements and the results were recorded, respectively. 1 mL of water dispersion of g-C3N4-(CH2)n-ImI+ (1 mg mL−1) was subjected to ζ potential measurements and the results were recorded, respectively. 100 μL of MDR-A. baumannii and S. aureus dispersion (in 1× PBS, 104 CFU mL−1) was mixed with 1 mL of water dispersion of g-C3N4-(CH2)n-ImI+ (1 mg mL−1) and incubated at 25 °C for 4 hours in the dark, respectively. The resulting bacteria were then collected by centrifuging at 5000 rpm for 5 min and re-dispersed in 1 mL of 1× PBS. The corresponding ζ potential measurements and the results were recorded, respectively.
2.10 Photocatalytic antibacterial performance of g-C3N4-(CH2)n-ImI+
20 μL of bacteria dispersion (MDR-A. baumannii and S. aureus) were transferred to a solid LB culture medium on 24-well plates (1.0 × 107 CFU mL−1). PBS solution of organometallic PSs was added to the bacteria dispersion. The final concentration of g-C3N4-(CH2)n-ImI+ for MDR-A. baumannii and S. aureus was 1 mg mL−1. After incubation of bacteria with the synthesized material for 20 min at 37 °C in the dark, white light (100 mW cm−2) was applied on the bacteria for 20, 40, 60, 80, 100 and 120 min. The bacteria with addition of neat g-C3N4 and without addition of g-C3N4 were used as the control. In the dark toxicity experiment, no light irradiation was applied on bacteria. After these treatments, 10 μL of bacteria dispersion was taken and diluted 103 fold and cultured on new solid LB medium in the dark for 24 hours. The bacterial killing experiment in the water supply was conducted using a similar procedure to the one above, using Slender West Lake, the Yangtze River, and urban water supply (N32°24′, E119°26′) instead of bacterial dispersion. All experiments were repeated 3 times. The antibacterial efficiency (Eb) was calculated using the formula:
where, C0 stands for the colony number of control group, and C stands for the colony number of the individual experimental group.
2.11 Cell viability of g-C3N4-(CH2)n-ImI+
Mouse fibroblast cells were seeded in a 96-well plate with a cell density of 5 × 103. Afterwards, the cells were incubated at 37 °C with 5% CO2 for 24 hours. g-C3N4-(CH2)n-ImI+ dispersion were mixed with cell culture to make solutions with a final concentration of 1 mg mL−1. The resulting solutions were subsequently added to the 96-well plate and the cells were further incubated in the dark at 37 °C with 5% CO2 for another 24 hours. Finally, the cell viability was analyzed using a CCK-8 cell counting kit according to the standard protocol. The experiments were done in triplicate.
3. Results and discussion
3.1 Syntheses and characterization of g-C3N4-(CH2)n-ImI+
The precursor g-C3N4 was synthesized through a classical method using melamine as the starting material. Typically, this synthesis method would yield g-C3N4 with reactive amine groups on the edge. Next, the obtained g-C3N4 reacted with excessive amount of dibromo alkane with different chain lengths to afford single bromo bearing g-C3N4-(CH2)n-Br (n = 2, 4, 8, 12 and 16). Finally, N-methyl imidazole reacted with the bromo bearing material to yield the desired material of g-C3N4-(CH2)n-ImI+ (Scheme 1).
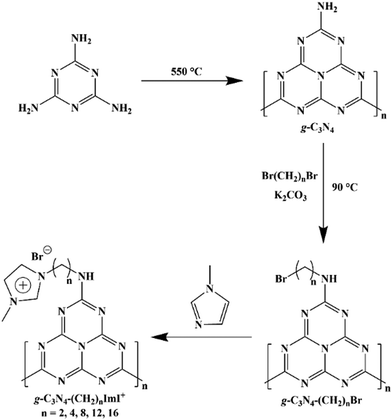 |
| Scheme 1 Synthetic route to g-C3N4-(CH2)n-ImI+. | |
The FT-IR spectra of carbon materials exhibited multiple peaks in the range of 1750–750 cm−1, which agreed with the spectral profile of synthesized g-C3N4 (Fig. 1a). To note, the classical triazine vibration peak (802 cm−1) has been observed in all the spectra. More intense signals in the range of 2700–3600 cm−1 have been observed with an increase in the alkane chain length. The C–H stretching signal in the range of 2830–2950 cm−1 that can be assigned to the alkane chain has also been observed in g-C3N4-(CH2)n-ImI+ with n = 4, 6, 8, 12 and 16 (Fig. 1b). The undetectable C–H stretching signal in g-C3N4-(CH2)2-ImI+ was suspected to be due to the relatively short chain length. Solid 13C NMR spectra of synthesized materials were recorded to further elaborate these structures. As shown in Fig. 1c, all the g-C3N4 materials exhibited identical peaks with δ of 244.25, 164.83, 156.54 and 85.22 ppm, suggesting that the decorating reaction did not break the skeleton of intrinsic g-C3N4. g-C3N4-(CH2)2-ImI+ exhibited a signal at 43.18 ppm, which could be assigned to the carbon in the alkane chain that connects the N-imidazole segment (Fig. 1d). For n = 4 to 16, the corresponding materials showed gradually increasing peak intensity around 30 ppm, indicating the successful introduction of long alkane chains.
