DOI:
10.1039/D4TB01782J
(Review Article)
J. Mater. Chem. B, 2025,
13, 103-116
Experimental and computational techniques to investigate the protein resistance of zwitterionic polymers
Received
9th August 2024
, Accepted 11th October 2024
First published on 14th November 2024
Abstract
Most surfaces undergo non-specific protein adsorption upon direct contact with protein-containing environments, resulting in the formation of a protein corona, and the nature and composition of the corona affect the properties of the material. Zwitterionic polymers have oppositely charged groups in their repeating units, which facilitate the formation of a hydration layer on the surface through electrostatic interactions. The hydration layer possesses a strong water-binding ability and can prevent protein adsorption. Therefore, the hydration effect of zwitterionic polymers has become a research focus, and many researchers have investigated this mechanism using experimental and computational methods. This paper reviews the experimental techniques and simulation methods to study the hydration effect of zwitterionic polymers and the advantages and disadvantages of different techniques are discussed.
1. Introduction
Proteins are macromolecules composed of aggregated amino acids, and they are abundant in various biological systems. Non-specific protein adsorption occurs on almost all surfaces of materials that come into direct contact with protein-containing surroundings, leading to the formation of a protein corona. The nature and content of the protein corona affect the destiny, absorption, and performance of nanoparticles (NPs) in biological settings.1 Protein adsorption is a rapid and dynamic process,2 primarily driven by hydrophobic–hydrophobic and electrostatic interactions.3 Upon adsorption, proteins reorganize their structures, aligning favorably for surface interactions, and the process can displace surface-bound water molecules and enhance system entropy. This dehydration-induced entropy gain characterizes the fundamental mechanism of protein adsorption.4 However, this prevalent phenomenon is sometimes unfavorable in biomedical and industrial applications, where the adsorption of biomolecules and the adhesion of cells to biomaterials may lead to functionality loss and innate complications, such as foreign body reaction, inflammation, and rapid clearance.5 Biofouling on medical devices, implants, and warships can severely affect their performance and cause numerous problems, such as tissue fibrosis, bacterial infections, and inefficient fuel consumption.6 Since protein adsorption is a critical initial step in many biofouling events, researchers aim to mitigate the problems associated with protein adsorption, thereby improving the performance and safety of biomedical devices and industrial systems.4
Many hydrophilic surfaces can reduce non-specific protein adsorption, with zwitterionic polymers being one such example.7 Zwitterionic polymers possess opposite charge groups in their repeating units. Equal amounts of positive and negative charge groups are uniformly distributed at the molecular level, ensuring an overall charge balance.4 The positively and negatively charged groups in zwitterionic polymers can form a hydrated layer on the surface through electrostatic interactions. The hydrated layer tends to bind with water, thus effectively resisting the adsorption of proteins from plasma.8 Unlike poly(ethylene glycol) (PEGs) and PEG-like materials, which bind water through hydrogen bonding, zwitterionic polymers retain water mainly through ionic dissolution. Charge neutrality and high hydrophilicity are the two main characteristics of these polymers.9 Despite some other potential mechanisms, the strong hydration effect of zwitterionic polymers is currently considered the main factor determining their resistance to protein adsorption. As a new generation of antifouling materials, zwitterionic polymers have attracted increasing attention in the past decades for biomedical applications.4 Currently, research on zwitterionic polymers is focused on the synthesis of novel zwitterionic materials, their performance optimization, and the expansion of their application fields. The unique properties of zwitterionic polymers stem from their “zwitterionic” nature, which makes them super hydrophilic. Moreover, minor modifications in the molecular structure profoundly affect the properties and applications of zwitterionic polymers. Variations in the physicochemical properties of the backbone and linker can also modulate the flexibility and mechanics of the material. The innate protein resistance of zwitterionic materials makes them promising materials for reducing protein adsorption.10
Various techniques have been employed to explore the exceptional resistance of zwitterionic polymers to protein adsorption. These techniques measure the changes in chemical and physical properties that directly or indirectly reflect the interactions between water, materials, and proteins, attempting to elucidate the mechanism of hydration effects. In tandem with experimental studies, numerous computational investigations have been conducted at the electronic, atomic, and coarse-grained resolution levels, offering insights into the interactions, dynamics, and structural alterations of proteins, surfaces, water, and ions in diverse amphiphilic material–protein systems.
This review aims to provide a comprehensive overview of the techniques used to study the resistance of zwitterionic polymers to protein adsorption. First, the tools to characterize zwitterionic polymers are discussed, such as (1) sum-frequency generation (SFG) vibrational spectroscopy to study the real-time interactions between proteins, water, and materials at the molecular level, (2) a contact angle method to assess the wettability of the surfaces of zwitterionic polymers, (3) X-ray photoelectron spectroscopy (XPS) to qualitatively and quantitatively determine the chemical states of the surface region of a material and discriminate between different types of surface chemical bonds, (4) atomic force microscopy (AFM) to visualize topographical images and show isostatic surfaces, (5) differential scanning calorimetry (DSC) to easily analyze the hydration state, (6) enzyme-linked immunosorbent assays (ELISA) to assess the differences in protein fouling on the surfaces of different materials, and (7) surface plasmon resonance (SPR) technology for the quantitative analysis of protein adsorption on material surfaces.11 Regarding computational methods, molecular dynamics (MD) simulations save time and effort to explore the interactions, structure, and dynamics of proteins, surfaces, water, ions, and other components under different conditions; Monte Carlo (MC) simulations can determine the optimal orientation of proteins during adsorption on surfaces, and molecular docking is used to rapidly screen the most favorable adsorption combinations of proteins on a large scale. The integration of different methods can leverage their unique strengths and compensate for individual limitations, enabling a comprehensive and efficient analysis of the properties of zwitterionic materials.
2. Experimental techniques
2.1. SFG vibrational spectroscopy
SFG vibrational spectroscopy can provide in situ information on a material surface.12 An SFG process involves two input photons and one outgoing photon. The entire frequency range of the input photons is reflected by the outgoing photons. Therefore, a second-order nonlinear optical process exists between the SFG signal and the two input beams (Fig. 1(a)).13 This technique is a potent tool for studying the in situ and real-time interactions between water, proteins, and materials because it can analyze the water structure on the surfaces of different materials at a molecular level.14 Although this technology is sensitive to surfaces, it may be subject to interference from other factors because of the specific excitation and detection conditions. Kondo et al. investigated the water structure in the vicinity of the thin films of zwitterionic materials using SFG vibrational spectroscopy.15 Their study showed that water molecules near the surface of zwitterionic polymers were less oriented than those in other materials. Therefore, the water layer was unlikely to be repelled by the zwitterionic material, implying a strong repulsion for non-specific protein adsorption.10 Leng et al. evaluated the surface hydration of sulfobetaine methacrylate (SBMA) and oligo ethylene glycol methacrylate (OEGMA) polymer brushes (referred to as pSBMA and pOEGMA, respectively) using SFG vibrational spectroscopy, both in situ and real-time, to probe the structural changes of water in contact with the protein solution (Fig. 1(b)). Most of the ordered water molecules were bound by strong hydrogen bonding at both pSBMA and pOEGMA surfaces, whereas some water molecules were bound by weak hydrogen bonding at the pOEGMA surface (Fig. 1(c)). The effects of free sulfobetaine (SB) and PEG on the hydration layer of protein molecules were also explored. These characterizations reveal that free SB molecules reinforce the protein hydration layer. In contrast, free PEG chains significantly disrupt the hydration layer and may interact directly with the protein surface. This result suggests that ionic solvation-induced hydration is greater than hydrogen-bond-induced hydration, which contributes to the excellent antifouling properties of zwitterionic polymers. Subsequently, this team assessed the hydration layers of antifouling materials and discovered that strong hydrogen bonding was essential for the development of a robust hydration layer as it prevented proteins from scaling at the polymer interface.13 The hydration formed by the zwitterionic polymer is stronger than that of PEG because electrostatic attraction enhances hydrogen bonding between water molecules and hydrophilic charged groups in the zwitterionic polymer. Using SFG, Sarker et al. found that the surface hydration and salt resistance of zwitterions may be affected by the length of the positive and negative charge spacing in the material.16 A shorter charge spacing reduces the dipole moment of zwitterions with near-neutral intrinsic net charges, weakens the electrostatic and dipole–dipole interactions with biofouling, and improves fouling resistance. Trimethylamine-N-oxide (TMAO) is a more effective antifouling material than carboxy betaine compounds because of its shorter zwitterionic spacing, stronger hydrogen bonding, less net charge, and smaller dipole moment. The stronger surface hydration observed on the polymeric trimethylamine-N-oxide (pTMAO) was also related to the shorter charge spacing, which counteracted the effect of salt on hydration reduction (Fig. 1(d)).
