H2O2-activated mitochondria-targeting photosensitizer for fluorescence imaging-guided combination photodynamic and radiotherapy†
Received
27th July 2024
, Accepted 7th November 2024
First published on 8th November 2024
Abstract
Radiotherapy is a primary modality in cancer treatment but is accompanied by severe side effects to healthy tissues and radiation resistance to some extent. To overcome these limitations, we developed a H2O2-responsive photosensitizer, CyBT, which could be activated by the upregulated H2O2 induced by radiotherapy, enabling near-infrared fluorescence imaging-guided combination photodynamic and radiotherapy. The synthesis of CyBT began with the covalent linkage of hemicyanine and a free radical TEMPO through the click reaction, which demonstrated superior photodynamic properties. Shielding of fluorescence and photodynamic activity was achieved by incorporating phenylboronic acid pinacol ester. In X-ray irradiated tumor cells, the upregulation of H2O2 activated CyBT, thereby restoring its fluorescence and photodynamic activity. Additionally, the positive charge of CyBT facilitated its targeting to the mitochondria within tumor cells for more efficiently triggering cell apoptosis. CyBT was co-assembled with a polymer PEG-b-PDPA to form acid-responsive nanoparticles (NPs-CyBT). This formulation enhanced tumor targeting, improved water solubility of CyBT, and extended in vivo circulation time. Utilizing fluorescence imaging to guide photodynamic and radiotherapy, NPs-CyBT can accurately target solid tumors in mice, and lead to tumor elimination, suggesting that it is a potential strategy for the effective treatment of malignant tumors.
1. Introduction
Radiotherapy (RT) is a highly effective and widely used cancer treatment in clinical practice,1 and most cancer patients will use RT as the best choice for controlling local tumor growth and metastasis in the therapeutic process.2 RT employs high-energy ionizing radiation, such as X-ray and γ-ray, to inflict direct or indirect damage on cancer cells by targeting their DNA and proteins through free radicals generated from water radiolysis.3,4 This activates cytotoxic signal pathways that promote the death of cancer cells, ultimately leading to the extinction of tumor.5 However, studies have shown that RT can exacerbate anoxia in tumor cells, resulting in the up-regulation of NADPH oxidase 4 (NOX4) protein expression,6,7 and reduces RT sensitivity through activation of the PI3K/AKT pathway,8 generating RT tolerance as a cytoprotective mechanism. This tolerance of cancer cells to ionizing radiation induced by RT can be the cause of failure of cancer treatment, tumor recurrence and metastasis.9 Furthermore, high doses of radiation intensity can also produce side effects, such as damage and necrosis of normal tissues, compromising treatment efficacy.10 Therefore, RT alone is often insufficient for clinical tumor treatment needs.
To enhance tumor therapy efficacy and minimize side effects, combination treatment strategies have been developed, including chemotherapy,11,12 immunotherapy13,14 and photodynamic therapy (PDT).15–21 PDT stands out for its non-invasiveness, high selectivity, and low drug resistance. It involves the application of a photosensitizer to the tumor area, which, upon exposure to light of specific wavelengths, undergoes a photochemical reaction to produce reactive oxygen species (ROS), leading to cancer cell death.22–24 Mitochondria play a crucial role in cellular processes such as energy supply, redox homeostasis, as well as cell growth, differentiation, and apoptosis.25,26 They are key determinants of cancer cell radiosensitivity27,28 and can modulate this sensitivity through mitochondrial autophagy.29,30 Mitochondria are also primary targets for ROS generated by PDT, leading to decreased membrane potential, reduced ATP production, and induction of apoptosis and mitochondrial autophagy.31,32 The synergy between PDT and ionizing radiation from RT can increase cancer cell apoptosis by inhibiting repair mechanisms,33 presenting an effective approach to tumor eradication with minimal adverse effects.
However, conventional photosensitizers have limitations, such as lack of targeting and indiscriminate activation in both normal and cancerous tissues due to continuous photosensitizing activity,34 and limited tissue penetration depth at short excitation wavelengths, restricting application to deep target tissues.35,36 To overcome these challenges, we developed a NIR mitochondria-targeting H2O2-activatable photosensitizer, CyBT, co-assembled into acid-responsive nanomicelles by methoxyl poly(ethylene glycol)-block-poly(2-diisopropylaminoethyl methacrylate-random-2-hydroxyethyl methacrylate) diblock copolymers (mPEG113-b-P(DPA60-r-HEMA8), namely PEG-b-PDPA) with acid-dissociative properties, for combined RT and PDT anti-tumor treatment. This system allows deeper tissue penetration and reduces side effects by “switching off” photosensitizer activity in normal tissues and “switching on” in tumors. To this end, the exposed hydroxyl group on hemicyanine was masked with boronic acid pinacol ester as removable cage units so that they could be easily converted into activatable photosensitizers with turn-on fluorescence based on the intramolecular charge transfer (ICT) mechanism, thus providing critical information about the location of the tumor and the photosensitizer. The 2,2,6,6-tetramethylpiperidine-N-oxyl (TEMPO) group was then connected via click chemistry to enhance singlet oxygen (1O2) yield and PDT efficiency. The resulting H2O2-activatable photosensitizer CyBT was encapsulated by PEG-b-PDPA, forming NPs-CyBT. These nanoparticles were ingested by tumor cells and dissociated to release photosensitizers under lysosomal acidic conditions. Finally, CyBT targeted mitochondria and was activated by RT-induced H2O2. The RT-activated photosensitizer's fluorescence guided precise PDT, significantly improves the therapeutic efficacy of combined RT and PDT (Scheme 1).
