Intrafibrillar calcium carbonate mineralization of electrospinning polyvinyl alcohol/collagen films with improved mechanical and bioactive properties†
Received
5th July 2024
, Accepted 24th October 2024
First published on 31st October 2024
Abstract
Collagen films play an essential role in guided bone-regeneration (GBR) techniques, which create space, promote cell adhesion, and induce osteogenic differentiation. It is therefore crucial to design appropriate GBR films to facilitate bone regeneration. However, current electrospun collagen scaffolds used as bioactive materials have limited clinical applications due to their poor mechanical properties. In this study, polyvinyl alcohol (PVA)/collagen (Col) films were electrospun by mixing PVA and type I collagen solution. For the first time, the intrafibrillar mineralization of aragonite nanocrystals within the PVA/Col fibrils was achieved, resulting in the formation of a hierarchical, bioactive film. The PVA/Col–CaCO3 film exhibited good mechanical properties, with hardness and Young's modulus values of 211.6 ± 0.1 MPa and 5.6 ± 1.7 GPa, respectively. Furthermore, bone marrow mesenchymal stem cells (BMSCs) inoculated onto the PVA/Col–CaCO3 film demonstrated robust adhesion and proliferation. The mineralized fibrils effectively stimulated the growth of BMSCs while suppressing cell apoptosis. Besides, the PVA/Col–CaCO3 film significantly induced the osteogenic differentiation of BMSCs, revealing its potential biomedical applications in hard tissue engineering.
1. Introduction
The natural process of bone healing following a bone fracture or other injuries is defined as bone repair.1,2 To create a proper space and surface for bone regeneration, the most common methods involve the use of bioactive auxiliary devices, such as artificial films and scaffolds.3–5 These are designed to promote cell adhesion, proliferation, and osteogenic differentiation while preventing connective tissues from filling bone defects.6 To date, biodegradable artificial films prepared with collagen fibrils have been the most frequently used in clinical guided bone-regeneration (GBR) operations, showing good biocompatibility and low complication rates.7 Recent research results have indicated that the mechanical properties of scaffolds’, including strength, stiffness, and elasticity, are essential elements that can directly affect cell adherence, proliferation, and differentiation.8 However, the main drawbacks of these films are their poor mechanical properties, combined with rapid degradation rates and low osteogenic activities, severely limiting their clinical effects. Specifically, collagen-based films can collapse and degrade easily, failing to sustain advantageous space for sufficient time and to provide abundant physical and chemical signals for bone regeneration.9,10
Nanofibrous electrospun films have gained increasing attention in the field of tissue engineering due to their numerous advantages, such as convenient manufacturing, abundant raw materials and good mechanical properties.11–13 Huang et al. constructed polylactic-co-glycolic acid (PLGA) composite electrospun scaffolds to promote the osteogenic differentiation of rat bone marrow-derived mesenchymal stem cells (rBMSCs).14 As a synthetic polymer, polyvinyl alcohol (PVA) has also been widely used in the biomedical field because of its inherent non-toxicity and superior physical properties compared to traditional scaffold materials.15 Although polymer composites produced via electrospinning exhibit a certain degree of mechanical strength, this remains insufficient for the requirements of GBR. What's more, they are also easily degraded and insufficient to induce osteoblast-related cells’ migration and differentiation in the long term.
Drawing inspiration from biological processes,16–18 Ping et al. revealed that the intrafibrillar mineralization of collagen generates contractile stresses, which may enhance the mechanical properties of collagen fibrils.19 Meanwhile, the intrafibrillar mineralization process could make the collagen fibrils resistant to degradation, and provide microstructural and microenvironmental advantages for the proliferation and differentiation of osteoblasts.20 Niu et al. synthesized a collagen/polyelectrolyte composite by cross-linking high molecular weight polyacrylic acid (HPAA) to collagen membranes, so as to achieve effective in situ intrafibrillar self-mineralization.21 In most cases, the collagen scaffolds are mineralized with hydroxyapatite (HAP) crystals to enhance their mechanical properties and promote osteogenesis.22
Similar to the bone, nacres and pearls are composed of multiscale ordered calcium carbonate (CaCO3) crystals (∼95 wt%) and an organic matrix (∼5 wt%).23In vitro and in vivo studies have demonstrated their osteogenic potentials. Lopez et al. found that nacre-based materials could lead to the formation of osteoid nodules induced by human osteoblasts.24 However, previous studies have generally ignored that there are three different crystal forms of calcium carbonate: calcite, vaterite, and aragonite.25 Minerals in living organisms, such as nacres, shells, and algae, are usually aragonite. Compared to the different structures, Feng et al. evidenced that calcium carbonate in the aragonite form was more beneficial for cell proliferation and differentiation.26 In order to prepare high-strength biofilms, the artificial materials can be obtained by simulating and controlling the biomineralization process. Nevertheless, the current organic–inorganic composites for bone healing are mainly composed of calcite crystals according to the reported research, and so far, the bioactive performances of CaCO3-based materials in various crystal forms still remain to be explored.
Hence, this study innovatively devised a multiscale ordered, highly hierarchical, organic–inorganic composite material. Through the electrospinning technique, an organic composite framework composed of polyvinyl alcohol (PVA) and collagen was fabricated first. Then, a hierarchically structural scaffold resembling natural bone tissue was obtained by mimicking the biomineralization process. Unlike previous HAP-based biomaterials, the effects of CaCO3-based-biomineralization on the mechanical properties of electrospun PVA/Col composite films were explored. The hardness and Young's modulus values of the mineralized biofilms were 211.6 ± 0.1 MPa and 5.6 ± 1.7 GPa, respectively. Also, bone marrow-derived mesenchymal stem cells (BMSCs) were seeded and cultured with these biofilms to investigate the influence of their topological structure and chemical release on cells. The results showed that the biologically-mineralized films demonstrated good mechanical properties and biocompatibility, which are beneficial for promoting cell proliferation and differentiation. This provides a novel strategy for manufacturing high-performance GBR films.
2. Experimental
2.1 Materials
Type I collagen (Cattle's Achilles tendon) was bought from Sangon Biotech (Shanghai, China). Polyacrylic acid (PAA, Mw 240
000) and 4-(2-hydroxyethyl) piperazine-1-ethane-sulfonic acid (HEPES) were provided by Alfa Aesar (Shanghai, China). Polyvinyl alcohol (PVA, Mw 80
000), Na2CO3, CaCl2, NaCl, and acetic acid were obtained from Sigma-Aldrich (Shanghai, China). All the chemical reagents were used directly without further purification.
2.2 Electrospinning PVA/Col films
The collagen solution (6 mg mL−1) was prepared by dissolving the collagen powder in 1 M acetic acid under magnetic stirring overnight. PVA solution at 10 wt% was obtained by dissolving the powder in 90 °C distilled water for 4 h and stirring until complete homogeneity was achieved. A PVA/Col blend solution was fabricated by mixing 10 wt% PVA solution and 6 mg mL−1 collagen solution, with a mass ratio of 1
:
1. The best electrospinning conditions for synthesizing the aligned PVA/Col nanofibers were as follows: a routine 10 mL syringe with a #20 flat head needle; needle collector distance of 15 cm; positive voltage of 15 kV, negative voltage of −6 kV; flow rate of 0.1 mm min−1; and rotating speed of 80 rpm min−1. The humidity was regulated to 40%, and the temperature to 30 °C. The non-aligned nanofibers were obtained by using a low-speed cylinder collector. The electrospun films were separated from the collector and vacuum dried at 40 °C for 24 h.
2.3 Preparation of the CaCO3 mineralization solution
The mineralization solution was prepared by the titration of 25 mL solution A (20 mM Na2CO3, 300 mM NaCl) to 25 mL solution B (20 mM CaCl2, 20 mM HEPES buffer, and 400 μg mL−1 PAA). This process was controlled by a high-resolution peristaltic pump at a speed of 2.5 mL min−1. The pH of the CaCO3 solution was finely adjusted to 9.0 by slowly dropping in 2 M NaOH.
2.4 Mineralization of the PVA/Col films
The newly fabricated PVA/Col films were immersed in the mineralization solution for more than 48 h, and then taken out. They were subsequently washed and rinsed using deionized water to remove possible contaminants. The PVA/Col–CaCO3 films were obtained after drying at room temperature. As for the control groups, one was the unmineralized PVA/Col film not placed in the mineralization solution, and the other one was the extrafibrillarly mineralized PVA/Col film (PVA/Col@CaCO3), obtained by placing it into the mineralization solution without PAA.