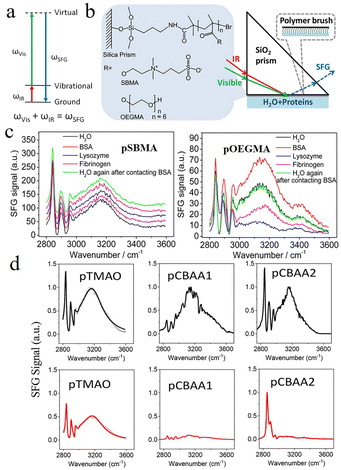 |
| Fig. 1 Exploration of hydration phenomena on zwitterionic interfaces through SFG vibrational spectroscopic analysis. (a) SFG energy level diagram.13 Reproduced from reference molecular level studies on interfacial hydration of zwitterionic and other antifouling polymers in situ with permission from Elsevier, copyright 2016. (b) Schematic illustration of SFG measurement and chemical structures of polymers.14,17 Reproduced from reference probing the surface hydration of nonfouling zwitterionic and poly(ethylene glycol) materials with isotopic dilution spectroscopy with permission from American Chemical Society, copyright 2015. (c) SFG spectra of pSBMA and pOEGMA in contact with water and protein solutions.17 Reproduced from reference probing the surface hydration of nonfouling zwitterionic and PEG materials in contact with proteins with permission from American Chemical Society, copyright 2015. (d) SFG spectra collected from zwitterionic polymers prepared based on TMAO, CBAA1 (carboxy betaine acrylamide (CBAA), –(CH2)n–, n = 1), and CBAA2 (–(CH2)n–, n = 2) in contact with water (colored black) and 0.5 M NaCl solution (colored red).16 Reproduced from reference hydration behaviors of non-fouling zwitterionic materials with permission from The Royal Society of Chemistry, copyright 2023. | |
2.2. Contact angle measurement
A contact angle is an angle formed at the interface between water, air, and a solid, and its value indicates the degree of surface wettability.18 A surface with a low contact angle suggests that water diffuses and attaches to the surface, whereas a surface with a high contact angle tends to repel water.19 Contact angle measurement applies to a wide range of material surfaces and is a straightforward method to assess wettability. However, the measured contact angle may be affected by a variety of factors, such as surface inhomogeneity, temperature, and pressure. Zhang et al. characterized the surface properties of a zwitterionic peptide (P(CB-EGxGlu), x = 1–3) with an oligo-glycol (EG) linker in the side chain utilizing a static water contact angle (SCA) analyzer. After the adsorption of zwitterionic peptides, the SCA of the gold surface decreased significantly, and a higher x value further decreased the SCA (Fig. 2(a)).6 P(CB-EG3Glu) has three times more EG repeating units per residue than P(CB-EG1Glu), which compensates for the reduced graft density and enhances hydration. This indicates that the antifouling properties can be improved by hydrogen-bond-induced hydration (EGx) combined with ionolysis. However, more caution is needed when considering longer EGx linkers because they can slightly decrease the graft density. When using CH2I2 as a probe liquid for contact angle measurements in water, a larger contact angle indicates a more hydrophilic surface. Mukai et al. found through contact angle measurements that poly(ether ketone) (PEEK) substrates grafted with poly(2-methacryloyloxyethyl choline methylphosphonate) (PMCPm) and poly(2-methacryloyloxyethyl phosphorylcholine) (PMPC) brushes exhibited higher hydrophilicity than bare PEEK substrates (Fig. 2(b)). The similar contact angles of PMCPm and PMPC brushes suggest their comparable hydrophilicity.20 Furthermore, Mihara et al. also reported that PMPC was more hydrophilic than its PMCP analogs, indicating that the substitution of alkoxy groups with methyl groups enhanced the hydrophilicity of hydrolysis-resistant quasi-CP polymer.21 Hladysh et al. assessed the hydrophilicity of L-lysine grafted α,β-poly[(2-propyne)-DL-aspartamide-co-(2-hydroxyethyl)-DL-aspartamide-co-(2-(4-hydroxyphenyl)ethyl)-DL-aspartamide] (Lys-P-HE-TyrAA) and Na-L-lysine grafted α,β-poly[(2-propyne)-DL-aspartamide-co-(2-hydroxyethyl)-DL-aspartamide-co-(2-(4-hydroxyphenyl)ethyl)-DL-aspartamide] (Na-Lys-P-HE-TyrAA) polyaspartic amides by contact angle studies.18 After polymer coating, the contact angle decreased with the introduction of zwitterionic groups, indicating that the zwitterionic polyaspartic amides formed a hydrophilic surface (Fig. 2(c)). Zhang et al. synthesized latex particles with zwitterionic poly-3-[dimethyl-[2-(2methylprop-2-enoyloxy)ethyl]azaniumyl]propane-1-sulfonate (PDMAPS) as the shell. The surface hydrophilicity of the latex film increased after water treatment, which was attributed to the migration of embedded hydrophilic PDMAPS blocks and the formation of extended polymer brushes on the top surfaces, which decreased the water contact angle.22
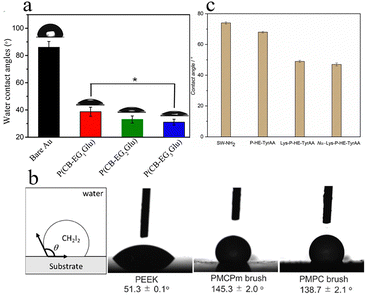 |
| Fig. 2 Measurement of contact angles for different polymers. (a) SCAs of all gold surfaces before and after modification with zwitterionic polypeptides P(CB-EGxGlu).6 Reproduced from reference investigation on the linker length of synthetic zwitterionic polypeptides for improved nonfouling surfaces with permission from American Chemical Society, copyright 2018. (b) Schematic illustration of the contact angle measurement in water using CH2I2 as a liquid probe; side-view images of CH2I2 droplets on PEEK substrates grafted with different polymer brushes.20 Reproduced from reference synthesis and hydration behavior of a hydrolysis-resistant quasi-choline phosphate zwitterionic polymer with permission from American Chemical Society, copyright 2020. (c) Contact angles of amine-functionalized silicon wafer (SW-NH2), Lys-PHE-TyrAA, Nα-Lys-P-HE-TyrAA and P-HE-TyrAA polyaspartamide-coated silicon wafers.18 Reproduced from reference zwitterionic polyaspartamides based on L-lysine side-chain moieties: synthesis, nonfouling properties and direct/indirect nanogel preparation with permission from Elsevier, copyright 2021. | |
2.3. XPS
XPS is a very powerful analytical technique for qualitative and quantitative determination of the chemical state of a surface region.23 It enables highly sensitive composition analysis by irradiating the surface with X-rays and measuring emitted electron kinetic energy from the top 1–10 nm on a sample.24 However, XPS data are collected from the topmost surface layer and can be influenced by surface impurities, oxidation, etc.25 Jiang et al. used XPS to detect the signals of carbon, nitrogen, oxygen, and silicon on the surface of polyurethane films (Fig. 3(a)).26 XPS data showed the presence of hydrophilic zwitterionic groups on the surface of polyurethane films. Mukai et al. employed XPS to confirm the presence of nitrogen and phosphorus on the (PMCPm)-grafted substrate (Fig. 3(b)), whereas these elements were absent from the bare PEEK substrate (Fig. 3(c)).20 Combined with the scanning electron microscope (SEM) results, the surface of the PMPC-grafted PEEK and PMCPm-grafted PEEK was smoother than that of bare PEEK substrate, indicating that the polymer brushes formed a uniform coating on PEEK.