 |
| Scheme 1 (A) The molecular structure and response process of CyBT probe and PEG-b-PDPA. (B) Schematic diagram of molecular coassembly, dissociation, and activation processes. (C) Schematic illustration of NPs-CyBT radiotherapy in vivo in conjunction with PDT. | |
2. Experimental
2.1. Synthesis of CyBT
CyB (68.0 mg, 0.1 mmol), 4-alkynylpropoxy-TEMPO (42.0 mg, 0.2 mmol) were dissolved in a mixed solution of CHCl3/CH3OH/H2O (6
:
0.5
:
0.5, v/v/v) under the protection of N2 and stirred for 15 min, then triethylamine and CuI (20 mg, 0.1 mmol) were added, the reaction was carried out at room temperature. Then, the solution was diluted with dichloromethane (DCM) and extracted three times with water and saturated NaHCO3. The organic phases were combined, dried over anhydrous sodium sulfate, and concentrated under reduced pressure. The crude product was purified by column chromatography on silica gel (DCM
:
CH3OH = 18
:
1) to give the pure product CyBT (40.0 mg, 60%). HRMS (ESI): calcd for C54H68BN5O6˙+, [M]: m/z 893.5257; found, 893.5305.
2.2. Preparation and characterization of NPs-CyBT
PEG-b-PDPA with superior pH sensitivity was synthesized by the reversible addition–fragmentation chain transfer polymerization (RAFT).37,38 PEG-b-PDPA (40 mg mL−1) and CyBT (4 mg mL−1) were added to 1 mL PBS (10 mM, pH 7.4) under ultrasonic conditions. Then the nanomicelle NPs-CyBT was obtained by centrifugation and concentration with a 30 kDa ultrafiltration tube. The particle size and polydispersity of the nanomicelles were further measured by dynamic light scattering (DLS), and the micelles were added to water, PBS, normal saline (NS) and 10% FBS to measure the stability within 24 h. Then, micelles were added to PBS solution with pH values of 7.4 and 6.0, and the particle size changes in PBS buffer with different pH values were tested by DLS, the morphologies were further characterized by transmission electron microscopy (TEM).
2.3. Cellular co-localisation of NPs-CyBT
HeLa cells were cultured in confocal dishes to an optimal density. The NPs-CyBT stock solution was diluted with Dulbecco's modified Eagle's medium (DMEM) to a final concentration of 10 μM. Following the removal of the spent medium and three rounds of washing with fresh medium, the cells were incubated with the newly prepared medium containing NPs-CyBT for various durations. Afterward, lysosomal and mitochondrial localization probes were introduced. Once the incubation with the probes was complete, the cells were washed and prepared for imaging.
2.4. Other experimental
All other experimental details can be found in the ESI.†
3. Results and discussion
3.1. Synthesis and characterization of CyBT
The excitation wavelength of hemicyanine dyes falls within the near-infrared region, which effectively minimizes interference from background fluorescence in biological systems, penetrates deeper into tissues, causes less damage to biological tissues, and offers high sensitivity. The hemicyanine dye CyOH is characterized by an azacyclic cation acting as an electron acceptor and a terminal hydroxyl group serving as an electron donor; these are connected by a conjugated bond to form a canonical D–π–A conjugate structure. To enhance the ROS generation efficiency of the CyOH probe, which has relatively poor photodynamic capabilities, we covalently attached the free radical group TEMPO to the CyOH probe via a click reaction, thereby creating a photosensitizer with enhanced photodynamic properties. To address the issue of non-specificity associated with “always-on” photosensitizers, we introduced a boronic acid pinacol ester as a H2O2-recognizable cage-like unit, which was linked to the hemicyanine structure by self-eliminating p-aminobenzyl alcohol, resulting in the activated photosensitizer CyBT. The successful synthesis of the compounds was confirmed by corresponding 1H NMR, 13C NMR, and HRMS-ESI analyses (Fig. S1–S11, ESI†).
Analysis of the absorption and fluorescence spectra revealed that CyBT exhibited maximum absorption peaks at 610 nm and 660 nm, with negligible fluorescence emission (Fig. 1A and B). Upon exposure to H2O2, the absorption spectrum experienced a redshift, and a pronounced fluorescence peak emerged at around 710 nm. This indicates that the presence of the phenylboronic acid ester group suppresses the intramolecular charge transfer (ICT) effect, consequently quenching fluorescence. The restoration of fluorescence upon H2O2 interaction suggests that the self-eliminating group is removed, unveiling the hydroxyl group and transforming CyBT into CyOT, which is accompanied by a color transition from blue to light green. As the incubation period increased, the fluorescence intensity of CyBT at 710 nm gradually heightened, reaching a plateau at 60 min (Fig. 1C and D).
 |
| Fig. 1 (A) and (B) Absorption (A) and fluorescence (B) spectra of CyBT (10 μM) before and after the addition of H2O2 (λex, 680 nm). (C) and (D) Time-dependent fluorescence spectra of CyBT with H2O2 and intensity changes at 710 nm. (E) and (F) Concentration-dependent fluorescence spectra of CyBT with H2O2 and intensity changes at 710 nm. The linear relationship of CyBT with H2O2. (G) Proposed mechanism of CyBT toward H2O2. (H) Mass spectra of CyBT treated with or without H2O2. | |
To further assess the responsive performance, we conducted fluorescence titration experiments by measuring the fluorescence spectra of CyBT following incubation with varying concentrations of H2O2. An incubation of CyBT with H2O2 concentrations ranging from 0 to 100 μM resulted in a concentration-dependent increase in fluorescence intensity, which peaked at 80 μM (Fig. 1E and F). The fluorescence intensity of CyBT demonstrated a strong linear correlation with the H2O2 concentration in the range of 0 to 60 μM. The data were analyzed to produce a linear regression equation with a R2 value of 0.99 and a limit of detection (LOD) calculated to be 157 nM (S/N = 3).