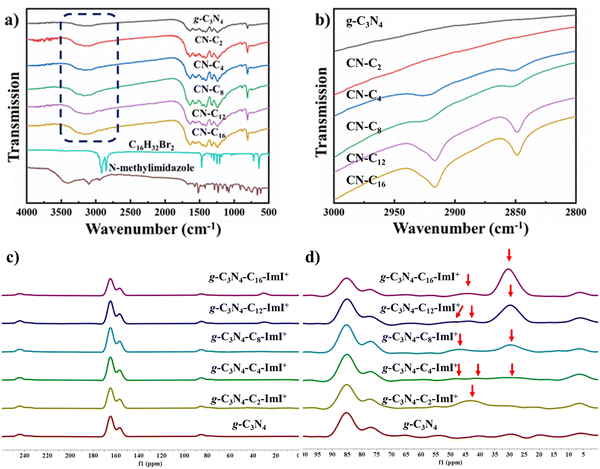 |
| Fig. 1 (a) Full FT-IR spectra of g-C3N4, g-C3N4-(CH2)n-ImI+ (n = 2, 4, 8, 12 and 16) and 1,16-dibromocetane (wavelength from 4000 to 500 cm−1). (b) Partial FT-IR spectra of g-C3N4, g-C3N4-(CH2)n-ImI+ (n = 2, 4, 8, 12 and 16) and 1,16-dibromocetane (wavelength from 3000 to 2800 cm−1). (c) Full solid 13C NMR spectra of g-C3N4 and g-C3N4-(CH2)n-ImI+ (n = 2, 4, 8, 12 and 16) (chemical shift from 280 to 0 ppm). (d) Partial solid 13C NMR spectra of g-C3N4 and g-C3N4-(CH2)n-ImI+ (n = 2, 4, 8, 12 and 16) (chemical shift from 100 to 0 ppm). | |
Intrinsic g-C3N4 exhibited two typical diffraction peaks, which emerged at 13.1° and 27.2° for 100 and 002 planes, respectively (Fig. 2a). Subsequent introduction of imidazolium side chains did not significantly alter the XRD spectral profile, suggesting the absence of significant crystal structure and ordering was influenced in the process of chemical derivatization. In addition, synthesized carbon materials exhibited similar aggregated particle like morphology with intrinsic g-C3N4 as characterized by SEM (Fig. S1, ESI†). Peaks at 784 and 1266 cm−1 from Raman spectra suggested the existence of a s-triazine ring structure in intrinsic g-C3N4 and g-C3N4-(CH2)n-ImI+ with n = 2, 8, 12 and 16 (Fig. 2b). Noticeably, relatively weak peaks and high background signals were observed in g-C3N4-(CH2)4-ImI+, which was suspected to be a high fluorescence signal from this material upon irradiation at 700 nm in the measurement of Raman spectra. Taken together, all the results suggested the successful introduction of cation side chains to intrinsic g-C3N4.
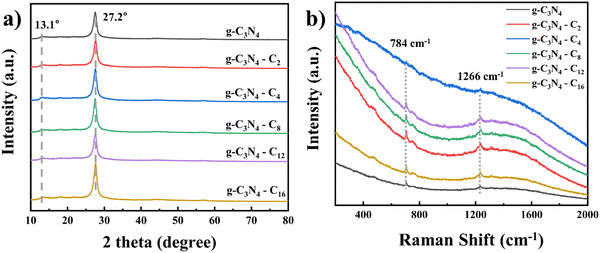 |
| Fig. 2 (a) XRD spectra of g-C3N4 and g-C3N4-(CH2)n-ImI+ (n = 2, 4, 8, 12 and 16). (b) Raman spectra of g-C3N4 and g-C3N4-(CH2)n-ImI+ (n = 2, 4, 8, 12 and 16). | |
3.2 Interactions of synthesized materials with bacteria
Before testing the light-assisted antibacterial effects of the synthesized material, the binding of the material towards bacteria was investigated using ζ potential measurement, which could reveal the interaction between carbon materials and bacteria.46 104 CFU mL−1 of Acinetobacter baumannii (Gram-negative) and Staphylococcus aureus (Gram-positive) was incubated with synthesized materials with a final concentration of 1 mg mL−1 for 4 hours. The result turned out that synthesized materials could bind to both bacteria due to the increase of the ζ potential. The ζ potential of both bacteria depends on the imidazolium side chain length (Table 1 and Fig. S2, ESI†). The ζ potential of bare A. baumannii and S. aureus was measured to be −14.87 ± 1.27 and −12.27 ± 0.71 mV, respectively. The addition of intrinsic g-C3N4 caused a slight increase of ζ potential to −11.10 ± 1.15 and −10.24 ± 1.10 mV, respectively. The introduction of imidazolium side chains (n = 2 to 8) to intrinsic g-C3N4 first increased the ζ potential, and the maxima values for A. baumannii and S. aureus were −2.79 ± 0.86 and −1.46 ± 0.72 mV with addition of C8-imidazolium side chains, respectively. Longer side chains (n = 12 and 16) resulted in a reduction of ζ potential. The corresponding values in the presence of C16-imidazolium side chains were −9.92 ± 0.52 and −8.41 ± 0.26 mV, respectively. As documented by Xu, the alkyl chain length displayed a critical role in bacterial membrane interaction of imidazolium type ionic liquids.47 In detail, short and middle chain lengths (carbon number = 3 and 8) displayed poor affinity and minimal disruption towards bacterial membrane. Appropriate chain lengths (n = 6, 10 and 12) exhibited the effects of membrane thinning and disorder, respectively. This was supposed to originate from the molecular size, hydrophobic/hydrophilic balance and bacterial membrane composition. In our case, we suppose that balancing hydrophilic and hydrophobic segment sizes is one of the determining factors in improving bacterial binding considering the structural similarity.
