DOI:
10.1039/D4VA00378K
(Tutorial Review)
Environ. Sci.: Adv., 2025,
4, 530-570
Emerging membrane technologies for sustainable water treatment: a review on recent advances†
Received
3rd November 2024
, Accepted 16th January 2025
First published on 17th January 2025
Abstract
The growing scarcity of freshwater resources, coupled with industrial pollution, necessitates the development of efficient and sustainable water treatment technologies. Membrane-based desalination and heavy metal removal processes are at the forefront of these technologies, providing efficient and reliable solutions to meet the growing demand for clean water. This study provides a comprehensive review on recent advancements in desalination technologies, focusing on emerging materials that have significantly influenced desalination and heavy metal removal performances. A meticulous screening of recent review papers on both along with experimental studies published within the last year is provided, thereby offering an updated perspective on the ongoing experiments dedicated to water treatment using membranes. Notably, this review considers various membrane types, including nanocomposites, biomimetic, thin-film composites, hybrids, and membranes associated with forward osmosis. Results indicate that nanocomposite membranes, thin-film composite membranes, and forward osmosis membranes are widely used for desalination and heavy metal removal compared to hybrid and biomimetic membranes. This widespread utilization can be attributed to their well-established fabrication techniques, robust mechanical properties, high removal%, and better scalability for industrial applications. In contrast, while hybrid and biomimetic membranes are promising, they are still under development and facing challenges pertaining to material synthesis, cost, and integration into existing systems.
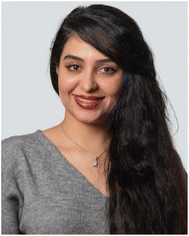 Sahar Foorginezhad | Sahar Foorginezhad is a PhD student in Energy Engineering in the Department of Engineering Sciences and Mathematics at Luleå University of Technology, Sweden (2021–2025). She earned her MSc in Nanochemical Engineering from Shiraz University, Iran (2018) and a BSc in Chemical Engineering from Shahid Bahonar University, Kerman, Iran, (2010). Currently, she is also a volunteer research assistant at Macquarie University, Australia. Her research background includes the fabrication of ceramic/polymeric membranes and nanomaterials for water treatment, development of superhydrophobic/self-cleaning coatings, CO2 capture, and the synthesis of ionic liquids and deep eutectic solvents. |
 Mohammad Mahdi Zerafat | Dr Mohammad Mahdi Zerafat earned his PhD in Nanotechnology (Chemical Engineering) from the University of Tehran (2012) and joined Shiraz University's Faculty of Advanced Technologies in the same year. Since 2016, he has been an Associate Professor and Director of the Membrane Separation Processes Research Laboratory. In 2018, he became the Deputy of Research and Education. Dr Zerafat's research focuses on nanotechnology, including the synthesis of nanomaterials, super-hydrophobicity, supercapacitors, and nanocomposites. He also specializes in membrane separation processes like nanofiltration, forward osmosis, ceramic microfiltration, and water treatment techniques for producing clean water to safeguard human health and the environment. |
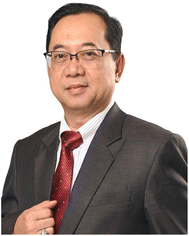 Ahmad Fauzi Ismail | Professor Datuk Ir. Ts. Dr Ahmad Fauzi bin Ismail is a renowned expert in membrane technology. He holds a BEng in Petroleum Engineering, an MSc in Chemical Engineering from UTM, and a PhD from the University of Strathclyde, UK. His research focuses on polymeric, inorganic, and mixed matrix membranes for applications in water desalination, wastewater treatment, and gas separation. He is the founding Director of the Advanced Membrane Technology Research Centre (AMTEC) and has developed 25 patents. He has published more than 1511 journal papers, 84 book chapters, and 12 books. He has received over 140 awards, including the Mustafa Prize Laureate in 2023. |
 Pei Sean Goh | Dr Pei Sean Goh received her PhD in Gas Engineering (2012) from Universiti Teknologi Malaysia (UTM). She is currently an Associate Professor in the School of Chemical and Energy Engineering at UTM, a Research Fellow at the Advanced Membrane Research Technology Research Centre (AMTEC), and the Head of the Nanostructured Materials Research Group. Her research focuses on the synthesis of nanostructures and polymer-based composites for membrane-based separation, particularly for acidic gas removal, desalination, and wastewater treatment. She is also an associate member of the Academy of Sciences Malaysia, member of Global Young Academy and the fellow of L'Oreal-UNESCO for Women in Science Malaysia. |
Environmental significance
Water scarcity and contamination by heavy metals pose serious threats to environmental and public health globally. Current desalination and heavy metal removal technologies often suffer from limitations in energy efficiency, sustainability, and effectiveness. This review examines the recent advances in membrane technologies, focusing on innovations that enhance the efficiency and environmental compatibility of desalination and heavy metal removal processes. The key findings highlight breakthroughs in material design and process optimization, presenting implications for reducing environmental impacts and improving access to clean water. Thus, this work underscores the need for continued innovation in membrane technologies to address critical water quality issues and supports sustainable environmental management.
|
1. Introduction
The past century has witnessed a rapid surge in global population, alongside explosive industrial development and escalating energy requirement. Consequently, water scarcity has emerged as a formidable global challenge, with projections indicating a worsening scenario in the future.1,2 The World Health Organization (WHO) reports a persistent rise in water pollution, identifying it as a significant contributor to the widespread illnesses and fatalities worldwide.3,4 Freshwater resources are limited, with roughly 97% of the global water reservoirs consisting of seawater, necessitating desalination for most applications and generally <1% of the world's water supply is readily available as freshwater.5,6 In this context, desalination technologies, offering the capability to harvest freshwater from saline sources, stand as a promising avenue for increasing freshwater supply and achieving global water security.7 Two primary approaches, namely, thermal/distillation and membrane separation, are mainly used for desalination, where membrane separation technology has garnered widespread attention over its counterpart owing to its high efficiency and low energy demand.8,9 A diverse range of water and wastewater filtration systems, including conventional pressure-driven seawater and brackish water reverse osmosis (RO), nanofiltration (NF), ultrafiltration (UF), and microfiltration (MF), have been employed in industrial plants. Additionally, osmotically driven technologies, such as forward osmosis (FO) and pressure retarded osmosis (PRO), have been extensively developed.10
Apart from the salt and mineral content of saline water, the escalating pace of industrialization and population growth has resulted in heavy metal contamination attributed to its widespread prevalence, significant toxicity, and facile mobility.11,12 The contemporary heavy metal pollution is predominantly driven by anthropogenic activities, such as electroplating, petroleum refining, and metal smelting. Excessive discharge of heavy metal ions, including Hg, Cd, Pb, As, and Cr, into water bodies, such as drinking water sources, poses significant health risks to humans.13,14 In this context, membrane separation technologies have also emerged as a promising route for large-scale applications owing to its outstanding heavy metal rejection capability and high operational efficiency.15,16
In general, the pressure-driven membrane technology using MF, UF, NF, and RO represents the most widely employed technique, wherein transmembrane pressure is used to facilitate the transport of feed stream across the membrane, while non-pressure-driven membrane processes such as FO present notable advantages for efficient separation, characterized by operation under mild conditions (atmospheric pressure) and potential for in situ separation during product synthesis.17 While membranes have been extensively employed for heavy metal removal and desalination, there remains room for improvement and challenges to overcome. These challenges include membrane fouling, material-based limitations, capital costs, reproducibility issues, detachment of membrane layers, low selectivity, and water flux drop. Addressing these challenges will be crucial for further advancements in membrane-based separation approaches for desalination and heavy metal removal.
In the rapidly evolving field of wastewater treatment using membranes, multiple review studies have summarized the experimental findings. It is crucial to compile current knowledge for ongoing and future research in wastewater treatment and environmental protection. However, many existing studies are still in the research phase and often lack comprehensive documentation of membrane properties. This gap poses challenges in selecting the most suitable membrane for specific wastewater conditions including pollutant type, concentration, temperature, pressure, and desired purity of water. To address these challenges, an updated database has been compiled, focusing on removal percentages and other key properties of commonly used membranes such as nanocomposites, thin-film composites, biomimetic membranes, forward osmosis membranes, and hybrid organic–inorganic membranes. This compilation aims to explore potential correlations among the properties, with a specific focus on developments from 2023 to 2024. Given the inconsistent reporting of key parameters such as removal efficiency, permeability, cost, long-term stability, durability, and fouling resistance, along with the interdependence of structural properties and surface chemistry, identifying a promising membrane becomes a complex task. Considering the methodology adopted in our previous study,18 this study provides a comprehensive literature review and screening of both review papers and experimental studies. The initial screening and survey are conducted based on removal efficiency as the primary criterion, and top-performing membranes are identified. The discussion further explores membrane performance and key properties including permeability, economic viability, fouling resistance, comparisons across various membrane types, and considerations of environmental impact and sustainability. Additionally, the role of artificial intelligence (AI) and machine learning (ML) in enhancing membrane-based processes is examined. Finally, the challenges facing the field and potential future directions are thoroughly analyzed.
2. Separation mechanisms
The membranes facilitate selective removal and separation using the unique physicochemical properties of solutes, including size, charge, and chemical composition. The interaction between solutes and the membrane matrix dictates which solutes are permeable and which are rejected.19
2.1. Size-based separation
Size exclusion is a fundamental mechanism in membrane-based separations, crucial for filtering particles based on their size, relative to the pore size of the membrane (Fig. 1). As represented in Fig. 2(a), particles smaller than the membrane pores can permeate through the membrane, while larger particles are excluded. In the context of hydrated ions and salts, steric hindrance plays a pivotal role: ions with a hydrated diameter exceeding the membrane pore size encounter significant resistance and are effectively rejected.22 This mechanism underscores the importance of pore size in determining the selectivity and efficiency of the membrane. For neutral solutes, size exclusion remains the primary mode of separation, highlighting its universal applicability in membrane filtration processes.23 As shown in Table 1, the sizes of common metal and salt ions are all less than 1 nm. Despite their small size, these ions can be effectively removed through size exclusion, provided that the membrane pore size is sufficiently small. This highlights the importance of precise pore size control in membrane fabrication to ensure high rejection rates of these ions.
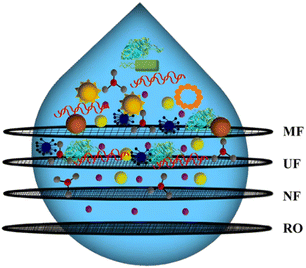 |
| Fig. 1 Schematic of particle separation via size-sieving using membranes (reprinted with permission from ref. 20, Elsevier 2023). | |
 |
| Fig. 2 Three primary membrane separation mechanisms: (a) size exclusion, (b) Donnan exclusion, and (c) dielectric exclusion (reprinted with permission from ref. 21, Wiley 2023). | |
Table 1 Size of bare and hydrated ions
Ion |
Bare ion size (Å) |
Ref. |
Hydrated ion size (Å) |
Ref. |
Li+ |
0.60 |
24
|
3.82 |
24 and 25 |
Ni2+ |
0.69 |
26
|
4.04 |
27
|
Na+ |
0.95 |
24
|
3.58 |
24 and 25 |
K+ |
1.33 |
24
|
3.31 |
24 and 25 |
Mg2+ |
0.72 |
25 and 28 |
4.28 |
24 and 25 |
Ca2+ |
1.00 |
25
|
4.12 |
24 and 25 |
Cl− |
1.81 |
24 and 28 |
3.32 |
24 and 25 |
SO42- |
2.90 |
24
|
3.79 |
24
|
NO3− |
2.64 |
24
|
3.35 |
24
|
Pb2+ |
1.63 |
29
|
4.01 |
24
|
Zn2+ |
0.74 |
30
|
4.30 |
24
|
Mn2+ |
0.80 |
30
|
4.38 |
24
|
Cr3+ |
0.62 |
31
|
4.61 |
24
|
Cu2+ |
0.73 |
32
|
4.19 |
24
|
Cd2+ |
0.95 |
32
|
4.26 |
25
|
One limitation of this model is its failure to consider concentration polarization (CP), which is crucial in membrane design. CP involves the accumulation of retained solutes in the boundary layer of the membrane, leading to a higher solute concentration at the membrane surface compared to the bulk solution. This concentration gradient is critical for both fouling and solute retention. Additionally, the separation of ionic species relies not only on size exclusion but also on the charge of the membrane, which plays a vital role in the separation process.23
2.2. Charge-based separation
In liquid-phase separations, membranes often incorporate charged functional groups that selectively bind to solutes with opposite charges (counterions) while repelling those with similar charges (co-ions) via the Donnan exclusion33 (Fig. 2(b)). Neutral solutes, which lack a charge, typically experience minimal interaction with these membranes.34 The Donnan exclusion, proposed in 1911, remains as a foundational theory for understanding ion distribution across semi-permeable membranes.35–37 This is particularly significant in ion-exchange membranes where electrical forces drive the process, and in nanofiltration membranes where pressure differentials play a crucial role.38
The Donnan exclusion relies on electrostatic interactions between the membrane's fixed charge groups and the ionic species. Membranes with negatively charged surfaces strongly repel anions, and the degree of exclusion increases with ionic charge density. Multivalent ions, possessing a higher charge density than their monovalent counterparts, generate stronger electrostatic interactions, leading to greater rejection.39,40 For instance, as shown by Epsztein et al.,39 fluoride ions with high charge density were rejected more effectively than chloride or nitrate ions under certain pH conditions due to the Donnan exclusion effects. Similarly, Balster et al.41 demonstrated that the transport of Ca2+ ions through sPEEK/PES blend membranes is significantly affected by both the conductivity and charge density of the membrane, where higher conductivity and charge density led to enhanced transport of these multivalent ions. Gilron et al.42 found that NF200 and NF45 membranes exhibited significant divalent ion rejection of >90% for MgSO4, while NaCl rejection remains comparatively low, often between 60 and 80%. Asante-Sackey et al.'s43 review highlighted the Donnan membrane process (DMP) as an efficient, energy-free method for the recovery and removal of multivalent ions such as Al3+, Fe3+, and Mg2+. Using Nafion 117 membranes, Mg2+ and Ca2+ removal rates of 50 and 20%, respectively, were reported, while Au+ recovery from electronic waste reached up to 89%.44–47 Seidel et al.48 investigated the impact of surface charge and pore size on the rejection of NaCl, CaCl2, and Na2SO4. Findings revealed that the rejection rates followed the order of Na2SO4 > NaCl > CaCl2, attributed to the Donnan exclusion principles, where ion rejection increases with higher charged co-ions and decreases with higher charged counter-ions. Specifically, Na2SO4 exhibited the highest rejection with a significant negative charge of co-ions. Conversely, CaCl2 showed the lowest rejection rate with a higher charge of counterions. These results indicate that ion separation is primarily governed by the combination of size and Donnan exclusion, whereas the observed rejection trends do not correspond to the sizes of the hydrated ions.
Nicolini et al.49 investigated the saline rejection performance of NF membranes, observing varying degrees of rejection for different salts in the following order: Na2SO4 > K2SO4 > CaSO4 > MgSO4 > NaCl. This sequence reflects the interactions of membranes with ions, where stronger rejection of sulfate salts (SO42−) is due to electrostatic repulsion with the negative charge of the membrane. Conversely, divalent cations (e.g., Ca2+ and Mg2+) exhibit greater attraction to the membrane, leading to higher rejection rates than that of monovalent cations such as Na+. Moreover, the concentration distribution of ions within the electrical double layer is significantly influenced by the surface potential, which regulates the partitioning of ions within the membrane, thereby determining ion concentrations and impacting their subsequent diffusion through the pores.38
Despite these insights, experimental data on the nuanced behavior of specific ions remain sparse, especially concerning the variable performance of membranes favoring monovalent or multivalent salts. Factors such as feedwater composition, membrane surface charge heterogeneity, and dynamic operating conditions introduce additional complexities.50–52 Addressing these gaps requires systematic studies that integrate advanced modeling approaches such as the Donnan steric pore model (DSPM) with dielectric exclusion, alongside empirical validations.38,53
2.3. Further mechanisms and considerations
Chemical properties of solutes play crucial roles in their transport and selectivity across membranes, extending beyond considerations of size and charge. Factors including hydrophobicity/hydrophilicity, polarity, polarizability, hydrogen bonding capabilities (both donating and accepting), and interactions governed by van der Waals forces have all been identified as influential in determining the solute behavior during membrane processes.54–59 Dielectric exclusion (Fig. 2(c)) refers to the phenomenon where ions interact with the bound electrical charges at the interface of materials with different dielectric constants such as the membrane matrix and the surrounding solvent. This mechanism is independent of ionic charge sign but enhances the rejection of ions with a higher valence due to their stronger polarization effects. This property is especially pronounced in confined membrane environments, where the altered dielectric properties of water further enhance ion rejection.38 Studies including that conducted by Suhalim et al.23 highlight the role of dielectric exclusion in supplementing the Donnan and size exclusion mechanisms, particularly for high-valence ions. This interaction induces polarization within the two media according to their dielectric properties, leading to the formation of polarization charge distribution at the interface surface.23 Zhu et al.60 investigated how dielectric exclusion affects the relationship between fixed charge distribution and electrolyte rejection performance. The findings indicated that reducing the dielectric constant enhances the rejection performance.
Despite extensively studied size and Donnan exclusion mechanisms, hydration-based mechanism has received relatively less attention in the literature. This approach relies on the affinity of water molecules to ions dissolved in the solution (Fig. 3(a)). Specifically, hydrated ions (ions surrounded by water molecules) exhibit different sizes and affinities towards the membrane matrix compared to their dehydrated counterparts (Table 1). This difference in hydration shell size can influence their transport through the membrane. For instance, larger hydrated ions may face steric hindrance within smaller membrane pores, leading to reduced permeation rates. Additionally, the hydration shells of ions can alter their effective charge and interactions with the membrane surface, influencing their rejection or permeation behavior23 (Fig. 3(b)). Chen et al.62 studied the mechanism of water transport and ion movement across the multilayer GO membrane. The results revealed that the difference in hydration radius between Mg(II) and Ca(II) ions influences their ability to penetrate GO membrane. Due to its smaller hydration radius, Mg(II) ions can more easily infiltrate bilayer GO membranes than Ca(II), which has a larger hydration radius.
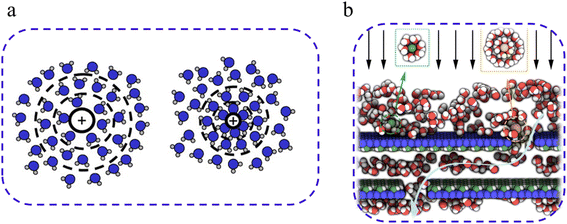 |
| Fig. 3 (a) Hydration shells around a large and a small ion (reprinted with permission from ref. 61) and (b) hydrated ions passing through the bilayer GO membrane (reprinted with permission from ref. 62). | |
Concentration polarization (CP) plays a pivotal role in the performance and sustainability of membrane-based separation processes, significantly influencing fouling and rejection efficiency. CP refers to the accumulation of solutes near the membrane surface due to selective rejection. This localized concentration gradient reduces the driving force for separation, impacting the overall solute rejection and increasing the energy demand for maintenance.63,64 The build-up of solutes exacerbates fouling by promoting the formation of a cake layer, a phenomenon termed cake-enhanced concentration polarization (CECP), comprising organic, inorganic, or biological foulants, not only acting as a secondary barrier to solute rejection but also enhancing the solute accumulation near the membrane surface while accelerating fouling.65
CP also influences the performance of FO membranes, particularly in terms of fouling and rejection efficiency. This process impacts both the osmotic driving force and the membrane's long-term efficiency. In this context, CP can be categorized into external concentration polarization (ECP)66 and internal concentration polarization (ICP).67 ECP occurs at the surface of the active layer, where solutes accumulate or are diluted, depending on the flow direction, and can be mitigated by effective crossflow or mixing. This phenomenon can be partially controlled through hydrodynamic modifications such as increasing flow velocity or turbulence. However, in long-term applications, it still contributes to fouling by facilitating the deposition of colloids, organic materials, and layers onto the membrane surface, further hindering the performance.68 However, ICP occurs within the porous support layer of asymmetric membranes with a more profound effect on performance, as it reduces the effective osmotic pressure differential, which in turn reduces the water flux.69 The structural characteristics of the support layer, such as tortuosity, thickness, and porosity, are pivotal in controlling the degree of ICP level.69,70 Additionally, reverse solute diffusion, where draw solutes migrate back into the feed solution, can interact with feed impurities to aggravate fouling, compounding the challenges posed by CP.71 Innovations such as electrospun nanofiber supports, which offer low tortuosity, high porosity, and minimal thickness, have demonstrated potentials in ICP reduction and maintaining high water flux over extended operational periods.72,73
3. Literature survey, analysis, and data extraction
3.1. Nanocomposite membranes
Membrane materials are classified into organic (polymeric) and inorganic (ceramic) types. Organic membranes are desirable for water treatment due to their ease of processing, low cost, and flexibility for surface modifications to enhance separation and antifouling properties. However, their major drawback is reduced flux at high temperatures due to polymer chain degradation.74 To address these issues, nanoparticles have been incorporated into polymeric membranes, resulting in superior properties, including reduced fouling and improved performance. Various types of nanocomposite membranes including mixed-matrix, thin-film, and surface-located nanocomposites, which offer enhanced thermal stability, mechanical strength, and overall efficiency in water treatment applications have been developed.75 Generally, the performance of nanocomposite membranes is highly influenced by the properties of the support layer, inner layer, and active layer. The support layer, which provides mechanical strength and structural integrity, should be highly porous and hydrophilic to minimize resistance to water flow and enhance permeability. The inner layer, often a polymeric matrix, is designed to reduce ICP, thereby improving flux and selectivity. The active layer, typically composed of polyamide or other selective polymers, is known for its selective rejection properties. Its thickness, surface morphology, and chemical functionalization determine the membrane's efficiency in rejecting salts and heavy metals while minimizing fouling (Fig. 4). By carefully optimizing these parameters, nanocomposite membranes can achieve enhanced performance in water treatment applications.