2.5 Materials characterization
The crystal structures of the samples were investigated via X-ray diffraction (XRD, Empyrean, Holland) with Cu Kα radiation (V = 45 kV, I = 40 mA) in the diffraction angle range of 20°–80°. The surface morphology of the mineralized films was revealed by field emission scanning electron microscopy (FESEM, SU8230, Japan) at 5 kV. The microstructural analysis was also performed via transmission electron microscopy (TEM, Talos F200S, America) and high-resolution transmission electron microscopy (HRTEM), with an energy-dispersive spectroscopy (EDS) detector. The phase identification and crystallographic orientation of the crystals were obtained by selected area electron diffraction (SAED). X-Ray photoelectron spectroscopy (XPS) measurements were conducted using a Thermo Fisher Escalab250Xi system (USA). The samples were also characterized by a Raman spectroscopy system (LABRAM HR Evolution, HORIBA Scientific, Japan), equipped with a 532 nm laser. Thermogravimetric analysis (TGA, STA449F3 Jupiter, Germany) was performed to ascertain the organic–inorganic content of the films. The analysis was performed in an air atmosphere, heated from 25 °C to 1000 °C, at a heating rate of 5 °C min−1. Fourier transform infrared spectroscopy (FTIR, Nexus, America) was used to detect the functional groups in the range from 4000–500 cm−1. The size distribution and surface charge of calcium carbonate precursors in the mineralization solution with different pH were respectively determined by dynamic light scattering (DLS) and using a Zetasizer ultra system (Malvern Panalytical, UK). For the in vitro degradation experiments, these films were incubated in 0.1 mg mL−1 Clostridium histolyticum collagenase (Sigma-Aldrich, USA) aqueous solution at 37 °C, which was renewed every 3 days. The original weight of each sample was marked as W0, and the subsequent weight (W1) after lyophilization was noted for each time point. The percentage remaining mass (%) was calculated as W1/W0 × 100%. The mechanical properties of the films were evaluated via nanoindentation tests (TI-980, Bruker-Hysitron, Germany). The hardness and Young's modulus of the samples were measured via the continuous stiffness measurement (CSM) method using a Berkovich indenter tip. The substrate effects were eliminated by ensuring that the depths of all the indentations did not exceed one-tenth of the total thickness of the films. The tensile strength of these films was determined using a self-designed mechanical testing setup (Fig. S6, ESI†). The samples were cut into 12 mm × 2 mm (length × width) stripes and subjected to tensile test with a loading speed of 20 μm s−1 until broken. For accurate and dependable results, each sample underwent at least three tests.
2.6 Cell culture
Bone marrow-derived mesenchymal stem cells (BMSCs) were isolated from the femurs and tibias of 4-week-old male Sprague Dawley (SD) rats. The BMSCs were cultured in α-MEM culture medium supplemented with 10% fetal bovine serum (FBS) and 1% penicillin/streptomycin in a humified incubator at a temperature of 37 °C and a partial pressure of 5% CO2. Prior to the cell experiments, the samples were soaked in 75% ethyl alcohol and sterilized with UV light irradiation for 2 h. Then, after the BMSCs had adhered on the cell-cultured plate for 12 h, the samples from each group were washed with phosphate-buffered saline (PBS) twice and put in the wells at a ratio of 10 mg mL−1 culture medium. The cells at passages 3–5 were used for the further tests.
2.7 Cell proliferation
In order to evaluate cell adhesion and viability, cell skeleton staining was performed after coculturing with samples for 24 h. Briefly, cells were washed twice with preheated PBS, fixed with 4% paraformaldehyde (PFA), treated with Triton X-100, and incubated with FITC (Yeasen, Shanghai, China) and 4′,6-diamidino-2-phenylindole (DAPI, Beyotime, Shanghai, China) for 30 min and 30 s, respectively. Cell adhesion was characterized by confocal laser scanning microscopy (CLSM, Leica-LCS-SP8-STED, Leica, Wetzlar, Germany; excitation/emission wavelength at 488/530 nm) and ImageJ software (NIH, Bethesda, MD, USA).
BMSCs were seeded at a density of 5 × 104 cells per well and cultured for 1, 3, 5, and 7 days. Medium containing 10% CCK-8 kit solution (Dojindo Kagaku Co, Kumamoto, Japan) was added to each well and then incubated in the dark at 37 °C for 60 min. Enzyme marker (PowerWave 340, BioTek Instruments, Winooski, VT, USA) was applied to record the absorbance of each well at 450 nm. The relative cell viability (%) was also calculated afterward, to allow evaluating the effects of the sample coculturing on cell proliferation compared to the blank control.
Crystal violet staining was also applied on cells cocultured for 24 h for cell viability characterization. After the old medium was removed, the cells were fixed with 4% PFA at room temperature for 15 min, then stained with 0.1% crystal violet (Beyotime Biotechnology, Shanghai, China) for another 15 min, and washed twice with PBS. Cell morphology was observed by optical microscopy.
2.8 Cell culture
After coculturing for 24 h with the samples, the calcein-AM/propidium iodide (PI) live–dead cell staining kit (Solarbio Life Sciences, Beijing, China) was adopted to evaluate cell death. Calcein-AM and PI were added to wells at a ratio of 1
:
3 and incubated for 20 min, carefully protected from light. An inverted fluorescence microscope (Olympus IX83, Olympus, Japan) was used to capture representative images and Image J software was applied for quantitative analysis.
Cell apoptosis evaluation was also performed using the Annexin V-FITC/PI apoptosis detection kit (Sangon Biotech, Shanghai, China), according to the manufacturer's instructions. BMSCs were seeded and cultured for 24 h as mentioned before, then washed with PBS and resuspended in 195 μL binding buffer (1×) at a density of 5 × 105 cells mL−1. Next, 5 μL Annexin V-FITC and 10 μL PI were added and incubated 10 min at room temperature, protected from light. Finally, the percentage of viable cells from each group was analyzed using a CytoFLEX flow cytometer (Beckman Coulter, Indianapolis, USA).
2.9 Osteogenic differentiation
Alkaline phosphatase (ALP) staining, immunofluorescence, and Alizarin Red S (ARS) staining were performed to assess the osteoinductive properties of the films. BMSCs were seeded at a density of 5 × 104 cells cm−2 and cultured until 80–90% confluence. Different materials were immersed in the culture medium. The sterilization process and ratio of materials to the culture medium were the same as previously described. To induce osteogenic differentiation, to the culture medium was added 10 mM β-glycerophosphate, 50 μg mL−1 ascorbic acid, and 1 nM dexamethasone, and the BMSCs were cultured for 7, 14, and 21 days.
For ALP staining and ALP activity measurements, the ALP assay kit (Beyotime, Shanghai, China) and BCIP/NBT color development kit (Beyotime, Shanghai, China) were utilized at 7 and 14 days. To evaluate the expression levels of osteopontin (OPN), immunofluorescence staining was performed at 7 and 14 days. Osteopontin polyclonal IgG antibody (Proteintech Group, USA), Cy3 AffiniPure Goat Anti-Rabbit IgG (H + L) (Biosharp, Anhui, China), FITC (Yeasen, Shanghai, China), and DAPI (Beyotime, Shanghai, China) were applied in accordance with the manufacturers’ protocols. Immunofluorescence characterization was performed using CLSM and analyzed with ImageJ software to evaluate the fluorescence intensity and distribution. Besides, after 21 days of osteogenic induction, the BMSCs were stained with Alizarin Red S solution (OriCell, Guangzhou, China) to assess mineralization. The calcium nodules were then dissolved in hexadecylpyridinium chloride for 1 h, and the absorbance was recorded at 562 nm for quantitative analysis of the mineral deposits
2.10 Statistical analysis
Statistical analysis was conducted using GraphPad Prism (GraphPad Prism software, USA) and Origin (OriginLab, USA). After being collected and calculated, the data of the present study were expressed as the mean ± standard deviation and found to follow the normal distribution. One-way ANOVA and Tukey's multiple comparison tests were used to compare the population means between different groups. The test level of difference was set at P < 0.05.