Table 1
ζ potential of multi drug resistant A. baumannii and S. aureus before and after treatment of g-C3N4 and g-C3N4-(CH2)n-ImI+ (n = 2, 4, 8, 12 and 16). [carbon materials] = 1.0 mg mL−1, bacterial density was 1.0 × 104 CFU mL−1, incubation time was 4 hours, and incubation temperature was 25 °C
Material |
ζ potential of the carbon material (mV) |
ζ potential of bacteria (mV) |
A. baumannii
|
S. aureus
|
Control |
— |
−14.87 ± 1.27 |
−12.27 ± 0.71 |
g-C3N4
|
+0.22 ± 0.56 |
−11.10 ± 1.15 |
−10.24 ± 1.10 |
g-C3N4-(CH2)2-ImI+
|
+3.91 ± 0.81 |
−8.36 ± 0.41 |
−7.86 ± 0.75 |
g-C3N4-(CH2)4-ImI+
|
+3.87 ± 0.77 |
−4.05 ± 0.51 |
−3.45 ± 1.00 |
g-C3N4-(CH2)8-ImI+
|
+4.34 ± 0.07 |
−2.79 ± 0.86 |
−1.46 ± 0.72 |
g-C3N4-(CH2)12-ImI+
|
+3.26 ± 0.41 |
−5.61 ± 0.40 |
−4.77 ± 1.18 |
g-C3N4-(CH2)16-ImI+
|
+3.09 ± 0.98 |
−9.92 ± 0.52 |
−8.41 ± 0.26 |
3.3 Photocatalytic antibacterial performance of synthesized materials
The side chain length dependent photo-assisted antibacterial performance of synthesized materials was investigated. The intrinsic g-C3N4 showed A. baumannii and S. aureus killing efficiency of 72.54 ± 3.49% and 75.92 ± 4.52% after light irradiation at 100 mW cm−2 for 120 min (the light source was a 300 W xenon lamp and light whose wavelength was less than 400 nm was filtered out), respectively. Bare light irradiation resulted in the corresponding efficiency of 59.32 ± 0.98% and 57.91 ± 5.16% under identical conditions, respectively. This indicated the limited light assisted antibacterial efficiency of intrinsic g-C3N4, which might be due to the lowest electrostatic interactions between the bacteria as suggested by the results in Table 1. Photocytotoxicity contributed to the dominating killing efficiency because the dark antibacterial performance of intrinsic g-C3N4 towards A. baumannii and S. aureus was 7.15 ± 3.98% and 14.02 ± 3.50%, respectively (Fig. 3a and b). The g-C3N4-(CH2)n-ImI+ displayed poor dark cytotoxicity (Table S1, ESI†) and noticeably enhanced photocytotoxicity towards both bacteria. The maxima antibacterial efficacy was observed using g-C3N4-(CH2)4-ImI+, which was 99.61 ± 0.12% for MDR A. baumannii and 99.06 ± 0.27% for S. aureus, respectively. The efficacy for other short chain (n = 2 and 8) attached g-C3N4-(CH2)n-ImI+ was also above 90%. Upon further extending the side chain, the photo-assisted antibacterial efficiency dropped to ∼89% (n = 12) and ∼86% (n = 16) for A. baumannii and S. aureus, respectively (Table S2, ESI† and Fig. 3). Fluorescence live/dead imaging using g-C3N4-(CH2)4-ImI+ towards S. aureus was conducted and the result demonstrated practical complete killing of the bacteria after light irradiation (irradiation time of 120 min, Fig. S3, ESI†). SEM photos of both bacteria and photos of colonies on culture plates of A. baumannii incubated with g-C3N4-(CH2)4-ImI+ without and with light irradiation are shown in Fig. S4 and S5 (ESI†). These results showed obvious collapse of the cell, indicating membrane damage after light irradiation. Previous ζ potential data might reveal strongest interaction between g-C3N4-(CH2)8-ImI+ and bacteria. However, the actual antibacterial performance (C4vs. C8) suggested that there might be other factors that influence the efficiency. In addition to the potential antibacterial performance, the cytotoxicity of synthesized materials was evaluated using L929 cells (mouse fibroblast). g-C3N4-(CH2)2-ImI+ displayed most pronounced 24 hours dark cytotoxicity of ∼75%, and the remaining materials showed negligible cytotoxicity (>90%, Fig. S6 and Table S3, ESI†).