CyBT exists in an “OFF” state due to its characteristic A–π–A backbone. However, upon interaction with H2O2, the phenyl borate group undergoes 1,6 self-elimination and subsequent hydrolysis, resulting in the formation of a D–π–A structure (Fig. 1G). This transformation enhances the ICT effect, leading to the emission of strong NIR-I fluorescence. Furthermore, the structures of both CyBT and CyOT were validated through high-resolution mass spectrometry, with observed m/z values of 893.5305 and 677.3991, respectively (Fig. 1H).
3.2. Selectivity and photodynamic properties of CyBT
Additionally, we evaluated the probe's selective response to H2O2 by monitoring fluorescence changes (Fig. 2A and B). The fluorescence of CyBT increased 10-fold after incubation with H2O2, while other analytes (oxidizing agents, amino acids, metal ions, anions, and similar interfering species) showed no significant fluorescence changes. For the anti-interference performance experiments, the result showed that CyBT could recognize H2O2 in the presence of various other substances (Fig. 2C and D). These findings indicate that the probe is highly selective and resistant to interference from other substances, confirming its ability to specifically recognize H2O2 in complex biological settings.
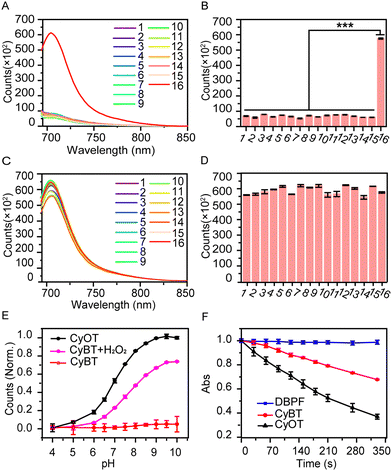 |
| Fig. 2 (A) and (B) Fluorescence spectra (A) and intensity changes (B) at 710 nm of CyBT in response to H2O2 and potential interfering substances. (1) Cu2+, (2) PBS, (3) ONOO−, (4) Cysteine, (5) NO2−, (6) ClO−, (7) Ca2+, (8) O2˙−, (9) Mg2+, (10) t-butyl hydroperoxide, (11) K+, (12) Cl−, (13) ˙OH, (14) 1O2, (15) Glutathione, (16) H2O2. (C) and (D) Fluorescence spectra (C) and intensity changes (D) at 710 nm of CyBT in response to H2O2 in the absence (16) and presence (1–15) of potential interfering substances. (E) The effect of pH on the response of CyBT to H2O2. (F) Absorbance of DPBF at 415 nm in DMSO with CyBT or CyOT upon 655 nm laser irradiation (80 mW cm−2) (mean ± SD, n = 3, *** p < =0.001 compared to H2O2 group). | |
Next, we assessed the fluorescence spectra of CyOT and CyBT in PBS solutions across a pH range of 4.0 to 10.0 (Fig. 2E). The fluorescence of CyOT at 710 nm increased progressively with rising pH values. In contrast, the fluorescence of the CyBT probe remained largely unaffected by varying pH levels, demonstrating its robust stability across a broad pH spectrum. Upon the introduction of H2O2, the fluorescence of the CyBT probe commenced to intensify, tracking the same growth pattern as CyOT. The fluorescence intensity at 710 nm remained consistent in PBS solution for a minimum of 3 h with measurements taken once per minute (Fig. S12, ESI†).
Given that the photodynamic properties of CyBT are unleashed post-activation, we evaluated the probe's photodynamic efficacy in solution using 1,3-diphenylisobenzofuran (DPBF) as 1O2 sensor. As shown in Fig. 2F, the absorbance of the DPBF solution containing CyOT at 415 nm under 655 nm laser irradiation rapidly diminished by 64% within 330 seconds, whereas the absorbance of CyBT decreased by 30%. This indicates that the activated CyOT has double the singlet oxygen generation capacity compared to the inactive CyBT probe, thereby exhibiting superior photodynamic activity. Moreover, it was evident that the phenolic hydroxyl group not only quenches the probe's fluorescence but also suppresses the generation of 1O2. Thus, CyBT can be used as an activated photosensitizer.
3.3. Preparation and characterization of NPs-CyBT
To enhance the biocompatibility and solubility of CyBT for both in vitro and in vivo applications, we co-assembled CyBT with the acid-responsive polymer PEG-b-PDPA to form nanoparticles, designated as NPs-CyBT. The polymer PEG-b-PDPA is composed of three covalently linked components: the acid-responsive DPA, HEMA, and the PEG chain. The co-assembly process involved dissolving PEG-b-PDPA and CyBT in N, N-dimethylformamide (DMF), followed by dropwise addition into PBS under sonication to form nanomicelles. We then varied the assembly conditions, including the ratios of PEG-b-PDPA to CyBT probe and solvent, and the types of solvents, to produce nanomicelle solutions with distinct sizes and dispersions. An optimized ratio of 5
:
1 (polymer to probe, w/w) was determined to prepare micelles with an average diameter of 90 nm and a polydispersity index (PDI) of 0.283, indicating a relatively uniform dispersion (Fig. 3A).