3. Results and discussion
3.1 Preparation and characterization
In short, the PVA/Col–CaCO3 film was obtained by immersing the PVA/Col film in the mineralization solution for more than 48 h (Fig. 1). First, the PVA/Col films were prepared by electrospinning a mixed solution of PVA and collagen. The schematic shows the SEM images of PVA/Col films, in which the collagen fibrils were coated with PVA, forming an interweaved network structure. Then, the PVA/Col film was put into the mineralization solution. As time progressed, semi-mineralized to completely mineralized fibrils could be obtained. For further discussion, Fig. 2(A)–(C) shows SEM images of the PVA/Col films, intrafibrillarly mineralized PVA/Col (PVA/Col–CaCO3) films, and extrafibrillarly mineralized PVA/Col films (PVA/Col@CaCO3). It could be clearly observed in Fig. 2(A) that the PVA/Col nanofibrils were uniformly dispersed and interwoven into a network structure. As for the single PVA/Col fibril, its diameter was about 160 ± 30.6 nm. In the FTIR spectrum, the characteristic peaks at 1567 and 854 cm−1 in the curve of PVA/Col belonged to the collagen and PVA, respectively (Fig. S1, ESI†). As shown in Fig. 2(B), in the mineralization solution groups with PAA, a significant increase in the diameters of nanofibrils was observed, indicating that the fibrils had undergone an intrafibrillar mineralization process. Fig. 2(B) indicates that platelet-like CaCO3 nanocrystals (Fig. S1, ESI†) were deposited on the fibrils’ surface of the PVA/Col film. The diameters of the fibrils changed from 160 ± 30.6 nm before mineralization (Fig. 2(A)) to 1000 ± 170 nm after intrafibrillar mineralization. The swelling of the PVA/Col fibrils during mineralization resulted from the penetration of the nanocrystals into the fibrils. Then, these nanocrystals continuously stacked and grew from inside to outside. From the XRD patterns (Fig. 2(D)), characteristic peaks of the PVA/Col–CaCO3 could be seen corresponding to the standard aragonite phase (PDF#00-003-1067). Also, the Raman spectrum (Fig. 2(E)) displayed characteristic peaks located at 206 and 705 cm−1, corresponding to the lattice vibration of aragonite crystals in the PVA/Col–CaCO3. The chemical state of the elements in PVA/Col–CaCO3 was analyzed by XPS (Fig. S2, ESI†). As shown in Fig. S2(A) (ESI†), peaks of Ca 2p, C1s, N1s, and O1s could be clearly found in the survey spectrum of PVA/Col–CaCO3. The appearance of the N1s peak depended on the protein content in the collagen. The high-resolution C1s spectrum could be divided into four peaks (Fig. S2(B), ESI†) related to C–C, C–N, C–O, and C
O, which were indexed to the covalent bonds, indicating the bonding between the CaCO3 crystal surfaces and collagen molecules.
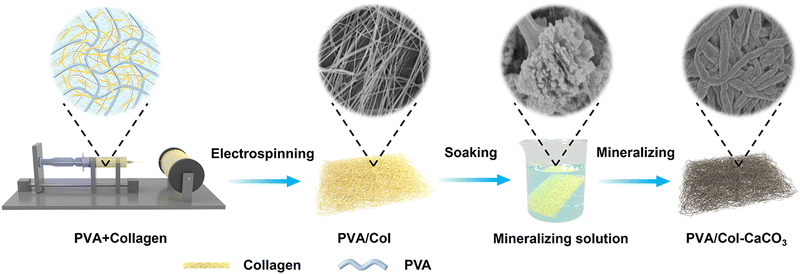 |
| Fig. 1 Schematic of the fabrication of PVA/Col–CaCO3 films. | |
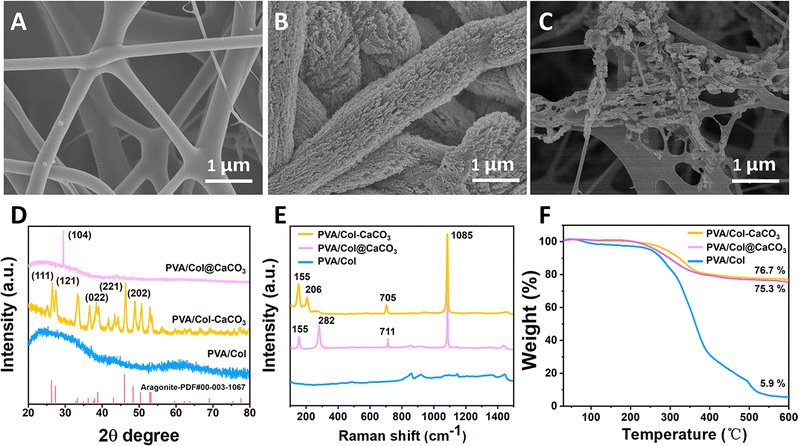 |
| Fig. 2 Mineralization of PVA/Col film with calcium carbonate. (A) SEM image of electrospun PVA/Col film. (B) SEM image of intrafibrillar mineralization of PVA/Col film (PVA/Col–CaCO3). (C) SEM image of extrafibrillar mineralization of PVA/Col film (PVA/Col@CaCO3). (D) XRD patterns, (E) Raman spectra and (F) TG curves. | |
For comparison, a SEM image of the PVA/Col films mineralized in the absence of PAA is presented in Fig. 2(C). It could be seen that there was no formation of precursors, and the CaCO3 calcite crystals were directly formed in solution and deposited on the surface of the PVA/Col films. The XRD pattern of PVA/Col@CaCO3 showed that the main diffraction peak could be assigned to the (104) crystal plane of the calcite phase (Fig. 2(D)). The Raman spectrum (Fig. 2(E)) displayed bands at 282 and 711 cm−1 related to the calcite crystals in PVA/Col@CaCO3. What's more, the FTIR spectrum (Fig. S3, ESI†) showed two characteristic bands at 1791 and 877 cm−1, corresponding to the C
O and v2 modes of calcium carbonate. Another two peaks at 1415 and 714 cm−1 were assigned to the characteristics v3 and v4 modes of crystalline calcite, while the peak at 708 cm−1 was indeed a characteristic absorption peak of aragonite.27 This result corresponded to the previous Raman spectrum. This confirmed that in the absence of PAA, calcium carbonate solution did not penetrate into the interior of the fibrils. Instead, it directly formed crystalline deposits on the surface of the PVA/Col film in the solution (such as can be seen in Fig. 2(C)), with a calcite crystal structure. However, when PAA was added into the mineralization solution, the formed liquid-phase precursors entered the interior of the fibrils,28 and mineralization occurred within the confined space, resulting in the formation of aragonite crystals.29 Also, the thermogravimetric analysis illustrated that the organic matrix constituted about 23.3% of the fully mineralized PVA/Col films (Fig. 2(F)). Biodegradability is an important indicator of bone-repair biomaterials. In this study, after a collagenase-based degradation test for 30 days in vitro, the residual mass of PVA/Col and PVA/Col@CaCO3 was higher than 20%. Also, PVA/Col–CaCO3 exhibited the slowest degradation rate with a retention of 86% of the initial weight, suggesting its promising application as a durable GBR film (Fig. S4, ESI†).
The magnified TEM images (Fig. 3(A)) showed that the size of the nanocrystals was consistent with the SEM data. The HRTEM analysis of the samples demonstrated uniform lattice fringes of various platelet-like particles, indicating a high degree of orientation (Fig. 3(B) and (C)). Additionally, the related SAED pattern illustrated characteristic diffraction spots corresponding to the rhombohedral structure of aragonite (Fig. 3(B) inset), signifying a continuous lattice fringes alignment and orientation of the nanoparticle building blocks. The crystal lattice with an interplanar spacing of 0.28 nm and 0.34 nm corresponded to the (002) and (111) planes of aragonite (Fig. 3(C)). Although the mineralized fibrils contained nanoparticle units, they did not exhibit the typical ring pattern of polycrystalline calcite. Intrafibrillar mineralization can be seen as a process of crystal growth within a confined space. Due to the intermolecular channels in the fibrils, the polymer-induced liquid precursors (PILPs) may diffuse along the central axis of the fibril.30 The crystallization of the precursors was regulated by the collagen molecules, and eventually the morphology and orientation of the nanocrystals were influenced by the gap zone of the collagen, resulting in a co-oriented platelet-like structure.25 It was also confirmed that the crystals grew aligned with the longitudinal axis of the fibrils, same as we mentioned previously. The EDS mapping indicated that the minerals were calcium carbonate phase, with C, Ca, and O elements uniformly distributed in the mineralized fibrils (Fig. 3(D)).