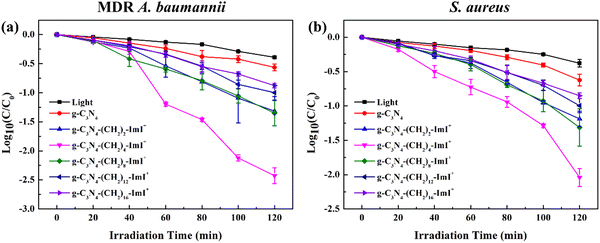 |
| Fig. 3 Time dependent photocatalytic anti-MDR A. baumannii (a) and S. aureus (b) of g-C3N4 and g-C3N4-(CH2)n-ImI+ (n = 2, 4, 8, 12 and 16). [carbon materials] = 1.0 mg mL−1, bacterial density was 1.0 × 107 mL−1, light intensity was 100 mW cm−2. | |
3.4 ROS production efficiency
Generation of reactive oxygen species (ROS) plays a critical role in photocatalytic antibacterial application. In addition to the bacteria interaction, we further investigated the impact of ROS generation between side chain lengths. 9,10-Anthracenediyl-bis(methylene)dimalonic acid (ABDA) was employed as a sensor for singlet oxygen 1O2, which might generate in the photocatalytic process. As shown in Fig. 4a, the application of light irradiation to ABDA solution for 120 min resulted in a slight reduction of absorbance at 378 nm, indicating neglectable 1O2 generation. In comparison, light irradiation to intrinsic g-C3N4 caused slightly pronounced reduced absorbance at 378 nm with increasing irradiation time. On introducing short imidazolium side chains (n = 2, 4 and 8), the absorbance reduction at 378 nm became clearer. Introducing longer cation chains (n = 12 and 16) conversely slowed the absorbance reduction at 378 nm. The summarized relationship between absorbance reduction at 378 nm and irradiation caused by different materials is shown in Fig. 4a. And the detailed absorption profile of individual materials in the presence of ABDA is shown in Fig. S7 (ESI†). Among all the materials, g-C3N4-(CH2)4-ImI+ displayed most significant irradiation time dependent irradiation. The relative 1O2 yield for intrinsic g-C3N4, g-C3N4-(CH2)12-ImI+ and g-C3N4-(CH2)16-ImI+ was calculated below 1‰. The corresponding values for g-C3N4-(CH2)2-ImI+ and g-C3N4-(CH2)8-ImI+ were 1.2‰ and 1.6‰, respectively. g-C3N4-(CH2)4-ImI+ displayed highest 1O2 yield (13.5‰). The bacterial membrane interaction indicated by ζ potential together with the 1O2 yield partially explained the discrepancy of photo-assisted bacteria inactivation efficiency of synthesized carbon materials.
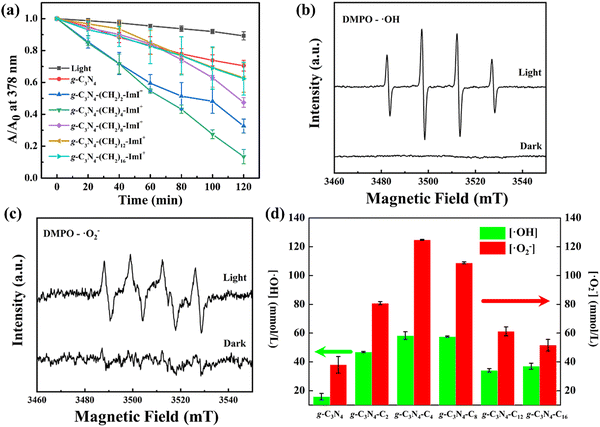 |
| Fig. 4 (a) Irradiation time dependent absorbance reduction of ABDA at 378 nm in the presence of g-C3N4 and g-C3N4-(CH2)n-ImI+ (n = 2, 4, 8, 12 and 16). (b) EPR spectra of ˙OH and (c) ˙O2− generation in the presence of g-C3N4-(CH2)4-ImI+ in the dark and after white light irradiation. (d) Amount of ˙OH and ˙O2− generated in the presence of g-C3N4 and g-C3N4-(CH2)n-ImI+ after white light irradiation. [carbon materials] = 1.0 mg mL−1, the light intensity was 100 mW cm−2, and the light irradiation time was 2 hours. | |
In addition to 1O2, the main ROS produced by g-C3N4 and the derivatives was reported to be the hydroxyl radical (˙OH), superoxide radical (˙O2−) and hydrogen peroxide (H2O2) as mediated by the photo-catalytic process. 5,5-Dimethyl-1-pyrrolidine N-oxide (DMPO) was used as a sensor to detect the generated ROS. In the obtained electronic paramagnetic resonance (EPR) spectra, g-C3N4-(CH2)4-ImI+ showed no signals for ˙OH and ˙O2− in the dark. Upon light irradiation, signals for ˙OH and ˙O2− were observed, indicating the generation of both ROS (Fig. 4b and c). As characterized by classical ˙OH and ˙O2− sensors (benzoic acid and nitroblue tetrazolium, respectively), intrinsic g-C3N4 displayed both ROS production capability (15.85 ± 2.26 mmol L−1 for ˙OH and 38.00 ± 5.72 mmol L−1 for ˙O2− after light irradiation for 2 hours, Fig. 4d and Table S3, ESI†). The photocatalytic generation amount of both ROS was observed to enhance after sidechain introduction. The most effective ROS sensitizer was g-C3N4-(CH2)4-ImI+ among all the derivatized materials, with an amount for ˙OH and ˙O2− of 58.29 ± 2.69 and 124.80 ± 0.29 mmol L−1, respectively. g-C3N4-(CH2)8-ImI+ showed resembling capabilities (57.58 ± 0.37 mmo L−1 for ˙OH and 108.80 ± 0.66 mmol L−1 for ˙O2−, respectively). With further longer side chain introduction, the amount for both ROS reduced (Table S4, ESI†). With a balance between bacterial membrane binding and ROS generation capabilities, the current result suggests that ROS generation plays a more critical role in disinfection performance.