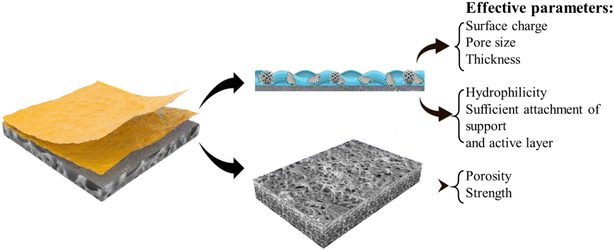 |
| Fig. 4 Schematic structure of thin-film nanocomposite membranes with effective parameters of each layer (reproduced with permission from ref. 76 Elsevier 2021, ref. 77 Elsevier 2020, and ref. 78 RSC 2018). | |
In a recent review, Cheng et al.79 studied the development of polymeric nanocomposite membranes and found that the sodium titanate nanobelt (Na-TNB) membrane showed 97.5% and 57.5% removal of Cs(I) and Sr(I), respectively,80 and (anatase titania/hydrous MgO)@PVC UF mixed matrix membrane (MMM) exhibited 97% and 98% removal of Cu(II) and Cd(II), respectively.81 In another review, Aryanti et al.82 surveyed ultra-low-pressure RO membranes for desalination and revealed that among a range on nanomaterial-incorporated TFN membranes, the polyamide TFC RO membrane prepared using nano-TiO2 showed a promising NaCl rejection up to 99.83% and 2.59 L m−2 h−1 bar−1 permeability,83 while the NaY zeolite-incorporated TFN membrane showed lower NaCl rejection (98.80%) and higher permeability (4.79 L m−2 h−1 bar−1).84 Another literature survey reported by Valamohammadi et al.85 considered the effects of carbon nanotubes (CNTs) on the performance and properties of nanocomposite membranes for wastewater treatment. Screening the reported literature revealed that the highest removal of salts and heavy metals is as follows: Na2SO4 (99%, 6.98 L m−2 h−1 bar−1 permeability) with CNT-enhanced TFN membrane,86 MgCl2 (97.36%, 7.57 L m−2 h−1 bar−1 permeability) with hyperbranched polyethyleneimine-modified multi-walled CTN (MWCNT)-incorporated NF,87 NaCl (98%, 1.50 L m−2 h−1 bar−1 permeability) with zwitterion-functionalized CNT nanocomposite membrane,88 MgSO4 (97.60%, 14.03 L m−2 h−1 bar−1 permeability) with modified hydroxyl-containing MWCNT-incorporated NF,89 as well as Zn(II) (99.06%), Cd(II) (96.72%), Cu(II) (95.84%), Ni(II) (94.63%), and Pb(II) (93.39%) with 7.57 L m−2 h−1 bar−1 permeability using a hyperbranched polyethyleneimine-modified MWCNT-incorporated NF membrane.87 Yu et al.90 reviewed studies reported on TFN membranes with a 2D nanomaterial interlayer. Regarding their literature survey on various pollutants, hierarchical flower-like MoS2-incorporated TFN membrane with >98% removal of Na2SO4 and MgSO4, and 18.3 L m−2 h−1 bar−1 permeability,91 GO-incorporated TFN membrane with >99.7% removal of NaCl and 3 L m−2 h−1 bar−1 permeability,92 and PEI/MOF-incorporated TFN with 95.50% removal of MgCl2 and 4.60 L m−2 h−1 bar−1 permeability93 delineated the highest removals. Here, the effect of GO and its 2D structure on the tortuosity of the pores and the improvement of water channels is prominent. Moreover, charge modification can help ionic removal. The effect of nanoparticles on the mechanical properties of the sublayer has also been reported in some studies.94 Nanoparticles in the membrane structure can affect hydrophilicity as well as the size and tortuosity of membrane pores and channels by intervening the structure formation process.95–97 This and similar studies provide valuable insights into selecting hydrophilic nanomaterials for incorporation into the support, interlayer, or active layer of NC membranes. Integrating these materials can significantly enhance the membrane flux and improve rejection rates through the combined effects of hydrophilicity and charge modification. This strategy effectively regulates membrane charge relative to the charge and size of the ions to be removed, offering a promising route for optimizing NC membrane performance. Proper selection of nanomaterials added to the active PA layer can result in thinner layers, leading to higher water flux values and improving membrane fouling performance.95,96
Table S1† summarizes the most recent studies on desalination and heavy metal removal using NC membranes in the 2023–2024 range. It can be noted that polysulfone (PSF) is one of the most common substrates or support materials due to promising properties including high thermal, mechanical, and hydrolytic strength.98 Besides, poly(m-phenylene isophthalamide),99 poly(vinylidene fluoride)-grafting-poly(acrylic acid),100 poly(vinyl butyral),101 cellulose acetate,102–104 polyamide 66,105 poly vinylidene fluoride,106 cellulose diacetate,107 polyphenylsulfone and polyvinylpyrrolidone,108,109 polyacrylonitrile,95,110,111 polyethylene,96 polyphenylsulfone,112,113 polyurethane,94 polyvinylidene fluoride,114,115 and poly(vinyl alcohol)116 have been used. Regarding Table S1,†Fig. 5(a) and (b) summarize the number of publications focused on salt and heavy metal removal using NC membranes in the 2023–2024 range. Moreover, the highest removals have been reported in these figures. In terms of salt removal, it can be observed that NaCl and Na2SO4 are mainly used as the common salts, while MgSO4 and MgCl2 are in the second place. Fewer studies are also focused on KNO3, CaCl2, and LiCl. According to Table 2, the highest removals of NaCl,114 Na2SO4,96 and MgSO4,95,96 were found to be 99.88, 99.80, and 99.50%, respectively. In terms of heavy metals, Pb(II), Cd(II), and Cu(II) are the most common species as the most abundant heavy metal pollutants in industrial wastewaters, where the highest removals belong to Cu(II),119 Cs(I),120 and As(V)96 with 100%, Zn(II) with 92.23% removal,121 and Cd(II),103 Pb(II),118 and Ni(II)117 with 99% removal.
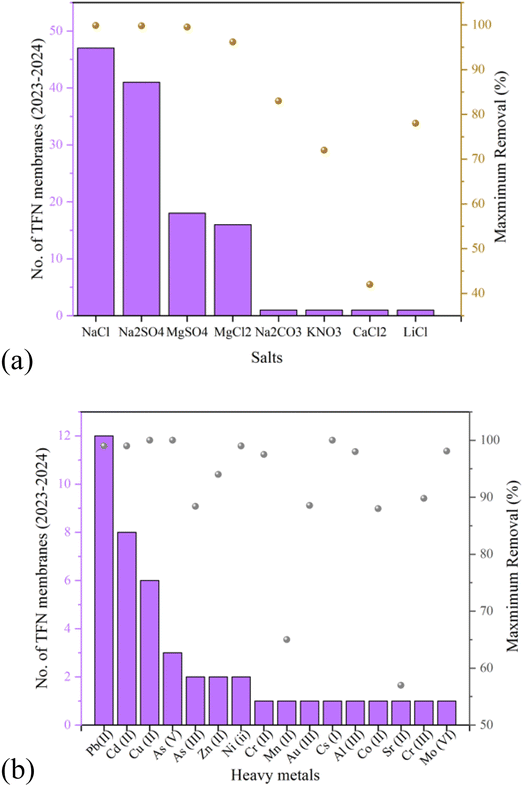 |
| Fig. 5 Most recent publications in the field of (a) salt and (b) heavy metal removal using NC membranes with the maximum removal%. | |
Table 2 Top NC membranes with the most promising salt and heavy metal removal%
Membrane matrix |
Nanoparticle |
Water permeability (L m−2 h−1 bar−1) |
Pollutant |
Removal% |
Ref. |
PVDF |
Perfluoroctylamine-graphene nano-sheets |
∼1.28 |
NaCl |
99.86 |
114
|
PE/PA |
TiO2 |
5 |
Na2SO4 |
99.80 |
96
|
PE/PA |
TiO2 |
5 |
MgSO4 |
99.50 |
96
|
PES |
WO3@GO |
445 |
Ni(II) |
99 |
117
|
PES |
MoO2@GO |
410 |
Ni(II) |
99 |
117
|
PES |
Amine, thiol-SiO2 |
6.70 |
Pb(II) |
99 |
118
|
CA |
Amine–SiO2 |
— |
Cd(II) |
99 |
103
|
PES/PA |
MCM-41 |
4.05 |
Cu(II) |
100 |
119
|
PES/PA |
Titania nanosheet |
1.57 |
Cs(I) |
100 |
120
|
PE/PA |
TiO2 |
5 |
As(V) |
100 |
96
|
Generally, the membrane separation efficiency relies on achieving a delicate balance between high rejection rates and permeability while maintaining low costs, which presents a great challenge in developing a sustainable membrane filtration system. However, literature review and screening highlight that the efforts to enhance removal rates often coincide with a decrease in permeability111,122–124 and vice versa.125,126 Overall, it is verified that nanoparticle incorporation help improve the porosity, hydrophilicity,127 and surface charge. Hence, high salt rejection can be attributed to the enhanced surface charge or dilution effect caused by water permeability enhancement.128,129 The highest improvement in NaCl rejection was observed using the membrane-incorporated PVA/attapulgite nanocomposite95 and MoO2@GO.117 Conversely, the overall removal of Na2SO4 was found to be higher than that of NaCl when using NC membranes. In this context, g-C3N4/CuFe2O4,130 chitosan/MWCNT,131 and rGO@Au111 demonstrated the highest improvement in Na2SO4 removal. In terms of MgSO4, nanoparticles of GO–ZnO122 and TiO2 (ref. 132 and 133) posed a higher impact on removal. Regarding MgCl2 removal, O–MoS2,134 SiO2,135 and (ZnFeCe) layered double hydroxide136 exhibited the most notable surge.
The highest improvement and change in permeability were observed with the addition of LAPONITE®/GO,94 GO,137 and cellulose NC.107 Upon closer analysis of these studies, it was observed that the addition of LAPONITE®/GO resulted in improved porosity and hydrophilicity, consequently enhancing permeability.94 The incorporation of GO into the polymeric hydrogel layer led to the improvement of hydrophilicity and reduction of surface roughness, along with a further decrease in surface charge. These properties combined with a reduction in layer thickness yielded a promising enhancement in water permeability.137 Additionally, the inclusion of high-aspect-ratio cellulose nanocrystals improved the hydrophilicity and water permeability due to the nanorods' percolation-induced nano-channels.107 Moreover, the polyamide layer is often charged and effectively rejects divalent ions and most organic solutes based on the Donnan repulsion and pore sieving approach.138,139 In this context, it was found that some studies have considered the cytotoxicity of the membranes caused by the possible detachment of nanomaterials94 and their entry into the flow, while others have remained silent on the subject.
Regarding Table S1,† it was noted that the addition of 2D nanoparticles to the membrane matrix led to the most promising improvement in permeability and rejection rates. It is reported that the selective mass transport using 2D nanomaterials is attributed to size and Donnan electrostatic exclusion.140 It is suggested that surface electrostatic charges block ions either on the pore edges of the nanopores or on the line edges and surfaces of nanosheets. Table 1 summarizes the size of bare and hydrated ions, which, together with the surface charge and pore size of the membranes documented in Table S1,† further confirm the removal mechanism of the membranes. Besides, other than tuning pore size and surface charge, decreasing membrane thickness can improve membrane permeability through diminishing solute diffusion friction.140
3.2. Thin-film composite (TFC) membranes
Thin-film composite (TFC) membranes stand as the premier technology for pressure-driven RO and NF water treatment processes. The promising separation performance, high water flux, and robust durability make them the leading choice for efficient water purification.141 In a recent review, Kadhom127 discussed the synthesis steps, basics, and alternatives of PA TFC membranes for desalination. They highlighted the cost-effectivity, easy preparation procedure of the active PA layer, and long-term operation of TFC membranes as the most promising advantage, while fouling and low thermal stability have been recognized as the most prominent shortcomings. Park et al.142 studied polyester-based TFC membranes for desalination. They considered polyester as a promising alternative to PA membranes with enhanced water permeability, chlorine resistance, oxidation stability, and efficient separation of multivalent ions. Regarding their survey, CD polyester/DMAP-reconstructed the β-CD-EDA/PAN membrane with 98.75–98.88% NaCl rejection and 4.94–5.33 L m−2 h−1 bar−1 permeability,143 PA TFC membrane with 99.6% of Na2SO4 rejection and 4.2 L m−2 h−1 bar−1 permeability,144 and resorcin 4 arene macrocycle/PSF membrane145 with 94.80% KOH, 93.85% LiOH, and 89.03% NaOH rejections and 8.14, 9.57, and 14.73 L m−2 h−1 bar−1 permeability, respectively, which exhibited the highest salt removal. In another recent review by Sarkar et al.,146 PA nanofilm composite NF membranes with 99.99% Na2SO4 removal and 32.1 L m−2 h−1 bar−1 permeability147 and dual-layer slot coating/polydopamine-coated PE membrane with 99.5% NaCl removal and 2.6 L m−2 h−1 bar−1 permeability148 showed the highest removal rates. Another review by Wu et al.149 reported that the PIP/PSF membrane with 99.7% Na2SO4 rejection and 17.5 L m−2 h−1 bar−1 permeability,150 ZCD-based TFC membrane with 99.8 and 98.9% rejection of CrCl3 and FeCl3, respectively,151 PHMTBA/PSF with 99% rejection of Na2SO4 and 43.1 L m−2 h−1 bar−1 permeability,152 and PVA/PIP/PSF membrane with 97.6% MgSO4 rejection and 35.5 L m−2 h−1 bar−1 permeability153 depicted the highest rejections. The literature survey compiled by Zhang et al.,154 revealed that m-phenylenediamine/trimesoyl chloride/PSF membrane with 99.4% NaCl rejection and 2.96 L m−2 h−1 bar−1 permeability,155 and piperazine/4-hydroxybenzenesulfonic acid sodium salt/PSF membrane with 99.1% Na2SO4 rejection and 34.4 L m−2 h−1 bar−1 permeability156 represented as the most promising membranes with the highest removal. An et al.157 studied in situ modification of NF and RO membranes through the incorporation of thin films and it was verified that the P(NIPAM-co-Am)/TFC with 98.6–98.8% NaCl rejection and ∼5.5 L m−2 h−1 bar−1 permeability158 showed the highest salt rejection. Liu et al.159 studied the effect of interlayer on the TFC performance. It was observed that the MXene/PIP/PSF membrane with 99.9% Na2SO4 rejection and 27.8 L m−2 h−1 bar−1 permeability,160 and MXene/Fe3O4/PS/PIP/PSF membrane with >97% MgCl2 rejection and 9.48 L m−2 h−1 bar−1 permeability161 represented the highest rejection values.
Improving water permeability without compromising the high rejection rate remains a major challenge in membrane-based technologies. To address this, recently, Sun et al.162 have developed TFC membranes using a tannic acid (TA)-MXene interlayer. The straightforward synthesis process involved incorporating tannic acid-functionalized MXene nanosheets onto a polyether sulfone (PES) substrate, followed by the interfacial polymerization of piperazine and trimesoyl chloride (Fig. 6). The resulting membrane demonstrated a 96% rejection rate for divalent ions and a water permeability of 22.3 L m−2 h−1 bar−1. Additionally, the membrane exhibited sustained performance in filtration tests lasting up to 140 h. These promising results indicate that the membrane has potential for high-efficiency applications. Further studies focusing on the fouling behavior and stability of the coated layer would be beneficial to ensure long-term operational stability.
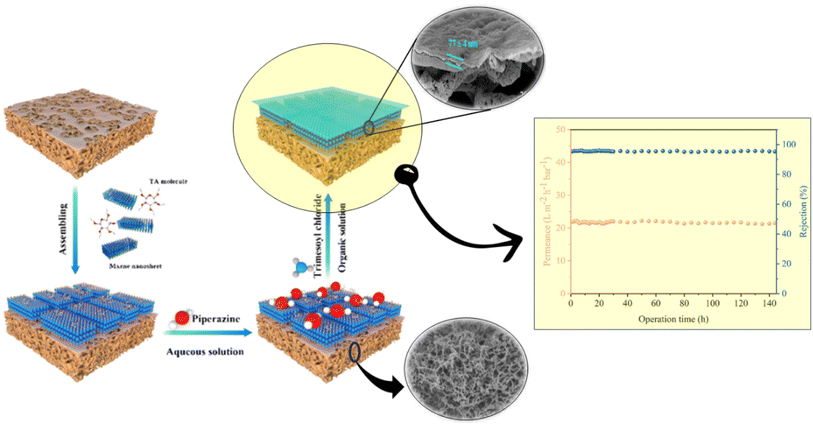 |
| Fig. 6 Process of fabricating an interlayer-enhanced TFC membrane (reproduced with permission from ref. 162, ACS 2024). | |
Table S2† summarizes the most recent studies focused on salt and heavy metal removal using composite membranes in the 2023–2024 range. Regarding the documented results, PSF is mainly used as the substrate, while polyacrylonitrile stands out as the second common substrate. Considering Table S2,†Fig. 7 summarizes the number of publications focused on salt and heavy metal removal using TFC membranes in the 2023–2024 range. Moreover, the highest removals are reported in Fig. 7 and Table 3. Similar to nanocomposite membranes, it can be observed that NaCl and Na2SO4 are the most common salts for such studies, while MgSO4 and MgCl2 are in the second place. Fewer studies are also focused on CaCl2, and LiCl. According to Table S2,† the highest removals of these salts were found to be NaCl with >99%,155,163 Na2SO4 with 99.7%,164 MgSO4 with 99.3%,165 and MgCl2 with 99.7%.166 In terms of heavy metal removal, it was noted that less focus has been devoted to TFC compared to TFN membranes for this purpose. However, the highest improvement/change in permeability was observed for PSF/L-arginine/polyamide,170 PSF/sulfonated GO/polyamide,171 and PSF/UiO-66-SO3H/polyamide,172 while the highest rejection improvement was obtained from GO-PVDF/CS,173 plasma-treated PE/PA/SDS,174 and PSF/PMMA grafted silica.163 Besides, from Table S2,† it was found that not all the reported studies have considered surface roughness, pore size, and surface charge of the membranes and their effect on removal rates. However, almost all of the reported membranes except that reported in ref. 175–178 exhibited a negative charge. Positive-charged membranes performed salt removal through size sieving combined with Donnan exclusion (repulsion), while negative-charged membranes fulfilled the removal via the combination of size sieving with the Donnan exclusion (attraction).
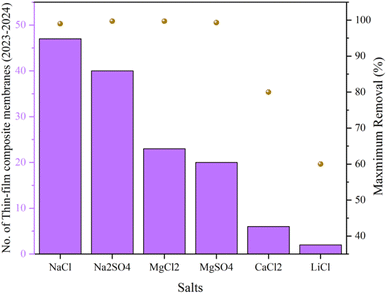 |
| Fig. 7 Most recent publications in the field of salt removal using TFC membranes with the maximum removal%. | |
Table 3 Top TFC membranes with the most promising salt and heavy metal removal%
Membrane matrix |
Nanoparticle |
Water permeability (L m−2 h−1 bar−1) |
Pollutant |
Removal% |
Ref. |
PSF |
Polymethyl acrylate grafted silica nanoparticles |
2–5 |
NaCl |
>99 |
163
|
PES |
PA (piperazine, TMC, 2-acrylamido-2-methyl-1-propanesulfonic acid) |
30.5 |
Na2SO4 |
99.7 |
164
|
PES |
PA (piperazine, 1,3,5-benzenetricarbonyl trichloride) |
15.80 |
MgSO4 |
99.3 |
165
|
PES |
PA (branched PEI, 1,4-phenylene diisocyanate, cyanuric chloride) |
1.25 |
MgCl2 |
99.7 |
166
|
Commercial PA-TFC BWRO |
Poly(acrylic acid)–polyamide |
1.69 |
As(III), As(V) |
96, 99.6 |
167
|
PSF |
Polyamide (polyethyleneimine, terephthaloyl chloride, piperazine) |
11 |
Heavy metals |
98–99 |
168
|
PSF |
Polyamide (m-phenylenediamine, trimesoyl chloride), Fe–TA complex |
3.58 |
Boron |
98.37 |
169
|
3.3. Biomimetic membranes
Biomimetic membranes are typically composed of synthetic materials incorporating key features of biological membranes, such as ionic channels, aquaporins, and selective transport proteins. To this end, potential biomimetic membrane materials include lipid bilayers, aquaporins, and a variety of membrane proteins, which can be integrated into membranes to enhance the permeability, selectivity, and resistance toward fouling.179 Aquaporins (AQPs) are transmembrane proteins found in mammalian and microbial cell membranes, facilitating water transport.180 Integrated into liposomes or polymersomes, they enhance the synthetic membrane performance by increasing both water permeability and selectivity.181 These membranes can be fabricated via assembling aquaporins into lipid membrane layers on a porous support or integrating aquaporin-containing vesicles into a thin-film rejection layer of the membrane.182Fig. 8(a–c) depicts the schematic of biomimetic membranes with sub-nanometer channels made by either aquaporin or synthetic nanochannels. Beyond their role as selective agents in membranes, aquaporins offer valuable insights into the synthetic channel design, showcasing an improved transport performance.186,187 While aquaporins and other protein nanochannels can be synthesized using established bioprocessing techniques, they are prone to denaturation and loss of functionality, and the channels often face stability challenges during membrane synthesis and utilization and may not offer the optimal pore-loading efficiency and functionality. Furthermore, synthetic bioinspired channels can address the instability and poor processability issues inherent in their biological counterparts. Porter et al.187 investigated the challenges and advancements in biomimetic desalination membranes. They identified aquaporins such as human Aqp1 and bacterial AqpZ as promising biological channels. Additionally, the study highlighted several innovative synthetic channels including cyclic peptide nanotubes, carbon nanotube porins, imidazole-derived quartet channels, pillararene-based channels, and aquafoldamer-based synthetic water channels as promising alternatives for improved desalination performance.