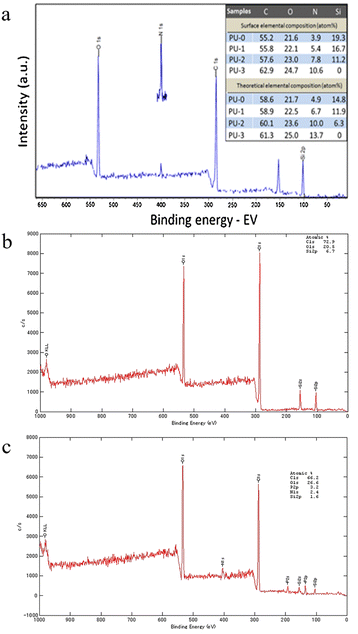 |
| Fig. 3 Comprehensive XPS analysis of different samples. (a) XPS spectra of the polyurethane film (PU-2). The inset table shows the surface and theoretical elemental concentrations of the samples.26 Reproduced from reference novel amphiphilic poly(dimethylsiloxane) based polyurethane networks tethered with carboxybetaine and their combined antibacterial and anti-adhesive property with permission from Elsevier, copyright 2017. (b) XPS spectrum of the bare PEEK substrate.20 Reproduced from reference synthesis and hydration behavior of a hydrolysis-resistant quasi-choline phosphate zwitterionic polymer with permission from American Chemical Society, copyright 2020. (c) XPS spectrum of the PMCPm brush grafted on the PEEK substrate.20 Reproduced from reference synthesis and hydration behavior of a hydrolysis-resistant quasi-choline phosphate zwitterionic polymer with permission from American Chemical Society, copyright 2020. | |
2.4. AFM
As a scanning probe microscope, AFM can measure the force difference between the probe and the sample to generate a topographical image by plotting the surface profile at a constant force (called a set point).27 Jiang et al. applied the tapping mode AFM method to study the surface morphology of polyurethane films (Fig. 4).26 All prepared polyurethane films had very flat and smooth surfaces; the hydrophobic polydimethylsiloxane (PDMS) segments showed soft features in dark color domains, whereas the hydrophilic zwitterionic segments showed hard features in bright color domains. The hydrophilic zwitterionic and hydrophobic PDMS segments are chemically incompatible because of their completely different polarities. Microphase separation occurred on the surface of polyurethane films with various aggregate morphologies. The “morphology” observed in AFM is from the isoforce1 surface, and the molecular shapes in the morphology diagrams usually reflect the forces. The relatively slow scanning speed is not suitable for the rapidly changing surfaces.27 This may be the reason why this technique has not been intensively used in recent years to investigate the zwitterionic properties.
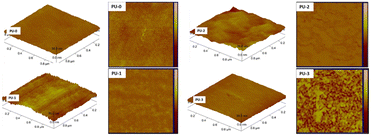 |
| Fig. 4 AFM three-dimensional images and two-dimensional phase images of the polyurethane (PU) networks in the samples of PU-0, PU-1, PU-2, PU-3(PU-0–PU-3 represent different formulations of the polyurethane network as well as the mole content of PCB and PDMS). The scale size of all AFM images is 1 μm.26 Reproduced from reference novel amphiphilic poly(dimethylsiloxane) based polyurethane networks tethered with carboxybetaine and their combined antibacterial and anti-adhesive property with permission from Elsevier, copyright 2017. | |
2.5. DSC
DSC is a powerful thermal analysis technique that measures the enthalpy change in the physical and chemical properties of materials with temperature or time.28 DSC is particularly competent for analyzing the states of water.29 However, DSC measurements can be affected by a variety of factors, such as heating rate and sample quality. Morisaku et al. investigated the hydration behavior of poly(2-methacryloyloxyethyl phosphorylcholine) (PMPC) hydrogels using DSC (Fig. 5(a)).30 They discovered that water molecules existed in two states in these hydrogels, i.e., freezable water and non-freezable water. The latter remained unfrozen because of electrostatic interactions and hydrogen bonding with the polymer chains. Compared to poly(methoxyethylene glycol)-monomethacrylate (p(Me(EG)nMA)) hydrogels, PMPC hydrogels exhibited a higher content of unfrozen water, indicating a stronger hydration capability. Mukai et al. used attenuated total reflection Fourier transform infrared spectroscopy (ATR-FTIR) and DSC to study the hydration structures of PMPC and the newly designed anti-hydrolytic PMCPm (Fig. 5(b)).20 DSC results revealed that the interaction strength between water and polymer determined the thermal behaviors, including the melting of free water, the recrystallization of frozen bound water, and the non-crystallization of non-frozen water. The presence of frozen water in PMCPm was confirmed, which indirectly indicated its potential for antifouling and biomedical applications.
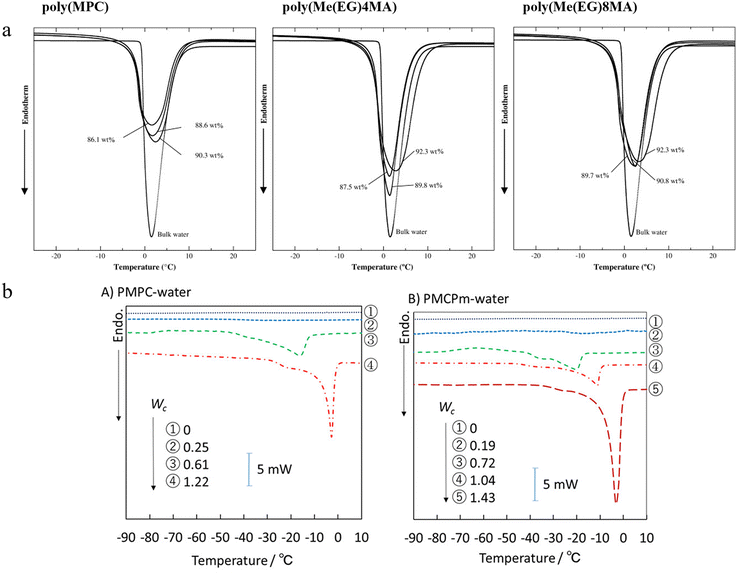 |
| Fig. 5 DSC curves of hydrogels and bulk water with various equilibrium water contents (EWCs) and water contents. (a) DSC thermograms at a heating rate of 5 °C min−1 for the poly(MPC), poly(Me(EG)4MA), and poly(Me(EG)8MA) hydrogels with different EWCs and for bulk water.30 Reproduced from reference hydration of phosphorylcholine groups attached to highly swollen polymer hydrogels studied by thermal analysis with permission from Elsevier, copyright 2008. (b) DSC thermograms (2nd heating cycle) of the hydrated PMPC and PMCPm with various water contents.20 Reproduced from reference synthesis and hydration behavior of a hydrolysis-resistant quasi-choline phosphate zwitterionic polymer with permission from American Chemical Society, copyright 2020. | |
2.6. ELISA
ELISA is an immunoassay in which an antigen or antibody is bound to the surface of a solid-phase target, and the detection of the target is achieved by using the specific binding of the antigen and antibody, as well as the enzyme-labeled on the antibody or antigen to catalyze the chromogenic reaction.31 Protein adsorption on different film surfaces can be assessed by this enzyme-linked immunosorbent assay. Jiang et al. employed ELISA to investigate the adsorption of proteins on hydrolyzed amphiphilic polyurethane films (Fig. 6(a)).26 They discovered that hydrophilic oligomer groups formed a dense water film on the surface to inhibit protein adsorption. Furthermore, the incorporation of PDMS segments with low surface energy and flexibility facilitated the reconstruction of surface molecules and polymeric groups on the polyurethane film, leading to a significant enhancement in the antifouling properties. Consequently, polyurethane materials treated by acid hydrolysis exhibited outstanding resistance to protein adsorption and bacterial adhesion. Zhang et al. performed a single protein adsorption assay using a quartz crystal dissipation microbalance (QCM-D) and monitored the adsorption process of the polymer in real time using confocal microscopy and scanning electron microscopy (Fig. 6(b)).6 The protein resistance of the naked gold surface was enhanced by the zwitterionic polypeptide coating. Li et al. used ELISA to analyze fibrinogen adsorption behaviors on zwitterionic phosphoserine-mimetic polymer (ZPS), tissue culture polystyrene (TCPS), non-zwitterionic PS-mimetic polymer (NZPS), and poly(2-methacryloyloxyethyl phosphorylcholine) (MPC) hydrogels and evaluated their anti-adsorption capabilities (Fig. 6(c)).32 All hydrogels showed excellent antifouling performance. Among them, ZPS and MPC could reduce fibrinogen adsorption by 85%, whereas NZPS only reduced fibrinogen adsorption by 40%antifouling. This indicates that ZPS, as an oligomer material, can effectively resist non-specific protein adsorption by enhancing the hydration effects. Chou and Ou assessed the protein adsorption capacity of poly(2-oxoethyl methacrylate-co-sulfobetaine methacrylate) (poly(OxMA-co-SBMA)) zwitterionic polymers using a continuous-wavelength enzyme-linked immunosorbent analyzer, revealing a significant enhancement in the anti-protein capability on the surface of silicon wafers grafted with this polymer. This research indicates that the reaction between the aldehyde zwitterionic copolymer and the amino surface generates a stable modified layer containing sufficient nanoscale copolymer grains to form an O20–S80 ratio in the grafted copolymer layer, thereby optimizing the antifouling properties. The neutral characteristic of the silyl group effectively avoids ionic interactions with charged proteins on the grafted silicon surface and enhances hydration effects, thereby preventing various protein adsorptions.33 Zhang et al. used negatively charged bovine serum albumin (BSA) and positively charged lysozyme as model foulants to test the antifouling properties of PDMAPS-containing latex films (Fig. 6(d)).22 It was demonstrated that PDMAPS brushes could reduce BSA adsorption to 1.9 μg cm−2. Compared to previously reported films with oligomer brushes,34 the PDMAPS-containing latex film exhibited stronger antifouling capabilities to prevent protein and bacterial adhesion.