3.5 Photoelectrochemical properties
Intrinsic and imidazolium side chain bearing g-C3N4 showed classical absorbance in the region of 350 to 450 nm (Fig. 5a). In order to explain the different ROS generation efficiency, we first determined the band gap of each carbon nitride as the intercept on the X-axis using the formula of
where, α is the light absorbance, h is the Planck constant, and ν is the absorbed light frequency. Because g-C3N4 is a kind of direct band gap semiconductor, n is 1/2 in this formula. The corresponding band gap (Eg) was determined to be in the range of 2.41 eV (g-C3N4-(CH2)4-ImI+) to 2.47 eV (g-C3N4, Fig. 5b and c). The Flat Band (FB) of synthesized g-C3N4 was determined as the intercept on the X-axis using the formula of
where f and Z′′ are the Mott–Schottky testing frequency and impedance, respectively. The corresponding FB position (EFB) of intrinsic g-C3N4 and g-C3N4-(CH2)n-ImI+ (n = 2, 4, 8, 12 and 16) is −0.72, −0.95, −0.74, −0.91, −0.84 and −0.93 eV, respectively (Fig. S8, ESI†). As an n-type semiconductor, the EFB was typically 0.1–0.3 eV positive compared to the conduction band position (ECB).48,49 The ECB was determined by minus 0.3 eV to corresponding FB, yielding individual values of −1.02, −1.25, −1.04, −1.21, −1.14, and −1.27 eV, respectively. Finally, the valence band position (EVB) was calculated as the additive sum of energy band gap (Eg) and ECB, yielding 1.45, 1.25, 1.37, 1.29, 1.34 and 1.23 eV, respectively. The current data suggested that chemical derivation would not significantly influence the ROS generation efficiency. Steady photoluminescence spectra could reveal the recombination rate of photogenerated charge carriers and electron hole pairs on the surface of the photocatalyst. As shown in Fig. 5d, cation side chain bearing carbon materials displayed significant reduced PL intensity after irradiated by 365 nm light, which suggested a lower electron–hole recombination rate compared to intrinsic g-C3N4, potentially allowing enhanced photocatalytic activity.
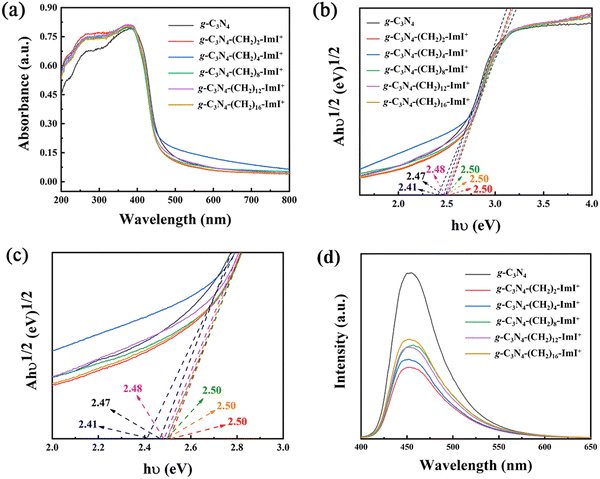 |
| Fig. 5 (a) UV-vis spectra of g-C3N4 and g-C3N4-(CH2)n-ImI+ (n = 2, 4, 8, 12 and 16). (b) and (c) Band gap calculation of carbon materials. (d) Fluorescent spectra of carbon materials. | |
The photocurrent of semiconducting materials would reveal electron–hole separation efficiency. As shown in Fig. 6a, intrinsic g-C3N4 exhibited a limited photocurrent. However, the photocurrent increased significantly after side chain introduction, and was found to be associated with the introduced side chain length. g-C3N4-(CH2)4-ImI+ showed the most pronounced photocurrent among the decorated carbon materials. In addition, according to the Nyquist plot, where x axis stands for the real part (Z′) and y axis stands for the imaginary part (Z′′), g-C3N4-(CH2)4-ImI+ displayed a relatively small semi-circle, suggesting small charge transfer resistance (Fig. 6b).50 This would further facilitate electron transfer across the electrode and electrolyte interface. The better electrical conductivity allows g-C3N4-(CH2)4-ImI+ to be a promising photocatalytic antibacterial material among the synthesized materials.