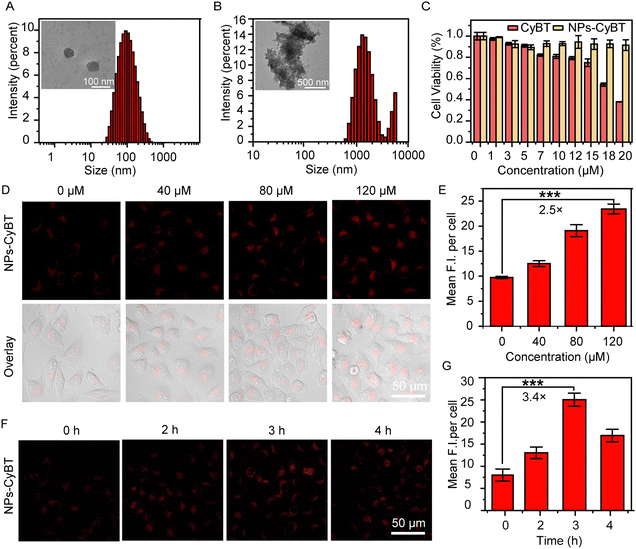 |
| Fig. 3 (A) DLS and TEM images of NPs-CyBT at pH 7.4. (B) DLS and TEM images of NPs-CyBT at pH 6.0. (C) Cytotoxicity of CyBT and NPs-CyBT. (D) Fluorescence images of different concentrations of H2O2 in HeLa cells. HeLa cells were pre-incubated with NPs-CyBT (10 μM). (E) Histogram of the mean in fluorescence intensity in (D). (F) Fluorescence images of different times after X-ray radiation in HeLa cells. (G) Histogram of the mean in fluorescence intensity in (F) (mean ± SD, n = 3, *** p < =0.001). | |
We further assessed the stability of NPs-CyBT nanomicelles in water, PBS, normal saline (NS), and 10% fetal bovine serum (FBS) (Fig. S13, ESI†). The uniformity of particle distribution revealed that the nanomicelles maintained consistent size and stable PDI within the range of 0.3–0.4 over 24 h, confirming their stability under physiological conditions. The acid-dissociation behavior of NPs-CyBT micelles at different pH levels was investigated by introducing the micelles into PBS at pH 7.4 and 6.0. The particle size increased from the initial 90 nm to approximately 3 μm (Fig. 3B). TEM imaging showed that the micelles were spherical with a size of 70–80 nm in PBS at pH 7.4, and they dissociated into irregular, fluffy aggregates in PBS at pH 6.0. Finally, using the standard curve of the probe CyBT (Fig. S14, ESI†), the encapsulation efficiency of the probe within the nanomicelle was calculated to be approximately 50%.
3.4. Fluorescence imaging of exogenous and endogenous H2O2 by NPs-CyBT
Given CyBT's robust response to H2O2 and its potent photodynamic properties, both CyBT and NPs-CyBT were deployed for cellular-level H2O2 imaging studies. HeLa cells, were selected as a model cell line for these experiments. The cytotoxicity of CyBT and NPs-CyBT was assessed using the MTT assay. After co-incubating HeLa cells with varying concentrations of CyBT for 12 h, cell viability remained above 80% at a concentration of 10 μM. Moreover, when HeLa cells were co-incubated with NPs-CyBT at concentrations ranging from 0 to 20 μM, cell viability exceeded 90%. These findings underscore the excellent biocompatibility of NPs-CyBT (Fig. 3C).
Following the cytotoxicity evaluation, the capacity of NPs-CyBT to image exogenous H2O2 in HeLa cells was further investigated. The cells were pre-incubated with NPs-CyBT (10 μM) for 2 h, and then the cells were treated with 0, 40, 80 and 120 μM of H2O2 for 1 h (Fig. 3D). Analysis with ImageJ software revealed a correlation between the fluorescence imaging signal intensity and the addition of exogenous H2O2.
HeLa cells pre-incubated with NPs-CyBT exhibited minimal red fluorescence before the introduction of H2O2; however, a significant enhancement in red fluorescence was observed upon H2O2 incubation, with signal intensity increasing proportionally to H2O2 concentration (Fig. 3E). The average fluorescence intensity increased by 2.5-fold when the H2O2 concentration was elevated to 120 μM. CyBT alone demonstrated a similar ability to image exogenous H2O2, with an average fluorescence intensity enhancement of 2.8-fold under identical conditions (Fig. S15, ESI†).