 |
| Fig. 8 (a) Biomimetic desalination membranes (reproduced with permission from ref. 183, Elsevier 2021), (b) structure of the lipid bilayer membrane incorporating aquaporins with covalent bond (reproduced with permission from ref. 184, RSC 2015), and (c) common nanochannels for desalination from biological aquaporin and gramicidin to bioinspired channels (reproduced with permission from ref. 185, Elsevier 2014). | |
Regarding the literature review and screening, it was noted that in the last year minor attention has been paid to the utilization of biomimetic membranes for desalination and heavy metal removal. Moreover, as expected, TFC and TFN membranes have been widely used for this purpose showing promising results. In 2023, Azarafza et al.188 considered protein-based membranes fabricated using aquaporin for desalination and separation purpose. They characterized these membranes by their high osmotic water permeability and excellent ability for small molecules removal. Based on their literature survey, PMOXA-PDMS-PMOXA/PCTE189 and AQP-DOPC/PSF181 membranes with 99% removal of NaCl, DOPC-PDA-PEI/PAI190 and POPC-POPG-Chol/bilayer polyelectrolyte-coated H-PANI UF191 membranes with 95% removal of MgCl2, and DOPC/DOTAP/liposome-embedded TFC with 95.9% MgCl2, 94.5% MgSO4, and 88.5% Na2SO4 rejections192 exhibited the highest removal rates. Table S3† summarizes the last published papers in the 2023–2024 period. Regarding the documented results, it can be observed that the overall salt and heavy metal removal of biomimetic membranes was found to be less than that of TFC and TFN membranes, while NaCl was the most common salt considered for treatment using biomimetic membranes. The analysis of Table S3† reveals that the PAN-PA-peptoid membrane exhibited the highest NaCl removal of 99.5%.193
One of the central challenges in fabricating biomimetic membranes is the integration of biological components into the membrane structure.188 The substrates for biomimetic membranes must provide mechanical strength, chemical stability, and biocompatibility while being compatible with protein compounds.194 For instance, the utilization of aquaporins necessitates stabilizing these proteins as functional groups, ensuring structural integrity under operational conditions.195 Various techniques such as vesicle fusion, interfacial polymerization, and covalent attachment have been employed to achieve this, each addressing specific hurdles such as protein denaturation and membrane fouling.188,194,196 Advanced polymeric materials, often modified with hydrophilic or zwitterionic groups, are increasingly employed to generate the desired interface.197,198
The realization of defect-free, cohesive, and industrial-scale production remains a formidable challenge, particularly in terms of aligning nanoscale biological features via macroscopic fabrication processes.199 Recent innovations have been sought to overcome these barriers. For instance, the incorporation of aquaporin proteins into TFC membranes has led to improved desalination efficiency, combining high water flux along with significant salt rejection.200 Innovations in nanocomposite materials such as integrating block copolymers with engineered channels have resulted in membranes exhibiting improved stability and reduced fouling tendencies. Nevertheless, achieving consistency in membrane performance during scale-up continues as a challenge.196,201
Ensuring the functional integrity of aquaporins into membranes during extraction and incorporation into the membranes is critical. Traditional lipid bilayers, while effective in mimicking the aquaporin natural environment, lack the mechanical robustness and stability required for industrial applications. Consequently, synthetic amphiphilic block copolymers have emerged as more viable alternatives, offering superior durability and flexibility.202 However, the compatibility of these polymers with aquaporins and the precision required in forming defect-free membranes pose additional complexities. The delicate balance of maintaining the native functionality of aquaporins while being integrated into a stable, scalable synthetic matrix remains a formidable barrier.203 Techniques such as vesicle embedding, interfacial polymerization, and chemical crosslinking have been developed to immobilize aquaporins effectively. However, these methods often encounter difficulties in the realization of defect-free, large-scale membranes.196,201,202
Recent advancements in artificial water channels (AWCs) offer a promising alternative, replicating the selectivity and efficiency of biological water channels through synthetic means. AWCs, such as carbon nanotube porins and imidazole-based channels, have shown comparable performance to aquaporins in laboratory settings. These structures are engineered to maintain molecular-scale precision, enhancing water permeability while preventing ion transport. However, replicating the precise structural and functional attributes of natural channels remains a concern.199
The path toward the widespread adoption of biomimetic membranes in practical applications is under considerations. The synthesis of biomimetic membranes involves a multi-step process, remaining as a unique technical obstacle.196 First, the production of aquaporins, either through natural extraction or recombinant techniques, is constrained by low yield, instability, and the hydrophobic nature. While advancements in recombinant protein technology have enabled higher yields, production remains costly and time intensive. Continued research into scalable fabrication techniques, such as roll-to-roll processing and advanced polymer blending, is essential for transitioning these membranes from experimental stages to industrially viable visions. Additionally, exploring hybrid designs merging the advantages of biological and artificial channels may pave the way for more robust and versatile systems.199 Another promising avenue is the hybrid integration of aquaporins with nanomaterials such as GO and CNT, which enhance the mechanical properties and fouling resistance of the membranes. These hybrid systems harness the strengths of both biological and synthetic components, providing a pathway toward more durable and efficient water purification systems. Furthermore, the use of advanced computational modeling and machine learning is enabling the optimization of membrane design at the molecular level, accelerating the development of next-generation biomimetic membranes.204–206 Additionally, the economic feasibility of large-scale production and deployment must be addressed for laboratory-to-industry transition of these technologies.188,207
3.4. Forward osmosis (FO) membranes
To address the limitations of conventional RO, forward osmosis (FO) technology has been proposed for seawater desalination.208 While FO offers the advantage of reduced energy consumption and appears well-suited for applications in arid regions where conventional energy sources may be scarce or costly, it confronts several significant challenges. Foremost among these challenges is the selection of an appropriate draw solute type and concentration. The draw solute must generate adequate transmembrane pressure to facilitate water transport while minimizing reverse salt flux, which can compromise process efficiency. Additionally, the choice of the membrane material is critical; it must exhibit high flux values to maximize water throughput while maintaining a low reverse solute flux to prevent contamination of the feed solution. Furthermore, the orientation of the membrane's active layer whether facing the draw solution or the feed solution poses a crucial consideration, as it can influence overall system performance. Moreover, the behavior of the membrane when exposed to feed solutions of different concentrations is a key area of concern. Understanding membrane performance, defined by water flux and reverse solute flux, is crucial for optimizing FO processes across diverse applications and environmental conditions.209 Addressing these challenges is imperative for unlocking the full potential of FO technology and expanding its practical applicability in diverse settings.
To date, substantial research has been devoted to improving the efficiency, reliability, and overall performance of FO technology. Abounahia et al.209 reviewed the commercially available FO membrane characteristics and the development of laboratory scale fabricated membranes based on cellulose triacetate and TFN membranes together with various draw agents and their effects on FO performance. The study highlighted that asymmetric membranes often underperform in FO processes. Moreover, the characteristics of the draw solute are critical, as ICP can lead to a reverse solute flux and a reduced water flux if the draw solute concentration decreases within the dense support layer. A promising approach to mitigate these issues involves utilization of highly porous, thin, and water-permeable support to enhance permeability. The ideal FO membrane should exhibit low ICP, high permeance, antifouling properties, chemical stability, sustained mechanical strength, and minimal reverse solute flux.210,211
In the pursuit of finding an ideal FO membrane, Abdul-Hussein et al.212 reviewed the most recent design and deployment of FO desalination technologies, their applications, and the remaining challenges and prospects. In this review, cellulose acetate, polyamide, and TFC membranes are considered as the common FO membranes for seawater desalination. NaCl and MgSO4 are highlighted as the primary minerals that can be effectively removed through the FO process. For high-salinity feed solutions, the state-of-the-art draw solutions including sodium sulfate, sodium chloride/magnesium chloride mixtures, calcium chloride, potassium phosphate, ammonia–carbon dioxide, polyethylene glycol, lithium chloride, and sodium alginate were recommended. In Ibraheem et al.'s213 review and screening, among a range of nanoparticles used for TFC membrane fabrication, including GO, TiO2, zeolite, SiO2, ZnO, Al2O3, Fe3O4, and MOF, the FO membrane made by PSF as support and MPD/TMC/GO as polyamide active layer with NaCl as a draw solute exhibited the highest NaCl removal of 98.71%,214 and FO membrane consisting of PSF/PEG/NMP support and MPD/GO as the active layer with NaCl as the draw solute showed the highest Pb, Cd, and Cr removal of 99.9, 99.7, and 98.3%, respectively.215 Reddy et al.216 reviewed the design, synthesis, and application of thermally responsive draw solutes used in the FO system. This paper considered ionic liquids (ILs) and hydrogels as advanced thermally responsive draw solutes. Kanagaraj et al.217 studied the TFC and TFN membranes used in the FO system. The results confirmed that the surface hydrophilicity, roughness, porosity, water permeation, solute retention, reverse solute permeation (RSP), and specific reverse salt permeation (SRSP) were significantly influenced by the alteration of the skin layer structure in FO membranes. Besides, TFN membranes demonstrated enhanced salt rejection and FO water flux, along with superior fouling resistance properties. Piash and Sanyal218 reviewed the strategies developed for FO support layer design. Among the screened membranes, the Al2O3/PSF membrane with 1 M of NaCl as the draw solute,219 Oasys TFC flat sheet membrane,220 sPPSU membrane with 2 M of NaCl as the draw solute,221 and PSF/PAN membrane with 1 M of NaCl as the draw solute222 showed the highest permeability of 8.43, 4.25, 3.7 and 3.68 L m−2 h−1 bar−1, respectively. Kallem et al.223 reviewed and screened the recent developments in electrospun nanofiber-based substrates used for the fabrication of composite membranes in the FO system. Documented data verified that PVDF–SiO2 depicted the highest salt removal of 99.7% (ref. 224) and PDA-modified electrospun nanofiber mat225 exhibited the complete removal of Zn(II), Fe(III), and Cr(VI). Tharayil et al.226 analyzed the current research trend on the FO system used in various fields including desalination, fertigation, pharmaceutical, dye and textile industries, algae dewatering, and diary processing. In terms of desalination, the TFN membrane with p-aramid nanofibers (ANFs) and sucrose solution as the draw solute showed the highest salt rejection of 98.6% (ref. 227) and NIPS TFN membrane215 exhibited the highest removal of 99.9, 99.7, and 98.3% for Pb, Cd, and Cr.
Selecting the appropriate draw solute is a critical challenge in FO. Extensive research has been dedicated to identifying novel draw solutes that can generate high transmembrane pressures while minimizing the reverse salt flux. Recent investigations have explored various promising alternatives including ILs,228–232 deep eutectic solvents (DES),233 magnetic nanoparticles (MNPs) coated with polymers,234 thermo-responsive nonionic amphiphilic copolymers,235 sugars,236 polyelectrolytes,237,238 hydrogels,239 surfactants,240–242 and carbon quantum dots (CQDs).243 Despite these advancements, many studies continue to evaluate the efficacy of FO membranes using 1 M NaCl as a standard draw solution, while novel draw solutes are often tested with commercial membranes. This highlights the need for more comprehensive evaluations that pair innovative draw solutes with specifically designed FO membranes to fully assess their potential and optimize FO performance.
The improvement of FO membrane characteristics remains an active area of research. Strategies to enhance the membrane performance often involve adjusting the hydrophilicity of various membrane layers. PES, PSF, and cellulose acetate (CA) are the primary polymers as the substrate, while PA serves as the top layer. Various approaches have been implemented to address these challenges. One promising method is the support-free preparation of the PA selective layer with a tunable thickness, combined with a highly porous support, to mitigate ICP as a major source of flux reduction.244–247 Additionally, the introduction of interlayers into composite structures has been proposed to minimize the structural parameter by creating tortuous paths, thereby reducing ICP. These interlayers include materials such as alginate hydrogel@MXene,248 cellulose nanocrystal (CNC),249 PVA–glutaraldehyde (GA) hydrogel,250 PDA/PEI/UiO-66,251 PDA/GO-Fe3+-TA,252 SA/UiO-66-NH2,253 chitosan/tannic acid,254 MoS2@NH2-UiO-66,255 alginate/Ca(II),256 aluminum tetra-(4-carboxyphenyl)porphyrin (Al-MOF),257 and polydopamine/GO.258 These materials enhance the membrane performance by reducing ICP and improving overall flux and solute rejection. By continuously refining these strategies and developing new materials, the efficiency and effectiveness of FO membranes can be significantly improved, paving the way for more practical and scalable applications.
The addition of hydrophilic nanostructures to the membrane support or the active layer such as sulfonated graphene oxide (SGO),259 CuBTC MOF,260 MAX phase (Ti3AlC2),261 NH2-GOQDs,262 PAM-grafted ZnO,263 ZnO,264 poly(sulfobetaine methacrylate) (PSBMA)-cellulose nanofibers (CNFs),265 NCQDs,266,267 MIL-53(Fe),268 covalent organic frameworks (COF) modified with TA,269 polyoxometalate-LDH,270 APTMS-TiO2,271 PAMAM-MNPs,234 and PEI:rGO/PDA,236 is proposed to obtain high water flux and retention rate. ICP reduction is also targeted by the integration of hydrophilic fillers such as Ag@NH2-UiO-66,272 MIL-53(Fe)@γ-Al2O3,273 and WS2-Cys-MOF nano-sheets274 by introducing water specific channels and antibacterial property into the molecular structure of the selective layer matrix. Antifouling and anti-biofouling characteristics comprise another approach, which can be achieved by the addition of photocatalytic nanostructures such as MoS2/PDIsm hetero-structured photo-catalysts,275 anatase TiO2,276 MoS2–Ag,277 and MoS2@zeolite X278 to the selective layer. Surfactant-induced intervention in interfacial polymerization using hexadecyltrimethylammonium toluene-p-sulphonate (CTAT) and the amine aqueous solution279 is also implemented to obtain highly permeable TFC membranes with promising selectivity. One promising approach to enhance water treatment performance is reported by Sun et al.,280 where a TFN PA layer was coated over the ceramic substrate using in situ-grown Zr-MOF (UiO-66-NH2) as the interlayer (Fig. 9). The results revealed that the interlayer decreased the film thickness, improved the cross-linking degree, enhanced the surface roughness, and resulted in an improved water flux (27.38 L m−2 h−1) with less reverse salt flux (3.45 g m−2 h−1). Besides, promising ion rejection (>94%) was observed using the as-prepared membranes.
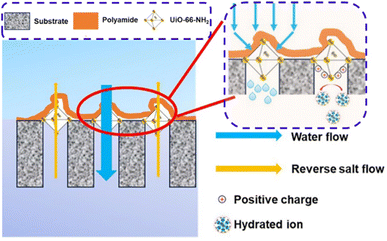 |
| Fig. 9 PA/Zr-MOF (UiO-66-NH2)/ceramic-based FO membrane (reprinted with permission from ref. 280, Elsevier 2024). | |
Although salt rejection and removal efficiency are crucial parameters for evaluating FO membranes, many studies have prioritized reporting high water flux and low reverse solute flux. High water flux indicates the efficiency of the membrane in permeating water, enhancing throughput and reducing costs. Low reverse solute flux, however, is crucial to prevent contamination and maintain the osmotic pressure gradient, ensuring the process efficiency and cost-effectiveness. Given these considerations, the top-performing membranes are selected based on their higher water flux and lower reverse solute flux and summarized in Table 4.
Table 4 Top FO membranes with the most promising salt and heavy metal removal%
FO membrane material |
Draw solute |
DI water flux (L m−2 h−1) |
Solution water flux (L m−2 h−1)/specific salt flux (g L−1) |
Reverse salt flux (g m−2 h−1) |
Ref. |
CTA and CA FO |
NaCl |
— |
1464.21–1627.12 |
164.79–870.44 |
281
|
Chitosan nanofibers/PA |
NaCl |
— |
95 |
∼7 |
282
|
107.53 |
∼28 |
125 |
∼37 |
131.13 |
∼42 |
PES-PA aquaporin TFC FO (A/S, Denmark) |
Zinc sulfate |
6.5 |
6.5 |
4 |
283
|
6.5 |
11.5 |
4.5 |
283
|
8.2 |
8.1 |
4.6 |
283
|
8.2 |
14 |
5 |
283
|
8.8 |
8.8 |
5.8 |
283
|
8.8 |
21.5 |
6.6 |
283
|
MWCNT10-PVA/PA |
NaCl |
— |
∼14 |
∼3 |
245
|
MWCNT20-PVA/PA |
∼10.3 |
∼2.5 |
245
|
MWCNT30-PVA/PA |
∼8.5 |
∼2.7 |
245
|
pCNT5-PVA/PA |
∼21 |
∼4 |
245
|
pCNT10-PVA/PA |
∼15.7 |
∼2.5 |
245
|
pCNT20-PVA/PA |
∼13 |
∼2.3 |
245
|
pCNT30-PVA/PA |
∼10.5 |
∼2.2 |
245
|
PVA1pCNT3-PA |
∼22.5 |
∼6.75 |
245
|
PVA1pCNT5-PA |
∼21.5 |
∼7.3 |
245
|
PVA1pCNT10-PA |
∼16 |
∼4 |
245
|
PVA0.25pCNT3-PA |
22.57 (0.45) |
10.12 |
245
|
PVA0.25pCNT3-PA |
30.16 (0.3) |
9.34 |
245
|
PVA0.25pCNT3-PA |
55.53 (0.46) |
25.37 |
245
|
PVA0.5pCNT3-PA |
∼27 |
∼6.5 |
245
|
PVA2pCNT3-PA |
17.47 |
∼7.2 |
245
|
3.5. Energy requirement
Using osmotic pressure gradients to transport water across a semi-permeable membrane, FO offers potentially more energy efficiency than RO, which relies on high pressures.284,285 However, understanding the energy dynamics of FO, particularly the draw solution regeneration requirement to recover fresh water, is crucial for evaluating its feasibility and scalability within the integrated systems.286 While the osmotic pressure gradient eliminates the need for external pressure application, the energy-intensive draw solution regeneration step can offset these savings if not optimized. The reports indicated that FO can operate with energy requirements of almost 0.25 kW h m−3, significantly lower than the typical 5 kW h m−3 required for RO.287–289 This represents an energy reduction of up to 80% for the separation. Comparatively, thermal desalination processes such as multi-stage flash (MSF) demand even higher energy inputs, often exceeding 10 kW h m−3, making FO particularly attractive in scenarios where energy is expensive.287
However, the energy profile of FO must also account for the method and efficiency of draw solution regeneration. The regeneration of draw solutions can be achieved through various methods including RO, NF, or thermal processes, each with distinct energy profiles. For instance, studies highlight that energy consumption in FO coupled with membrane distillation or NF ranges between 0.6 and 2.5 kW h m−3 of produced water, depending on the feedwater salinity and operational conditions.290,291 These values often place FO systems competitive with RO, particularly for brackish water desalination, where FO benefits from reduced fouling and maintenance demands. For example, NH3 and CO2 (ref. 292–294)-based draw solutions are thermally regenerated, demanding ∼2–3 kW h m−3. This brings the total energy consumption closer to 3–5 kW h m−3, depending on operational parameters and specific system designs.295–297 In contrast, magnetic nanoparticle-based draw solutions, which can be regenerated using external magnetic fields, show promise for reducing energy costs, although their scalability is challenging.208
Studies have demonstrated that advanced draw solutes, such as multi-functional ones can also optimize regeneration energy, achieving osmotic pressures >3000 mOsm per kg with manageable recovery requirements.298 The FO process inherent energy efficiency is further amplified in hybrid systems combining FO and RO. In such configurations, FO is used for feed-water pre-concentrate, reducing the osmotic pressure gap the RO system must overcome. Hybrid FO-RO systems have demonstrated energy reductions of up to 25% compared to standalone RO, with the overall consumption decline of ∼1.5 kW h m−3.295,299 This approach not only optimizes energy consumption but also mitigates the fouling and scaling issues that typically plague high-pressure systems, enhancing long-term operational efficiency and reducing maintenance costs.297 Unlike pressure-driven processes, FO benefits from reversible fouling behavior due to the absence of significant hydraulic forces on the membrane surface. This reduces the frequency and cost of membrane cleaning and replacement, further improving its lifecycle cost-effectiveness. Various structures of FO, TFC or double-skinned membranes have also demonstrated enhanced performance, achieving water flux rates of 10–20 L m−2 h−1 under typical conditions, depending on the draw solution and feedwater salinity.296,300
3.6. Hybrid organic–inorganic membranes
Hybrid organic–inorganic membranes represent a promising advancement in the field of desalination and heavy metal removal. By synergistically integrating the advantageous properties of both organic polymers and inorganic materials, these membranes deliver superior performance, enhanced stability, and resistance to fouling. It is suggested that hybrid adsorptive membranes outperform conventional polymer membranes in removing pollutants from aqueous media. These advanced membranes facilitate rapid solute transport by replacing molecular diffusion mechanisms with high filtration rate systems, thereby improving the overall performance in water purification applications.301 In terms of FO system, studies have confirmed that organic–inorganic hybrid nanocomposite membranes exhibit substantially higher water flux, mechanical strength, chemical stability, selectivity, and hydrophilicity than the conventional polymeric FO membranes.302 The incorporation of inorganic nanomaterials into FO membranes aims to optimize the membrane structure, reduce ICP, mitigate fouling, and overcome performance trade-offs. Enhanced membrane performance results from the interactions between nanomaterials and polymer molecular linkers, along with the functional groups present on the nanomaterial surfaces.303 In Song et al.'s study,304 high-performance double-skinned FO membranes were developed with promising solute rejection via the incorporation of polydopamine and CNTs through interfacial polymerization in the active layer. The results underscored the significant influence of CNTs on the membrane characteristics and the hybrid membranes exhibited a higher FO water flux and remarkable antifouling capacity compared to the conventional membranes. In 2017, Sun et al.302 reviewed the organic–inorganic nanocomposite FO membranes fabricated using CNTs, GO, halloysite nanotubes, as well as TiO2, silica, and Ag nanoparticles. Besides, the effect of modification methods on the FO performance was studied. Studies revealed that although there have been notable improvements in performance, achieving practical applications and commercialization remains a challenge owing to the high cost of nanomaterials, non-homogeneous dispersion by aggregation, weak bonds between membrane matrix and the nanoparticle, long-term stability, etc. Despite the diverse research focus in this area, there remains a noticeable scarcity of comprehensive review papers that systematically analyze and consolidate the advancements in hybrid materials for water treatment.
The long-term stability and cost-effectiveness of hybrid membranes are pivotal in the advancement of desalination technologies. Hybrid membranes integrating materials such as mesoporous silica and organo-silica as common samples have demonstrated promising results in terms of durability, operational stability, and economic viability. For example, a study evaluating silica-P123 membranes for brackish water desalination highlighted their excellent robustness and salt rejection, achieving >99% rejection while maintaining structural integrity over prolonged utilization.305 The hybrid organo-silica species, in particular, displayed superior hydrostability due to reduced silanol content, a critical factor in maintaining membrane performance under harsh conditions.306 Similarly, the integration of UF and NF in hybrid systems for brackish water treatment, as tested in remote Australian national parks, underscores the viability of hybrid membranes in resource-limited environments. These systems effectively managed high turbidity and salinity levels, ensuring water quality while operating under variable energy inputs such as solar power. Specific energy consumption was maintained below 5 W h L−1, showcasing their efficiency.307 Large-scale implementations of hybrid systems further illustrate their cost-effectiveness. In regions such as the Middle East, where water scarcity is acute, hybrid RO-multistage flash (MSF) systems have been successfully deployed, achieving significant reductions in freshwater production costs. These systems optimize energy use and enhance water recovery rates, addressing key limitations of standalone membrane or thermal technologies.308,309
The integration of organic and inorganic materials in hybrid membranes offers a balance between performance and cost, with substantial cost reductions compared to pure inorganic membranes. Organic membranes, primarily polymeric, are known for their flexibility, cost-effectiveness, and ease of fabrication.310 In contrast, inorganic membranes, particularly ceramic-based ones, exhibit exceptional mechanical, thermal, and chemical stability, albeit at significantly higher costs, ranging from 500 to 3000 $ per m2 (ref. 311 and 312) compared to 20–200 $ per m2 for polymeric membranes.313,314 Hybrid membranes achieve cost-effectiveness by addressing the limitations of individual components. For instance, ceramic membranes fabricated from natural minerals or industrial waste materials can reduce material costs to 2–130 $ per m2.315 By combining these with polymeric materials, hybrid membranes can achieve enhanced performance at a fraction of the cost associated with pure ceramic membranes. For example, hybrid membranes such as those combining a low-cost Daramic framework (31.52 $ per m2) with Nafion resin achieved a cost of <50% of pure Nafion membranes. However, it is important to note that not all reported studies have considered the price of the membranes or conducted a detailed techno-economic analysis.316
The most recent organic–inorganic hybrid membranes are summarized in Table S5.† Among them, Table 5 highlights the membranes that outperform others in terms of high salt and heavy metal removal percentages. The analysis of these tables reveals a predominant focus on the use of hybrid organic–inorganic membranes for heavy metal removal compared to salt removal in recent studies. This trend probably reflects the specific affinity and effectiveness of hybrid membranes in capturing heavy metal ions through tailored surface functionalization and enhanced adsorption mechanisms.