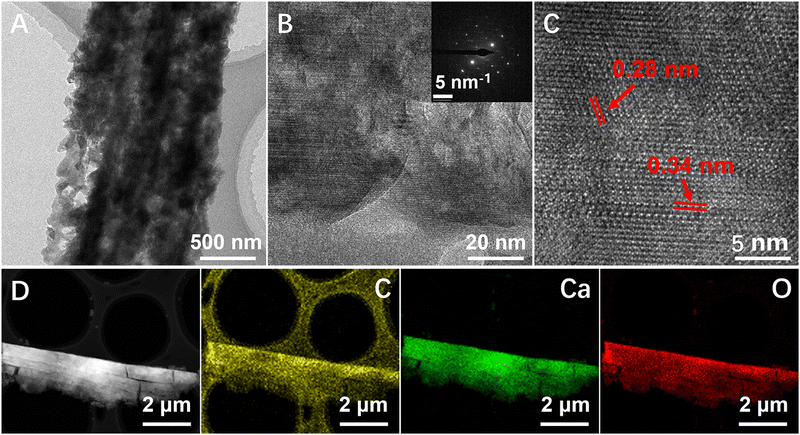 |
| Fig. 3 (A) TEM image of a fully mineralized PVA/Col fibril (PVA/Col–CaCO3). (B) and (C) HRTEM images of the crystal. Inset is the single crystal-like SAED. (D) EDS mapping of a fully mineralized PVA/Col fibril. | |
The intrafibrillar mineralization of the PVA/Col films could be distinctly observed through the changes in the morphology (Fig. 4(A)–(C)). After placing the PVA/Col film in the mineralization solution for a certain period, as the amorphous precursors entered the interior of the fibrils, the mineralized regions appeared in segmented crystals along parts of the fibrils (Fig. 4(A)). Aragonite crystals accumulated locally and grew along the longitudinal axis of the fibrils until they reached full maturity over time (Fig. 4(B)). Fig. 4(C) confirmed the uniform growth of CaCO3 crystals, displaying a short platelet-like structure within the fibrils. As the mineralization time increased, the film underwent continuous growth, forming patch-like mineralized regions (Fig. 4(D)). Eventually, these regions covered the entire film, achieving complete mineralization. It is well known that intrafibrillar mineralization can be attributed to PILP entering the interior of the fibrils, and then the precursors nucleate and crystallize within the fibrils.31 To further confirm the entry of PILP into the PVA/Col film, the zeta potentials of the amorphous precursors in the mineralization solution and the PVA/Col film were tested separately, as shown in Fig. 4(E) and (F). The zeta potential of the amorphous calcium carbonate exhibited a negative charge (Fig. 4(E)). The zeta potential of the PVA/Col film was essentially stable at around −4.3 mV, independent of the pH value (Fig. 4(F)). Nevertheless, the gap region of single collagen fibrils was typically positively charged. Consequently, a potential difference existed between the PVA/Col fibrils and amorphous precursors, facilitating the entry of PILP for mineralization via electrostatic interaction. Fig. 4(G) shows the intrafibrillar mineralization process of the single fibril of the PVA/Col film. The single fibril was the organic composite framework, formed by wrapping PVA on the outer surface of collagens. Through biomineralization simulation, the PILP adhered and penetrated into the fibrils, inducing intrafibrillar mineralization and consequently forming a bone-like hierarchical structure.
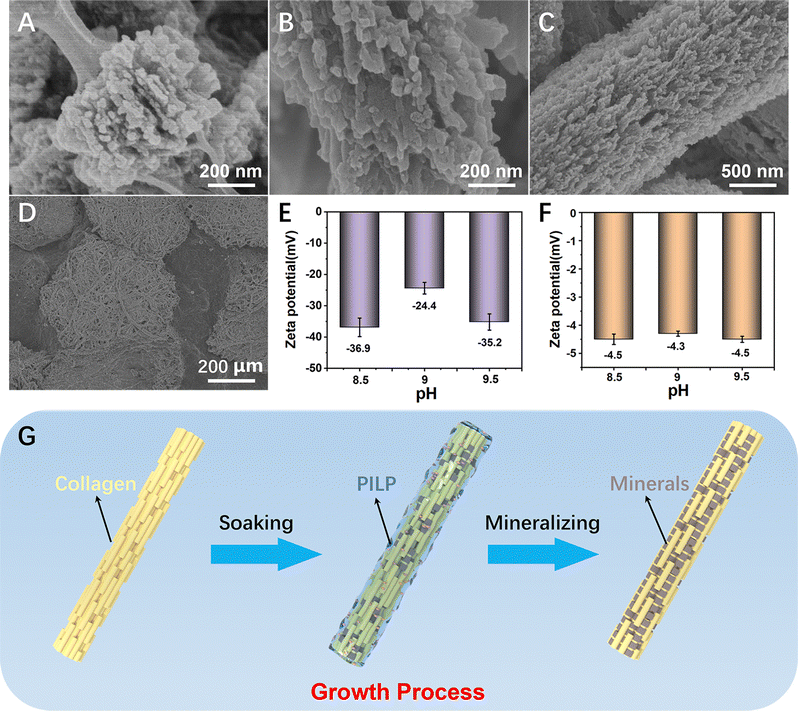 |
| Fig. 4 Process of intrafibrillar single fibril (PVA/Col film) mineralization of calcium carbonate. (A) SEM image of the partial mineralized single fibril. (B) SEM image of a fracture area of a mineralized single fibril. (C) SEM image of a fully mineralized single fibril. (D) SEM image of mineralized regions in the PVA/Col film. (E) and (F) Zeta potentials of the amorphous precursors and the PVA/Col film. (G) Schematic of the process of intrafibrillar mineralization. | |
3.2 Mechanical properties
Nanoindentation measurements were employed to measure the hardness (H) and Young's modulus (EY) values of the synthetic samples. In order to avoid substrate and surface effects, the H and EY of the composites were evaluated by the continuous stiffness measurement (CSM) method with Berkovich probes at a loading of 1500 μN, which did not exceed a 10% depth of total thickness. The synthetic (PVA/Col–CaCO3) films exhibited H and EY values of 211.6 ± 0.1 MPa and 5.6 ± 1.7 GPa, respectively (Fig. 5(D) and (E)), which were comparable to those of general biofilms (Table S1, ESI†). The hardness and Young's modulus values of PVA/Col–CaCO3 were much higher than those of PVA/Col (H = 113.5 ± 0.1 MPa, EY = 2.1 ± 1.2 GPa). This could be explained based on the finding that after mineralization within collagen fibrils, the directional arrangement of minerals greatly improved the overall strength. The synthetic (PVA/Col–CaCO3) contained 76.7 wt% of inorganic minerals, due to the intrafibrillar mineralization of CaCO3, which improved the mechanical properties of the composite materials. The tensile strength is also an important functional parameter for GBR films. Tensile tests were thus carried out to measure the tensile stress–strain performances of these films. As presented in Fig. 5(A)–(C), PVA/Col–CaCO3 exhibited a statistically higher elastic modulus (209.9 MPa) than that of PVA/Col (18.4 MPa) and PVA/Col@CaCO3 (125.1 MPa). In addition, the tensile strength of PVA/Col–CaCO3 (9.6 MPa) was significantly higher than that of PVA/Col (2.6 MPa) and PVA/Col@CaCO3 (7.4 MPa). Also, the fracture energy of PVA/Col–CaCO3 was also the highest. The above results strongly implied the enhanced mechanical performances of PVA/Col–CaCO3, attributed to the synergistic reinforcement of the intrafibrillar mineralization and the bone-like hierarchical structure.