After establishing the probe's ability to image exogenous H2O2, we explored its performance in detecting endogenous cellular H2O2. Studies have shown that X-ray irradiation upregulates NOX4 enzyme expression, leading to increased ROS production, including H2O2. Prior experiments have indicated that H2O2 can activate CyBT and trigger its photodynamic capabilities. However, the limited PDT efficacy due to low H2O2 levels in tumor cells resulted in suboptimal therapeutic outcomes. We aimed to stimulate the production of substantial H2O2 in cells through X-ray irradiation to further activate PDT, synergizing with radiotherapy for enhanced treatment effects. Thus, we utilized NPs-CyBT to monitor endogenous cellular H2O2 changes induced by X-ray. HeLa cells pre-incubated with NPs-CyBT were subjected to an 8 Gy radiation dose for 4 minutes using an irradiator, followed by incubation for various durations to allow for imaging and quantitative fluorescence analysis via confocal microscopy (Fig. 3F and G). The results indicated that the red fluorescence in HeLa cells progressively intensified over time, peaking at 3 h with a 3.4-fold increase in average fluorescence intensity, after which the fluorescence began to diminish. This demonstrated that X-ray irradiation could indeed induce cellular H2O2 production, effectively activating the CyBT probe. CyBT imaging also revealed a 2.5-fold fluorescence enhancement at 3 h, followed by a decline (Fig. S16, ESI†), aligning with our initial experimental findings.
3.5. Mitochondria targeting of NPs-CyBT
Mitochondria, as pivotal subcellular organelles, play an integral role in signal transduction and apoptosis. Moreover, the negative potential of the inner mitochondrial membrane draws cationic substances to accumulate there. Compared to their normal counterparts, tumor cells exhibit a higher mitochondrial membrane potential, leading to a preferential accumulation and retention of cationic mitochondrial targeting agents within cancer cells. Furthermore, mitochondria initiate apoptosis immediately upon damage inflicted by photosensitizers.
In this study, tracking the subcellular localization of the NPs-CyBT micelles after cellular uptake was crucial. To this end, the micelles were co-incubated with cells for varying durations, followed by the addition of commercial lysosomal and mitochondrial probes for co-localization assays. The degree of co-localization in each merged image was quantified using Pearson's correlation coefficient (PCC), a metric that measures the overlap between two parameters. Initially, the NPs-CyBT micelles were found to enter the lysosomes, with a PCC value peaking at 0.89 after 4 h, indicating a strong co-localization as visualized by the synchronized undulation of red and green fluorescence signals within the cells when analyzed using ImageJ (Fig. 4A and B). Over time, the co-localization with lysosomes diminished (Fig. S17, ESI†), while the co-localization with mitochondria improved, reaching a maximum PCC value of 0.84 at 12 h (Fig. 4C and Fig. S18, S19, ESI†), further confirmed by line scans across the cells using ImageJ (Fig. 4D). Thus, the co-localization monitoring experiments revealed that NPs-CyBT micelles were initially internalized by cells via endocytosis, leading to their accumulation in lysosomes. Within the acidic environment in lysosomes (pH < 6.0), the acid-responsive dissociation of the micelles occurred. Concurrently, the positively charged CyBT probe released from the lysosomes was directed to the negatively charged mitochondria through electrostatic interactions, facilitating a gradual transfer to the mitochondria.
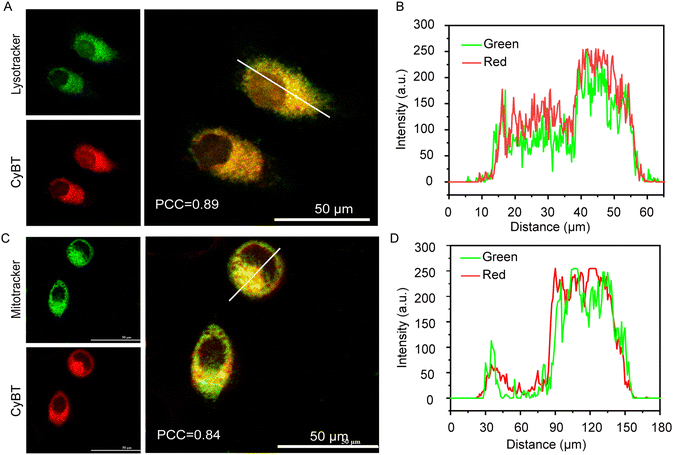 |
| Fig. 4 (A) Fluorescence imaging of HeLa cells preincubated with NPs-CyBT for 4 h and co-localized with the commercial lysotracker probe. (B) Pulling lines through ImageJ for the cell in (A). (C) Fluorescence imaging of cells preincubated with NPs-CyBT for 12 h co-localized with the mitotracker probe. (D) Pulling lines through ImageJ for the cell in (C). | |
3.6. Phototoxicity of NPs-CyBT upon laser and X-ray irradiation
Building on the promising outcomes of NPs-CyBT in imaging both exogenous and endogenous H2O2 and its mitochondrial co-localization at the cellular level, we proceeded to assess the probe's capacity to generate ROS for photodynamic therapy. Firstly, DCFH-DA was utilized to detect ROS production following X-ray and 655 nm laser irradiation. Quantitative fluorescence analysis revealed a 6.3-fold promotion in green fluorescence intensity when the incubation period was extended to 2 h (Fig. 5A and B), indicative of the photodynamic properties of NPs-CyBT. Subsequently, we evaluated the cell apoptosis performance of NPs-CyBT by using a live-dead staining kit. HeLa cells pre-cultured with NPs-CyBT nanoparticles and CyBT were irradiated with 655 nm laser in the presence/absence of X-ray, respectively. After further staining, it was found that most of the cells died after X-ray as well as laser irradiation. In contrast, only a few cells in the laser irradiated group that was preincubated with CyBT were dead (Fig. 5C), and these results further indicated that NPs-CyBT has excellent photodynamic properties with a good killing effect after activation.