Table 5 Top hybrid organic–inorganic membranes with the most promising salt and heavy metal removal%
Membrane matrix |
Feature |
Pollutant |
Permeability (L m−2 h−1 bar−1) |
Rejection (%) |
Ref. |
Graphene oxide hybrid membrane-Fe3O4@amyloid fibrils nanoclusters intercalated into the GO sheets |
Heavy metal removal |
As(III), Pb(II), Cd(II), Hg(II), Cu(II), Zn(II), Ni(II), Co(II) and Cr(III) |
— |
>99.9 |
317
|
MIL-101(Cr) and MIL-101(Cr)@GO doped PVA |
Desalination by pervaporation |
NaCl |
9.7 kg m−2 h−1 |
>99.99 |
318
|
SiO2/PVDF-TMC |
Membrane distillation |
NaCl |
16.08 kg m−2·h |
>99.9 |
319
|
PVDF/SMA@ polyvinylamine (PVAM)-tannic acid (TA)-metal ion |
High permselectivity |
Mg(II), Ca(II) |
53.4 |
>99 |
320
|
Carboxyl functionalization of hybrid organosilica RO |
Desalination |
NaCl, MgCl2, MgSO4 |
∼3.5, 3.2, 3 (×10−13 m3 m−2 s Pa) |
∼97–99 |
321
|
Graphene QDs doped PVA hybrid membranes |
Desalination via pervaporation |
NaCl |
17.09 kg m−2 h−1 |
>99.6 |
322
|
PES-PTL |
Antifouling properties |
Cu(II), Ni(II), Cd(II), Pb(II) |
39.5 |
>99 |
323
|
TA-ZIF-8 |
Improved permeation |
NaCl |
56 L m−2 h−1 |
∼99 |
324
|
PES-Na-A zeolite/oxycut residue NF |
Cr(III) removal |
Cr(III) |
— |
98 |
325
|
Covalent organic framework (COF) membrane |
Na+/Mg2+ permselectivity |
Na+, Mg(II), Cl− |
Na(I) permeance 13 × 105–14.2 × 105 mol m−2 h−1 |
Mg(II) 85–97 |
326
|
One of the most critical hurdles lies in achieving the uniform dispersion of inorganic fillers within the polymeric matrix. Poor dispersion often leads to nanoparticle aggregation, resulting in non-uniform material distribution that adverse impacts the mechanical strength and functional performance of the membranes. Mendes-Felipe et al.327 emphasized that ensuring compatibility between the polymeric matrix and the inorganic nanoparticles, such as TiO2 or MOFs, frequently requires surface modification of the fillers to improve interfacial bonding. For instance, MXenes, characterized by high surface area, tunable functional groups, and exceptional electrical conductivity, are prone to restacking or oxidation under ambient conditions, diminishing the availability of active sites which compromise mechanical flexibility.328,329 To address these issues, researchers have employed surface engineering techniques such as functionalization of MXenes by hydrophilic/phobic groups or intercalating molecules to prevent restacking. These modifications enhance chemical interactions between MXenes and polymer matrices, resulting in more stable and efficient hybrid membranes.330–332
Similarly, nanocellulose-based hybrid membranes offer an environmentally sustainable alternative by enhancing the renewable and biodegradable properties of cellulose nanomaterials. Cellulose nanocrystals (CNCs) and cellulose nanofibers (CNFs) provide exceptional mechanical strength and hydrophilicity, significantly enhancing the antifouling properties and water flux of hybrid membranes. However, the inherently hydrophilic nature poses integration challenges when combined with hydrophobic polymer matrices, often leading to phase separation or compromised structural integrity.328 To address this, surface modifications such as acetylation or the grafting by functional groups have been implemented to improve the compatibility of nanocelluloses with the polymeric matrix.333,334 These strategies not only facilitate uniform dispersion but also enable the development of membranes with tailored functionalities, such as enhanced selectivity or antimicrobial properties.
Another innovative direction involves the incorporation of metallic or photocatalytic nanoparticles, such as Ag and ZnO, into hybrid membranes, providing antimicrobial activity and photocatalytic degradation of pollutants. For example, Ag nanoparticles have been widely utilized to impart antibacterial properties to ref. 335, while TiO2 nanoparticles induce photocatalytic activity under UV.336 However, challenges such as nanoparticle leaching, secondary contamination, and limited stability under harsh operational conditions must be addressed to ensure long-term effectiveness and environmental safety.329
Recent advancements in fabrication techniques have also been instrumental in overcoming the challenges. Approaches such as interfacial polymerization, electrospinning, and phase inversion have enabled precise control over membrane structure and the distribution of additives within the matrix. Interfacial polymerization, for example, has been employed to fabricate TFC membranes characterized by exceptional uniformity and functional integration. Similarly, electrospinning has facilitated the fabrication of membranes with hierarchical pore structures, significantly enhancing permeability and selectivity. Additionally, layer-by-layer assembly has emerged as a promising method for designing hybrid membranes with tailored functionalities such as pH-responsive or stimuli-sensitive separation features.328,329
Another challenge lies in maintaining the structural integrity and mechanical robustness of hybrid membranes, especially in higher-filler-content condition. High filler loads, although beneficial for improving catalytic or adsorptive properties, often compromise the flexibility and durability of the membrane. The development of novel polymeric matrices such as PES or PVDF, known for their inherent mechanical strength and thermal stability, has shown promise in overcoming these limitations. In this context, researchers have suggested strategies such as cross-linking or blending different polymers to achieve an optimal balance between flexibility and rigidity.337
Scalability is another hurdle in the commercialization of hybrid membranes. While laboratory-scale studies have demonstrated their potential, scaling up to industrial production often encounters issues such as reproducibility, cost-effectiveness, and material stability. For instance, the fabrication of MXene-based membranes involves intricate etching and intercalation processes not easily adaptable for large-scale manufacturing. Similarly, the use of high-cost precursors, such as noble metal nanoparticles, poses economic challenges limiting widespread adoption. Addressing these issues requires innovations in synthesis methods, such as greener and more cost-effective fabrication routes, as well as the development of robust and scalable production systems.328,329
4. Comparison and discussion
The application of membrane-based technologies in water treatment has gained substantial traction due to their efficiency in removing contaminants such as salts and heavy metals. These technologies have revolutionized water purification processes, providing high selectivity and permeability. However, despite their widespread use and established benefits, significant challenges and gaps persist within each type of membrane that must be addressed to further enhance their performance and broaden their applicability.
4.1. Removal efficiency
Traditional RO and FO membrane technologies have undergone extensive evaluation for eliminating trace contaminants from wastewater.338 The significantly low content of pollutants necessitates high pressure in RO processes for an effective removal, rendering such operations economically unfeasible in many regions. Furthermore, FO membranes with low operational pressure requirement demonstrate limited efficacy in rejecting small neutral organics.339 Biomimetic membranes present a more cost-effective solution for eliminating trace organic contaminants due to their ability to enhance freshwater permeability and accommodate low-pressure operations. In terms of aquaporin-based biomimetic membranes as promising candidates in this category, the main challenge would be the extraction of aquaporin and fabrication of related biomimetic membrane, offering durability and long-term stability against saline water and cleaning procedure. Besides, the upscaling of these membranes is a challenge due to not commercially available aquaporin, difficult production procedure, and compatibility requirement of the host membrane with the protein, which limits the options.340,341
4.2. Permeability
The substrate plays a crucial role in membrane permeability. An optimal substrate should exhibit hydrophilicity to ensure high water flux. However, excessively high substrate hydrophilicity can diminish adhesion between the active layer and the substrate. Besides, substrates with significant variations in pore size may lead to uneven active layer thickness due to preferential penetration of the polymer solution into larger pores compared to smaller ones.342,343 The selection of the right substrate involves ensuring high flux, compatibility for uniformity and defect-free performance, and strong adhesion within the active layer. As expected, the incorporation of nanomaterials in various membranes systems, whether in RO or FO systems, has significantly improved water permeability and desalination rates. Among a range of nanomaterials, carbon-based ones, in particular, have been extensively researched. However, these materials are not optimal for commercial use since their expensive manufacturing processes hinder large-scale production and economic feasibility in desalination. There is often a trade-off between membrane permeability and selectivity, with improvements in one typically resulting in compromises in the other. However, membranes incorporating nanostructured materials such as mixed-matrix nanocomposite membranes (MMNMs) and TFN have shown exceptional properties. These nanostructured membranes offer synergistic benefits, including enhanced permeability, elevated rejection rates, high thermal and mechanical stability, and antifouling/biofouling characteristics, making them highly effective for water treatment applications. However, the primary challenge in this context lies in uniformly dispersing inorganic nanoparticles within the polymer matrix. Aggregation is a frequent issue, which obstructs the consistent distribution of nanomaterials throughout the polymer structure.344 Selecting the best filler for nanocomposite membranes is a challenge due to improving specific membrane features while negatively affecting others. Therefore, based on the desired characteristics of the outflow, and considering the stability, durability, and cost of the membrane, the optimal nanocomposite can be selected to improve desired properties without compromising the overall membrane performance. In spite of significant progress in developing nanocomposite membranes for water treatment, further gaps still need to be addressed. The fabrication of nanocomposite membranes is a complex and meticulous process. Researchers have primarily adopted two different approaches for this purpose,345–347 such as (i) entrapment of nanoparticles into the polymer matrix during the fabrication process using the phase inversion method and (ii) applying a nanoparticle coating to the surface of the membrane. Future research should focus on refining the fabrication techniques to improve the performance and stability of nanocomposite membranes. Additionally, investigating new types of nanoparticles and their interactions with various polymer matrices could yield better fouling resistance, higher selectivity, and increased mechanical strength.
The permeability of the selected TFN, TC, and biomimetic membranes is summarized in Fig. 10. As depicted in the figure, with the exception of one biomimetic membrane (GSNA348) exhibiting the highest permeability of 2900 L m−2 h−1 bar−1, the average permeability of TFN membranes surpasses that of TC and biomimetic membranes. The enhanced permeability of TFN membranes can be attributed to (i) the hydrophilicity of the membrane matrix caused by the incorporation of nanoparticles, facilitating water transport and permeation through the membrane, (ii) more porous and interconnected structure compared to TC and biomimetic membranes, and (iii) increased surface roughness due to the presence of nanoparticles, which can also contribute to improved fouling resistance. Reduced fouling can maintain the membrane's permeability over extended periods of operation, as the accumulation of foulants on the membrane surface is minimized. Moreover, to improve the performance of FO system, a diverse array of draw solutes have been explored, including organic and inorganic solutions, functional nanoparticles, ILs, thermolytic solutions, switchable polarity solvents, thermo-responsive draw solutes, hydrogels, dendrimers, and fertilizers. Despite these advancements, identifying an optimal draw solute that is cost-effective while generating sufficient osmotic pressure remains a significant challenge.209
 |
| Fig. 10 Permeability of the selected membranes obtained through literature survey and screening in the present study. | |
4.3. Economic viability
The economic feasibility of advanced membrane technologies plays a critical role in their adoption and scalability across water treatment applications. Costs associated with different membrane types vary significantly, reflecting differences in material composition, fabrication processes, and operational requirements. Among these, ceramic membranes, while having higher upfront costs, ranging from 500 to 3000 $ per m2 compared to 20–400 $ per m2 for polymeric membranes, offer unparalleled long-term advantages. Their lifespan of 15–20 years, compared to 5–8 years for polymeric membranes, reduces the frequency of replacements and lowers overall capital expenditures.310,349–352 Nanostone Water Inc. conducted a comprehensive 10 years study comparing ceramic and polymeric membranes, revealing that ceramic membranes can achieve ∼55% reduction in overall system costs.353 Moreover, their robust structure, resistance to fouling, and compatibility with aggressive cleaning agents result in lower maintenance costs and increased operational reliability.351,352,354,355 Ceramic membranes also demonstrate superior operational efficiency, with flux capacities exceeding 200 L m−2 h−1 and reaching up to 381 L m−2 h−1 in some cases, substantially higher than the typical 68 L m−2 h−1 of polymeric membranes.351,352,354 This enhanced throughput capacity translates to fewer membranes required per system, reducing the footprint and infrastructure costs. These benefits are especially pronounced in challenging water treatment scenarios, such as the treatment of saline water, where ceramic membranes outperform polymeric alternatives in both efficiency and durability.310,351,352,354
In comparison, other advanced membrane technologies such as FO, TFC, and nanocomposite membranes also exhibit distinct cost and performance profiles. FO membranes, for instance, consume less energy due to their low-pressure operation but may incur higher overall costs due to the need for draw solution regeneration and susceptibility to fouling.127 TFC membranes, which pair an affordable support layer with a thin PA selective layer, offer a balance between cost and performance. However, issues related to fouling and material stability persist, prompting the ongoing research into improved formulations and coatings.127 Additionally, real-world case studies have shown that advanced TFC membranes reduce energy consumption by 15–45% and require smaller infrastructure footprints due to high water permeability and selectivity.356,357 The scalability of TFC membranes is also evidenced by their successful integration into existing manufacturing lines, enabling adaptation to industrial processes.127 For instance, Low et al.358 demonstrated the feasibility of large-scale manufacturing by scaling up the fabrication of PES hollow fibers and the TFC coating process from the laboratory to the industrial scale. Furthermore, pilot-scale implementations, such as those employing 4- and 8-inch TFC membrane modules, have further validated their scalability and operational viability in real-world settings. These implementations not only showcase consistent salt rejection rates >97% but also achieve power densities of up to 5 W m−2 in pressure-retarded osmosis applications, underscoring their practical viability.359 Additionally, advancements in TFC manufacturing techniques have enabled promising integration into large-scale desalination systems, with modules featuring diameters of up to 8-inch and capacities reaching 24 m3 per day, demonstrating operational feasibility in real-world industrial setups.358,360
Nanocomposite membranes, while offering enhanced selectivity and permeability, are associated with higher costs due to the expense of nanomaterials and the precise fabrication techniques required.127,361 Biomimetic membranes, designed to mimic natural biological processes, exhibit remarkable efficiency but remain prohibitively expensive due to their complex fabrication and reliance on sophisticated biological materials.127 Traditional water treatment methods such as coagulation, sedimentation, and sand filtration may have lower initial capital costs but often involve higher operational expenditures due to significant chemical inputs, frequent media replacement, and extensive sludge management. In contrast, ceramic membranes, especially when integrated with advanced processes such as oxidation or electrochemical treatments, deliver superior contaminant removal efficiencies with reduced post-treatment requirements.310,351,354 This combination of high performance and lower long-term costs positions ceramic membranes as competitive alternatives for industrial and municipal water treatment.351,352,362 From a sustainability standpoint, ceramic membranes present clear environmental advantages. Their extended lifespan minimizes waste from replacements, and their resistance to fouling reduces the need for intensive cleaning chemicals, thereby lowering environmental impact. These attributes align with global efforts to enhance resource efficiency and adopt sustainable water treatment practices.351,361,363 Lifecycle cost analyses have shown that ceramic membranes can achieve a net cost benefit of 7–15% over polymeric membranes when considering their extended lifespan, higher flux capacity, and reduced maintenance requirements.352–354
4.4. Fouling
Fouling of membranes arises from the accumulation or adsorption of solutes/solvents on both the membrane surface and within its pore structure.364 RO membrane fouling remains a significant challenge, prompting extensive research into nanotechnology for developing fouling-resistant TFN-RO membranes. Despite promising advancements, concerns persist over the high costs, scalability issues, and health and safety implications associated with nanoparticle additives. Further exploration of surface modification techniques is essential to confirm their efficacy in prolonged operational scenarios. Future research should prioritize long-term fouling tests to assess the durability of modified RO membranes. Additionally, optimizing the balance between membrane transport properties and antifouling capabilities is crucial for enhancing membrane performance.365 One significant limitation of currently developed nanomaterial-based desalination membranes is their susceptibility to biofouling. Biofouling occurs when biological materials, such as bacteria, algae, and other microorganisms, accumulate on the membrane surface, leading to a decline in performance and an increase in maintenance costs. To address this challenge, researchers have suggested the application of nanomaterials with antimicrobial properties, such as Ag and Cu, as coatings on desalination membranes. These nanomaterials can inhibit the growth and adhesion of microorganisms on the membrane surface, thereby enhancing the membrane's longevity and efficiency. The integration of Ag- and Cu-based nanomaterials as antimicrobial coatings offers a promising solution to mitigate biofouling, ensuring more sustainable and effective desalination processes.366 Bioinspired approaches used for fouling mitigation offer promising research directions, particularly by incorporating biological molecules that have evolved to resist severe fouling. For example, lysozyme, a natural and inexpensive source of L-arginine, can be grafted onto the polyamide surface via chemical reactions, demonstrating excellent antifouling properties. However, common modification approaches may increase membrane resistance or deteriorate the crosslinking structure of the PA layer. Additionally, the integration of biological materials with synthetic components is still in its early stages, primarily involving simple biological molecules and proteins within basic polymers. Recent discoveries of new proteins with unique functions and detailed atomic structures present opportunities for the fabrication of advanced membrane systems. Future research should focus on optimizing these bioinspired modifications to balance antifouling capabilities with membrane transport properties and stability.367,368 To decrease the fouling of the membranes, studies have used either a wide variety of materials, including dopamine,369 Mxene,370 layered double hydroxides (LDH) nanosheets,371 graphene quantum dots (GQDs),372 and chitosan/graphene oxide,373 or physical/chemical cleaning approaches.
Besides, enhancing the efficiency of desalination processes can be achieved through the design of suitable pretreatment methods, making the overall process more economic. Membrane-based pretreatment stands out as a competitive technological alternative to conventional methods, particularly in RO desalination, which can reduce the production cost of produced water. Ceramic filters are particularly advantageous due to their durability, ease of use, and resistance against operational pressure. Moreover, pretreatment methods can be effectively combined with conventional pretreatment techniques to enhance the overall system performance. Additionally, integrating renewable energy sources can further reduce energy costs, making membrane-based desalination technologies more cost-effective. Future research should explore various pretreatment design configurations such as combining conventional processes with MF, UF, FO, RO, membrane distillation (MD), etc., to optimize the efficiency and economic viability of desalination system.374
Conventional strategies such as membrane pretreatment, careful selection of membrane materials, and maintaining optimal operating conditions have proven insufficient to fully resolve this issue. Hence, antifouling techniques have emerged as essential solutions to address fouling by targeting membrane properties such as pore size, hydrophilicity, and porosity.375 Antifouling approaches can be broadly categorized into passive antifouling, active antifouling, and surface modification techniques.376 Among these, surface modification has garnered significant attention due to its cost-effectiveness and ability to alter membrane surface characteristics to substantially enhance performance. By reducing foulant adhesion and deposition, surface modifications not only improve operational efficiency but also extend membrane lifespan, thereby lowering overall maintenance costs.377 These modifications also minimize protein and particle adsorption on the membrane surface, a critical factor in maintaining consistent filtration performance.378 Surface modification techniques are further divided into physical, chemical, and hybrid (combined) methods, each offering unique advantages. Physical modifications involve techniques such as blending, coating, and adsorption, while chemical modifications include advanced approaches such as grafting and plasma treatments. Hybrid methods combine elements of both physical and chemical modifications, enabling tailored solutions for specific fouling challenges.377
A comprehensive analysis of contemporary approaches reveals considerable progress in the use of nanomaterials, polymeric modifications, and bioinspired designs. Nanomaterials have become pivotal in antifouling research due to their unique surface properties, scalability, and multifunctionality. GO and CNTs are among the most explored materials for improving hydrophilicity and fouling resistance.379 GO, with their abundant functional groups such as hydroxyl, carboxyl, and epoxy, enhances membrane hydrophilicity and provides antimicrobial properties.380 These features reduce foulant adhesion and biofilm formation while improving permeability. Similarly, CNTs, when incorporated into polymer matrices, enhance mechanical robustness and provide a smooth surface that reduces the accumulation of biofoulants. Studies have demonstrated that CNT-modified membranes show significant reductions in biofouling while maintaining high separation performance.381,382 MOFs contribute to antifouling by enhancing surface charge, hydrophilicity, and selective rejection properties, particularly for heavy metals such as lead and cadmium. Nanodiamonds, with their superior hydrophilic characteristics, not only mitigate biofouling but also improve the flux recovery ratio after fouling, making them highly effective for sustainable operations.383,384
Another area of innovation lies in zwitterionic and biomimetic surfaces. Zwitterionic polymers mimic the antifouling mechanisms of natural membranes by creating a hydration layer that resists the adhesion of organic and biological foulants.385–387 This approach is particularly effective against protein fouling, a common issue in desalination membranes. Biomimetic surfaces inspired by natural antifouling systems, such as fish scales and marine organisms, are gaining traction, utilizing hierarchical micro- and nano-structures to physically prevent the attachment of foulants while maintaining high permeability.388,389
Functionalized surface modifications further expand the scope of antifouling strategies. By grafting hydrophilic polymers or embedding antimicrobial agents onto the membrane surface, researchers have achieved membranes that exhibit dual antifouling and antibacterial properties. Functionalized GO, for instance, has been used to improve fouling resistance and microbial inhibition by incorporating active sites that disrupt microbial adhesion and growth.390–393 Similarly, carboxylated nanodiamonds enhance hydrophilicity and reduce interactions with biofoulants.384 Photocatalytic coatings offer an active mechanism to mitigate fouling. Materials such as TiO2 are incorporated into membranes to provide self-cleaning capabilities under UV light. These coatings actively degrade organic contaminants and biofilms, reducing the need for chemical cleaning and prolonging membrane life. Emerging research on photocatalytic membranes focuses on enhancing their efficiency under visible light, broadening their applicability in diverse water treatment settings.394,395 Despite these advancements, challenges remain in translating laboratory-scale innovations to large-scale applications.
Scalability, cost, and long-term stability of these antifouling strategies are critical concerns. Additionally, the environmental impact of certain nanomaterials necessitates the development of greener, more sustainable alternatives.381 Future research must prioritize the synthesis of multifunctional coatings that address not only fouling resistance but also mechanical durability and compatibility with existing membrane production processes.396 Moreover, the integration of smart materials capable of real-time fouling detection and self-cleaning mechanisms could revolutionize the field.397
4.5. Comparison across different types of membranes
Based on the literature review performed, Table 6 summarizes the key performance metrics for widely recognized membranes used in water/wastewater treatment. While direct comparative analysis across all membrane types is challenging due to variability in operating conditions, feedwater characteristics, and test protocols reported in the literature, this table documents the available data and findings to provide an overall perspective. RO and NF are highly effective for salt and heavy metal removal but are hindered due to low permeability and high fouling potential. FO represents a versatile option with moderate removal% and permeability while highly influenced by the draw solution. Furthermore, UF and MF are indispensable as pretreatment techniques, offering high permeability and fouling resistance but limited selectivity for dissolved solutes. Fouling resistance varies significantly among the membranes. FO membranes generally exhibit moderate fouling due to the low operational pressure. RO membranes, while highly selective, are prone to scaling and biofouling, necessitating rigorous pretreatment stages. NF membranes demonstrate a moderate fouling tendency, particularly from organic and biological fouling. In contrast, UF and MF membranes offer the highest fouling resistance, as their larger pore sizes allow easy backwashing and cleaning to restore performance.