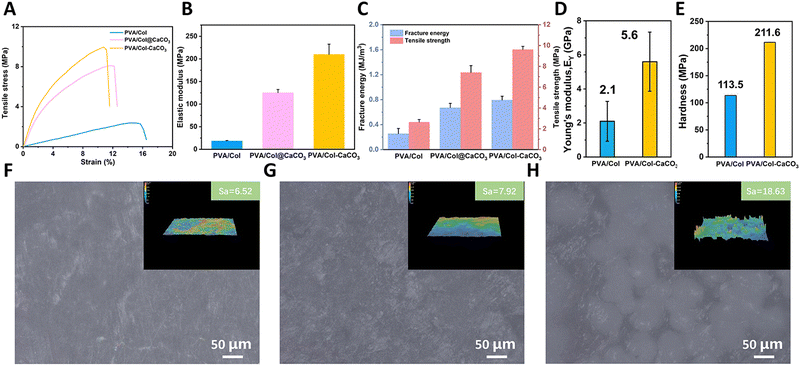 |
| Fig. 5 (A)–(C) Tensile stress–strain curves of the different films and quantitative results of the elastic modulus, fracture energy, and tensile strength. (D)–(E) Mechanical properties of PVA/Col and PVA/Col–CaCO3 tested via nanoindentation tests. (F)–(H) Surface optical microscopy photos of PVA/Col, PVA/Col–CaCO3, and PVA/Col@CaCO3. Inset shows the surface roughness of the films. | |
From Fig. S5 (ESI†), we can see that there were large deviations in the results for the hardness and Young's modulus for PVA/Col@CaCO3 when tested in different areas. The maximum value of Young's modulus was 31.5 GPa and the maximum value of hardness was 4.1 MPa, while the minima were 0.5 GPa and 0.1 MPa, respectively, which show huge gaps. According to previous research, it is believed that the mechanical behaviors of GBR films should be as close to natural bone tissue as possible.32 It has been reported that the elastic moduli of cortical bone and cancellous bone are 12–20 GPa and 0.52–0.62 GPa, respectively.33,34 However, there may also be adverse effects if the films behave excessively tough in some area as they could puncture the soft tissue and expose it to the oral environment accidentally.35 In our study, the mechanical modulus of the PVA/Col–CaCO3 film, which was close to that of natural cancellous bone, was significantly improved compared with pure collagen films and was more even than the PVA/Col@CaCO3 films. By detecting the surface roughness (Sa) of those films, it could be found that the Sa of PVA/Col@CaCO3 was 18.63 μm, which was twice as high as the other two groups (Fig. 5(F)–(H) inset). Fig. 5(F)–(H) present surface optical microscope photos of the three groups. The PVA/Col@CaCO3 film was uneven with some white bulges (CaCO3 particles), while the other two groups were relatively smooth. This was attributed to the non-addition of PAA, which resulted in the direct nucleation and growth of CaCO3 in the solution. Then those calcite crystals deposited freely on the surface of the PVA/Col film. Therefore, its surface was bumpy and uneven with the presence of a large number of CaCO3 particles. When the nanoindentation probe hit the surface of the CaCO3 particles, a higher hardness and Young's modulus appeared, resulting in the uneven strength and poor mechanical properties of the PVA/Col@CaCO3 film. In contrast, for the intrafibrillarly mineralized films with the addition of the PAA, the aragonite nanocrystals were oriented in the fibrils, forming a bone-like hierarchical structure, which enhanced the physical properties. This demonstrated that the improvement of the mechanical properties by intrafibrillar mineralization was far superior to that of extrafibrillar mineralization, and the pre-stress generated by it may play an important role.19
3.3 Cell proliferation
BMSCs were cocultured with samples from various groups for cytocompatibility evaluation, according to previous research.14,36 The proliferation, adhesion, and apoptosis of BMSCs were investigated at multiple time points. A blank control group (only pure culture medium added) was set up to assess the impacts of the materials on cell growth.
To evaluate the impacts of the samples on the fundamental process of cell proliferation, the cytoskeleton structures of BMSCs from different groups were detected in vitro. As exhibited in the representative images, F-actin and the nuclei of BMSCs were stained in green and blue by phalloidin and DAPI under CLSM observation, respectively (Fig. 6(A)). In the first 24 h of cell culture, cells were evenly distributed throughout all groups, and the morphology of the cytoskeleton was relatively uniform, with nuclei and microtubes arranging randomly. The cell adhesion density of the PVA/Col–CaCO3 group displayed no significant difference compared with the other groups (Fig. 6(C)). Quantitative analysis of the mean fluorescence intensity was also conducted, which revealed a noticeably stronger signal for microtubes in the PVA/Col–CaCO3 group, indicating the full extension of the cytoskeleton (Fig. 6(C), P < 0.05). The mean fluorescence intensity of the PVA/Col@CaCO3 group was the lowest among the four groups, however. Crystal violet staining was next performed to examine BMSCs adhesion after coculturing with blank medium, PVA/Col, PVA/Col–CaCO3 and PVA/Col@CaCO3. It was found that PVA/Col–CaCO3 promoted cell proliferation whereas the PVA/Col@CaCO3 group exhibited an apparently lighter hue and collapsed cell morphology (Fig. S7, ESI†).
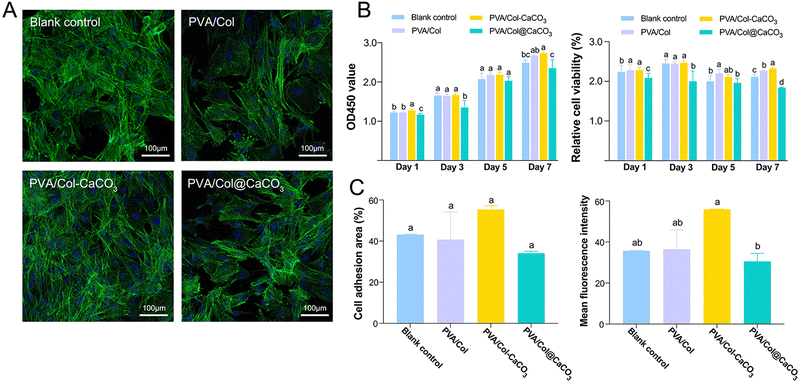 |
| Fig. 6 Evaluation of cell proliferation in vitro. (A) Representative fluorescent images of BMSCs cultured with various samples for 1 day. Cell skeleton and nuclei were stained with green and blue, respectively. (B) OD 450 nm and relative cell viability of BMSCs cultured with various samples detected by a CCK-8 kit on days 1, 3, 5, and 7. (C) Quantitative analysis of the cell adhesion area and mean fluorescence intensity quantification of the cell skeleton from each group on day 1. Data are expressed as mean values ± standard deviations. Groups labeled with the same superscript letters are not significantly different (P > 0.05). | |
In terms of the CCK-8 experiments, the quantity of BMSCs was considerably increased when prolonging the timeframe of cell culture. Meanwhile, it was evident that PVA/Col–CaCO3 obviously boosted the relative viability of BMSCs compared with the other groups at the same time points (Fig. 6(B), P < 0.05). Regarding the OD450 value, a similar trend was indicated. Nevertheless, the PVA/Col@CaCO3 group showed the slightest suppression of cell proliferation among all the groups, demonstrating a slight inhibitory effect on cell growth.
3.4 Cell apoptosis
Live/dead cells staining was performed first. The results showed that BMSCs exposed to PVA/Col–CaCO3 exhibited the most intact cell morphology and the lowest apoptotic rate (Fig. 7(A) and (C), P < 0.05). The effects of the materials on cell apoptosis were further investigated by flow cytometry (Fig. 7(B)). All the groups displayed acceptable levels of apoptosis, with the PVA/Col–CaCO3 group showing the lowest apoptotic tendency of BMSCs (Fig. 7(B)). Besides, a striking finding from Fig. 7(B) is that the application of PVA/Col@CaCO3 sharply raised the cell ratios in terms of Q3, corresponding to the early apoptosis of cells. This was further demonstrated by the quantitative assessment of apoptosis in Fig. 7(D), indicating that co-culture with PVA/Col–CaCO3 had an inhibitory effect on cell death, while PVA/Col@CaCO3 improved the apoptosis rate of BMSCs (P < 0.05).