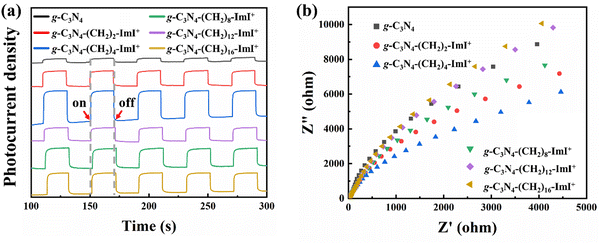 |
| Fig. 6 (a) Photocurrent spectra of g-C3N4 and g-C3N4-(CH2)n-ImI+ (n = 2, 4, 8, 12 and 16). (b) EIS spectra of carbon materials. | |
3.6 Water supply disinfection
Water supply recognized to be one of the bacteria sources and closely related to human health.51 On the basis of optimization of the antibacterial carbon material structure and the possible mechanism involving the structure–activity relationship, we further evaluated the time dependent light assisted disinfection efficiency of g-C3N4-(CH2)4-ImI+ (Table S5, ESI†). We selected urban water, Yangtze River water and the Slender West Lake water as samples. As shown in Fig. S9 (ESI†), with increasing irradiation time, the optimized material exhibited enhanced efficiencies in the three water samples. The corresponding bacterial killing rate amounted to 98.93 ± 7.87% (Urban water), 99.26 ± 2.18% (the Yangtze River) and 97.78 ± 1.77% (the Slender West Lake water) after light irradiation for 120 min ([g-C3N4-(CH2)4-ImI+] = 1.0 mg mL−1). Thus, g-C3N4-(CH2)4-ImI+ exhibited good water supply disinfection capability.
4. Conclusions
Imidazolium cations and alkane chains with varied lengths (carbon numbers of 2, 4, 8, 12 and 16) were appended to intrinsic g-C3N4. The carbon materials were fully characterized and the introduction of side chains did not alter the core s-triazine structure of intrinsic g-C3N4. ζ potential data revealed that the cation segment bearing g-C3N4 exhibited enhanced bacteria membrane interactions for both Gram-positive (MDR A. baumannii) and Gram-negative (S. aureus) bacteria compared with intrinsic g-C3N4 in an alkane chain length dependent manner. Among all the synthesized materials, g-C3N4-(CH2)8-ImI+ displayed strongest interactions with both bacteria. The following photocatalytic antibacterial results indicated that short and middle alkane chain (n = 2, 4 and 8) attached g-C3N4 were more potent antibacterial materials compared to intrinsic and long alkane chain attached (n = 12 and 16) g-C3N4. The most efficient photocatalytic antibacterial material in the current array was g-C3N4-(CH2)4-ImI+, which exhibited >99% antibacterial efficiency under identical conditions. Further experiments suggested that g-C3N4-(CH2)4-ImI+ was characterized with most pronounced ROS (1O2, ˙OH and ˙O2−). Corresponding mechanism studies proved that g-C3N4-(CH2)4-ImI+ exhibited higher photocurrent density and smaller charge transfer resistance, which would favor relatively more efficient ROS generation capability. Taken together, the current manuscript suggested introduction of cations appended to intrinsic g-C3N4 is one of the strategies to enhance the photocatalytic antibacterial performance, in the aspect of better bacterial interactions and ROS generation capability. Furthermore, this study suggested that the alkane chain length is critical in terms of antibacterial performance. The material with best antibacterial performance displayed neglectable cytotoxicity. In addition, g-C3N4-(CH2)4-ImI+ showed good light assisted water supply disinfection capability. We hope that the current result could provide potential insights for further g-C3N4 based antibacterial material design.
Data availability
All relevant data are within the paper.
Conflicts of interest
There are no conflicts of interest to declare.
Acknowledgements
This work was supported by the National Natural Science Foundation of China (Grants No. 21375116), the Natural Science Foundation of Jiangsu Province (Grants BK20190905 and BK20190903), the Natural Science Foundation of the Jiangsu Higher Education Institutions of China (Grant 19KJB430043), the Major Research Foundation of Jiangsu Science and Technology Department (Grant BE2017628), the “Lv Yang Jin Feng” project, the Yangzhou University Clinical Conversion Foundation “AHYZUZHXM 202108”, Project of Yangzhou Social Development “YZ2020071” and Key Projects of Jiangsu Provincial Health Commission “ZDB2020019” and Young scientists lifting project of Jiangsu Province, China (TJ-2022-072)
References
- K. S. Ikuta, L. R. Swetschinski and M. Naghavi, Lancet, 2022, 400, 2221–2248 CrossRef.