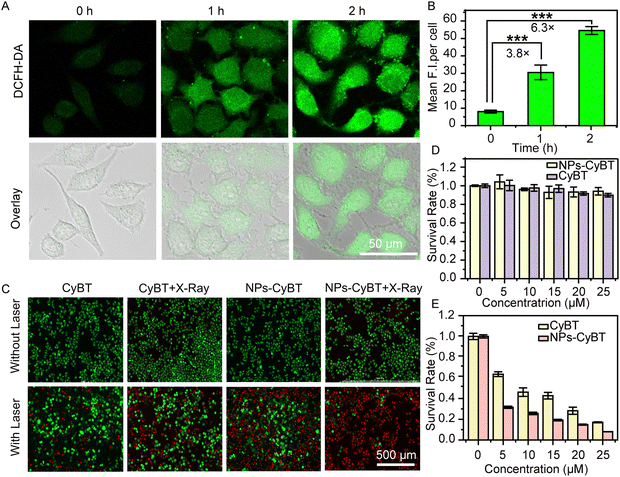 |
| Fig. 5 (A) Fluorescence imaging of cells pre-incubated with NPs-CyBT probes that were left to stand for different times after X-ray irradiation and then light irradiation. (B) Histogram of the mean in fluorescence intensity in (A). (C) Live-dead staining assays to analyze cell death induced by CyBT and NPs-CyBT with or without a laser and X-ray irradiation. (D) Radiotoxicity of CyBT and NPs-CyBT. (E) Radiotoxicity and Phototoxicity of CyBT and NPs-CyBT. | |
At the same time, we explored the effects of irradiation time on cell viability. Combined with Fig. S20 and S21 (ESI†), the cell death rate of cells preincubated with NPs-CyBT micelles was close to 100% after 3 min of laser irradiation; under the same conditions, the apoptosis rate of cells preincubated with CyBT probes was 20-30%, and the cells were completely dead only after 9 min. These experimental results indicate that the combination of NPs-CyBT with radiotherapy significantly improves the efficacy of photodynamic therapy.
To ascertain the impact of X-ray on cellular photodynamic therapy, we investigated the effect of X-ray alone on cells. The experimental results demonstrated that X-ray had a negligible impact on cell survival (Fig. 5D). Subsequently, MTT experiments also confirmed the killing effect of the probe on cell viability by X-ray followed by laser irradiation. Cells preincubated with CyBT and NPs-CyBT were exposed to an 655 nm laser at 100 mW cm−2 after X-ray irradiation, and it was found that the phototoxicity of NPs-CyBT to the cells was greater than that of CyBT (Fig. 5E), thereby confirming that the construction of NPs-CyBT micelles further potentiates the photodynamic therapy effects of CyBT.
3.7. Theranostic application of NPs-CyBT in HeLa tumor-bearing mouse model
Motivated by the remarkable performance of NPs-CyBT in living cells, we extended its application to HeLa tumor-bearing mouse models. The fluorescence images of mice were captured over a 72 h period following various treatments, including PBS, NPs-CyBT, and NPs-CyBT + X-Ray (Fig. 6A and B). The control group exhibited no significant fluorescent signal. In contrast, the NPs-CyBT group demonstrated a gradual enhancement in fluorescence intensity over time, reaching a peak at 9 h with a 5.0-fold increase, then plateaued, and began to decline at 12 h. Most notably, when mice were pretreated with X-ray, the fluorescence signal in the NPs-CyBT group was further intensified, showing a 10.0-fold increase at 48 h before gradually diminishing (Fig. 6C and Fig. S22, S23, ESI†). These results confirmed that X-ray induced the production of H2O2 and activated the NPs-CyBT nanomicelles. Thus, the entire process of monitoring intratumoral H2O2 and the subsequent induction of H2O2 post radiotherapy was visually presented.
 |
| Fig. 6 (A) Schematic diagram of the treatment and imaging mechanism. (B) Fluorescence imaging of the HeLa tumor-bearing mice following i.t. injection of NPs-CyBT (2 mg kg−1) for different times (λex = 680 nm, λem = 720 nm). (C) Relative fluorescence intensity at the tumor site in (B) (n = 3). (D) Treatment schedule for NPs-CyBT based tumor suppression experiments. (E) Tumor growth inhibition curves and (F) body weight changes of mice treated with PBS, PBS + X-Ray, NPs-CyBT, NPs-CyBT + Laser, NPs-CyBT+ X-Ray + Laser. CyBT at 2 mg kg−1 body weight. n = 5 mice per group. (G) Tumor images of different groups after 21 days therapy (n = 5). (a) PBS, (b) PBS + X-Ray, (c) NPs-CyBT, (d) NPs-CyBT + Laser, (e) NPs-CyBT+ X-Ray + Laser. | |
Subsequently, we evaluated the in vivo antitumor efficacy of NPs-CyBT. Tumor-bearing mice were divided into five groups (n = 5): PBS, PBS + X-Ray, NPs-CyBT, NPs-CyBT + Laser, and NPs-CyBT + X-Ray + Laser. The treatment regimen involved intratumoral injections on a 4-day cycle, and the mice were treated according to the outlined schedule (Fig. 6D). Tumor volume and body weight changes were monitored every two days for 21 days. Compared to other treatments, the NPs-CyBT + X-Ray + Laser therapy resulted in the smallest tumor volumes (Fig. 6E), with complete tumor regression by the end of the 11th day and no recurrence or metastasis observed for the subsequent 10 days. This demonstrated that X-ray-induced H2O2 release was able to activate the CyBT probe more extensively, enhancing the photodynamic therapy effect and more effectively inhibiting tumor progression. The body weights of mice treated with different methods remained stable throughout the observation period (Fig. 6F), indicating negligible side effects from the prepared nanomicelles. After 21 days, the mice were sacrificed, and the tumor growth in the NPs-CyBT + X-Ray + Laser group showed significant inhibition based on the collected images of tumor appearance (Fig. 6G) and changes in tumor weight (Fig. S24, ESI†). All of the above data demonstrated that NPs-CyBT was capable of monitoring H2O2 levels at the tumor site and exhibited therapeutic potential when combined with radiotherapy.