Table 6 Comparison of the recognized membrane separation technologies70,398–402
Membrane matrix |
MF |
UF |
NF |
RO |
FO |
Monovalent ions removal% (Na, K, Cl, NO3) |
<5 |
<40 |
<50 |
>98 |
50–90% (depends on draw solute) |
Bivalent ions removal% (Ca, Mg, SO4, CO3) |
>90 |
>99 |
Heavy metal removal% |
<10 |
20–50 |
50–95 |
98–99.9 |
60–90 |
Permeability (L m−2 h−1 bar−1) |
>1000 |
10–1000 |
1.5–30 |
1–10 |
5–50 |
Pressure (bar) |
0.1–3 |
0.5–10 |
2–40 |
10–75 |
Osmotic pressure |
Fouling resistance |
Very high |
High |
Moderate |
Low |
Moderate |
Typical indicator of fouling behavior |
Flux recovery: >95% after backwashing or chemical cleaning; resistant to particulate fouling |
Flux recovery: >90% after chemical cleaning |
Flux decline: 10–50%; less prone to scaling compared to RO |
Flux decline: 30–70% (depending on pretreatment) |
Flux decline: 20–40% over time; higher tolerance to organic fouling but sensitive to scaling |
4.6. Environmental impact and sustainability consideration
The environmental sustainability of membrane technologies spans the entire lifecycle, from fabrication to operational implementation and eventual disposal. While membranes play a pivotal role in water purification systems, their production processes often rely on energy-intensive methods and fossil-derived materials, raising concerns about carbon emissions and resource depletion.403,404 Similarly, end-of-life management poses challenges, as many membranes are non-biodegradable and require innovative disposal or recycling strategies to mitigate environmental harm. Addressing these issues is critical to aligning membrane technologies with global sustainability goals, ensuring their continued contribution to water security without compromising ecological integrity.
4.6.1 Environmental footprint.
The production of membranes predominantly relies on polymeric or ceramic materials, each with distinct environmental implications. Polymeric membranes, such as those fabricated from PVDF, PSF, or CA, are synthesized through energy-intensive processes involving organic solvents and non-renewable petrochemical feedstocks. The widespread use of volatile organic compounds (VOCs) during fabrication not only elevates the carbon footprint but also poses risks to air quality and human health if improperly managed.405,406 Ceramic membranes, often derived from metal oxides or carbon-based materials, involve high-temperature sintering processes that result in elevated energy consumption and greenhouse gas (GHG) emissions. However, their longer operational lifespan and durability offset these initial environmental costs in long-term applications.354,405,407 For example, modern seawater reverse osmosis (SWRO) plants typically exhibit energy consumption rates in the range of 3–4 kW h m−3, corresponding to net CO2 emissions of 0.4–1.8 kg m−3.403 Recent advancements such as incorporating nanostructured materials including graphenes or MOFs have enhanced the separation performance and fouling resistance, potentially reducing the need for frequent replacements and chemical cleaning.408,409 Bio-based membranes are emerging as green alternatives, utilizing renewable feedstocks such as nanocellulose or chitosan. These materials exhibit low environmental footprints during fabrication and biodegradability. However, achieving scalability while maintaining performance is still a challenge.410,411
Desalination technologies generate substantial volumes of brine, characterized by elevated total dissolved solids (TDS). For instance, the Ashkelon desalination plant, one of the largest facilities globally and the most prominent in the Levant Basin, processes ∼315 million m3 (MCM) of seawater annually. The plant discharges brine with a TDS concentration of 7.35%, ∼1.86 times higher than that of seawater, at a rate of 160 MCM per year.412 Brine reject often contains residues of pretreatment and cleaning chemicals employed to mitigate biofouling, suspended solids, foaming, and corrosion. Additionally, side reactions between these chemicals can result in the formation of halogenated organics and trace heavy metals from corrosion processes. These contaminants, albeit typically at low concentrations, are continuously discharged into the marine environments.404 Of particular concern is the release of toxic antifoulants and anti-scalants. For instance, 10–25% of the Cl2 used as antifoulant during desalination is residual, posing a significant hazard to aquatic ecosystems. Furthermore, cleaning chemicals and their additives such as dodecylbenzene sulfonate and sodium perborate can adversely affect marine life in case of untreated discharge. The environmental implications of such chemical discharge underscore the need for more sustainable and ecologically responsible brine management practices.404,413
4.6.2 Lifecycle assessments.
Operational energy consumption is a critical factor in the environmental impact of membrane separation technologies, particularly for energy-intensive processes such as RO desalination. TFC membranes, widely used for RO, require substantial energy inputs to maintain high operational pressures due to the high flux and salt rejection. While these systems have improved energy efficiency through innovations such as energy recovery devices and pressure exchangers, the energy demands remain high.408,414,415 Fouling, a common operational challenge, exacerbates environmental impacts by necessitating frequent cleaning with harsh chemicals which shortens membrane lifespan and generates secondary pollutants.416 Advances in surface modification techniques such as grafting hydrophilic or nanocomposite layers have shown promise in mitigating fouling and extending operational stability.417,418 Nanocellulose-based membranes are a notable breakthrough, offering biodegradability, mechanical robustness, and enhanced hydrophilicity. These membranes operate with minimal chemical additives, making them environmentally friendly. However, concerns on biofouling limit their broader adoption.405
4.6.3 Sustainable disposal and recycling pathways.
The disposal of membranes, particularly polymeric ones, poses substantial environmental challenges. These materials are often non-biodegradable, contributing to persistent waste when disposed in landfills. Incineration, while capable of energy recovery, emits CO2 and other pollutants, further aggravating environmental concerns.406 Recycling polymeric membranes is difficult due to their complex compositions, complicating material separation and reuse.354 Ceramic membranes offer a more sustainable end-of-life trajectory. Their inert nature allows for repurposing into construction materials or recycling via pyrolysis to recover the embedded components. Hybrid membranes containing nanomaterials such as GO may also benefit from advanced recovery methods, although all remain in early development stages.405
4.6.4 Toward sustainable membrane systems.
Achieving sustainability in membrane technologies necessitates a shift toward circular economy principles. This includes adopting greener fabrication techniques, such as water-based solvents or bio-based precursors, and designing membranes with extended operational lifespans. Lifecycle assessment (LCA) frameworks should guide the development of new membranes to ensure environmental impacts to be minimized in all stages.405 Emerging innovations including biodegradable membranes and low-energy fabrication processes highlight the potential for reducing the environmental footprint of membrane separation systems. Integrating renewable energy sources with hybrid systems and promoting fouling-resistant technologies can further contribute to sustainability. By addressing the full lifecycle, from raw materials to disposal, membrane technologies can evolve into environmentally responsible solutions for water purification and resource management.354,419
5. Artificial intelligence (AI) and machine learning (ML)
Membrane-based desalination technologies have made significant advancements, while fouling, high energy consumption, and operational inefficiencies continue to impede the effectiveness and scalability, stemming from the complex, nonlinear interactions between operational parameters, membrane properties, and environmental factors. Traditional experimental approaches, while valuable, are constrained in their ability to comprehensively model these dynamic processes. Consequently, they often fail to predict long-term system behavior or optimize performance across varying operational conditions, particularly in large-scale applications where real-world variability plays a crucial role.
The incorporation of artificial intelligence (AI) and machine learning (ML) into membrane-based technologies is transforming the field, offering innovative solutions to key challenges such as fouling mitigation, energy efficiency, and system optimization.420 These computational tools excel in analyzing complex, nonlinear interactions inherent to desalination systems, enabling accurate predictions, efficient designs, and real-time adjustments, surpassing traditional modeling techniques. A critical contribution of AI lies in the prediction and management of fouling, a persistent challenge in desalination processes. Advanced algorithms such as artificial neural networks (ANNs), fuzzy logic (FL), and genetic programming (GP) effectively model nonlinear relationships between operational parameters such as transmembrane pressure, permeate flux, and feedwater characteristics. ANNs have achieved prediction accuracies >95%, enabling the anticipation of fouling events and the implementation of proactive strategies to maintain membrane performance and extend operational lifespan.421–424 Deep learning models and hybrid systems have further advanced fouling control, dynamically adapting operational parameters such as feed flow rates and pressure, which has been shown to reduce fouling rates by up to 25%.422,425 AI and ML also play a transformative role in optimizing energy efficiency and operational parameters. Algorithms such as particle swarm optimization (PSO) and genetic algorithms (GA) analyze large datasets to identify optimal feed pressure, recovery rates, and energy consumption strategies, resulting in a 20% reduction in energy demand for RO systems.423,426–428 Hybrid models that combine empirical data with AI algorithms enhance adaptability, enabling real-time adjustments to sustain system efficiency under varying conditions. These methods not only lower energy requirements but also reduce operational costs, aligning with sustainability goals in the desalination industry.423,427
In addition to operational optimization, AI has contributed to the design of next-generation membranes. By integrating convolutional neural networks (CNNs) with molecular dynamics simulations, researchers have developed nanoporous membranes with superior ion selectivity and water flux, achieving up to a 30% performance improvement compared to conventional membranes.421,426,427 These advancements highlight the potential of AI-driven tools in advancing material science for desalination applications. Real-time monitoring and control of desalination systems have also been revolutionized by AI-powered sensors and reinforcement learning algorithms. These systems dynamically adjust feed flow rates, pressures, and operational strategies, reducing response times and minimizing maintenance needs. Such improvements have been instrumental in achieving sustainable operation, with hybrid models reducing prediction errors to <5%, compared to 12% for traditional mathematical models.423,425 Moreover, AI has been increasingly integrated into renewable energy-driven desalination systems, optimizing both energy input and water production to create economically viable solutions for large-scale applications. These systems demonstrate AI potential in both addressing technical challenges and promoting environmentally friendly desalination practices.424,427 Despite the significant progress, challenges remain in scaling AI applications for large desalination plants and improving the interpretability of complex models.429,430 Future efforts are expected to focus on hybrid systems that combine multiple ML techniques with mechanistic models, offering enhanced robustness and scalability. Additionally, as AI evolves, its role in automating real-time operations and developing next-generation membranes is likely to revolutionize the desalination industry further, making it more efficient, cost-effective, and sustainable.
6. Future perspective
Material innovations hold immense promise in overcoming current challenges in membrane performance and durability. The integration of nanomaterials such as TiO2 has demonstrated promising improvements in water flux and salt rejection due to their hydrophilicity, mechanical stability, and antifouling properties, creating a conducive environment for extensive hydrogen bonding with membrane polymers, enhancing permeability and selectivity.431 Future work could explore alternative metal oxides, graphene derivatives, or hybrid nanostructures to further optimize the membrane properties.432,433 Polymeric membranes, while cost-effective and widely used, face challenges such as fouling and limited chemical resistance. Advanced materials such as GO, nanocomposites, and hybrid polymer–ceramic membranes offer promising solutions. GO, for instance, has shown potential in enhancing membrane hydrophilicity and anti-fouling properties, thereby improving performance and durability.431 Similarly, research into ceramic membranes should focus on reducing production costs and improving scalability, as these membranes provide superior thermal and chemical resistance, making them suitable for harsh feedwater conditions.
Additionally, natural polymers such as CA, when combined with nanomaterials, provide a sustainable and efficient pathway for FO membrane development. These materials not only enhance the hydrophilic characteristics but also offer scalable fabrication potential through phase inversion and other nanocomposite techniques.434,435 Developing modular and scalable FO systems using membranes optimized with innovative materials will allow for comprehensive performance evaluations under real-world conditions. Pilot studies should address key parameters such as flux stability, fouling resistance, and energy efficiency to validate their feasibility in wastewater treatment and desalination processes.433,436 Moreover, testing FO systems with diverse feed solutions, ranging from industrial wastewater to brackish water, will help determine the robustness of these technologies across various applications. Incorporating modeling techniques such as response surface methodology (RSM) could also guide the optimization of process conditions and reduce experimental overheads during scale-up.437–439
In terms of biomimetic membranes, emerging materials such as aquaporins, aligned CNTs, graphene-based membranes, and MOFs present promising opportunities to enhance membrane performance.435,440 Self-assembled block copolymers and nanofabrication techniques could enable the precise customization of membrane properties, creating modular designs tailored for specific applications.441,442 Additionally, the development of renewable polymers and the adoption of environmentally benign solvents in membrane fabrication will help align with sustainability goals, reducing environmental impacts during production and disposal.440 To translate laboratory-scale successes into practical solutions, pilot-scale studies are essential. These efforts should validate the scalability and robustness of novel membranes under diverse operational conditions. For example, ultrathin PA membranes require further exploration of substrate-induced limitations and defect-free fabrication processes to realize their full potential in achieving high water permeance and selective ion transport.443
Sustainability considerations are also critical for future advancements in membrane technology. Lifecycle analysis of membrane modules including strategies for recycling and reuse must be prioritized to ensure long-term environmental benefits.
7. Conclusion
Water treatment is of paramount importance for ensuring access to clean and safe water, as a critical resource for public health, agriculture, and industry. The present study systematically reviewed and screened recent literature, both review papers and experimental research, on desalination and heavy metal removal using various advanced membrane technologies, including nanocomposites, thin-film composites, biomimetic materials, forward osmosis, and hybrid membranes. The screening process revealed a significant number of studies being published annually, highlighting the rapid advancements and the necessity for continuous updates to previous reviews. This review has consolidated the most recent findings, identifying the top 10 membranes with the highest removal efficiency as the primary criterion from each category. An updated assessment of the state-of-the-art membrane technologies for water treatment is provided. The performance of each membrane type is discussed, emphasizing the need for further research to address existing challenges and optimizing the trade-offs between different determining factors including economic viability, permeability, removal efficiency, fouling, comparison across different types of membranes, and environmental impact and sustainability consideration. The incorporation of artificial intelligence (AI) and machine learning (ML) into membrane-based desalination technologies has also been studied. Future advancements in materials science, fabrication techniques, and long-term testing will be crucial for developing next-generation membranes that are more efficient, cost-effective, and sustainable for large-scale water treatment applications.
Data availability
No primary research results, software or code have been included and no new data were generated or analyzed as part of this review.
Author contributions
Sahar Foorginezhad contributed to the writing of the original draft, review and editing, investigation, and methodology. Dr Mohammad Mahdi Zerafat was responsible for the writing of the original draft, review and editing, investigation, and supervision. Prof. Ahmad Fauzi Ismail and Dr Pei Sean Goh provided supervision, administration, review and editing support, and fund acquisition.
Conflicts of interest
The authors declare that they have no known competing financial interests or personal relationships that could have appeared to influence the work reported in this paper.
Acknowledgements
A. F. Ismail and P. S. Goh would like to acknowledge the support provided by Ministry of Higher Education under SATREPS grants (4L977).
References
- M. M. Pendergast and E. M. Hoek, A review of water treatment membrane nanotechnologies, Energy Environ. Sci., 2011, 4(6), 1946–1971 RSC.
- S. Foorginezhad, G. Rezvannasab and M. Asadnia, Natural clay membranes: A sustainable and affordable solution for treating dye solutions, coal mine washery waste, and aquaculture wastewater, J. Water Process Eng., 2023, 54, 104012 CrossRef.
-
Supply WUJW and Programme SM, Progress on Sanitation and Drinking Water: 2015 Update and MDG Assessment, World Health Organization, 2015 Search PubMed.
- F. Saadat, M. M. Zerafat and S. Foorginezhad, Adsorption of copper ions from aqueous media using montmorillonite-Al 2 O 3 nano-adsorbent incorporated with Fe 3 O 4 for facile separation, Korean J. Chem. Eng., 2020, 37, 2273–2286 CrossRef CAS.
- S. G. Chaudhri, J. C. Chaudhari and P. S. Singh, Fabrication of efficient pervaporation desalination membrane by reinforcement of poly (vinyl alcohol)–silica film on porous polysulfone hollow fiber, J. Appl. Polym. Sci., 2018, 135(3), 45718 CrossRef.
- W. Kaminski, J. Marszalek and E. Tomczak, Water desalination by pervaporation–Comparison of energy consumption, Desalination, 2018, 433, 89–93 CrossRef CAS.
- Y. Li, E. R. Thomas, M. H. Molina, S. Mann, W. S. Walker and M. L. Lind,
et al., Desalination by membrane pervaporation: A review, Desalination, 2023, 547, 116223 CrossRef CAS.
- N. Abdullah, N. Yusof, A. Ismail and W. Lau, Insights into metal-organic frameworks-integrated membranes for desalination process: A review, Desalination, 2021, 500, 114867 CrossRef CAS.
- S. Foorginezhad and M. Zerafat, Preparation of low-cost ceramic membranes using Persian natural clay and their application for dye clarification, Desalin. Water Treat., 2019, 145, 378–392 CrossRef CAS.
- P. Goh and A. Ismail, A review on inorganic membranes for desalination and wastewater treatment, Desalination, 2018, 434, 60–80 CrossRef CAS.
- M. Hashim, S. Mukhopadhyay, J. N. Sahu and B. Sengupta, Remediation technologies for heavy metal contaminated groundwater, J. Environ. Manage., 2011, 92(10), 2355–2388 CrossRef CAS PubMed.
- L. Xu, T. Wang, J. Wang and A. Lu, Occurrence, speciation and transportation of heavy metals in 9 coastal rivers from watershed of Laizhou Bay, China, Chemosphere, 2017, 173, 61–68 CrossRef CAS PubMed.
- M. Riaz, M. Kamran, Y. Fang, Q. Wang, H. Cao and G. Yang,
et al., Arbuscular mycorrhizal fungi-induced mitigation of heavy metal phytotoxicity in metal contaminated soils: A critical review, J. Hazard. Mater., 2021, 402, 123919 CrossRef CAS PubMed.
- H. Xiang, X. Min, C.-J. Tang, M. Sillanpää and F. Zhao, Recent advances in membrane filtration for heavy metal removal from wastewater: A mini review, J. Water Process Eng., 2022, 49, 103023 CrossRef.
- W. Chen, Y. Su, J. Peng, X. Zhao, Z. Jiang and Y. Dong,
et al., Efficient wastewater treatment by membranes through constructing tunable antifouling membrane surfaces, Environ. Sci. Technol., 2011, 45(15), 6545–6552 CrossRef CAS PubMed.
- N. Abdullah, R. Gohari, N. Yusof, A. Ismail, J. Juhana and W. Lau,
et al., Polysulfone/hydrous ferric oxide ultrafiltration mixed matrix membrane: preparation, characterization and its adsorptive removal of lead (II) from aqueous solution, Chem. Eng. J., 2016, 289, 28–37 CrossRef CAS.
- Y. Hu, Grand challenge in membrane applications: Liquid, Front. Membr. Sci. Technol., 2023, 2, 1177528 CrossRef.
- S. Foorginezhad, G. Yu and X. Ji, Reviewing and screening ionic liquids and deep eutectic solvents for effective CO2 capture, Front. Chem., 2022, 10, 951951 CrossRef CAS PubMed.
- S. M. Dischinger, D. J. Miller, D. A. Vermaas and R. S. Kingsbury, Unifying the Conversation: Membrane Separation Performance in Energy, Water, and Industrial Applications, ACS ES&T Eng., 2024, 4(2), 277–289 Search PubMed.
- Z. Samavati, A. Samavati, P. S. Goh, A. F. Ismail and M. S. Abdullah, A comprehensive review of recent advances in nanofiltration membranes for heavy metal removal from wastewater, Chem. Eng. Res. Des., 2023, 189, 530–571 CrossRef CAS.
- O. A. Kazi, W. Chen, J. G. Eatman, F. Gao, Y. Liu and Y. Wang,
et al., Material design strategies for recovery of critical resources from water, Adv. Mater., 2023, 35(36), 2300913 CrossRef CAS PubMed.
- R. Zhang, J. Tian, S. Gao and B. Van der Bruggen, How to coordinate the trade-off between water permeability and salt rejection in nanofiltration?, J. Mater. Chem. A, 2020, 8(18), 8831–8847 RSC.
- N. S. Suhalim, N. Kasim, E. Mahmoudi, I. J. Shamsudin, A. W. Mohammad and Z. F. Mohamed,
et al., Rejection mechanism of ionic solute removal by nanofiltration membranes: an overview, Nanomaterials, 2022, 12(3), 437 CrossRef CAS PubMed.
- E. Nightingale Jr, Phenomenological theory of ion solvation. Effective radii of hydrated ions, J. Phys. Chem., 1959, 63(9), 1381–1387 CrossRef.
- A. Volkov, S. Paula and D. Deamer, Two mechanisms of permeation of small neutral molecules and hydrated ions across phospholipid bilayers, Bioelectrochem. Bioenerg., 1997, 42(2), 153–160 CrossRef CAS.
- K. Kuma, A. Usui, W. Paplawsky, B. Gedulin and G. Arrhenius, Crystal structures of synthetic 7 Å and 10 Å manganates substituted by mono-and divalent cations, Mineral. Mag., 1994, 58(392), 425–447 CrossRef CAS PubMed.
- Z. Jia and Y. Wang, Covalently crosslinked graphene oxide membranes by esterification reactions for ions separation, J. Mater. Chem. A, 2015, 3(8), 4405–4412 RSC.
-
J. G. Speight, Lange's Handbook of Chemistry, McGraw-Hill, New York, 2005 Search PubMed.
- A. P. Barranco, F. C. Piñar, O. P. Martínez and E. T. García, Effects of MnO2 additive on the properties of PbZrO3–PbTiO3–PbCu1/4Nb3/4O3 ferroelectric ceramic system, J. Eur. Ceram. Soc., 2001, 21(4), 523–529 CrossRef CAS.
- E. A. Permyakov, Metal binding proteins, Encyclopedia, 2021, 1(1), 261–292 CrossRef.
- K. Selim, F. El Hosiny, M. Abdel Khalek and I. Osama, Kinetics and thermodynamics of some heavy metals removal from industrial effluents through electro-flotation process, Colloid Surf. Sci., 2017, 2, 47–53 Search PubMed.
- M. Arshadi, M. J. Amiri and S. Mousavi, Kinetic, equilibrium and thermodynamic investigations of Ni (II), Cd (II), Cu (II) and Co (II) adsorption on barley straw ash, Water Resour. Ind., 2014, 6, 1–17 CrossRef.