- D. R. Osmon, E. F. Berbari, A. R. Berendt, D. Lew, W. Zimmerli, J. M. Steckelberg, N. Rao, A. Hanssen and W. R. Wilson, Clin. Infect. Dis., 2013, 56, 1–10 CrossRef.
- R. Laxminarayan, A. Duse, C. Wattal, A. K. M. Zaidi, H. F. L. Wertheim and N. Sumpradit,
et al.
, Lancet Infect. Dis., 2013, 13, 1057–1098 CrossRef.
- E. Tacconelli, E. Carrara, A. Savoldi, S. Harbarth, M. Mendelson and D. L. Monnet,
et al.
, Lancet Infect. Dis., 2018, 18, 318–327 CrossRef PubMed.
- T. U. Berendonk, C. M. Manaia, C. Merlin, D. Fatta-Kassinos, E. Cytryn and F. Walsh,
et al.
, Nat. Rev. Microbiol., 2015, 13, 310–317 CrossRef CAS.
- E. D. Brown and G. D. Wright, Nature, 2016, 529, 336–343 CrossRef CAS PubMed.
- J. M. Munita and C. A. Arias, Microbiol. Spectrum, 2016, 4(2) DOI:10.1128/microbiolspec.vmbf-0016-2015.
- L. L. Ling, T. Schneider, A. J. Peoples, A. L. Spoering, I. Engels and B. P. Conlon,
et al.
, Nature, 2015, 517, 455–459 CrossRef CAS PubMed.
- M. Kang, C. Zhou, S. Wu, B. Yu, Z. Zhang, N. Song, M. M. S. Lee and W. Xu, J. Am. Chem. Soc., 2019, 141, 16781–16789 CrossRef CAS PubMed.
- X. Li, D. Lee, J. D. Huang and J. Yoon, Angew. Chem., Int. Ed., 2018, 57, 9885–9890 CrossRef CAS.
- F. Vatansever, W. C. M. A. de Melo, P. Avci, D. Vecchio, M. Sadasivam, A. Gupta and R. Chandran,
et al.
, FEMS Microbiol. Rev., 2013, 37, 955–989 CrossRef CAS PubMed.
- B. Ran, Z. Wang, W. Cai, L. Ran, W. Xia, W. Liu and X. Peng, J. Am. Chem. Soc., 2021, 143, 17891–17909 CrossRef CAS PubMed.
- K. Liu, Y. Liu, Y. Yao, H. Yuan, S. Wang, Z. Wang and X. Zhang, Angew. Chem., Int. Ed., 2013, 52, 8285–8289 CrossRef CAS PubMed.
- X. Li, H. Bai, Y. Yang, J. Yoon, S. Wang, X. Zhang and S. Adv, Mater., 2019, 31, 1805092 Search PubMed.
- S. Liu, H. Yuan, H. Bai, P. Zhang, F. Lv, L. Liu, Z. Dai, J. Bao and S. Wang, J. Am. Chem. Soc., 2018, 140, 2284–2291 CrossRef CAS PubMed.
- H. Chong, C. Nie, C. Zhu, Q. Yang, L. Liu, F. Lv and S. Wang, Langmuir, 2012, 28, 2091–2098 CrossRef CAS PubMed.
- C. Zhou, Z. Ding, W. Guan and B. Z. Tang, Aggregate, 2023, 5, e366 CrossRef.
- W. Liu, H. Gu, B. Ran, W. Liu, W. Sun, D. Wang, J. Du and J. Fan, Sci. China. Mater., 2022, 65, 845–854 CrossRef CAS PubMed.
- X. Li, F. Fang, B. Sun, C. Yin, J. Tan, Y. Wan, J. Zhang and P. Sun,
et al.
, Nanoscale Horiz., 2021, 6, 177–185 RSC.
- B. Wang, G. Feng, M. Seifrid, M. Wang, B. Liu and G. C. Bazan, Angew. Chem., Int. Ed., 2017, 56, 16063–16066 CrossRef CAS PubMed.
- C. Zhang, K. Wang, X. Guo and Y. Tang, J. Mater. Chem. C, 2022, 10, 2600–2607 RSC.
- J. Wang, B. Hao, K. Xue, H. Fu, M. Xiao, Y. Zhang, L. Shi and C. Zhu, Adv. Mater., 2022, 34, 2205653 CrossRef CAS.
- H. Chen, S. Li, M. Wu, K. Kenry, Z. Huang, C. S. Lee and B. Liu, Angew. Chem., Int. Ed., 2020, 59, 632–636 CrossRef CAS PubMed.
- M. R. Hamblin, Curr. Opin. Microbiol., 2016, 33, 67–73 CrossRef CAS PubMed.
- H. Shen, L. Zhang, M. Liu and Z. Zhang, Theranostics, 2012, 2, 283–294 CrossRef CAS PubMed.
- J. Huang, W. Ho and X. Wang, Chem. Commun., 2014, 50, 4338–4340 RSC.
- X. Kong, X. Liu, Y. Zheng, P. K. Chu, Y. Zhang and S. Wu, Mater. Sci. Eng., R, 2021, 145, 100610 CrossRef.