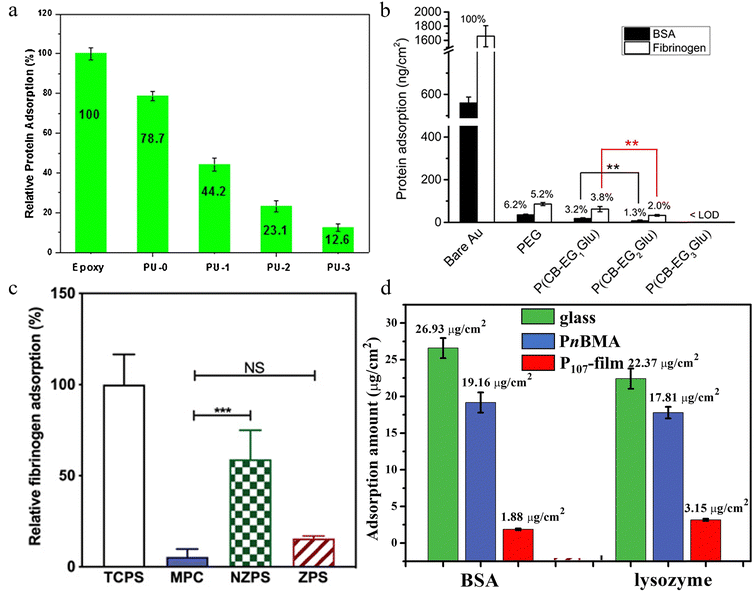 |
| Fig. 6 Protein adsorption studies on diverse surface materials and coatings. (a) Relative non-specific protein adsorptions on different PU network films and epoxy control, where the protein adsorption of the epoxy surface was set at 100%.26 Reproduced from reference novel amphiphilic poly(dimethylsiloxane) based polyurethane networks tethered with carboxybetaine and their combined antibacterial and anti-adhesive property with permission from Elsevier, copyright 2017. (b) The amount of BSA and fibrinogen on bare and different polymer-coated gold surfaces.6 Reproduced from reference investigation on the linker length of synthetic zwitterionic polypeptides for improved nonfouling surfaces with permission from American Chemical Society, copyright 2018. (c) Fibrinogen adsorbed onto TCPS, MPC, NZPS, and ZPS hydrogel surfaces measured by ELISA.32 Reproduced from reference De novo design of functional zwitterionic biomimetic material for immunomodulation with permission from Wiley, copyright 2020. (d) The amounts of adsorbed BSA and lysozyme on the surfaces of the glass slide, PnBMA film, and P107-film.22 Reproduced from reference nynamic surfaces of latex films and their antifouling applications with permission from Elsevier, copyright 2024. | |
2.7. SPR
SPR technology relies on the shift in resonance angle caused by the changes in the dielectric constant of the metal surface when proteins adsorb onto zwitterionic materials.35,36 By monitoring this change, quantitative analysis of protein adsorption on the material surface can be achieved.37 Li et al. investigated the effect of protein conformation on the antifouling performance using SPR to measure the real-time modification of the zwitterionic peptide and the non-specific adsorption of proteins. The SPR results showed that the antifouling property of the loop-like zwitterionic peptide was better than the linear-like zwitterionic peptide due to the rigidity and hydrophilicity of the cyclic structure (Fig. 7(a) and (b)).38 Feng et al. evaluated the foulant-membrane interaction using SPR. The results showed that the successful grafting of the amine N-oxide brush layer resulted in a more hydrophilic surface (Fig. 7(c) and (d)).39
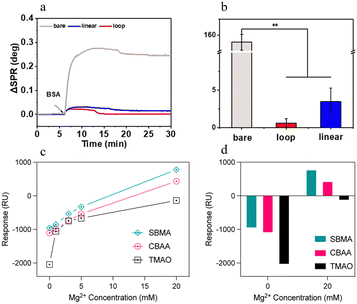 |
| Fig. 7 SPR analysis on zwitterionic polymers. (a) and (b) SPR curves and adsorption quantities after injecting 1 mg mL−1 BSA on different surfaces.38 Reproduced from reference lubricin-inspired loop zwitterionic peptide for fabrication of superior antifouling surfaces with permission from American Chemical Society, copyright 2021. (c) and (d) SPR responses to alginate adsorption on zwitterion polymer surfaces as a function of Mg2+ concentration.39 Reproduced from reference bioinspired N-oxide-based zwitterionic polymer brushes for Robust fouling-resistant surfaces with permission from American Chemical Society, copyright 2023. | |
These experimental studies explored the antifouling properties of zwitterionic polymers from different perspectives. The water structure near the zwitterionic film was investigated, and it was found that the introduction of amphiphilic groups reduced the contact angle. The water molecules near the surface of the zwitterion were less oriented, and the compromised electrostatic attraction could enhance the hydrogen bonding between the water molecules and expose the hydrophilic charged groups in the zwitterion. Strong hydrogen bonding was the key to the formation of a robust hydrated layer. Cationic solvation-induced hydration was stronger than hydrogen bonding-induced hydration, and this superior hydration property gives the zwitterion excellent antifouling properties. The shortened charge spacing also improves the fouling resistance. SFG and XPS provide information on the interfacial molecular structures and chemical states, whereas AFM visually displays the surface morphology and interaction forces. DSC analyzes the changes in thermal stability, and ELISA quantitatively detects the mass of adsorbed proteins. SPR technology enables real-time monitoring of binding and dissociation processes between proteins and material surfaces. Although experimental work can exhaustively study all aspects of the factors affecting the antifouling properties of zwitterionic polymers, it is time-consuming and labor-intensive; simulations can compensate for this shortcoming, and the combination of the two is a good way to improve efficiency.
3. Simulation
In experiments, the time required for protein absorption far exceeds the time scale of 100 ns–ms in conventional molecular dynamics.40 Molecular simulation techniques can overcome this problem. Contrary to experimental approaches for direct assessment of protein adsorption on antifouling surfaces, molecular simulations quantify subtle structural, dynamic, and interaction alterations of zwitterionic materials/surfaces under varying conditions, which can facilitate the understanding of component/structure-based antifouling properties at atomic scales.4 MD simulations are generally used to explore the interactions, structure, and dynamic changes in proteins, surfaces, water, ions, and other components under different conditions; MC simulations can determine the optimal orientation of proteins during adsorption on surfaces; molecular docking techniques are suitable for screening the most favorable adsorption combinations of proteins on surfaces in a rapid and large-scale manner.
3.1. MD simulations
With significant improvements in computation power, MD simulations have enabled researchers to model and simulate more complex and larger polymer-grafted surfaces in the absence and presence of fouling. In recent years, MD simulations have been mainly used to study the interaction between polymer and hydration layer, the grafting density of zwitterionic materials, and the stacking structure of amphiphilic polymer brushes.
3.1.1 Interaction.
Li et al. conducted MD simulations to study TMAO small molecules and PTMAO oligomers containing 10 residues in an aqueous solution. The simulation reveals that TMAO oxygen atoms accept more hydrogen bonds on average and exhibit longer hydrogen bond lifetimes than PEG oligomer (OEG) oxygen atoms (Fig. 8(a) and (b)).5 This suggests that PTMAO retains the hyperhydrophilicity observed in TMAO small molecules. The persistent strong interactions between TMAO and water molecules, including longer hydrogen bond lifetimes and tight hydration shells, were the primary reasons for the hyperhydrophilicity of PTMAO. Li et al. and Zhao et al. designed zwitterionic peptides and used MD simulations to confirm the presence of hydrogen bonds (Fig. 8(c)) and strong interaction forces (Fig. 8(d) and (e)) between the peptides and water molecules. The hydrogen bonds and interaction forces help to form a dense hydration layer on the surface of the material to resist non-specific adsorption.41 Sarker et al. used ab initio MD simulations to study the hydration behavior of zwitterions, including TMAO and carboxylated beta cycles with different charge separation distances and found that the interactions between hydrogen bonding, net charge, and dipole moments were critical for the fouling resistance of zwitterionic materials.17 A shorter zwitterionic spacing strengthens the hydrogen bonding with water due to enhanced electrostatic and inductive interactions, increases charge transfer, and improves structural stability, thus preventing the attachment of biomolecules.