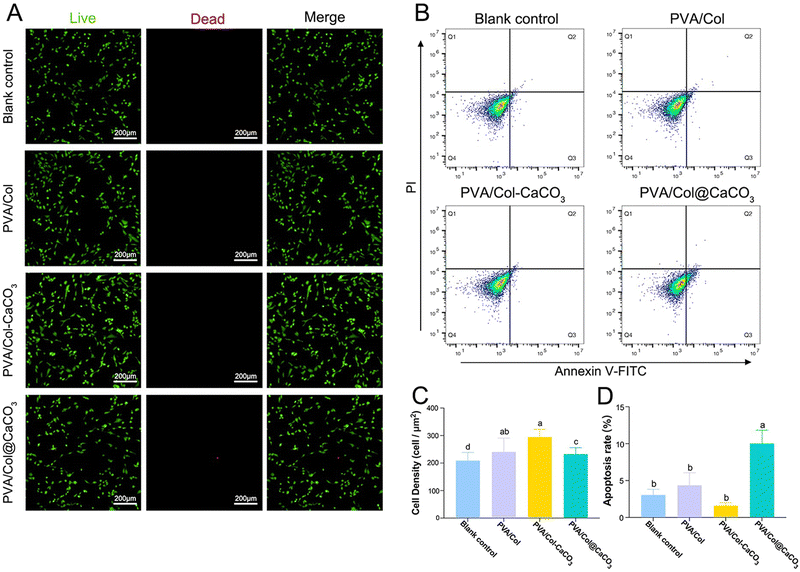 |
| Fig. 7 Evaluation of cell apoptosis in vitro. (A) Representative live/dead staining fluorescent images of BMSCs cultured with various samples for 1 day. Live and dead cells were marked with green and red fluorescence, respectively. (B) Flow cytometry of BMSCs cultured with various samples for 1 day. (C) Quantitative analysis of cell density characterized by live/dead cell staining from each group on day 1. (D) Quantitative analysis of the apoptosis rate characterized by flow cytometry from each group on day 1. Data are expressed as mean values ± standard deviations. Groups labeled with the same superscript letters are not significantly different (P > 0.05). | |
3.5 Osteogenic differentiation
We further investigated the osteogenic potential of the intrafibrillarly mineralized films, extrafibrillarly mineralized films, and pure films, in comparison to the blank control group. Alkaline phosphatase is an early-stage biomarker of osteogenesis, the increased expression of which indicates enhanced osteogenic differentiation.37 To assess this, ALP staining and both semi-quantitative and quantitative analysis were performed after osteogenic induction for 7 and 14 days. ALP staining showed that the BMSCs cultured with PVA/Col–CaCO3 films exhibited the strongest dark purple or blue color at both time points (Fig. 8(A)). The quantitative results of ALP activity and the stained regions confirmed that the PVA/Col–CaCO3 group stimulated the highest ALP expression level among all the groups (Fig. 8(B), P < 0.05). In addition, osteopontin, which accumulates in the mineralized bone matrix during osteogenesis, was evaluated through immunofluorescence staining on days 7 and 14 (Fig. 9(A) and (B)). OPN plays a crucial role in promoting osteoblast differentiation and enhancing ALP activity.38 Our results indicated a significant increase in OPN expression in the PVA/Col–CaCO3 group, with an improved intensity and distribution of red fluorescent signals in the cell matrix (Fig. 9(C) and (D), P < 0.05).
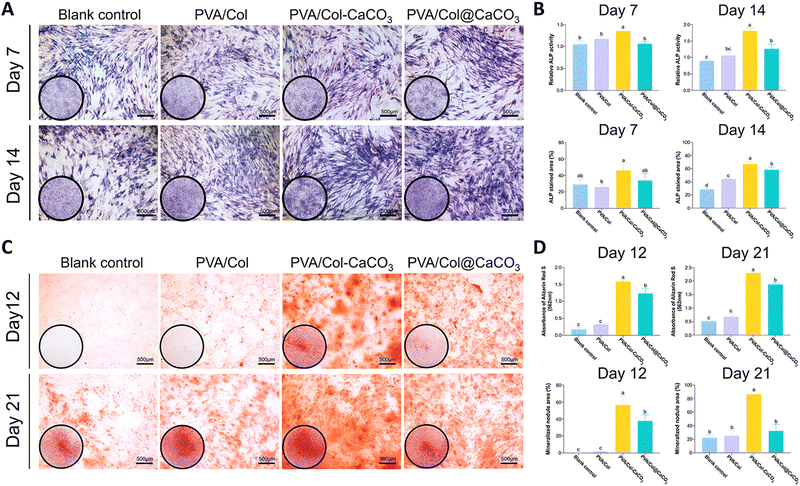 |
| Fig. 8 ALP and Alizarin Red S staining of BMSCs after osteogenic differentiation. (A) Representative ALP staining images of BMSCs cultured with different materials for 7 and 14 days. (B) Quantification of the ALP activity and the percentage of ALP-stained area of BMSCs cultured with different materials for 7 and 14 days. (C) Representative Alizarin Red S staining images of BMSCs cultured with different materials for 12 and 21 days. (D) OD 562 nm of the Alizarin Red S staining and the percentage of ARS-stained area of BMSCs cultured with different materials for 12 and 21 days. Data are expressed as mean values ± standard deviations. Groups labeled with the same superscript letters are not significantly different (P > 0.05). | |
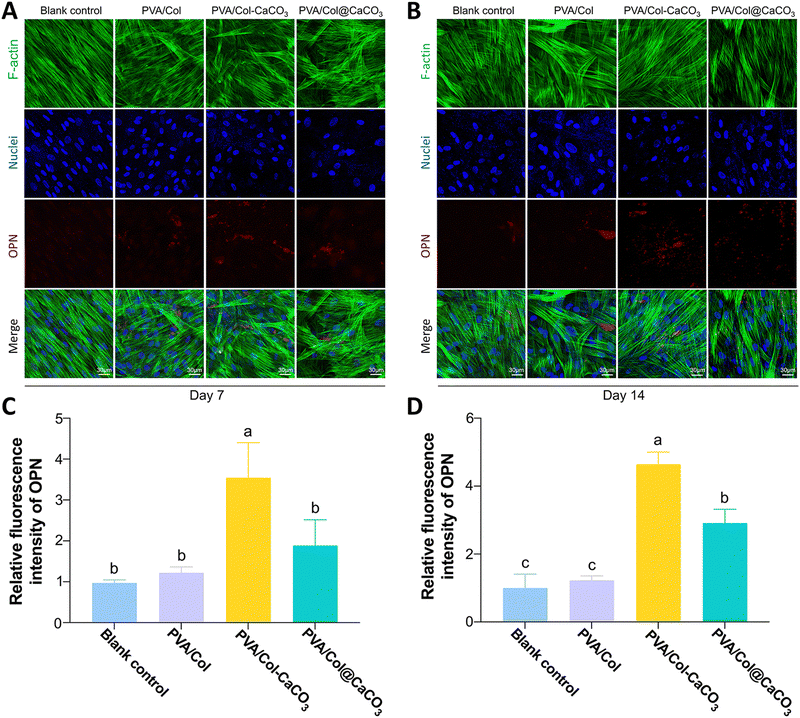 |
| Fig. 9 Immunofluorescence images and quantification of OPN in BMSCs cultured with different materials for 7 and 14 days. (A) Representative confocal immunofluorescence images of BMSCs cultured with various materials for 7 days. (B) Representative confocal immunofluorescence images of BMSCs cultured with various materials for 14 days. (C) Quantitative statistics of OPN of BMSCs after 7 days’ incubation. (D) Quantitative statistics of OPN of BMSCs after 14 days’ incubation. Data are expressed as mean values ± standard deviations. Groups labeled with the same superscript letters are not significantly different (P > 0.05). | |
The formation of mineralized nodules is a hallmark of osteoblast maturation and bone matrix formation.39 Alizarin Red S staining was conducted on days 12 and 21. ARS could chelate calcium salts and form a distinctive red-orange complex that serves as an indicator of osteogenic differentiation. Both the staining and quantitative analysis showed that the intrafibrillarly mineralized films displayed intensely red-stained areas, signifying the extensive deposition of calcium salts (Fig. 8(C) and (D), P < 0.05). Notably, on day 21, the PVA/Col–CaCO3 group exhibited the largest areas of red-stained calcium nodules, confirming the highest degree of mineralization and successful osteogenic differentiation (Fig. 8(D)).
Excellent biocompatibility, clinical management capabilities, and spatial manufacturing qualities are among the key characteristics required of bone-regeneration materials.40,41 Two main factors may explain the best BMSCs proliferation and osteogenic potential of the PVA/Col–CaCO3 group. First, based on the PILP theory, amorphous precursors were introduced to fabricate intrafibrillarly mineralized bone-healing bioactive films.21 Such kinds of materials are considered to promote cellular behaviors and tissue regeneration owing to their bone-like physical properties, biomimetic surface nanomorphology, and so on.36,42,43
In addition, the topical chemical signals may also hold the key to success. As reported, the dissolution and hydrolysis of calcium carbonate crystals in the microenvironment can release Ca2+ ions and increase the environmental pH. Previous work indicated that an alkaline microenvironment may help to stimulate the activity of osteogenic cells and facilitate osteogenesis.26 What's more, evidence suggests that calcium ions play a role in the proliferation and differentiation of diverse cells related to the osteogenesis process through activating specific pathways, such as the Wnt/β-catenin signaling pathway.44,45 Our present work has shown that the intrafibrillar aragonite crystals displayed a higher solubility rate than the extrafibrillar calcite crystals, which may also explain the better BMSCs proliferation and osteogenic differentiation induced by the dissolved calcium ions. Besides, earlier studies have confirmed the bioactivity of PVA on stem cell differentiation behaviors, and our study was consistent with this.46,47
In recent years, intrafibrillarly mineralized materials have been considered to have the capacity to affect stem cell migration, promoting osteogenesis, angiogenesis, and boosting the release of growth factors.48 However, previous work has mainly focused on apatite- or silicon-based scaffolds.49,50 There is scant research about the application of calcium–carbonate-mineralized collagen films on cell behaviors and bone-tissue engineering. Our experiment results confirmed that intrafibrillarly mineralized collagen films with aragonite crystals have functions for inhibiting apoptosis and facilitating osteogenic differentiation, revealing their enormous potentials in hard tissue regeneration.