- J. Kamcev, D. R. Paul and B. D. Freeman, Ion activity coefficients in ion exchange polymers: applicability of Manning's counterion condensation theory, Macromolecules, 2015, 48(21), 8011–8024 CrossRef CAS.
- L. Ma, L. Gutierrez, M. Vanoppen, C. Aubry and A. Verliefde, Transport of uncharged organics in ion-exchange membranes: experimental validation of the solution-diffusion model, J. Membr. Sci., 2018, 564, 773–781 CrossRef CAS.
- F. G. Donnan, The theory of membrane equilibria, Chem. Rev., 1924, 1(1), 73–90 CrossRef CAS.
- F. G. Donnan, Theorie der Membrangleichgewichte und Membranpotentiale bei Vorhandensein von nicht dialysierenden Elektrolyten. Ein Beitrag zur physikalisch-chemischen Physiologie, Z. Elektrochem. Angew. Phys. Chem., 1911, 17(14), 572–581 CrossRef CAS.
- F. G. Donnan, Theory of membrane equilibria and membrane potentials in the presence of non-dialysing electrolytes. A contribution to physical-chemical physiology, J. Membr. Sci., 1995, 100(1), 45–55 CrossRef CAS.
- D. Lu, Z. Yao, L. Jiao, M. Waheed, Z. Sun and L. Zhang, Separation mechanism, selectivity enhancement strategies and advanced materials for mono-/multivalent ion-selective nanofiltration membrane, Adv. Membr., 2022, 2, 100032 CrossRef.
- R. Epsztein, E. Shaulsky, N. Dizge, D. M. Warsinger and M. Elimelech, Role of ionic charge density in donnan exclusion of monovalent anions by nanofiltration, Environ. Sci. Technol., 2018, 52(7), 4108–4116 CrossRef CAS PubMed.
- H. Rosentreter, C. Scope, T. Oddoy, A. Lerch and J. Meier-Haack, Monovalent selective ion exchange membranes: A review on preparation processes, applications, performance criteria and sustainability aspects, Desalination, 2024, 118412 Search PubMed.
- J. Balster, O. Krupenko, I. Pünt, D. Stamatialis and M. Wessling, Preparation and characterisation of monovalent ion selective cation exchange membranes based on sulphonated poly (ether ether ketone), J. Membr. Sci., 2005, 263(1–2), 137–145 CrossRef CAS.
- J. Gilron, N. Gara and O. Kedem, Experimental analysis of negative salt rejection in nanofiltration membranes, J. Membr. Sci., 2001, 185(2), 223–236 CrossRef CAS.
- D. Asante-Sackey, S. Rathilal, E. Kweinor Tetteh, E. O. Ezugbe and L. V. Pillay, Donnan membrane process for the selective recovery and removal of target metal ions—A mini review, Membranes, 2021, 11(5), 358 CrossRef CAS PubMed.
- P. Prakash and A. K. SenGupta, Selective coagulant recovery from water treatment plant residuals using Donnan membrane process, Environ. Sci. Technol., 2003, 37(19), 4468–4474 CrossRef CAS PubMed.
- J. Keeley, P. Jarvis, A. D. Smith and S. J. Judd, Coagulant recovery and reuse for drinking water treatment, Water Res., 2016, 88, 502–509 CrossRef CAS PubMed.
- P. Prakash, D. Hoskins and A. K. SenGupta, Application of homogeneous and heterogeneous cation-exchange membranes in coagulant recovery from water treatment plant residuals using Donnan membrane process, J. Membr. Sci., 2004, 237(1–2), 131–144 CrossRef CAS.
- Q. Wang, J. J. Lenhart and H. W. Walker, Recovery of metal cations from lime softening sludge using Donnan dialysis, J. Membr. Sci., 2010, 360(1–2), 469–475 CrossRef CAS.
- A. Seidel, J. J. Waypa and M. Elimelech, Role of charge (Donnan) exclusion in removal of arsenic from water by a negatively charged porous nanofiltration membrane, Environ. Eng. Sci., 2001, 18(2), 105–113 CrossRef CAS.
- J. V. Nicolini, C. P. Borges and H. C. Ferraz, Selective rejection of ions and correlation with surface properties of nanofiltration membranes, Sep. Purif. Technol., 2016, 171, 238–247 CrossRef CAS.
- Y. Roy and D. M. Warsinger, Effect of temperature on ion transport in nanofiltration membranes: Diffusion, convection and electromigration, Desalination, 2017, 420, 241–257 CrossRef CAS.
- K. Wang, X. Wang, B. Januszewski, Y. Liu, D. Li and R. Fu,
et al., Tailored design of nanofiltration membranes for water treatment based on synthesis–property–performance relationships, Chem. Soc. Rev., 2022, 51(2), 672–719 RSC.
- X. Wang, N. Wang, X. Li and Q.-F. An, A review of nano-confined composite membranes fabricated inside the porous support, Adv. Membr., 2021, 1, 100005 CrossRef.
- W. R. Bowen, A. W. Mohammad and N. Hilal, Characterisation of nanofiltration membranes for predictive purposes—use of salts, uncharged solutes and atomic force microscopy, J. Membr. Sci., 1997, 126(1), 91–105 CrossRef CAS.
- L. M. Robeson, Correlation of separation factor versus permeability for polymeric membranes, J. Membr. Sci., 1991, 62(2), 165–185 CrossRef CAS.
- S. Wang, X. Li, H. Wu, Z. Tian, Q. Xin and G. He,
et al., Advances in high permeability polymer-based membrane materials for CO 2 separations, Energy Environ. Sci., 2016, 9(6), 1863–1890 RSC.
- L. Braeken, R. Ramaekers, Y. Zhang, G. Maes, B. Van der Bruggen and C. Vandecasteele, Influence of hydrophobicity on retention in nanofiltration of aqueous solutions containing organic compounds, J. Membr. Sci., 2005, 252(1–2), 195–203 CrossRef CAS.
- A. R. Verliefde, E. R. Cornelissen, S. G. Heijman, E. M. Hoek, G. L. Amy and B. V. Bruggen,
et al., Influence of solute− membrane affinity on rejection of uncharged organic solutes by nanofiltration membranes, Environ. Sci. Technol., 2009, 43(7), 2400–2406 CrossRef CAS PubMed.
- S. Darvishmanesh, J. Degrève and B. Van der Bruggen, Mechanisms of solute rejection in solvent resistant nanofiltration: the effect of solvent on solute rejection, Phys. Chem. Chem. Phys., 2010, 12(40), 13333–13342 RSC.
- M. Staikova, F. Wania and D. Donaldson, Molecular polarizability as a single-parameter predictor of vapour pressures and octanol–air partitioning coefficients of non-polar compounds: a priori approach and results, Atmos. Environ., 2004, 38(2), 213–225 CrossRef CAS.
- Y. Zhu, H. Zhu, G. Li, Z. Mai and Y. Gu, The effect of dielectric exclusion on the rejection performance of inhomogeneously charged polyamide nanofiltration membranes, J. Nanopart. Res., 2019, 21(10), 217 CrossRef.
- B. Tansel, J. Sager, T. Rector, J. Garland, R. F. Strayer and L. Levine,
et al., Significance of hydrated radius and hydration shells on ionic permeability during nanofiltration in dead end and cross flow modes, Sep. Purif. Technol., 2006, 51(1), 40–47 CrossRef CAS.
- B. Chen, H. Jiang, X. Liu and X. Hu, Molecular insight into water desalination across multilayer graphene oxide membranes, ACS Appl. Mater. Interfaces, 2017, 9(27), 22826–22836 CrossRef CAS PubMed.
- O. T. Mahlangu, M. M. Motsa, T. I. Nkambule and B. B. Mamba, Rejection of trace organic compounds by membrane processes: Mechanisms, challenges, and opportunities, Rev. Chem. Eng., 2023, 39(5), 875–910 CrossRef CAS.
- W. Bai, L. Samineni, P. Chirontoni, I. Krupa, P. Kasak and A. Popelka,
et al., Quantifying and reducing concentration polarization in reverse osmosis systems, Desalination, 2023, 554, 116480 CrossRef CAS.
- E. M. Hoek and M. Elimelech, Cake-enhanced concentration polarization: a new fouling mechanism for salt-rejecting membranes, Environ. Sci. Technol., 2003, 37(24), 5581–5588 CrossRef CAS PubMed.
- J. R. McCutcheon and M. Elimelech, Influence of concentrative and dilutive internal concentration polarization on flux behavior in forward osmosis, J. Membr. Sci., 2006, 284(1–2), 237–247 CrossRef CAS.
- G. D. Mehta and S. Loeb, Internal polarization in the porous substructure of a semipermeable membrane under pressure-retarded osmosis, J. Membr. Sci., 1978, 4, 261–265 CrossRef CAS.
- P. S. Goh, A. F. Ismail, B. C. Ng and M. S. Abdullah, Recent progresses of forward osmosis membranes formulation and design for wastewater treatment, Water, 2019, 11(10), 2043 CrossRef CAS.
- Q. Yang, J. Lei, D. D. Sun and D. Chen, Forward osmosis membranes for water reclamation, Sep. Purif. Rev., 2016, 45(2), 93–107 CrossRef CAS.
- T. Y. Cath, A. E. Childress and M. Elimelech, Forward osmosis: Principles, applications, and recent developments, J. Membr. Sci., 2006, 281(1–2), 70–87 CrossRef CAS.
- S. Zou, Y. Gu, D. Xiao and C. Y. Tang, The role of physical and chemical parameters on forward osmosis membrane fouling during algae separation, J. Membr. Sci., 2011, 366(1–2), 356–362 CrossRef CAS.
- S. Hong and M. Elimelech, Chemical and physical aspects of natural organic matter (NOM) fouling of nanofiltration membranes, J. Membr. Sci., 1997, 132(2), 159–181 CrossRef CAS.
- A. Seidel and M. Elimelech, Coupling between chemical and physical interactions in natural organic matter (NOM) fouling of nanofiltration membranes: implications for fouling control, J. Membr. Sci., 2002, 203(1–2), 245–255 CrossRef CAS.
- P. Karami, B. Khorshidi, M. McGregor, J. T. Peichel, J. B. Soares and M. Sadrzadeh, Thermally stable thin film composite polymeric membranes for water treatment: A review, J. Cleaner Prod., 2020, 250, 119447 CrossRef CAS.
- D. Qadir, H. Mukhtar and L. K. Keong, Mixed
matrix membranes for water purification applications, Sep. Purif. Rev., 2017, 46(1), 62–80 CrossRef.
- Y. Cao, J. Luo, C. Chen and Y. Wan, Highly permeable acid-resistant nanofiltration membrane based on a novel sulfonamide aqueous monomer for efficient acidic wastewater treatment, Chem. Eng. J., 2021, 425, 131791 CrossRef CAS.
- X. Kang, X. Liu, J. Liu, Y. Wen, J. Qi and X. Li, Spin-assisted interfacial polymerization strategy for graphene oxide-polyamide composite nanofiltration membrane with high performance, Appl. Surf. Sci., 2020, 508, 145198 CrossRef CAS.
- S. Abdikheibari, W. Lei, L. F. Dumée, N. Milne and K. Baskaran, Thin film nanocomposite nanofiltration membranes from amine functionalized-boron nitride/polypiperazine amide with enhanced flux and fouling resistance, J. Mater. Chem. A, 2018, 6(25), 12066–12081 RSC.
- Y. Cheng, C. Xia, H. A. Garalleh, M. Garaleh, N. T. L. Chi and K. Brindhadevi, A review on optimistic development of polymeric nanocomposite membrane on environmental remediation, Chemosphere, 2023, 315, 137706 CrossRef CAS PubMed.
- T. Wen, Z. Zhao, C. Shen, J. Li, X. Tan and A. Zeb,
et al., Multifunctional flexible free-standing titanate nanobelt membranes as efficient sorbents for the removal of radioactive 90Sr2+ and 137Cs+ ions and oils, Sci. Rep., 2016, 6(1), 1–10 CrossRef PubMed.
- M. Delavar, G. Bakeri, M. Hosseini and N. Nabian, Fabrication and characterization of polyvinyl chloride mixed matrix membranes containing high aspect ratio anatase titania and hydrous manganese oxide nanoparticle for efficient removal of heavy metal ions: Competitive removal study, Can. J. Chem. Eng., 2020, 98(7), 1558–1579 CrossRef CAS.
- P. Aryanti, M. Afred, A. Wardani, G. Lugito, G. Kadja and I. Wenten,
et al., Ultra low-pressure reverse osmosis (ULPRO) membrane for desalination: Current challenges and future directions, Desalination, 2023, 116650 CrossRef CAS.
- A.-hM. A. El-Aassar, Improvement of reverse osmosis performance of polyamide thin-film composite membranes using TiO2 nanoparticles, Desalin. Water Treat., 2015, 55(11), 2939–2950 CrossRef CAS.
- H. Dong, L. Zhao, L. Zhang, H. Chen, C. Gao and W. W. Ho, High-flux reverse osmosis membranes incorporated with NaY zeolite nanoparticles for brackish water desalination, J. Membr. Sci., 2015, 476, 373–383 CrossRef CAS.
- E. Valamohammadi, F. Behdarvand, T. Mohammadi, M. A. Tofighy and Z. Moghiseh, Effects of carbon nanotubes on structure, performance and properties of polymer nanocomposite membranes for water/wastewater treatment applications: a comprehensive review, Polym. Bull., 2023, 80(11), 11589–11632 CrossRef CAS.
- J. Nan Shen, C. Chao Yu, H. Min Ruan, C. ie Gao and B. Van der Bruggen, Preparation and characterization of thin-film nanocomposite membranes embedded with poly (methyl methacrylate) hydrophobic modified multiwalled carbon nanotubes by interfacial polymerization, J. Membr. Sci., 2013, 442, 18–26 CrossRef.
- M. Peydayesh, T. Mohammadi and O. Bakhtiari, Water desalination via novel positively charged hybrid nanofiltration membranes filled with hyperbranched polyethyleneimine modified MWCNT, J. Ind. Eng. Chem., 2019, 69, 127–140 CrossRef CAS.
- W.-F. Chan, E. Marand and S. M. Martin, Novel zwitterion functionalized carbon nanotube nanocomposite membranes for improved RO performance and surface anti-biofouling resistance, J. Membr. Sci., 2016, 509, 125–137 CrossRef CAS.
- H.-Z. Zhang, Z.-L. Xu, H. Ding and Y.-J. Tang, Positively charged capillary nanofiltration membrane with high rejection for Mg2+ and Ca2+ and good separation for Mg2+ and Li+, Desalination, 2017, 420, 158–166 CrossRef CAS.
- Y. Yu, X. Zhang, P. Lu, D. He, L. Shen and Y. Li, Enhanced Separation Performance of Polyamide Thin-Film Nanocomposite Membranes with Interlayer by Constructed Two-Dimensional Nanomaterials: A Critical Review, Membranes, 2022, 12(12), 1250 CrossRef CAS PubMed.
- X. Wang, Y. Liu, K. Fan, P. Cheng and S. Xia, Nano-striped polyamide membranes enabled by vacuum-assisted incorporation of hierarchical flower-like MoS2 for enhanced nanofiltration performance, J. Membr. Sci., 2023, 668, 121250 CrossRef CAS.
- J. Shi, W. Wu, Y. Xia, Z. Li and W. Li, Confined interfacial polymerization of polyamide-graphene oxide composite membranes for water desalination, Desalination, 2018, 441, 77–86 CrossRef CAS.
- H. Zhang, D. Taymazov, M.-P. Li, Z.-H. Huang, W.-L. Liu and X. Zhang,
et al., Construction of MoS2 composite membranes on ceramic hollow fibers for efficient water desalination, J. Membr. Sci., 2019, 592, 117369 CrossRef CAS.
- D. Upreti, A. Rajendran, N. Lenka, R. Srivastava, R. S. Gupta and B. Maiti,
et al., Designing a robust biocompatible porous polymeric membrane using Laponite and graphene oxide for versatile and selective adsorption of water contaminants, Chem. Eng. J., 2023, 464, 142738 CrossRef CAS.
- Y. Li, G. Zhao, G. Pan, Y. Zhang, Y. Guo and H. Yu,
et al., Polyvinyl alcohol/attapulgite interlayer modulated interfacial polymerization on a highly porous PAN support for efficient desalination, J. Membr. Sci., 2023, 675, 121517 CrossRef CAS.
- S. Amiri, M. Sheydaei, T. He and V. Vatanpour, Polyethylene-Supported Thin-Film Nanocomposite Reverse Osmosis Membranes via Oxygen Plasma Pretreatment Modification and Nanotitanium Dioxide Incorporation for Efficient As (V) Removal, Ind. Eng. Chem. Res., 2023, 62(43), 17959–17973 CrossRef.
- S. Foorginezhad and M. Zerafat, Microfiltration of cationic dyes using nano-clay membranes, Ceram. Int., 2017, 43(17), 15146–15159 CrossRef CAS.
- Y. H. Cho, H. W. Kim, S. Y. Nam and H. B. Park, Fouling-tolerant polysulfone–poly (ethylene oxide) random copolymer ultrafiltration membranes, J. Membr. Sci., 2011, 379(1–2), 296–306 CrossRef CAS.
- Z.-Z. Zhao, T. Wang, X. Zheng, Y. Ren and Z.-P. Zhao, MOF-808/Polyamide Thin-Film Nanocomposite Membranes for Efficient Nanofiltration, ACS Appl. Nano Mater., 2023, 6(19), 17615–17625 CrossRef CAS.
- Z. Wu, J. Zeng, Z. Yuan, R. Zhang, X. Huang and F. Feng,
et al., Polyamide/UiO-66-NH2 nanocomposite membranes by polyphenol interfacial engineering for molybdenum (VI) removal, Desalination, 2023, 563, 116716 CrossRef CAS.
- H. Azad, M. Mohsennia, C. Cheng and A. Amini, Alkaline treatment of antifoulant PVB/SA-grafted ε-MnO2 adsorptive nanocomposite membrane for synchronous separation of pollutants from pharmaceutical effluents, J. Water Process Eng., 2023, 53, 103756 CrossRef.
- N. S. de Resende, A. L. Ohland, V. M. Salim and C. P. Borges, Transport properties investigations of nanocomposite membranes incorporating titanate nanowires for water desalination, J. Appl. Polym. Sci., 2023, 140(31), e54229 CrossRef CAS.
- S. Kumarage, I. Munaweera, C. Sandaruwan, L. Weerasinghe and N. Kottegoda, Electrospun amine-functionalized silica nanoparticles–cellulose acetate nanofiber membranes for effective removal of hardness and heavy metals (As (v), Cd (ii), Pb (ii)) in drinking water sources, Environ. Sci. Water Res. Technol., 2023, 9(10), 2664–2679 RSC.
- A. Afzal, F. Shahid, M. Rafique and I. Nazir, Manganese spinel ferrites-composite nanotubes impregnated thermally endured cellulose acetate membranes for superior desalination application, Asia-Pac. J. Chem. Eng., 2023, e2914 CrossRef CAS.
- T. Subrahmanya, J.-Y. Lin, J. Widakdo, H. F. M. Austria, Y.-H. Chiao and T.-H. Huang,
et al., Effect of functionalized nanodiamonds and surfactants mediation on the nanofiltration performance of polyamide thin-film nanocomposite membranes, Desalination, 2023, 555, 116540 CrossRef CAS.
- H. Razzaq, A. Siddique, S. Farooq, S. Razzaque, B. Ahmad and S. Tahir,
et al., Hybrid ionogel membrane of PVDF incorporating ionic liquid-reduced graphene oxide for the removal of heavy metal ions from synthetic water, Mater. Sci. Eng., B, 2023, 296, 116680 CrossRef CAS.
- E. Ostadi and N. Mohammadi, Does pervasive interconnected network of cellulose nanocrystals in nanocomposite membranes address simultaneous mechanical strength/water permeability/salt rejection improvement?, Carbohydr. Polym., 2024, 325, 121588 CrossRef CAS PubMed.
- M. A. Naji, H. Salimi-Kenari, Q. F. Alsalhy, R. A. Al-Juboori, N. Huynh and K. T. Rashid,
et al., Novel MXene-Modified Polyphenyl Sulfone Membranes for Functional Nanofiltration of Heavy Metals-Containing Wastewater, Membranes, 2023, 13(3), 357 CrossRef CAS PubMed.
- J. Wang, X. Zhao, H. Qu, J. Xu and J. Ma, Rationally regulating the PES substrate by introducing ZIF-8 with tunable shapes for optimal thin-film composite membrane, Appl. Surf. Sci., 2023, 615, 156181 CrossRef CAS.
- X.-H. Li, H.-J. Yang, Y.-X. Ma, T.-Z. Li, W.-L. Meng and X.-M. Zhong, A novel PAN/GO electrospun nanocomposite fibrous membranes with rich amino groups for highly efficient adsorption of Au (III), Diamond Relat. Mater., 2023, 137, 110175 CrossRef CAS.
- S. Gu, W. Zhu, B. Sun, D. Wang and L. Zhang, Construction of rGO@ AuNPs incorporated antifouling nanofiltration membrane with elevated filtration performance, J. Environ. Chem. Eng., 2023, 11(5), 110860 CrossRef CAS.
- M. Alhoshan, J. Alam, A. K. Shukla and A. A. Hamid, Polyphenylsulfone membrane blended with polyaniline for nanofiltration promising for removing heavy metals (Cd2+/Pb2+) from wastewater, J. Mater. Res. Technol., 2023, 24, 6034–6047 CrossRef CAS.
- Z. Hosseini and A. Kargari, Fabrication and characterization of hydrophobic/hydrophilic dual-layer polyphenyl sulfone Janus membrane for application in direct contact membrane distillation, Desalination, 2024, 571, 117100 CrossRef CAS.
- J. M. Luque-Alled, S. Leaper, A. Abdel-Karim, C. Skuse and P. Gorgojo, PVDF membranes containing alkyl and perfluoroalkyl-functionalized graphene nanosheets for improved membrane distillation, J. Environ. Chem. Eng., 2023, 11(3), 109898 CrossRef CAS.
- A. Siddiqa, S. Haider, I. Abid, S. Farooq, N. Ahmed and S. Fazil,
et al., Preparation, characterization of PVDF/PVP/MMT mixed matrix membranes and their application for the removal of heavy metals from wastewater, J. Dispersion Sci. Technol., 2023, 1–14 Search PubMed.
- L. Chandra, K. Vinothkumar and R. G. Balakrishna, MIL-100 (Fe) integrated fibrous polyvinyl alcohol graft on cellulose acetate towards the development of green membranes; Application in multi solute rejection, J. Environ. Chem. Eng., 2023, 11(3), 109851 CrossRef CAS.
- F. Soomro, F. H. Memon, M. A. Khan, M. Iqbal, A. Ibrar and A. A. Memon,
et al., Ultrathin Graphene Oxide-Based Nanocomposite Membranes for Water Purification, Membranes, 2023, 13(1), 64 CrossRef CAS PubMed.