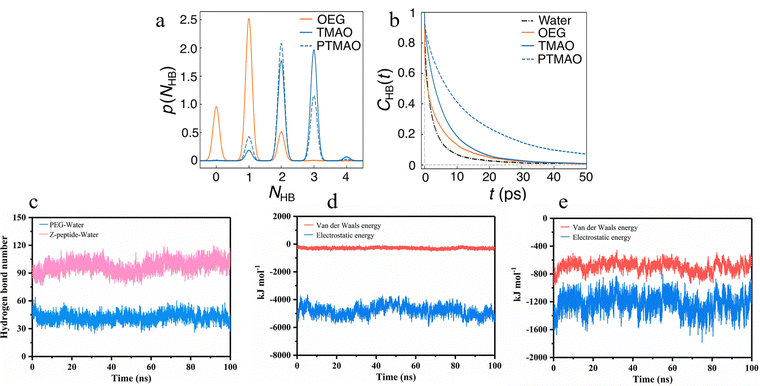 |
| Fig. 8 Molecular interactions and hydrogen bond dynamics in water–solute systems. (a) Probability distributions for the number of hydrogen bonds, NHB, between water and the OEG, TMAO, and PTMAO oxygen.5 Reproduced from reference trimethylamine N-oxide-derived zwitterionic polymers: a new class of ultralow fouling bioinspired materials with permission from Wiley, copyright 2019. (b) Autocorrelation function for tHB, CHB(t), for water–TMAO, PTMAO, and OEG oxygen and water–water hydrogen bonds.5 (c) The number of hydrogen bonds formed between the PEG molecule/Z-peptide molecule and water molecules varied with the simulation time.41 Reproduced from reference engineering an antifouling electrochemical aptasensor based on a designed zwitterionic peptide for tetracycline detection in milk with permission from Elsevier, copyright 2023. (d) The interaction energy between PEG and water molecules with the change of simulation time.41 Reproduced from reference engineering an antifouling electrochemical aptasensor based on a designed zwitterionic peptide for tetracycline detection in milk with permission from Elsevier, copyright 2023. (e) The interaction energy between Z-peptide and water molecules with the change of simulation time.41 Reproduced from reference Engineering an antifouling electrochemical aptasensor based on a designed zwitterionic peptide for tetracycline detection in milk with permission from Elsevier, copyright 2023. | |
Although the above simulations highlighted the significant contribution of hydrogen bonding between zwitterionic polymers and water molecules, as well as interaction forces, to the fouling resistance of materials, the intrinsic link between different zwitterionic structures, hydrogen bonding, and interaction forces remained unclear. Therefore, it is necessary to further investigate the relationship between the grafting profile of polymeric materials and the surface hydration layer to understand the mechanisms of fouling resistance.
3.1.2 Graft density.
Xiang et al. analyzed the surface hydration and antifouling properties of poly(sulfobetaine)-grafted (pSB) brushes via steered MD simulations.42 They discovered that grafting density mediated antifouling, where high grafting promoted hydration layer-based resistance to proteins, whereas intermediate and low grafting densities induced steric repulsion due to protein compression. Cheung et al. employed MD simulations to investigate the influence of grafting density on the conformational arrangements and hydration characteristics of a range of zwitterionic “peptoid” brushes.43 Their results revealed that at “low” and “intermediate” grafting densities, the presence of charged monomers/residues could enhance chain flexibility and increase water densities in the vicinity of the peptoid brush chains. Conversely, at high grafting densities, these positive effects were suppressed, which was primarily attributed to a reduction in free volume. Liu et al. conducted a potential mean force (PMF)-MD simulation and found that increasing the grafting density of poly(vinylidene fluoride) (PVDF-g-DMAPS) membranes could enhance the rejection of sodium alginate (SAs) (Fig. 9). The high SA rejection is attributed to the synergistic effect of stable hydration layer and electrostatic repulsion.44 The stable hydration layer prevents the hydrophobic groups of SAs from binding to the hydrophobic skeleton of the membrane, while the coordination layer and electrostatic repulsion jointly form an energy barrier, effectively repelling SAs from the membrane surface.
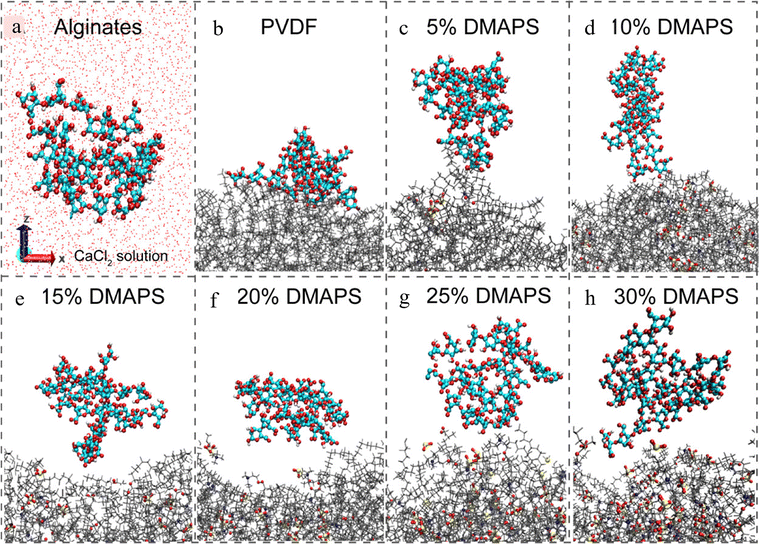 |
| Fig. 9 Natural states of alginate molecules in the brine solution (a) and at the PVDF membrane surfaces with different RDMAPS ([2-(methacryloyloxy) ethyl]dimethyl-(3sulfopropyl) (DMAPS) grafting ratio) (b)–(h) from MD simulations.44 Reproduced from reference understanding the antifouling mechanism of zwitterionic monomer-grafted polyvinylidene difluoride membranes: a comparative experimental and molecular dynamics simulation study with permission from American Chemical Society, copyright 2019. | |
Although simulations underscore the significance of polymer chain dynamics and structure in surface hydration, the role of grafting density is not well understood. In addition, these simulations cannot establish the relationship between the anti-adhesion properties and the structure of polymer brushes, and the effect of hydration forces on the anti-adhesion of proteins on polymer brushes remains unclear.45 Therefore, investigating protein adsorption on these surfaces is essential to elucidate the correlation between polymer grafting structure and hydration layer dynamics at the polymer–water interface.4 Regev et al. pioneeringly integrated experimental and computational approaches to investigate the influence of zwitterionic polymer chain density on antifouling properties (Fig. 10(a)).46 Their results highlighted the flux-dependent antifouling capacity of poly(3-[2-(N-methacryloylethyl)-N,N-dimethylammonio]propane sulfonate) (poly(SPE)). Under a dynamic flow, high-density poly(SPE) surfaces outperform low-density ones by fostering a robust hydration layer that mitigates foulant adsorption. Zhu et al. simulated the vapor-induced phase separation (VIPS) synthesis of trimethylamine oxide-derived polymer-grafted poly(vinylidene fluoride) (PVDF-g-PTMAO) membranes using MD (Fig. 10(b)).47 They found that the grafting ratio affected the membrane hydration, with higher ratios enhancing the hydrogen bonding and hydration layer stability by limiting water diffusion. Song et al. constructed a polymer brush model to assess the influence of grafting density on structural stability (Fig. 10(c)).45 The correlation between hydration properties and the structural, kinetic, and interactive facets of polymer–water and polymer–protein interfaces were studied. Polymer brushes electrostatically attracted water, bolstering hydrogen bonding within the hydrated layers, whereas hydration repulsion promoted polymer–protein separation. Simulations showed that an elevated grafting density strengthened the structural integrity, hydration, and protein adhesion resistance.