4. Conclusions
In conclusion, we have successfully designed and synthesized a H2O2-activated, mitochondria-targeting photosensitizer, CyBT, which was further assembled into acid-responsive nanomicelles using the acid-dissociable polymer PEG-b-PDPA for both in vitro and in vivo bio-imaging and combined therapeutic applications. Our approach involved the selection of boronic acid pinacol ester as a H2O2-recognizable caged unit, which was conjugated to the hemicyanine structure through self-eliminating p-aminobenzyl alcohol, and linked to TEMPO groups to boost 1O2 production, thereby enhancing the efficacy of PDT. The resulting nanoparticles, encapsulating CyBT (NPs-CyBT), were internalized by tumor cells, undergoing self-dissociation into small-molecule photosensitizers under the acidic conditions of the lysosome. These molecules were then directed to the mitochondria, activated by radiotherapy-induced H2O2, and exposed the tumor to precision PDT. Most notably, NPs-CyBT was effectively applied to monitor H2O2 levels in a HeLa tumor-bearing mouse model of radiotherapy tolerance and to synergize with PDT, playing a significant role in inhibiting tumor growth and preventing recurrence and metastasis. We are confident that NPs-CyBT will emerge as an excellent platform for the non-invasive theranostics of H2O2-related diseases in the future.
Data availability
All data generated or analysed during this study are available, including the manuscript and its ESI.†
Conflicts of interest
There are no conflicts to declare.
Acknowledgements
This study was financially supported by the National Natural Science Foundation of China (No. 21775046, 22074043, and 32311530041) and the Science and Technology Commission of Shanghai Municipality (No. 3490712700).
References
- L. Zheng, R. Zhu, L. Chen, Q. Fu, J. Li, C. Chen, J. Song and H. Yang, Nano Res., 2021, 14, 3744–3755 CrossRef CAS.
- C. Y. Y. Yu, H. Xu, S. Ji, R. T. K. Kwok, J. W. Y. Lam, X. Li, S. Krishnan, D. Ding and B. Z. Tang, Adv. Mater., 2017, 29, 1606167 CrossRef PubMed.
- Y. Chen, P. Gao, T. Wu, W. Pan, N. Li and B. Tang, Chem. Commun., 2020, 56, 10621–10630 RSC.
- Y. Ren, P. Yang, C. Li, W. Wang, T. Zhang, J. Li, H. Li, C. Dong, W. Meng and H. Zhou, Cell Death Discovery, 2023, 9, 267 CrossRef CAS PubMed.
- G. Petroni, L. C. Cantley, L. Santambrogio, S. C. Formenti and L. Galluzzi, Nat. Rev. Clin. Oncol., 2022, 19, 114–131 CrossRef CAS PubMed.
- C.-H. Hsieh, C.-P. Wu, H.-T. Lee, J.-A. Liang, C.-Y. Yu and Y.-J. Lin, Free Radical Biol. Med., 2012, 53, 649–658 CrossRef CAS PubMed.
- T. Shono, N. Yokoyama, T. Uesaka, J. Kuroda, R. Takeya, T. Yamasaki, T. Amano, M. Mizoguchi, S. O. Suzuki, H. Niiro, K. Miyamoto, K. Akashi, T. Iwaki, H. Sumimoto and T. Sasaki, Int. J. Cancer, 2008, 123, 787–792 CrossRef CAS.
- L. Xiong, B. Tan, X. Lei, B. Zhang, W. Li, D. Liu and T. Xia, IUBMB Life, 2021, 73, 1092–1102 CrossRef CAS PubMed.
- T. B. Steinbichler, J. Dudás, S. Skvortsov, U. Ganswindt, H. Riechelmann and I.-I. Skvortsova, Mol. Cancer, 2019, 18, 58 CrossRef PubMed.
- E. Fernström, K. Minta, U. Andreasson, Å. Sandelius, P. Wasling, A. Brinkmalm, K. Höglund, K. Blennow, J. Nyman, H. Zetterberg and M. Kalm, J. Intern. Med., 2018, 284, 211–225 CrossRef PubMed.
- Y. H. Kim and M. Mishima, J. Clin. Oncol., 2016, 34, 767 CrossRef.
- Y. Philippou, H. Sjoberg, A. D. Lamb, P. Camilleri and R. J. Bryant, Nat. Rev. Urol., 2020, 17, 321–338 CrossRef.
- A. Nguyen, S. Kumar and A. A. Kulkarni, Small Methods, 2022, 6, 2200718 CrossRef CAS PubMed.