- K. M. Alotaibi, A. K. Shukla, E. Bajuayfir, A. A. Alotaibi, M. H. Mrad and F. A. Gomaa,
et al., Ultrasound-Assisted Synthesis of MSNs/PS Nanocomposite Membranes for Effective Removal of Cd2+ and Pb2+ ions from Aqueous Solutions, Ultrason. Sonochem., 2023, 106497 CrossRef CAS PubMed.
- Z. Fallahnejad, G. Bakeri and A. Ismail, Incorporation of internal surface functionalized MCM-41 in thin film nanocomposite NF membrane for desalination applications, J. Polym. Res., 2023, 30(8), 297 CrossRef CAS.
- N. A. Ahmad, L. J. Tam, P. S. Goh, N. Azman, A. F. Ismail and K. Jamaluddin,
et al., Treatment of radionuclide-containing wastewater using thin film composite reverse osmosis membrane with spray coating-assembled titania nanosheets, J. Environ. Chem. Eng., 2023, 11(5), 110540 CrossRef CAS.
- S. Foorginezhad, M. M. Zerafat, Y. Mohammadi and M. Asadnia, Fabrication of tubular ceramic membranes as low-cost adsorbent using natural clay for heavy metals removal, Cleaner Eng. Technol., 2022, 10, 100550 CrossRef.
- O. T. Mahlangu, G. Mamba and B. B. Mamba, A facile synthesis approach for GO-ZnO/PES ultrafiltration mixed matrix photocatalytic membranes for dye removal in water: Leveraging the synergy between photocatalysis and membrane filtration, J. Environ. Chem. Eng., 2023, 11(3), 110065 CrossRef CAS.
- P. Li, Z. Gu, C. Bai, Y. Xu, J. Tang and J. Ren,
et al., Effects of MOFs size and functional group on the pore structure and performance of thin-film nanocomposite membranes, J. Membr. Sci., 2023, 686, 121979 CrossRef CAS.
- H. Ranjbaran, E. Ameri and B. Dehghani, Preparation of dendrimer/TiO2 polysulfone nanofiltration membrane to improve antibacterial, antifouling and separation performance of contaminants (heavy metals, salts, dyes), Polym. Bull., 2023, 1–24 Search PubMed.
- F. Yang, F. Cui, Y. Di Yuan, X. Yu and D. Zhao, Mechanistic insights into the role of nanoparticles towards the enhanced performance of thin-film nanocomposite membranes, J. Membr. Sci. Lett., 2023, 3(1), 100046 CrossRef.
- H. Yang, G. Dong, L. Qin, Q. Hua, J. Zhu and Y. Zhang, Polyamide nanofiltration membranes mediated by mesoporous silica nanosheet interlayers display substantial desalination performance enhancement, J. Membr. Sci., 2024, 693, 122387 CrossRef CAS.
- M. Kadhom, A review on the polyamide thin film composite (TFC) membrane used for desalination: Improvement methods, current alternatives, and challenges, Chem. Eng. Res. Des., 2023, 191, 472–492 CrossRef CAS.
- M. Kadhom, W. Hu and B. Deng, Thin film nanocomposite membrane filled with metal-organic frameworks UiO-66 and MIL-125 nanoparticles for water desalination, Membranes, 2017, 7(2), 31 CrossRef PubMed.
- K. Goh, H. E. Karahan, L. Wei, T.-H. Bae, A. G. Fane and R. Wang,
et al., Carbon nanomaterials for advancing separation membranes: A strategic perspective, Carbon, 2016, 109, 694–710 CrossRef CAS.
- S. A. Aktij, M. Hosseininejad, M. D. Firouzjaei, S. Farhadi, M. Elliott and A. Rahimpour,
et al., High perm-selectivity and performance of tuned nanofiltration membranes by merging carbon nitride derivatives as interphase layer for efficient water treatment, J. Water Process Eng., 2023, 56, 104432 CrossRef.
- M. Batool, M. A. Abbas, I. A. Khan, M. Z. Khan, M. Saleem and A. U. Khan,
et al., Response Surface Methodology Modeling Correlation of Polymer Composite Carbon Nanotubes/Chitosan Nanofiltration Membranes for Water Desalination, ACS ES&T Water, 2023, 3(5), 1406–1421 Search PubMed.
- J. Alam, A. K. Shukla, L. Arockiasamy and M. Alhoshan, Scale Design of Dual-Layer Polyphenylsulfone/Sulfonated Polyphenylsulfone Hollow Fiber Membranes for Nanofiltration, Membranes, 2023, 13(8), 714 CrossRef CAS PubMed.
- Y. Xie, L. Cheng, F. Liu and J. Li, TiO2-Decorated Photocatalytic Nanofiltration Membranes for Enhanced Self-Cleaning and Separation Performance, ACS ES&T Water, 2023, 3(10), 3418–3427 Search PubMed.
- Q. Jiang, Y. Wang, Y. Li, J. Luo and J. Xiong, Nanocomposite substrate-supported nanofiltration membrane for efficient treatment of rare earth wastewater, Results Eng., 2023, 18, 101040 CrossRef CAS.
- H. Wu, H. Zhao, Y. Lin, X. Liu, L. Wang and H. Yao,
et al., Positively-charged PEI/TMC nanofiltration membrane prepared by adding a diamino-silane coupling agent for Li+/Mg2+ separation, J. Membr. Sci., 2023, 672, 121468 CrossRef CAS.
- C. Balcik, B. Ozbey-Unal, B. Sahin, E. Buse Aydın, B. Cifcioglu-Gozuacik and R. Keyikoglu,
et al., Development of ZnFeCe Layered Double Hydroxide Incorporated Thin Film Nanocomposite Membrane with Enhanced Separation Performance and Antibacterial Properties, Water, 2023, 15(2), 264 CrossRef CAS.
- S. Amiri, V. Vatanpour and T. He, Antifouling thin-film nanocomposite NF membrane with polyvinyl alcohol-sodium alginate-graphene oxide nanocomposite hydrogel coated layer for As (III) removal, Chemosphere, 2023, 322, 138159 CrossRef CAS PubMed.
- L. E. Peng, Z. Yao, Z. Yang, H. Guo and C. Y. Tang, Dissecting the role of substrate on the morphology and separation properties of thin film composite polyamide membranes: seeing is believing, Environ. Sci. Technol., 2020, 54(11), 6978–6986 CrossRef CAS PubMed.
- Z. Thong, G. Han, Y. Cui, J. Gao, T.-S. Chung and S. Y. Chan,
et al., Novel nanofiltration membranes consisting of a sulfonated pentablock copolymer rejection layer for heavy metal removal, Environ. Sci. Technol., 2014, 48(23), 13880–13887 CrossRef CAS PubMed.
- J. Safaei, P. Xiong and G. Wang, Progress and prospects of two-dimensional materials for membrane-based water desalination, Mater. Today Adv., 2020, 8, 100108 CrossRef.
- C. Fritzmann, J. Löwenberg, T. Wintgens and T. Melin, State-of-the-art of reverse osmosis desalination, Desalination, 2007, 216(1–3), 1–76 CrossRef CAS.
- S. Park, R. Patel and Y. C. Woo, Polyester-based thin-film composite membranes for nanofiltration of saline water: A review, Desalination, 2023, 117138 Search PubMed.
- H. Wu, Y. Liu, H. Zhang, J. Wang and Z. Wang, Rapid construction of cyclodextrin polyester layer on polyamide for preparing highly permeable reverse osmosis membrane, J. Membr. Sci., 2022, 660, 120862 CrossRef CAS.
- S. Xiong, D. Y. Zhang, S. Mei, J. Liu, Y. S. Shi and Y. Wang, Thin film composite membranes containing intrinsic CD cavities in the selective layer, J. Membr. Sci., 2018, 551, 294–304 CrossRef CAS.
- Q. Hu, D. Li, S. Liu, G. Yan, J. Yang and G. Zhang, Effects of alkali on the polyester membranes based on cyclic polyphenols for nanofiltration, Desalination, 2022, 533, 115774 CrossRef CAS.
- P. Sarkar, C. Wu, Z. Yang and C. Y. Tang, Empowering ultrathin polyamide membranes at the water–energy nexus: strategies, limitations, and future perspectives, Chem. Soc. Rev., 2024, 4374–4399 RSC.
- P. Sarkar, S. Modak and S. Karan, Ultraselective and highly permeable polyamide nanofilms for ionic and molecular nanofiltration, Adv. Funct. Mater., 2021, 31(3), 2007054 CrossRef CAS.
- S.-J. Park and J.-H. Lee, Fabrication of high-performance reverse osmosis membranes via dual-layer slot coating with tailoring interfacial adhesion, J. Membr. Sci., 2020, 614, 118449 CrossRef CAS.
- X. Wu, T. Chen, G. Dong, M. Tian, J. Wang and R. Zhang,
et al., A critical review on polyamide and polyesteramide nanofiltration membranes: Emerging monomeric structures and interfacial polymerization strategies, Desalination, 2024, 117379 CrossRef CAS.
- Y. Qian, H. Li, J. Lu, D. Lu, H. Jin and Z. Xia,
et al., Inhibiting polyamide intrusion of thin film composite membranes: strategies and environmental implications, Environ. Sci. Technol., 2023, 57(29), 10860–10869 CrossRef CAS PubMed.
- B.-X. Gu, H.-H. Wu, D. Sun, Y.-L. Ji and C.-J. Gao, Zwitterionic cyclodextrin membrane with uniform subnanometre pores for high-efficient heavy metal ions removal, J. Membr. Sci., 2023, 688, 122123 CrossRef CAS.
- Y. Tang, L. Cao, L. Xu, Z. Wang, Q. Shi and Y. Zhang,
et al., Dependable performance of thin film composite nanofiltration membrane tailored by capsaicin-derived self-polymer, Polymers, 2022, 14(9), 1671 CrossRef CAS PubMed.
- Q. An, F. Li, Y. Ji and H. Chen, Influence of polyvinyl alcohol on the surface morphology, separation and anti-fouling performance of the composite polyamide nanofiltration membranes, J. Membr. Sci., 2011, 367(1–2), 158–165 CrossRef CAS.
- X. Zhang, H. Zhang, L. Wang, J. Wang, X. Wang and J. Hao, Probing the construction mechanism of polyamide membranes regulated by interfacial polymerization from the novel micro-and macro-perspectives: A review, Desalination, 2024, 117422 CrossRef CAS.
- C. Jiang, Z. Fei and Y. Hou, High-performance polyamide reverse osmosis membrane containing flexible aliphatic ring for water purification, Polymers, 2023, 15(4), 944 CrossRef CAS PubMed.
- M. Zhang, J. Yuan, Z. Yin, N. A. Khan, C. Yang and M. Long,
et al., Organic salt modulated preparation of ultra-thin and loose polyamide nanofiltration membranes with enhanced
performance, J. Membr. Sci., 2023, 680, 121739 CrossRef CAS.
- M. An, L. Gutierrez, A. D'Haese, L. Tan, A. Verliefde and E. Cornelissen, In-situ modification of nanofiltration and reverse osmosis membranes for organic micropollutants and salts removal: A review, Desalination, 2023, 116861 CrossRef CAS.
- S. Yu, X. Liu, J. Liu, D. Wu, M. Liu and C. Gao, Surface modification of thin-film composite polyamide reverse osmosis membranes with thermo-responsive polymer (TRP) for improved fouling resistance and cleaning efficiency, Sep. Purif. Technol., 2011, 76(3), 283–291 CrossRef CAS.
- M. Liu, L. Zhang and N. Geng, Effect of Interlayer Construction on TFC Nanofiltration Membrane Performance: A Review from Materials Perspective, Membranes, 2023, 13(5), 497 CrossRef CAS PubMed.
- X. Zhu, X. Zhang, J. Li, X. Luo, D. Xu and D. Wu,
et al., Crumple-textured polyamide membranes via MXene nanosheet-regulated interfacial polymerization for enhanced nanofiltration performance, J. Membr. Sci., 2021, 635, 119536 CrossRef CAS.
- A. Wang, H. Xu, J. Fu, T. Lin, J. Ma and M. Ding,
et al., Enhanced high-salinity brines treatment using polyamide nanofiltration membrane with tunable interlayered MXene channel, Sci. Total Environ., 2023, 856, 158434 CrossRef CAS PubMed.
- Y. Sun, W. Yang, Y. Wang, F. Yang, X. Li and Y. Yang,
et al., MXene Film as an Interlayer for Thin-Film Composite Membranes with High-Performance Nanofiltration, ACS Appl. Polym. Mater., 2024, 7801–7808 CrossRef CAS.
- A. Swain, S. Adarsh, A. Biswas, S. Bose, B. C. Benicewicz and S. K. Kumar,
et al., Enhanced efficiency of water desalination in nanostructured thin-film membranes with polymer grafted nanoparticles, Nanoscale, 2023, 15(28), 11935–11944 RSC.
- Z. Wang, L. Bai, J. Wang, H. Liang and G. Li, Visible light-induced crosslinking enables highly permeable and chlorine-resistant polyamide membranes for water purification, J. Membr. Sci., 2023, 683, 121819 CrossRef CAS.
- Y. Zhang, Y. Chen, M. Wang, W. Su, H. Li and P. Li,
et al., Preparation of high temperature resistant polyamide composite nanofiltration membranes by thermally assisted interfacial polymerization, J. Membr. Sci., 2023, 687, 122020 CrossRef CAS.
- G. Zhao, Y. Zhang and Y. Liu, pH stable thin film composite poly (amine-urea) nanofiltration membranes with excellent rejection performance, Sep. Purif. Technol., 2023, 320, 124108 CrossRef CAS.
- Y. Chen and Y. Cohen, RO membrane with a surface tethered polymer brush layer for enhanced rejection of nitrate, boron, and arsenic, J. Membr. Sci. Lett., 2023, 3(2), 100062 CrossRef.
- P. Bera and S. K. Jewrajka, Regenerable planting of multifunctional amine: Regeneration-enhanced antifouling/antiscaling properties and performance of thin film composite nanofiltration membrane, J. Membr. Sci., 2024, 692, 122292 CrossRef CAS.
- Q. Zhao, D. L. Zhao, L. Y. Ee, T.-S. Chung and S. B. Chen, In-situ coating of Fe-TA complex on thin-film composite membranes for improved water permeance in reverse osmosis desalination, Desalination, 2023, 554, 116515 CrossRef CAS.
- F. I. Azmi, P. S. Goh, A. F. Ismail, N. A. Ahmad and M. N. Z. Abidin, Enhancing the antifouling and chlorine resistance capabilities of thin-film composite reverse osmosis via surface grafting of dipeptide, React. Funct. Polym., 2023, 192, 105708 CrossRef CAS.
- M. Koli, R. Ranjan and S. P. Singh, Functionalized graphene-based ultrafiltration and thin-film composite nanofiltration membranes for arsenic, chromium, and fluoride removal from simulated groundwater: Mechanism and effect of pH, Process Saf. Environ. Prot., 2023, 179, 603–617 CrossRef CAS.
- Q. Luo, J. Li, P. Yun, L. Qian, J. Zhang and C. He,
et al., Thin-film composite membrane for desalination containing a sulfonated UiO-66 material, J. Mater. Sci., 2023, 58(7), 3134–3146 CrossRef CAS.
- L. T. Le, P. N. Vu, H. T. Nguyen, M. Van Nguyen, H. T. Bui and H. Q. Tran,
et al., An Adsorptive Membrane Based on Electrospun Graphene Oxide/Poly (vinylidene) Fluoride Fiber Coated with Chitosan for Removal of Manganese Ions from Aqueous Solutions Using a Simple Filtration Process, Fibers Polym., 2023, 24(9), 3047–3060 CrossRef CAS.
- S. Bakhodaye Dehghanpour, M. Razavi, F. Parvizian, V. Vatanpour and T. He, Investigation of SDS effect on the properties and performance of nanofiltration membranes prepared on polyolefin support, J. Iran. Chem. Soc., 2024, 21(2), 535–548 CrossRef CAS.
- U. Baig, S. M. S. Jillani and A. Waheed, Decoration of β-Cyclodextrin and Tuning Active Layer Chemistry Leading to Nanofiltration Membranes for Desalination and Wastewater Decontamination, Membranes, 2023, 13(5), 528 CrossRef CAS PubMed.
- Q. Wang, Y. Wang, Y. Huang, H. Wang, Y. Gao and M. Zhao,
et al., Polyethyleneimine (PEI) based positively charged thin film composite polyamide (TFC-PA) nanofiltration (NF) membranes for effective Mg2+/Li+ separation, Desalination, 2023, 565, 116814 CrossRef CAS.
- E. Wang, S. Liu, L. Liu, L. Han and B. Su, Positively charged thin-film composite hollow fiber nanofiltration membrane via interfacial polymerization and branch polyethyleneimine modification for Mg2+/Li+ separation, J. Membr. Sci. Lett., 2023, 3(2), 100061 CrossRef.
- Z. Lü, G. Ding, M. Liu, S. Yu and C. Gao, Improved separation performance, anti-fouling property and durability of polyamide-based RO membrane by constructing a polyvinyl alcohol/polyquaternium-10 surface coating layer, Desalination, 2023, 564, 116755 CrossRef.
- R. Sengur-Tasdemir, S. Aydin, T. Turken, E. A. Genceli and I. Koyuncu, Biomimetic approaches for membrane technologies, Sep. Purif. Rev., 2016, 45(2), 122–140 CrossRef CAS.
- K. Ishibashi, S. Kondo, S. Hara and Y. Morishita, The evolutionary aspects of aquaporin family, Am. J. Physiol.: Regul., Integr. Comp. Physiol., 2011, 300(3), R566–R76 CrossRef CAS PubMed.
- Y. Li, S. Qi, M. Tian, W. Widjajanti and R. Wang, Fabrication of aquaporin-based biomimetic membrane for seawater desalination, Desalination, 2019, 467, 103–112 CrossRef CAS.
- P. Goh, T. Matsuura, A. Ismail and N. Hilal, Recent trends in membranes and membrane processes for desalination, Desalination, 2016, 391, 43–60 CrossRef CAS.
- Y. J. Lim, K. Goh, G. S. Lai, C. Y. Ng, J. Torres and R. Wang, Fast water transport through biomimetic reverse osmosis membranes embedded with peptide-attached (pR)-pillar [5] arenes water channels, J. Membr. Sci., 2021, 628, 119276 CrossRef CAS.
- W. Ding, J. Cai, Z. Yu, Q. Wang, Z. Xu and Z. Wang,
et al., Fabrication of an aquaporin-based forward osmosis membrane through covalent bonding of a lipid bilayer to a microporous support, J. Mater. Chem. A, 2015, 3(40), 20118–20126 RSC.
- Y.-x Shen, P. O. Saboe, I. T. Sines, M. Erbakan and M. Kumar, Biomimetic membranes: A review, J. Membr. Sci., 2014, 454, 359–381 CrossRef CAS.
- T. Ren, M. Erbakan, Y. Shen, E. Barbieri, P. Saboe and H. Feroz,
et al., Membrane protein insertion into and compatibility with biomimetic membranes, Adv. Biosyst., 2017, 1(7), 1700053 CrossRef PubMed.
- C. J. Porter, J. R. Werber, M. Zhong, C. J. Wilson and M. Elimelech, Pathways and challenges for biomimetic desalination membranes with sub-nanometer channels, ACS Nano, 2020, 14(9), 10894–10916 CrossRef CAS PubMed.
- A. Azarafza, M. A. Islam, Y. Golpazirsorkheh, I. Efteghar, M. Sadrzadeh and M. Kamkar,
et al., Aquaporin-Based Biomimetic Membranes for Low Energy Water Desalination and Separation Applications, Adv. Funct. Mater., 2023, 33(21), 2213326 CrossRef CAS.
- H. Wang, T. S. Chung, Y. W. Tong, K. Jeyaseelan, A. Armugam and Z. Chen,
et al., Highly permeable and selective pore-spanning biomimetic membrane embedded with aquaporin Z., Small, 2012, 8(8), 1185–1190 CrossRef CAS PubMed.
- X. Li, R. Wang, F. Wicaksana, C. Tang, J. Torres and A. G. Fane, Preparation of high performance nanofiltration (NF) membranes incorporated with aquaporin Z, J. Membr. Sci., 2014, 450, 181–188 CrossRef CAS.
- G. Sun, T.-S. Chung, K. Jeyaseelan and A. Armugam, A layer-by-layer self-assembly approach to developing an aquaporin-embedded mixed matrix membrane, RSC Adv., 2013, 3(2), 473–481 RSC.
- Y. Yang, Y. Li, K. Goh, C. H. Tan and R. Wang, Liposomes-assisted fabrication of high performance thin film composite nanofiltration membrane, J. Membr. Sci., 2021, 620, 118833 CrossRef CAS.
- S.-J. Park, M.-S. Lee, H. Yoon, J. H. Kim, S. Jeon and S. S. Shin,
et al., Biomimetic peptoid-assisted fabrication of antibiofouling thin-film composite membranes, Chem. Eng. J., 2023, 478, 147468 CrossRef CAS.
- A. Fuwad, H. Ryu, N. Malmstadt, S. M. Kim and T.-J. Jeon, Biomimetic membranes as potential tools for water purification: Preceding and future avenues, Desalination, 2019, 458, 97–115 CrossRef CAS.
- C. Tang, Y. Zhao, R. Wang, C. Hélix-Nielsen and A. Fane, Desalination by biomimetic aquaporin membranes: Review of status and prospects, Desalination, 2013, 308, 34–40 CrossRef CAS.
- E. Abaie, L. Xu and Y.-X. Shen, Bioinspired and biomimetic membranes for water purification and chemical separation: A review, Front. Environ. Sci. Eng., 2021, 15, 1–33 CrossRef.
- Y.-L. Ji, Q.-F. An, Y.-S. Guo, W.-S. Hung, K.-R. Lee and C.-J. Gao, Bio-inspired fabrication of high perm-selectivity and anti-fouling membranes based on zwitterionic polyelectrolyte nanoparticles, J. Mater. Chem. A, 2016, 4(11), 4224–4231 RSC.
- X. Zhao, L. Cheng, R. Wang, N. Jia, L. Liu and C. Gao, Bioinspired synthesis of polyzwitterion/titania functionalized carbon nanotube membrane with superwetting property for efficient oil-in-water emulsion separation, J. Membr. Sci., 2019, 589, 117257 CrossRef CAS.
- L. B. Huang, M. Di Vincenzo, Y. Li and M. Barboiu, Artificial water channels: towards biomimetic membranes for desalination, Chem.–Eur. J., 2021, 27(7), 2224–2239 CrossRef CAS PubMed.
- Y. Zhao, C. Qiu, X. Li, A. Vararattanavech, W. Shen and J. Torres,
et al., Synthesis of robust and high-performance aquaporin-based biomimetic membranes by interfacial polymerization-membrane preparation and RO performance characterization, J. Membr. Sci., 2012, 423, 422–428 CrossRef.
- N. Kučerka, J. Gallová and D. Uhríková, The membrane structure and function affected by water, Chem. Phys. Lipids, 2019, 221, 140–144 CrossRef PubMed.