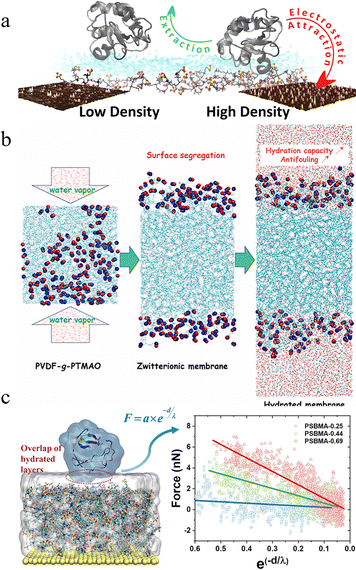 |
| Fig. 10 Molecular simulations of grafted polymers. (a) Simulation of the impact of grafted oligomer chain density on antifouling performance.46 Reproduced from reference critical surface density of zwitterionic polymer chains affect antifouling properties with permission from Elsevier, copyright 2022. (b) VIPS process of MD simulation in the preparation of PVDF-g-PTMAO membranes.47 Reproduced from reference simulated preparation and hydration property of a new-generation zwitterionic modified PVDF membrane with permission from Elsevier, copyright 2022. (c) Simulation of the impact of grafting density on the structural stability of polymer brushes.45 Reproduced from reference study of hydration repulsion of zwitterionic polymer brushes resistant to protein adhesion through molecular simulations with permission from American Chemical Society, copyright 2024. | |
3.1.3 Stacked structures.
Despite the investigation of the impact of graft density on surface hydration, polymer kinetics, and antifouling performance, prior simulations and experiments have overlooked the stacking structure of amphiphilic polymer brushes. This knowledge gap impedes the comprehensive understanding of the molecular–scale relationship between surface hydration and protein resistance. To bridge this gap, Liu et al. conducted MD simulations to study the water structure, dynamics, orientation, and protein adsorption on zwitterionic poly(ethylene glycol) polymer brushes. Steered MD simulations were used to assess the surface resistance to proteins (Fig. 11(a)).40 The results indicated that the carbon spacer length between the zwitterionic groups and the anionic group significantly impacted the antifouling properties. A positive correlation between the polymer brush surface hydration and atomic-level antifouling capacity was observed. Li et al. incorporated neutral amino acids with varying hydrophobicities (Ser, Gly, and Leu) into lysine-glutamic acid peptides. MD simulations revealed that hydrophilic Ser could develop a hydrophilic monolayer to enhance antifouling. Gly promoted interchain crosslinking, and Leu increased hydrophobicity, dehydrating the monolayer and diminishing antifouling (Fig. 11(b)).48 Using atomic MD simulations, Li et al. showed that cyclic zwitterionic peptides were stiffer than linear zwitterionic peptides, which prevented end penetration into the fouling and thus weakened zwitterionic peptide–fouling interactions.38 Cyclic zwitterionic peptides with less chain aggregation could form more hydrogen bonds with water (Fig. 11(c)), and the charge distribution of cyclic zwitterions was more homogeneous, which improved hydrophilicity and antifouling properties.
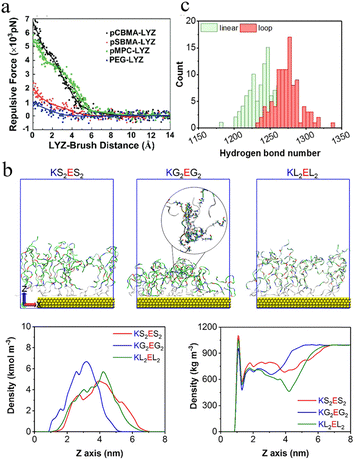 |
| Fig. 11 Molecular dynamics of protein–polymer interactions and water dynamics in zwitterionic systems. (a) Repulsive force–distance profiles between lysozyme and pCBMA, pSBMA, pMPC, and PEG brushes.40 Reproduced from reference molecular simulations and understanding of antifouling zwitterionic polymer brushes with permission from Royal Society of Chemistry, copyright 2020. (b) MD simulation snapshots of KS2ES2, KG2EG2, and KL2EL2 (serine (S), glycine (G), leucine (L), and lysine–glutamic acid (KE)). Density distributions of peptide layers and water in different systems.48 Reproduced from reference effect of hydrophobicity and charge separation on the antifouling properties of surface-tethered zwitterionic peptides with permission from American Chemical Society, copyright 2021. (c) Distribution of hydrogen bonds between zwitterionic peptides and water.38 Reproduced from reference lubricin-inspired loop zwitterionic peptide for fabrication of superior antifouling surfaces with permission from American Chemical Society, copyright 2021. | |
The above studies revealed the antifouling mechanisms of different polymer systems through MD simulations, which provided important theoretical guidance for optimizing the design of polymer brushes and developing efficient antifouling coatings. However, MD simulations are computationally expensive, requiring considerable computational resources and time to tune the model parameters to match the experimental data. Therefore, they can be combined with other simulation techniques, such as MC and molecular docking techniques, to enhance the effectiveness of the simulation.
3.2. Other simulations
The main simulation techniques used in conjunction with MD simulations were MC and molecular docking. The MC method, which is a probabilistic modeling approach, infers unknown properties through multiple simulations. Its application to polymerization reactions enables the comprehensive acquisition of polymer microstructural data, offering a straightforward modeling solution to explore the intricate polymerization mechanisms.49 MC simulation has been widely applied in polymer science to investigate polymer network structures, elastic behaviors, inter-brush forces, blend phase diagrams, etc.50 Liu et al. performed MC simulations to determine the optimal orientation of lysozyme on different zwitterionic polymers and PEG brushes (Fig. 12(a)).40
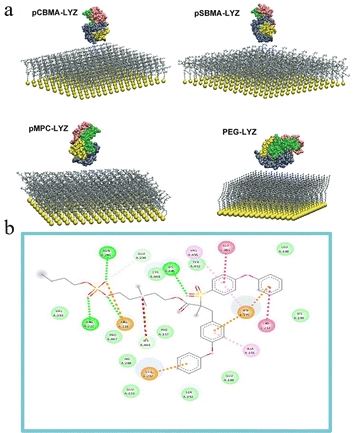 |
| Fig. 12 Molecular recognition and orientation of proteins on polymer surfaces and ligand interactions. (a) Optimal orientations of lysozyme on pCBMA, pSBMA, pMPC, and PEG brushes in the lowest energy state, as determined by MC simulations.40 (b) 2D interaction diagrams of molecular docking showing the interaction between HSA and butyl (2-((2(methacryloyloxy)ethyl)dimethylammonio)ethyl) phosphate (MBP)-polyethersulfone (PES) ligand.51 Reproduced from reference influence of dipole orientation of zwitterionic materials on hemodialysis membrane interactions with human serum proteins with permission from MDPI, copyright 2023. | |
Computational docking is a high-quality model to predict the binding conformation and binding free energy of small molecule ligands to large molecule targets.51 Nazari & Abdelrasoul employed molecular docking to forecast protein–ligand interactions, elucidating binding sites and energies of the complexes.51 The zwitterion binds to albumin (HSA) through hydrogen bonding with Asn295, Arg218, Lys436, Glu292, Lys195, and Arg222, while non-specific interactions, including hydrophobic contacts and electrostatic attractions, can be discerned between the ligand and HSA protein (Fig. 12(b)).
Both MD and MC simulations study individual zwitterionic systems in a “one-off” manner, lacking data-driven capabilities. Owing to the high computational cost, it is impossible to quickly predict the performance of many zwitterions from a given database or to build a foreign dataset by studying a sufficiently large number of zwitterions. MD offers access to dynamic properties, such as transport coefficients, time-dependent responses, rheology, and spectra. In comparison, MC offers advantages to studying static system properties, particularly when full equilibration is unfeasible due to lengthy dynamics.50 The disadvantages of molecular docking simulations are the lack of dynamic details and the influence of the environment on the binding complex. Although the time and length scales of current simulations remain a common barrier to modeling realistic antifouling experiments, this gap is expected to be bridged by continued innovation, advancement, and integration of algorithms and hardware.4
Table 1 summarizes the characteristics of each technique. These techniques are often used in combination when investigating the anti-protein adsorption properties of zwitterionic materials. For example, SFG reveals the dynamic behavior of the ionic groups on the surface; contact angle measurements reflect the surface wettability and the potential for protein adsorption. The combination of these techniques can reveal the relationship between surface molecular motions and wettability to further understand the anti-adsorption mechanism. The chemical composition of the surface can be analyzed by XPS, and the surface morphology can be observed by AFM; their combination can be used to understand the comprehensive effect of surface properties on anti-sorption performance. The thermal data provided by DSC can be combined with the MD/MC simulation results to verify the accuracy of the prediction and further study the effect of thermal performance on the anti-sorption performance. Quantitative data provided by ELISA may be integrated with the predictions from molecular docking techniques to validate the accuracy of the models. This can help to design new zwitterionic polymers with better anti-sorption properties for biomedical applications, such as cardiovascular implants and drug carriers, as well as to evaluate the anti-sorption properties of different zwitterionic polymers in seawater and provide a scientific basis for the selection of materials for ocean engineering.