Nevertheless, the application of collagen films with extrafibrillar CaCO3 deposition may lead to opposite outcomes, as revealed in the present study. SEM images revealed that (Fig. 2(C)) CaCO3 nanocrystals were bound loosely on PVA/Col networks, which may be prone to detach from the films, deposit on cell adhesion surface and intensely contact with cells, thus blocking the extension of cell antenna. Meanwhile, the PVA/Col@CaCO3 films induced osteogenic differentiation to some extent, which may be related to the dissolution of calcium ions from the materials. Similarly, specific and complex impacts have been discovered when much nanomaterials, such as hydroxyapatites, contact directly with cells.51–53 Our future research aims to focus on elucidating the various signaling pathways to explore the complicated mechanism how intrafibrillarly mineralized and extrafibrillarly mineralized CaCO3 materials influence osteogenic differentiation.
4. Conclusions
In summary, this work provides a novel method for simulating a natural hierarchical structure. PVA/Col–CaCO3, resembling a bone-like hierarchical structure, was successfully synthesized and utilized as a biofilm for bone repair. The aragonite crystal form was successfully fabricated through the intrafibrillar mineralization of PVA/Col film, and contained oriented aragonite nanocrystals that enhanced the mechanical properties. The hardness of the PVA/Col–CaCO3 film could reach 211.6 ± 0.1 MPa, with a Young's modulus of 5.6 ± 1.7 GPa. Also, the tensile strength of PVA/Col–CaCO3 was 9.6 MPa, demonstrating excellent mechanical properties. However, the use of PVA/Col@CaCO3 (extrafibrillar mineralization) resulted in surface roughness, causing an uneven hardness and Young's modulus distribution. Therefore, intrafibrillar mineralization plays an important role in improving the mechanical properties of the electrospun films. It could realize the application of pre-stressing in natural collagen-based composites. Additionally, the PVA/Col–CaCO3 film provided an effective stimulation of the micromorphology and chemical signals for BMSCs growth, showing the potential for promoting osteogenic differentiation. Furthermore, the in vitro osteogenic differentiation demonstrated that the PVA/Col–CaCO3 film significantly induced the osteogenesis of BMSCs. The detailed signaling pathways and the in vivo performance require further validation. Consequently, PVA/Col–CaCO3 is regarded as a biomaterial with excellent physical and chemical properties, along with favorable biocompatibility and osteoinductive activity, thereby holding great promise in bone-repair applications.
Author contributions
Yin Liu and Xin Gao: conceptualization, methodology, investigation, formal analysis, data curation, visualization, writing – original draft preparation. Yuqi Li: formal analysis, data curation. Anqi Gao: investigation. Zhuozhi Zheng: investigation. Jingjiang Wei: investigation. Hongye Yang: investigation, resources. Hao Xie: investigation, resources. Hao Wang: investigation, resources. Weimin Wang: investigation, resources. Hang Ping: conceptualization, writing – reviewing and editing, supervision, funding acquisition. Zhengyi Fu: conceptualization, supervision, funding acquisition, project administration.
Data availability
The authors confirm that the data supporting the findings of this article are available within the article and in its ESI.†
Conflicts of interest
There are no conflicts to declare.
Acknowledgements
The authors thank Dr Liang Bin (Core Facility, Center of Biomedical Analysis, Tsinghua University) for technical support with confocal laser scanning microscopy. This work has been supported by the National key Research and Development Program of China (2021YFA0715700), by the National Natural Science Foundation of China (52422204, 52372091, 52402114), by the Guangdong Basic and Applied Basic Research Foundation (2024A1515030097), by the Hubei Provincial Nature Science Foundation of China (2024AFA002), by the Open Fund of Hubei Longzhong Laboratory (2022ZZ-12).
References
- Y. M. Kolambkar, J. D. Boerckel and K. M. Dupont,
et al., Spatiotemporal delivery of bone morphogenetic protein enhances functional repair of segmental bone defects, Bone, 2011, 49(3), 485–492 CrossRef CAS.
- G. L. Koons, M. Diba and A. G. Mikos, Materials design for bone-tissue engineering, Nat. Rev. Mater., 2020, 5(8), 584–603 CrossRef CAS.
- Y. Du, J. L. Guo, J. Wang, A. G. Mikos and S. Zhang, Hierarchically designed bone scaffolds: From internal cues to external stimuli, Biomaterials, 2019, 218, 119334 CrossRef CAS PubMed.
- C. Wang, W. Huang and Y. Zhou,
et al., 3d printing of bone tissue engineering scaffolds, Bioact. Mater., 2020, 5(1), 82–91 Search PubMed.
- S. Bose, M. Roy and A. Bandyopadhyay, Recent advances in bone tissue engineering scaffolds, Trends Biotechnol., 2012, 30(10), 546–554 CrossRef CAS PubMed.
- R. Dimitriou, G. I. Mataliotakis, G. M. Calori and P. V. Giannoudis, The role of barrier membranes for guided bone regeneration and restoration of large bone defects: Current experimental and clinical evidence, BMC Med., 2012, 10(1), 81 CrossRef PubMed.
- H. J. Zhang, W. P. Ma and H. S. Ma,
et al., Spindle-like zinc silicate nanoparticles accelerating innervated and vascularized skin burn wound healing, Adv. Healthcare Mater., 2022, 11(10), 2102359 CrossRef CAS.
- Y.-X. Ma, K. Jiao and Q.-Q. Wan,
et al., Silicified collagen scaffold induces semaphorin 3a secretion by sensory nerves to improve in situ bone regeneration, Bioact. Mater., 2022, 9, 475–490 CAS.
- Q. Wang, J. Xu and H. Jin,
et al., Artificial periosteum in bone defect repair-a review, Chin. Chem. Lett., 2017, 28(9), 1801–1807 CrossRef CAS.
- Y. Zhang, W.-L. Gao and Z.-Y. Liu,
et al., Mineralization and osteoblast behavior of multilayered films on tio2 nanotube surfaces assembled by the layer-by-layer technique, Chin. Chem. Lett., 2016, 27(7), 1091–1096 CrossRef CAS.
- J. Ye, X. Shi and X. Y. Chen,
et al., Chitosan-modified, collagen-based biomimetic nanofibrous membranes as selective cell adhering wound dressings in the treatment of chemically burned corneas, J. Mater. Chem. B, 2014, 2(27), 4226–4236 RSC.
- A. Baradaran-Rafii, E. Biazar and S. Heidari-Keshel, Cellular response of limbal stem cells on polycaprolactone nanofibrous scaffolds for ocular epithelial regeneration, Curr. Eye Res., 2016, 41(3), 326–333 CAS.
- X. Yu, M. Gholipourmalekabadi and X. Wang, er al. Three-dimensional bioprinting biphasic multicellular living scaffold facilitates osteochondral defect regeneration, Interdiscip. Mater., 2024, 3(5), 738–756 Search PubMed.
- Y. Zhu, F. Song and L. H. Yanyun Ju,
et al., Erratum: Nac-loaded electrospun scaffolding system with dual compartments for the osteogenesis of rbmscs in vitro erratum, Int. J. Nanomed., 2019, 14, 1705 CrossRef.
- C. Tang, C. D. Saquing, J. R. Harding and S. A. Khan,
In situ cross-linking of electrospun poly(vinyl alcohol) nanofibers, Macromolecules, 2010, 43(2), 630–637 CrossRef CAS.
- J. Xie, H. Ping and T. Tan,
et al., Bioprocess-inspired fabrication of materials with new structures and functions, Prog. Mater. Sci., 2019, 105, 100571 CrossRef.
- Q. Wang, L. Hu and R. Tang,
et al., Expanding from materials to biology inspired by biomineralization, Interdiscip. Mater., 2024, 3(2), 165–188 CAS.
- L. Li and Q. Cheng, Anisotropic thermally conductive films based on two-dimensional nanomaterials, Interdiscip. Mater., 2024, 3(6) DOI:10.1002/idm2.12204.
- H. Ping, W. Wagermaier and N. Horbelt,
et al., Mineralization generates megapascal contractile stresses in collagen fibrils, Science, 2022, 376(6589), 188 CrossRef CAS PubMed.