- Q. Shang, Y. Dong, Y. Su, F. Leslie, M. Sun and F. Wang, Adv. Drug Delivery Rev., 2022, 185, 114308 CrossRef CAS PubMed.
- Y. An, D. Xu, X. Wen, C. Chen, G. Liu and Z. Lu, Adv. Healthcare Mater., 2024, 13, 2301326 CrossRef CAS PubMed.
- J. Li, T. Wang, F. Jiang, Z. Hong, X. Su, S. Li and S. Han, J. Mater. Chem. B, 2021, 9, 5829–5836 RSC.
- W. Cao, Y. Zhu, F. Wu, Y. Tian, Z. Chen, W. Xu, S. Liu, T. Liu and H. Xiong, Small, 2022, 18, 2204851 CrossRef CAS PubMed.
- J. Xu, Y. Lai, F. Wang, Z. Zou, R. Pei, H. Yu and Z. Xu, Chin. Chem. Lett., 2023, 34, 108332 CrossRef CAS.
- J. Gao, X. Jiang, S. Lei, W. Cheng, Y. Lai, M. Li, L. Yang, P. Liu, X. Chen, M. Huang, H. Yu, H. Xu and Z. Xu, Nat. Commun., 2024, 15, 6608 CrossRef CAS.
- Y. Lai, Y. Dang, F. Li, C. Ding, H. Yu, W. Zhang and Z. Xu, Adv. Funct. Mater., 2022, 32(23), 2200016 CrossRef CAS.
- Z. Zhu, Y. Feng, Q. Tian, J. Li, C. Liu, Y. Cheng, S. Zhang, Y. Dang, J. Gao, Y. Lai, F. Zhang, H. Yu, W. Zhang and Z. Xu, JACS Au, 2024, 4, 4032–4042 CrossRef CAS PubMed.
- W. Cao, Y. Zhu, F. Wu, Y. Tian, Z. Chen, W. Xu, S. Liu, T. Liu and H. Xiong, Small, 2022, 18, 2204851 CrossRef CAS.
- Kenry and B. Liu, Acc. Mater. Res., 2022, 3, 721–734 CrossRef CAS.
- L. K. McKenzie, H. E. Bryant and J. A. Weinstein, Coord. Chem. Rev., 2019, 379, 2–29 CrossRef CAS.
- P. E. Morales, C. Arias-Durán, Y. Ávalos-Guajardo, G. Aedo, H. E. Verdejo, V. Parra and S. Lavandero, Mol. Aspects Med., 2020, 71, 100822 CrossRef.
- S.-F. Wang, L.-M. Tseng and H.-C. Lee, J. Biomed. Sci., 2023, 30, 61 CrossRef CAS PubMed.
- T. Shimura, J. Kobayashi, K. Komatsu and N. Kunugita, Cell Cycle, 2016, 15, 1099–1107 CrossRef CAS.
- Y. Zhang, C. Pang, C. Zhang, Y. Wang, P. Wang, Y. Chen, J. Wang, Y. Hu, C. Liu, H. Liang, G. Xie and J. Ou, Cell. Mol. Life Sci., 2023, 80, 242 CrossRef CAS PubMed.
- G. Lu, H. W. S. Tan, T. Schmauck-Medina, L. Wang, J. Chen, Y.-L. Cho, K. Chen, J.-Z. Zhang, W. He, Y. Wu, D. Xia, J. Zhou, E. F. Fang, L. Fang, W. Liu and H.-M. Shen, Autophagy, 2022, 18, 2865–2879 CrossRef CAS.
- R. Wang, Y. Shang, B. Chen, F. Xu, J. Zhang, Z. Zhang, X. Zhao, X. Wan, A. Xu, L. Wu and G. Zhao, Cell Death Dis., 2022, 13, 851 CrossRef CAS PubMed.
- Y. Huang, Z. Xiao, Z. Guan, Y. Shen, Y. Jiang, X. Xu, Z. Huang and C. Zhao, J. Controlled Release, 2020, 319, 119–134 CrossRef CAS PubMed.
- D. Kessel and J. J. Reiners, Autophagy, 2020, 16, 2098–2101 CrossRef CAS.
- L. Kma and T. J. Baruah, Biotechnol. Appl.
Bio., 2022, 69, 248–264 CrossRef CAS.
- F. Xu, H. Li, Q. Yao, H. Ge, J. Fan, W. Sun, J. Wang and X. Peng, Chem. Sci., 2019, 10, 10586–10594 RSC.
- Q. Kong, B. Ma, T. Yu, C. Hu, G. Li, Q. Jiang and Y. Wang, New J. Chem., 2020, 44, 9355–9364 RSC.
- Y. Lai, Y. Dang, Q. Sun, J. Pan, H. Yu, W. Zhang and Z. Xu, Chem. Sci., 2022, 13, 12511–12518 RSC.
- B. Hou, J. Ye, J. Li, Z. Xu and H. Yu, Nano Today, 2022, 47, 101661 CrossRef.
- J. Gao, B. Hou, Q. Zhu, L. Yang, X. Jiang, Z. Zou, X. Li, T. Xu, M. Zheng, Y.-H. Chen, Z. Xu, H. Xu and H. Yu, Nat. Commun., 2022, 13, 4318 CrossRef CAS PubMed.
|
This journal is © The Royal Society of Chemistry 2025 |
Click here to see how this site uses Cookies. View our privacy policy here.