Table 1 Summary of the characteristics of each technique
Name of the technique |
Advantages |
Drawbacks |
Ref. |
Experimental work |
SFG vibrational spectroscopy |
High sensitivity |
Requires complex equipment and a high level of skill to operate. |
9 and 52
|
Can be monitored in real-time. |
High cost. |
Provides a wealth of spectral information. |
|
Contact angle measurement |
Straightforward. |
Vulnerability. |
53 and 54
|
|
Suitable for different types of solid surfaces. |
Requires human judgment and manipulation, which may introduce errors. |
XPS |
Can be qualitative and quantitative. |
High equipment cost. |
55 and 56
|
High sensitivity. |
A high level of skill is required. |
|
Limitations of detection. |
|
Vulnerability. |
AFM |
Nano sale resolution |
High equipment cost. |
57 and 58
|
Information on the mechanical properties of surfaces is available. |
High technical requirements. |
Wide range of applicable inspection types. |
Scanning speeds are relatively slow and may not be suitable for rapidly changing surfaces. |
DSC |
It is possible to measure changes in material properties with temperature and time. |
High equipment cost. |
59 and 60
|
Easy analysis on the state of the structure. |
Requires a high level of skill and maintenance. |
|
It may be influenced by several factors. |
ELISA |
High specificity and sensitivity. |
Pre-processing steps can be complex. |
53 and 61
|
Can be used to assess protein fouling on different film surfaces. |
Certain reaction conditions are required. |
Results may show non-specific adsorption and false positives/negatives. |
|
SPR |
High sensitivity. |
Complex instrumentation. |
62 and 63
|
Quantitative analysis |
Sensitivity to environmental conditions. |
Real-time monitoring. |
Surface preparation and modification. |
Label-free detection. |
Limited throughput. |
|
Simulation |
MD simulation |
High authenticity. |
Heavy calculations. |
64–66
|
Complex systems can be modeled. |
Long simulation time. |
Micro-mechanisms can be studied. |
Sensitive to initial conditions. |
Flexibility. |
|
MC simulation |
Statistically strong. |
Less dynamic. |
50
|
Computationally efficient. |
Lack of physical intuition. |
Easy to implement. |
Susceptible astringency and |
Molecular docking technology |
Predictive. |
Accuracy. |
67 and 68
|
Fast and effective. |
Heavy calculations. |
Inexpensive. |
Results need to be interpreted in the context of the experiment. |
The abovementioned technologies assist scientists in gaining a deeper understanding of the antifouling mechanisms of zwitterionic polymers, which ensure their widespread use in biomedicine (e.g., reducing thrombosis and foreign body reactions in heart valves, vascular stents, and dialysis membranes), industrial separation (e.g., antifouling membranes in distillation and seawater desalination), cell preservation, and antibacterial protection. These practical applications demonstrate the enormous potential of zwitterionic polymers for continuous improvements in device performance, water quality safety, cellular health, and antibacterial properties.
4. Conclusions
The resistance of zwitterionic polymers to protein adsorption has been investigated using several experimental and modeling techniques. Experimental techniques allow a detailed and accurate study of various factors affecting the hydration effect and the fouling resistance mechanism of zwitterionic polymers. The low directionality of water molecules near the surface of zwitterionic polymers and strong hydrogen bonding are crucial considerations for the prevention of protein adsorption. Shortening the charge spacing can weaken the electrostatic and dipole–dipole interactions and improve the fouling resistance. However, experimental techniques are time-consuming and difficult to rapidly analyze all aspects of the principles. In contrast, simulations can quickly obtain microstructural information about the materials. In recent years, simulation research has focused on the interactions between zwitterionic polymers and the hydration layer, grafting densities of zwitterionic materials, and stacking structures. However, simulation work has the drawbacks of high computational effort and lack of physical intuition. Therefore, the combination of experimental and modeling techniques is essential to analyze the resistance of polymers to protein adsorption and to understand the principles underlying this process. There are also some potential issues in these studies; for example, the effect of zwitterionic chain density on antifouling must be considered under both dynamic and static conditions. Some studies have tried only one scenario before concluding that high density is good for antifouling, or low density is good for antifouling. Inconsistent results often stem from the use of different experimental and simulation techniques. Therefore, comprehensive information can be obtained only by combining several techniques.
Data availability
No primary research results, software, or code have been included and no new data were generated or analyzed as part of this review.
Conflicts of interest
There are no conflicts to declare.
Acknowledgements
This work was supported by Natural Science Foundation of China (grant no. 32472424) and the 2115 Talent Development Program of China Agricultural University.
References
- C. Corbo, R. Molinaro, A. Parodi, N. E. Toledano Furman, F. Salvatore and E. Tasciotti, Nanomedicine, 2016, 11, 81–100 CrossRef CAS PubMed.
-
T. A. Horbett and R. A. Latour, Biomaterials Science, Elsevier, 2020, pp. 645–660 Search PubMed.
- Q. Wei, T. Becherer, S. Angioletti-Uberti, J. Dzubiella, C. Wischke, A. T. Neffe, A. Lendlein, M. Ballauff and R. Haag, Angew. Chem., Int. Ed., 2014, 53, 8004–8031 CrossRef CAS PubMed.
- Q. Li, C. Wen, J. Yang, X. Zhou, Y. Zhu, J. Zheng, G. Cheng, J. Bai, T. Xu, J. Ji, S. Jiang, L. Zhang and P. Zhang, Chem. Rev., 2022, 122, 17073–17154 CrossRef CAS PubMed.
- B. Li, P. Jain, J. Ma, J. K. Smith, Z. Yuan, H.-C. Hung, Y. He, X. Lin, K. Wu, J. Pfaendtner and S. Jiang, Sci. Adv., 2019 DOI:10.1126/sciadv.aaw9562.
- C. Zhang, J. Lu, Y. Hou, W. Xiong, K. Sheng and H. Lu, ACS Appl. Mater. Interfaces, 2018, 10, 17463–17470 CrossRef CAS.
- L. Zhang, Z. Cao, T. Bai, L. Carr, J.-R. Ella-Menye, C. Irvin, B. D. Ratner and S. Jiang, Nat. Biotechnol., 2013, 31, 553–556 CrossRef CAS PubMed.
- L. R. Carr, Y. Zhou, J. E. Krause, H. Xue and S. Jiang, Biomaterials, 2011, 32, 6893–6899 CrossRef CAS.
- Z. Chen, Langmuir, 2022, 38, 4483–4489 CrossRef CAS.
- Q. Shao and S. Jiang, Adv. Mater., 2015, 27, 15–26 CrossRef CAS PubMed.
- L. Mi, M. M. Giarmarco, Q. Shao and S. Jiang, Biomaterials, 2012, 33, 2001–2006 CrossRef CAS PubMed.
- Y. R. Shen, J. Phys. Chem. C, 2012, 116, 15505–15509 CrossRef CAS.
- C. Leng, S. Sun, K. Zhang, S. Jiang and Z. Chen, Acta Biomater., 2016, 40, 6–15 CrossRef CAS.
- C. Leng, H.-C. Hung, O. A. Sieggreen, Y. Li, S. Jiang and Z. Chen, J. Phys. Chem. C, 2015, 119, 8775–8780 CrossRef CAS.
- T. Kondo, K. Nomura, M. Gemmei-Ide, H. Kitano, H. Noguchi, K. Uosaki and Y. Saruwatari, Colloids Surf., B, 2014, 113, 361–367 CrossRef CAS PubMed.
- P. Sarker, T. Lu, D. Liu, G. Wu, H. Chen, M. S. J. Sajib, S. Jiang, Z. Chen and T. Wei, Chem. Sci., 2023, 14, 7500–7511 RSC.
- C. Leng, H.-C. Hung, S. Sun, D. Wang, Y. Li, S. Jiang and Z. Chen, ACS Appl. Mater. Interfaces, 2015, 7, 16881–16888 CrossRef CAS.
- S. Hladysh, D. Oleshchuk, J. Dvořáková, A. Golunova, P. Šálek, J. Pánek, O. Janoušková, D. Kaňková, E. Pavlova and V. Proks, Eur. Polym. J., 2021, 148, 110347 CrossRef CAS.
- T. Huhtamäki, X. Tian, J. T. Korhonen and R. H. A. Ras, Nat. Protoc., 2018, 13, 1521–1538 CrossRef.
- M. Mukai, D. Ihara, C.-W. Chu, C.-H. Cheng and A. Takahara, Biomacromolecules, 2020, 21, 2125–2131 CrossRef CAS.
- S. Mihara, K. Yamaguchi and M. Kobayashi, Langmuir, 2019, 35, 1172–1180 CrossRef CAS PubMed.
- J. Zhang, C. Wang and H. Zhao, J. Colloid Interface Sci., 2024, 654, 1281–1292 CrossRef CAS PubMed.
- R. Ghobeira, P. S. Esbah Tabaei, R. Morent and N. De Geyter, Surf. Interfaces, 2022, 31, 102087 CrossRef CAS.