- C.-y Wang, K. Jiao and J.-f Yan,
et al., Biological and synthetic template-directed syntheses of mineralized hybrid and inorganic materials, Prog.
Mater. Sci., 2021, 116 Search PubMed.
- J. Li, J.-F. Yan and Q.-Q. Wan,
et al., Matrix stiffening by self-mineralizable guided bone regeneration, Acta Biomater., 2021, 125, 112–125 CrossRef CAS PubMed.
- K. Chatzipanagis, C. G. Baumann and M. Sandri,
et al., In situ mechanical and molecular investigations of collagen/apatite biomimetic composites combining Raman spectroscopy and stress-strain analysis, Acta Biomater., 2016, 46, 278–285 CrossRef CAS PubMed.
- F. Ren, X. Wan, Z. Ma and J. Su, Study on microstructure and thermodynamics of nacre in mussel shell, Mater. Chem. Phys., 2009, 114(1), 367–370 CrossRef CAS.
- E. Lopez, B. Vidal and S. Berland,
et al., Demonstration of the capacity of nacre to induce bone formation by human osteoblasts maintained in vitro, Tissue Cell, 1992, 24(5), 667–679 CrossRef CAS PubMed.
- Y. Xu, F. Nudelman and E. D. Eren,
et al., Intermolecular channels direct crystal orientation in mineralized collagen, Nat. Commun., 2020, 11(1), 5068 CrossRef CAS PubMed.
- Q. Huang, Y. Liu, Z. Ouyang and Q. Feng, Comparing the regeneration potential between plla/aragonite and plla/vaterite pearl composite scaffolds in rabbit radius segmental bone defects, Bioact. Mater., 2020, 5(4), 980–989 Search PubMed.
- Z. Zou, W. J. E. M. Habraken and G. Matveeva,
et al., A hydrated crystalline calcium carbonate phase: Calcium carbonate hemihydrate, Science, 2019, 363(6425), 396 CrossRef CAS PubMed.
- L. B. Gower, Biomimetic model systems for investigating the amorphous precursor pathway and its role in biomineralization, Chem. Rev., 2008, 108(11), 4551–4627 CrossRef CAS PubMed.
- H. Ping, H. Xie and Y. Wan,
et al., Confinement controlled mineralization of calcium carbonate within collagen fibrils, J. Mater. Chem. B, 2016, 4(5), 880–886 RSC.
- M. J. Olszta, X. Cheng and S. S. Jee,
et al., Bone structure and formation: A new perspective, Mater. Sci. Eng., R, 2007, 58(3–5), 77–116 CrossRef.
- L.-n Niu, S. E. Jee and K. Jiao,
et al., Collagen intrafibrillar mineralization as a result of the balance between osmotic equilibrium and electroneutrality, Nat. Mater., 2017, 16(3), 370–378 CrossRef CAS PubMed.
- W. Chen, K. Liu and X. Liao,
et al., Harmonizing thickness and permeability in bone tissue engineering: A novel silk fibroin membrane inspired by spider silk dynamics, Adv. Mater., 2024, 36(13), 2310697 CrossRef CAS.
- J. Y. Rho, T. Y. Tsui and G. M. Pharr, Elastic properties of human cortical and trabecular lamellar bone measured by nanoindentation, Biomaterials, 1997, 18(20), 1325–1330 CrossRef CAS.
- T. Lu, J. Wen and S. Qian,
et al., Enhanced osteointegration on tantalum-implanted polyetheretherketone surface with bone-like elastic modulus, Biomaterials, 2015, 51, 173–183 CrossRef CAS PubMed.
- J.-I. Sasaki, G. L. Abe and A. Li,
et al., Barrier membranes for tissue regeneration in dentistry, Biomater. Invest. Dent., 2021, 8(1), 54–63 CAS.
- X. Wu, W. Peng and G. Liu,
et al., Extrafibrillarly demineralized dentin matrix for bone regeneration, Adv. Healthcare Mater., 2023, 12(12), e2202611 CrossRef.
- N. Amiryaghoubi, M. Fathi and N. N. Pesyan,
et al., Bioactive polymeric scaffolds for osteogenic repair and bone regenerative medicine, Med. Res. Rev., 2020, 40(5), 1833–1870 CrossRef CAS PubMed.
- L. Chen, K. Shi and N. Ditzel,
et al., Kiaa1199 deficiency enhances skeletal stem cell differentiation to osteoblasts and promotes bone regeneration, Nat. Commun., 2023, 14(1), 2016 CrossRef CAS PubMed.
- X. Feng, C. Wang and B. Ji,
et al., Cd_99 g1 neutrophils modulate osteogenic differentiation of mesenchymal stem cells in the pathological process of ankylosing spondylitis, Ann. Rheum. Dis., 2024, 83(3), 324–334 CrossRef PubMed.
- J. Wang, Y. Qu and C. Chen,
et al., Fabrication of collagen membranes with different intrafibrillar mineralization degree as a potential use for gbr, Mater.
Sci. Eng., C, 2019, 104, 109959 CrossRef CAS PubMed.
- Y. H. Yang, T. P. Xu, H. P. Bei, Y. J. Zhao and X. Zhao, Sculpting bio-inspired surface textures: An adhesive janus periosteum, Adv. Funct. Mater., 2021, 31, 37 Search PubMed.
- H. Liu, Y. Shi and Y. Zhu,
et al., Bioinspired piezoelectric periosteum to augment bone regeneration via synergistic immunomodulation and osteogenesis, ACS Appl. Mater. Interfaces, 2023, 15(9), 12273–12293 CrossRef CAS PubMed.
- W. Fang, H. Ping and Y. Huang,
et al., Growth of mineralized collagen films by oriented calcium fluoride nanocrystal assembly with enhanced cell proliferation, J. Mater. Chem. B, 2021, 9(33), 6668–6677 RSC.
- W. Liu, C. C. Le and D. Wang,
et al., Ca(2+)/cam/camk signaling is involved in cadmium-induced osteoclast differentiation, Toxicology, 2020, 441, 152520 CrossRef CAS PubMed.
- A. P. Kusumbe, S. K. Ramasamy and R. H. Adams, Coupling of angiogenesis and osteogenesis by a specific vessel subtype in bone, Nature, 2014, 507(7492), 323–328 CrossRef CAS.
- S. H. Oh, D. B. An, T. H. Kim and J. H. Lee, Wide-range stiffness gradient pva/ha hydrogel to investigate stem cell differentiation behavior, Acta Biomater., 2016, 35, 23–31 CrossRef CAS PubMed.
- W. Liang, W. He and R. Huang,
et al., Peritoneum-inspired janus porous hydrogel with anti-deformation, anti-adhesion, and pro-healing characteristics for abdominal wall defect treatment, Adv. Mater., 2022, 34(15), e2108992 CrossRef.
- A. Ressler, M. Antunović and L. Teruel-Biosca,
et al., Osteogenic differentiation of human mesenchymal stem cells on substituted calcium phosphate/chitosan composite scaffold, Carbohydr. Polym., 2022, 277, 118883 CrossRef CAS.
- H. Wang, J. Tian and Y. Jiang,
et al., A 3d biomimetic optoelectronic scaffold repairs cranial defects, Sci. Adv., 2023, 9(7), eabq7750 CrossRef CAS.
- J. Zheng, N. Rahman and L. Li,
et al., Biofunctionalization of electrospun fiber membranes by lbl-collagen/chondroitin sulfate nanocoating followed by mineralization for bone regeneration, Mater. Sci. Eng., C, 2021, 128, 112295 CrossRef CAS.
- M. C. Matesanz, M. J. Feito and M. Oñaderra,
et al., Early in vitro response of macrophages and t lymphocytes to nanocrystalline hydroxyapatites, J. Colloid Interface Sci., 2014, 416, 59–66 CrossRef CAS PubMed.
- C. Y. Tay, W. Fang and M. I. Setyawati,
et al., Nano-hydroxyapatite and nano-titanium dioxide exhibit different subcellular distribution and apoptotic profile in human oral epithelium, ACS Appl. Mater. Interfaces, 2014, 6(9), 6248–6256 CrossRef CAS PubMed.
- R. Shu, R. McMullen, M. J. Baumann and L. R. McCabe, Hydroxyapatite accelerates differentiation and suppresses growth of mc3t3-e1 osteoblasts, J. Biomed. Mater. Res., Part A, 2003, 67(4), 1196–1204 CrossRef CAS PubMed.
|
This journal is © The Royal Society of Chemistry 2025 |
Click here to see how this site uses Cookies. View our privacy policy here.