- G. Liao, F. He, Q. Li, L. Zhong, R. Zhao, H. Che, H. Gao and B. Fang, Prog. Mater. Sci., 2020, 112, 100666 CrossRef CAS.
- W. Wang, J. C. Yu, D. Xia, P. K. Wong and Y. Li, Environ. Sci. Technol., 2013, 47, 8724–8732 CrossRef CAS PubMed.
- T. Arumugham, R. G. Amimodu, N. J. Kaleekkal and D. Rana, J. Environ. Sci., 2019, 82, 57–69 CrossRef CAS.
- K. K. Das, S. Patnaik, S. Mansingh, A. Behera, A. Mohanty, C. Acharya and K. M. Parida, J. Colloid. Interface Sci., 2020, 561, 551–567 CrossRef CAS.
- M. Ghanbari and M. Salavati-Niasari, Ecotoxicol. Environ. Saf., 2021, 208, 111712 CrossRef CAS PubMed.
- H. P. Lee and A. K. Gaharwar, Adv. Sci., 2020, 7, 200863 Search PubMed.
- J. Li, Y. Liu, Y. Xu, L. Li, Y. Sun and W. Huang, Coord. Chem. Rev., 2020, 415, 213318 CrossRef CAS.
- C. Tang, C. Liu, Y. Han, Q. Guo, W. Ouyang, H. Feng, M. Wang and F. Xu, Adv. Healthcare Mater., 2019, 8, 1801534 CrossRef PubMed.
- Q. Wang, L. Zhang, Y. Guo, M. Shen, M. Wang, B. Li and J. Shi, Chem. Eng. J., 2020, 396, 125347 CrossRef CAS.
- L. Xu, H. Li, P. Yan, J. Xia, J. Qiu, Q. Xu, S. Zhang, H. Li and S. Yuan, J. Colloid. Interface Sci., 2016, 483, 241–248 CrossRef CAS.
- M. Yuan, Z. Teng, S. Wang, Y. Xu, P. Wu, Y. Zhu, C. Wang and G. Wang, Chem. Eng. J., 2020, 391, 123506 CrossRef CAS.
- Z. Teng, N. Yang, H. Lv, S. Wang, M. Hu, C. Wang, D. Wang and G. Wang, Chem, 2019, 5, 664–680 CAS.
- H. Chong, X. Liu, S. Fang, X. Yang, Y. Fei, T. Wang, L. Liu and Y. Kan,
et al.
, Adv. Sci., 2024, 11, 2306936 CrossRef CAS PubMed.
- D. Demberelnyamba, K. S. Kim, S. Choi, S. Y. Park, H. Lee, C. J. Kim and I. D. Yoo, Bioorg. Med. Chem., 2004, 12, 853–857 CrossRef CAS PubMed.
- J. N. Pendleton and B. F. Gilmore, Int. J. Antimicrob. Agents, 2015, 46, 131–139 CrossRef CAS PubMed.
- E. Novello, G. Scalzo, G. D’Agata, M. G. Ruacci, L. Ambrosio, A. Soriente, B. Tomasello, C. Restuccia, L. Parafati, G. M. L. Consoli, L. Ferreri, A. Rescifina, C. Zagni and D. C. Zampino, Pharmaceutics, 2024, 16, 642 CrossRef CAS.
- Z. Teng, H. Lv, C. Wang, H. Xue, H. Pang and G. Wang, Carbon, 2017, 113, 63–75 CrossRef CAS.
- J. Dai, X. Wu, S. Ding, X. Lou, F. Xia, S. Wang and Y. Hong, J. Med. Chem., 2020, 63, 1996–2012 CrossRef CAS PubMed.
- R. Qi, N. Zhang, P. Zhang, H. Zhao, J. Liu, J. Cui, J. Xiang, Y. Han, S. Wang and Y. Wang, ACS Appl. Mater. Interfaces, 2020, 12, 17220–17229 CrossRef CAS PubMed.
- L. Zheng, J. Li, M. Yu, W. Jia, S. Duan, D. Cao, X. Ding, B. Yu, X. Zhang and F. J. Xu, J. Am. Chem. Soc., 2020, 142, 20257–20269 CrossRef CAS PubMed.
- S. Che, X. Zhou, L. Zhang, D. Su, T. Wang and C. Wang, Chem. – Asian J., 2022, 17, e202200095 CrossRef CAS PubMed.
- X. Zhou, T. Wang, L. Zhang, S. Che, H. Liu, S. Liu and C. Wang,
et al.
, Appl. Catal., B, 2022, 316, 121614 CrossRef CAS.
- Z. Teng, W. Cai, W. Sim, Q. Zhang, C. Wang, C. Su and T. Ohno, Appl. Catal., B, 2021, 282, 119589 CrossRef CAS.
- H. Liu, T. Wang, S. Liu, X. Zhou, L. Zhang, Y. Sun, Y. Hu, M. Shakouri, Y. Zhang, Z. Teng, X. Zhang, G. Wang and C. Wang, Chem. Eng. J., 2024, 498, 155359 CrossRef CAS.
|
This journal is © The Royal Society of Chemistry 2025 |
Click here to see how this site uses Cookies. View our privacy policy here.