- P. S. Esbah Tabaei, M. Asadian, R. Ghobeira, P. Cools, M. Thukkaram, P. G. Derakhshandeh, S. Abednatanzi, P. Van Der Voort, K. Verbeken, C. Vercruysse, H. Declercq, R. Morent and N. De Geyter, Carbohydr. Polym., 2021, 253, 117211 CrossRef CAS PubMed.
- P. S. Esbah Tabaei, R. Ghobeira, P. Cools, F. Rezaei, A. Nikiforov, R. Morent and N. De Geyter, Polymer, 2020, 193, 122383 CrossRef.
- J. Jiang, Y. Fu, Q. Zhang, X. Zhan and F. Chen, Appl. Surf. Sci., 2017, 412, 1–9 CrossRef CAS.
- T. Sumikama, Biophys. Rev., 2023, 15, 2059–2064 CrossRef CAS.
- Q. Zheng, Y. Zhang, M. Montazerian, O. Gulbiten, J. C. Mauro, E. D. Zanotto and Y. Yue, Chem. Rev., 2019, 119, 7848–7939 CrossRef CAS PubMed.
- P. Talik and U. Hubicka, J. Therm. Anal. Calorim., 2018, 132, 445–451 CrossRef CAS.
- T. Morisaku, J. Watanabe, T. Konno, M. Takai and K. Ishihara, Polymer, 2008, 49, 4652–4657 Search PubMed.
- K. Shah and P. Maghsoudlou, Br. J. Hosp. Med., 2016, 77, C98–C101 CrossRef PubMed.
- B. Li, Z. Yuan, P. Jain, H.-C. Hung, Y. He, X. Lin, P. McMullen and S. Jiang, Sci. Adv., 2020, 6, eaba0754 Search PubMed.
- Y.-N. Chou and M.-Z. Ou, ACS Appl. Polym. Mater., 2023, 5, 5411–5428 CrossRef CAS.
- C. Zhang, J. Zhou, X. Ye, Z. Li and Y. Wang, Macromolecules, 2021, 54, 4236–4245 CrossRef CAS.
- W. Yang, S. Chen, G. Cheng, H. Vaisocherová, H. Xue, W. Li, J. Zhang and S. Jiang, Langmuir, 2008, 24, 9211–9214 CrossRef CAS PubMed.
- M. Vrabcová, M. Spasovová, M. Houska, K. Mrkvová, N. S. Lynn, L. Fekete, O. Romanyuk, A. Dejneka and H. Vaisocherová-Lísalová, Prog. Org. Coat., 2024, 188, 108187 CrossRef.
- N. D. Brault, C. Gao, H. Xue, M. Piliarik, J. Homola, S. Jiang and Q. Yu, Biosens. Bioelectron., 2010, 25, 2276–2282 CrossRef CAS PubMed.
- C. Li, Y. Xia, C. Liu, R. Huang, W. Qi, Z. He and R. Su, ACS Appl. Mater. Interfaces, 2021, 13, 41978–41986 CrossRef CAS.
- Z. Feng, X. Feng and X. Lu, Environ. Sci. Technol., 2023, 57, 7298–7308 CrossRef CAS PubMed.
- Y. Liu, D. Zhang, B. Ren, X. Gong, L. Xu, Z.-Q. Feng, Y. Chang, Y. He and J. Zheng, Environ. Sci. Technol., 2020, 8, 3814–3828 CAS.
- Y. Li, X. Pu, M. Niu, X. Fan, Y. Ding, W. Ma and Y. Gu, Food Control, 2023, 153, 109929 CrossRef CAS.
- Y. Xiang, R.-G. Xu and Y. Leng, Langmuir, 2018, 34, 2245–2257 CrossRef CAS.
- D. L. Cheung and K. H. A. Lau, Langmuir, 2019, 35, 1483–1494 Search PubMed.
- Z.-Y. Liu, Q. Jiang, Z. Jin, Z. Sun, W. Ma and Y. Wang, ACS Appl. Mater. Interfaces, 2019, 11, 14408–14417 CrossRef CAS PubMed.
- X. Song, J. Man, Y. Qiu, J. Wang, R. Li, Y. Zhang, G. Cui, J. Li, J. Li and Y. Chen, ACS Appl. Mater. Interfaces, 2024, 16(14), 17145–17162 CrossRef CAS PubMed.
- C. Regev, Z. Jiang, R. Kasher and Y. Miller, Appl. Surf. Sci., 2022, 596, 153652 CrossRef CAS.
- H. Zhu, Z. Chen, L. Qin, L. Zhang and J. Zhou, J. Membr. Sci., 2022, 652, 120498 CrossRef CAS.
- C. Li, M. Li, W. Qi, R. Su and J. Yu, Langmuir, 2021, 37, 8455–8462 CrossRef CAS.
- D. P. Kroese, T. Brereton, T. Taimre and Z. I. Botev, Wiley Interdiscip. Rev. Comput. Stat., 2014, 6, 386–392 CrossRef.
- D. Meimaroglou and C. Kiparissides, Ind. Eng. Chem. Res., 2014, 53, 8963–8979 CrossRef CAS.
- S. Nazari and A. Abdelrasoul, Appl. Sci., 2023, 13, 12777 CrossRef CAS.
- C. A. D. Grosso, C. Leng, K. Zhang, H.-C. Hung, S. Jiang, Z. Chen and J. J. Wilker, Chem. Sci., 2020, 11, 10367–10377 RSC.
- S. Hladysh, D. Oleshchuk, J. Dvořáková, I. Šeděnková, M. Filipová, Z. Pobořilová, J. Pánek and V. Proks, J. Appl. Polym. Sci., 2022, 139, 52099 CrossRef CAS.
- A. Venault, S.-H. Tang, H.-F. Lin, C.-L. Liu and Y. Chang, J. Membr. Sci., 2023, 685, 121962 CrossRef CAS.
- N. Burmeister, C. Vollstedt, C. Kröger, T. Friedrich, N. Scharnagl, M. Rohnke, E. Zorn, S. G. Wicha, W. R. Streit and W. Maison, Colloids Surf., B, 2023, 224, 113195 CrossRef CAS PubMed.
- J. Zhang, S. Bo, H. Feng, B. Yu, Q. Yu, W. Yang, X. Pei and F. Zhou, Coatings, 2021, 11, 1164 CrossRef CAS.
- J. Zhang, L. M. A. Ali, D. Durand, M. Gary-Bobo and P. Hesemann, Langmuir, 2024, 40, 3463–3471 CAS.
- T. B. Donadt and R. Yang, Adv. Mater. Interfaces, 2021, 8, 2001791 CrossRef CAS.
- G. Ma, F. Ji, W. Lin and S. Chen, J. Biomater. Sci., Polym. Ed., 2022, 33, 1012–1024 CrossRef CAS PubMed.
- A. Venault, R.-J. Zhou, T. A. Galeta and Y. Chang, J. Membr. Sci., 2022, 658, 120760 CrossRef CAS.
- J. Ma, W. Lin, L. Xu, S. Liu, W. Xue and S. Chen, Langmuir, 2020, 36, 3251–3259 CrossRef CAS PubMed.
- C.-J. Huang, L. Mi and S. Jiang, Biomaterials, 2012, 33, 3626–3631 CrossRef CAS.
- C. Gao, G. Li, H. Xue, W. Yang, F. Zhang and S. Jiang, Biomaterials, 2010, 31, 1486–1492 CrossRef CAS.
- C. Li, C. Liu, M. Li, X. Xu, S. Li, W. Qi, R. Su and J. Yu, Biomacromolecules, 2020, 21, 2087–2095 CrossRef CAS PubMed.
- Z. Yang, X. Zhang, M. Xie, H.-C. Wu, T. Yoshioka, D. Saeki and H. Matsuyama, J. Membr. Sci., 2020, 614, 118515 CrossRef CAS.
- Q. Shao and S. Jiang, J. Phys. Chem. B, 2014, 118, 7630–7637 CrossRef CAS PubMed.
- D. J. Miller, D. R. Dreyer, C. W. Bielawski, D. R. Paul and B. D. Freeman, Angew. Chem., Int. Ed., 2017, 56, 4662–4711 CrossRef CAS.
- Z. X. Wang, S. S. Yao, Z. F. Han, Z. Li, Z. W. Wu, H. H. Hao, D. Q. Feng and H. Zhu, Ecotoxicol. Environ. Saf., 2024, 274, 116187 CrossRef CAS PubMed.
|
This journal is © The Royal Society of Chemistry 2025 |
Click here to see how this site uses Cookies. View our privacy policy here.