- R. Sen Gupta, N. Padmavathy and S. Bose, The journey of water remediation through biomimetic strategies: A mechanistic insight, Adv. Sustainable Syst., 2021, 5(12), 2100213 CrossRef CAS.
- P. Wagh and I. C. Escobar, Biomimetic and bioinspired membranes for water purification: A critical review and future directions, Environ. Prog. Sustainable Energy, 2019, 38(3), e13215 CrossRef.
- J. Geng, K. Kim, J. Zhang, A. Escalada, R. Tunuguntla and L. R. Comolli,
et al., Stochastic transport through carbon nanotubes in lipid bilayers and live cell membranes, Nature, 2014, 514(7524), 612–615 CrossRef CAS PubMed.
- R. H. Tunuguntla, R. Y. Henley, Y.-C. Yao, T. A. Pham, M. Wanunu and A. Noy, Enhanced water permeability and tunable ion selectivity in subnanometer carbon nanotube porins, Science, 2017, 357(6353), 792–796 CrossRef CAS PubMed.
- V. Freger, Selectivity and polarization in water channel membranes: lessons learned from polymeric membranes and CNTs, Faraday Discuss., 2018, 209, 371–388 RSC.
- M. Errico, C. Madeddu, M. F. Bindseil, S. D. Madsen, S. Braekevelt and M. S. Camilleri-Rumbau, Membrane assisted reactive distillation for bioethanol purification, Chem. Eng. Process., 2020, 157, 108110 CrossRef CAS.
- A. Aende, J. Gardy and A. Hassanpour, Seawater desalination: A review of forward osmosis technique, its challenges, and future prospects, Processes, 2020, 8(8), 901 CrossRef CAS.
- N. Abounahia, I. Ibrar, T. Kazwini, A. Altaee, A. K. Samal and S. J. Zaidi,
et al., Desalination by the forward osmosis: Advancement and challenges, Sci. Total Environ., 2023, 163901 CrossRef CAS PubMed.
- T.-S. Chung, X. Li, R. C. Ong, Q. Ge, H. Wang and G. Han, Emerging forward osmosis (FO) technologies and challenges ahead for clean water and clean energy applications, Curr. Opin. Chem. Eng., 2012, 1(3), 246–257 CrossRef CAS.
- J. Wei, C. Qiu, C. Y. Tang, R. Wang and A. G. Fane, Synthesis and characterization of flat-sheet thin film composite forward osmosis membranes, J. Membr. Sci., 2011, 372(1–2), 292–302 CrossRef CAS.
- S. T. Abdul-Hussein, M. H. Al-Furaiji, H. Meskher, D. Ghernaout, M. Fal and A. M. ALotaibi,
et al., Prospects of forward osmosis-based membranes for seawater mining: Economic analysis, limitations and opportunities, Desalination, 2024, 579, 117477 CrossRef CAS.
- B. M. Ibraheem, S. A. Aani, A. A. Alsarayreh, Q. F. Alsalhy and I. K. Salih, Forward osmosis membrane: review of fabrication, modification, challenges and potential, Membranes, 2023, 13(4), 379 CrossRef CAS PubMed.
- M. J. Park, S. Phuntsho, T. He, G. M. Nisola, L. D. Tijing and X.-M. Li,
et al., Graphene oxide incorporated polysulfone substrate for the fabrication of flat-sheet thin-film composite forward osmosis membranes, J. Membr. Sci., 2015, 493, 496–507 CrossRef CAS.
- A. Saeedi-Jurkuyeh, A. J. Jafari, R. R. Kalantary and A. Esrafili, A novel synthetic thin-film nanocomposite forward osmosis membrane modified by graphene oxide and polyethylene glycol for heavy metals removal from aqueous solutions, React. Funct. Polym., 2020, 146, 104397 CrossRef CAS.
- A. S. Reddy, V. P. Wanjari and S. P. Singh, Design, synthesis, and application of thermally responsive draw solutes for sustainable forward osmosis desalination: A review, Chemosphere, 2023, 317, 137790 CrossRef CAS PubMed.
- P. Kanagaraj, M. Shanmugaraja, D. Rana, M. Sureshkumar, K. Mahendraprabhu and I. M. Mohamed,
et al., Development of high performance thin-film (nano) composite membranes for forward osmosis desalination applications-a review, Mater. Sci. Eng., B, 2024, 299, 116966 CrossRef CAS.
- K. S. Piash and O. Sanyal, Design Strategies for Forward Osmosis Membrane Substrates with Low Structural Parameters—A Review, Membranes, 2023, 13(1), 73 CrossRef CAS PubMed.
- W. Ding, Y. Li, M. Bao, J. Zhang, C. Zhang and J. Lu, Highly permeable and stable forward osmosis (FO) membrane based on the incorporation of Al 2 O 3 nanoparticles into both substrate and polyamide active layer, RSC Adv., 2017, 7(64), 40311–40320 RSC.
- J. T. Arena, S. S. Manickam, K. K. Reimund, P. Brodskiy and J. R. McCutcheon, Characterization and performance relationships for a commercial thin film composite membrane in forward osmosis desalination and pressure retarded osmosis, Ind. Eng. Chem. Res., 2015, 54(45), 11393–11403 CrossRef CAS.
- G. Han, B. Zhao, F. Fu, T.-S. Chung, M. Weber and C. Staudt,
et al., High performance
thin-film composite membranes with mesh-reinforced hydrophilic sulfonated polyphenylenesulfone (sPPSU) substrates for osmotically driven processes, J. Membr. Sci., 2016, 502, 84–93 CrossRef CAS.
- S. Shokrollahzadeh and S. Tajik, Fabrication of thin film composite forward osmosis membrane using electrospun polysulfone/polyacrylonitrile blend nanofibers as porous substrate, Desalination, 2018, 425, 68–76 CrossRef CAS.
- P. Kallem, R. P. Pandey, H. M. Hegab, M. Patel, S. W. Hasan and F. Banat, Forward osmosis membranes for desalination and wastewater treatment: Review of recent advances in electrospun nanofiber-based substrates, challenges, and future research recommendations, J. Environ. Chem. Eng., 2023, 110231 CrossRef CAS.
- M. Obaid, Z. K. Ghouri, O. A. Fadali, K. A. Khalil, A. A. Almajid and N. A. Barakat, Amorphous SiO2 NP-incorporated poly (vinylidene fluoride) electrospun nanofiber membrane for high flux forward osmosis desalination, ACS Appl. Mater. Interfaces, 2016, 8(7), 4561–4574 CrossRef CAS PubMed.
- L. Meng, F. Niu, P. Deng, N. Li, Y. Lv and M. Huang, Electrospun nanofiber supports with bio-inspired modification enabled high-performance forward osmosis composite membranes, Compos. Commun., 2020, 22, 100473 CrossRef.
- J. M. Tharayil, P. Chinnaiyan, D. M. John and M. Kishore, Environmental sustainability of FO membrane separation applications–Bibliometric analysis and state-of-the-art review, Results Eng., 2023, 101677 Search PubMed.
- L. Miao, T. Jiang, S. Lin, T. Jin, J. Hu and M. Zhang,
et al., Asymmetric forward osmosis membranes from p-aramid nanofibers, Mater. Des., 2020, 191, 108591 CrossRef CAS.
- J. Moon and H. Kang, Thermo-responsive tributyl-4-vinylbenzylphosphonium alkanesulfonate ionic liquid-based draw solute for forward osmosis, J. Ind. Eng. Chem., 2024, 129, 413–423 CrossRef CAS.
- Y. Cho and H. Kang, Thermosensitive magnetic ionic liquids with different heterocyclic moieties as draw solutes for forward osmosis, Desalination, 2024, 569, 117045 CrossRef CAS.
- J. Moon and H. Kang, Anion Effect on Forward Osmosis Performance of Tetrabutylphosphonium-Based Draw Solute Having a Lower Critical Solution Temperature, Membranes, 2023, 13(2), 211 CrossRef CAS PubMed.
- Y. Cho and H. Kang, Dual-responsive imidazolium-based ionic liquid with different alkyl chains as draw solutes for forward osmosis, J. Environ. Chem. Eng., 2023, 11(3), 109734 CrossRef CAS.
- J. Moon and H. Kang, Effect of cation alkyl chain length on 3-sulfopropylmethacrylate-based draw solutes having lower critical solution temperature, RSC Adv., 2023, 13(12), 8291–8298 RSC.
- S. Baruah, A. Patel, A. K. Mungray and A. A. Mungray, Performance evaluation of deep eutectic solvent as a draw solute and vertical up-flow forward osmosis module for desalination of seawater, Environ. Sci. Pollut. Res., 2023, 30(11), 32108–32116 CrossRef CAS PubMed.
- A. Hassanein, M. Hafiz, M. K. Hassan, M. M. Ba-Abbad, A.-E. Maryam and R. Alfahel,
et al., Developing sustainable draw solute for forward osmosis process using poly (amidoamine) dendrimer coated magnetic nanoparticles, Desalination, 2023, 564, 116800 CrossRef CAS.
- Y. Xu, Y.-N. Wang, J. Y. Chong and R. Wang, Thermo-responsive nonionic amphiphilic copolymers as draw solutes in forward osmosis process for high-salinity water reclamation, Water Res., 2022, 221, 118768 CrossRef CAS PubMed.
- M. Edokali, R. Bocking, M. Mehrabi, A. Massey, D. Harbottle and R. Menzel,
et al., Chemical modification of reduced graphene oxide membranes: enhanced desalination performance and structural properties for forward osmosis, Chem. Eng. Res. Des., 2023, 199, 659–675 CrossRef CAS.
- D. Emadzadeh, A. Atashgar and B. Kruczek, Novel Polyelectrolyte-Based Draw Solute That Overcomes the Trade-Off between Forward Osmosis Performance and Ease of Regeneration, Membranes, 2022, 12(12), 1270 CrossRef CAS PubMed.
- M. Tang, Y. Chen, H. Xie, Q. Chen, X. Yu and R. Ou, Application of Poly (diallyl dimethylammonium chloride) as a Forward Osmosis Draw Agent: The Quantified Effect of Osmotic Pressure and Viscosity, ACS ES&T Water, 2023, 3(8), 2797–2804 Search PubMed.
- Z. Pan, X. Yang, Y. Liang, M. Lyu, Y. Huang and H. Zhou,
et al., Chromium-containing wastewater reclamation via forward osmosis with sewage sludge ash temperature-sensitive hydrogel as draw agent, J. Water Process Eng., 2023, 51, 103422 CrossRef.
- H. T. Nguyen, N. C. Nguyen, S.-S. Chen, H. C. Duong, M. L. Nguyen and C.-S. Tran,
et al., Exploration of a cost-effective draw solution based on mixing surfactant and sodium chloride for forward osmosis desalination process, Environ. Technol. Innovation, 2023, 30, 103088 CrossRef CAS.
- A. K. Ahangar and M. Taghavijeloudar, Desalination and extraction of high value bio-products from algal-rich seawater using Rhamnolipid as a bio draw solution in forward osmosis, Desalination, 2023, 568, 117017 CrossRef CAS.
- M. A. Halakarni, A. Samage, A. Mahto, V. Polisetti and S. Nataraj, Forward osmosis process for energy materials recovery from industrial wastewater with simultaneous recovery of reusable water: a sustainable approach, Mater. Today Sustainability, 2023, 22, 100361 CrossRef.
- A. H. Kamel, Q. F. Alsalhy, S. S. Ibrahim, K. A. Faneer, S. A. Hashemifard and A. Jangizehi,
et al., Novel sodium and potassium carbon quantum dots as forward osmosis draw solutes: Synthesis, characterization and performance testing, Desalination, 2023, 567, 116956 CrossRef CAS.
- S.-B. Kwon, S. Jeong, S. Hong and S. Lee, Support-free assembled molecular layer by layer-HPAN forward osmosis membranes, Desalination, 2024, 570, 117067 CrossRef CAS.
- Y.-W. Huang, C.-Y. Lee and N.-H. Tai, Support-free multi-walled carbon nanotubes-based membrane for forward osmosis applications, J. Water Process Eng., 2023, 54, 104022 CrossRef.
- S.-B. Kwon, Y. Kim, S. Lee and S. Hong, High-performance support-free molecular layer-by-layer assembled forward osmosis membrane incorporated with graphene oxide, J. Membr. Sci., 2024, 689, 122152 CrossRef CAS.
- Y. Zhou, Y. Shi, D. Cai, W. Yan, Y. Zhou and C. Gao, Support-free interfacial polymerized polyamide membrane on a macroporous substrate to reduce internal concentration polarization and increase water flux in forward osmosis, J. Membr. Sci., 2024, 689, 122165 CrossRef CAS.
- H. Wen, N. Xu, F. Soyekwo, P. Dou and C. Liu, Towards enhanced performance of fertilizer-drawn forward osmosis process coupled with sludge thickening using a thin-film nanocomposite membrane interlayered with Mxene scaffolded alginate hydrogel, J. Membr. Sci., 2023, 685, 121899 CrossRef CAS.
- H. Huang, H. Zhang, F. Xiao, J. Liang and Y. Wu, Efficient removal of fluoride ion by the composite forward osmosis membrane with modified cellulose nanocrystal interlayer, Results Eng., 2023, 20, 101449 CrossRef CAS.
- L. Jiang, M. Rastgar, A. Mohammadnezhad, C. Wang and M. Sadrzadeh, Development of Superpermeable Wood-Based Forward Osmosis Membranes, ACS Sustain. Chem. Eng., 2023, 11(10), 4155–4167 CrossRef CAS.
- W. Zhao, X. Zhang, C. Li, L. Xu, Q. Meng and G. Zhang, Polyamide Membrane Tailored by Codeposition of a UiO-66 Metal–Organic Framework Nanostructure-Based Interlayer for Forward Osmosis, ACS Appl. Nano Mater., 2023, 6(8), 6901–6910 CrossRef CAS.
- T.-X. Ren, M.-X. Zhang, Z.-L. Xu, P.-P. Li, D. Pandaya and X.-G. Jin,
et al., Forward osmosis TFN membrane prepared by adjusting a PDA/GO-Fe3+-TA interlayer on porous CTA substrates, Sep. Purif. Technol., 2024, 330, 125353 CrossRef CAS.
- M. He, Y. Li, L. Feng, J. Wang, Z. Liu and L. Wang,
et al., Mechanism for regulation of a forward osmosis membrane by the SA/UiO-66-NH2 composite interlayer and the resulting lithium ion concentration performance, J. Cleaner Prod., 2023, 410, 137282 CrossRef CAS.
- H. Zhang, J. Liang, Z. Li, F. Xiao, Y. Wu and C. Zhang, Preparation of Forward osmosis composite
membrane with Chitosan/Tannic acid co-deposition interlayer and its fluoride removal performance, Sep. Purif. Technol., 2024, 330, 125300 CrossRef CAS.
- J. Wang, L. Wang, M. He, X. Wang, Y. Lv and D. Huang,
et al., Highly permeable thin film nanocomposite membrane utilizing a MoS2@ NH2-UiO-66 interlayer for forward osmosis removal of Co2+, Sr2+ and Cs+ nuclide ions, Appl. Surf. Sci., 2023, 611, 155618 CrossRef CAS.
- H. Rafiee, A. Shakeri and H. Mahdavi, Layer-by-layer assembly of alginate/Ca2+ as interlayer on microfiltration substrate: Fabrication of high selective thin-film composite forward osmosis membrane for efficient heavy metal ions removal, Chem. Eng. Res. Des., 2022, 188, 564–574 CrossRef CAS.
- H. Liu, B. Li, P. Zhao, R. Xu, C. Y. Tang and W. Song,
et al., Fabrication of novel thin-film composite membrane based on ultrathin metal-organic framework interlayer for enhancing forward osmosis performance, Chin. Chem. Lett., 2023, 34(12), 108369 CrossRef CAS.
- P. Karami, M. M. Haque Mizan, B. Khorshidi, S. Aghapour Aktij, A. Rahimpour and J. B. Soares,
et al., Novel Forward Osmosis Membranes Engineered with Polydopamine/Graphene Oxide Interlayers: Synergistic Impact of Monomer Reactivity and Hydrophilic Interlayers, Ind. Eng. Chem. Res., 2023, 62(30), 11965–11976 CrossRef CAS.
- J. Yu, W. Jing, E. Liu, S. Du, H. Cai and H. Du,
et al., Sulfonated graphene oxide modified polysulfone-polyamide forward osmosis membrane and its application in fluorine-containing wastewater treatment, Mater. Chem. Phys., 2024, 313, 128757 CrossRef CAS.
- R. Lakra, M. R. Bilad, M. Balakrishnan and S. Basu, Development of high-performance CuBTC MOF-based forward osmosis (FO) membranes and their cleaning strategies, Chem. Eng. Res. Des., 2023, 190, 566–579 CrossRef CAS.
- S. T. Abdul-Hussein, Q. F. Alsalhy, M. H. Al-Furaiji, F. Russo, G. Chiappetta and G. Di Luca,
et al., Systematic investigation of MAX phase (Ti3AlC2) modified polyethersulfone membrane performance for forward osmosis applications in desalination, Arabian J. Chem., 2024, 17(1), 105475 CrossRef CAS.
- N. Li, Y. Zhang, P. Li, B. Zhu, W. Wang and Z. Xu, Enhanced permeability and biofouling mitigation of forward osmosis membranes via grafting graphene quantum dots, Front. Chem. Sci. Eng., 2023, 17(10), 1470–1483 CrossRef CAS.
- R. Ghalavand, M. Mokhtary, A. Shakeri and O. Alizadeh, Polyacrylamide-grafted zinc oxide (ZnO-g-PAM) nanoparticles as a promising nanofiller for thin-film nanocomposite forward osmosis membranes, J. Nanopart. Res., 2023, 25(1), 12 CrossRef CAS.
- G. Hakimi, A. Shakeri and H. Salehi, In-situ generated ZnO nanoparticles for enhanced performance of thin film nanocomposite membrane in forward osmosis process, J. Appl. Polym. Sci., 2024, 141(7), e54951 CrossRef CAS.
- M. Yassari, A. Shakeri, P. Karami and M. Sadrzadeh, Hydrophilic Antifouling Thin-Film Nanocomposite Forward Osmosis Membranes: Effect of Zwitterion-Functionalized Carbon Nanofiber Modification, Ind. Eng. Chem. Res., 2023, 63(1), 566–578 CrossRef.
- R. Mazhari, Y. Bide, S. S. Hosseini and S. Shokrollahzadeh, Modification of polyacrylonitrile TFC-FO membrane by biowaste-derived hydrophilic N-doped carbon quantum dots for enhanced water desalination performance, Desalination, 2023, 565, 116888 CrossRef CAS.
- W.-S. Zou, P. Wu, B. Xiao, X. Chen, W. Kong and Y. Li,
et al., Integration of nitrogen-doped carbon dots onto active layer of forward osmosis membrane for highly efficient antibacteria and enhanced membrane performances, J. Environ. Chem. Eng., 2023, 11(2), 109468 CrossRef CAS.
- M. Golgoli, J. Farahbakhsh, A. H. Asif, M. Khiadani, A. Razmjou and M. L. Johns,
et al., Harnessing the power of metal-organic frameworks to develop microplastic fouling resistant forward osmosis membranes, J. Membr. Sci., 2023, 682, 121766 CrossRef CAS.
- M. Vafaei, S. Farnia and A. Shakeri, Covalent organic frameworks modified with TA embedded in the membrane to improve the separation of heavy metals in the FO, Surf. Interfaces, 2023, 39, 102963 CrossRef CAS.
- M. Amini, A. Haji Hosseinzadeh, M. Nikkhoo, M. Hosseinifard, A. Namvar and H. Naslhajian,
et al., High-Performance Novel Polyoxometalate-LDH Nanocomposite-Modified Thin-Film Nanocomposite Forward Osmosis Membranes: A Study of Desalination and Antifouling Performance, Langmuir, 2023, 39(41), 14528–14538 CrossRef CAS PubMed.
- Y. Zhu, Y. Xu, S. Chen, Y. Zhou, J. Zhu and G. Chen, (3-Aminopropyl) trimethoxysilane-Functionalized Titanium Dioxide Thin-Film Nanocomposite Membrane: Enhanced Rejection Performance for Unary and Binary High-Salt Wastewater, ACS Appl. Polym. Mater., 2023, 5(12), 10137–10147 CrossRef CAS.
- Z. Geng, X. Zhao, Y. Fan, C. Wang, H. Huo and X. Yang,
et al., Multi-functional Ag@ NH2-UiO-66/PAES-COOH self-supporting symmetric hybrid membrane for forward osmosis separation, J. Membr. Sci., 2023, 675, 121538 CrossRef CAS.
- T. Li, X. Ba, X. Wang, Z. Wang, J. Yang and Y. Cui,
et al., MIL-53 (Fe)@ γ-Al 2 O 3 nanocomposites incorporated cellulose acetate for forward osmosis membranes of high desalination performance, Environ. Eng. Res., 2023, 28(1), 210448 CrossRef.
- M. Bagherzadeh, M. Nikkhoo, M. M. Ahadian, A. Bayrami and M. Amini, Polyethersulfone/polyamide thin-film nanocomposite membrane decorated by WS2-Cys-UiO-66-(CO2H) 2 nanocomposites for forward osmosis, J. Environ. Chem. Eng., 2023, 11(3), 109959 CrossRef CAS.
- P. Zhao, Y. Hu, X. An, R. Ji, H. Liu and H. Zhao,
et al., Polymeric PDI-based photocatalytic nanoarchitectures promoting the performance of thin film composite membrane for forward osmosis water purification, Chem. Eng. J., 2023, 476, 146747 CrossRef CAS.
- Y. Hu, P. Zhao, H. Liu, X. Yi, W. Song and X. Wang, Photocatalytic thin film composite forward osmosis membrane for mitigating organic fouling in active layer facing draw solution mode, Chin. Chem. Lett., 2023, 34(6), 107931 CrossRef CAS.
- T. Kim, Y. Lee, E. Kim and K. Kim, Fouling-resistant surface modification of forward osmosis membranes using MoS2-Ag nanofillers, Surf. Interfaces, 2023, 38, 102844 CrossRef CAS.
- M. Amini, M. Nikkhoo, M. Bagherzadeh, M. M. Ahadian, A. Bayrami and H. Naslhajian,
et al., High-Performance Novel MoS2@ Zeolite X Nanocomposite-Modified Thin-Film Nanocomposite Forward Osmosis Membranes: A Study of Desalination and Antifouling Performance, ACS Appl. Mater. Interfaces, 2023, 15(33), 39765–39776 CrossRef CAS PubMed.
- X. Zhang, H.-M. Cui, Y. Gao, Z.-W. Yan, X. Yan and Y. Chen,
et al., Surfactant-induced intervention in interfacial polymerization to develop highly-selective thin-film composite membrane for forward osmosis process, Desalination, 2023, 558, 116617 CrossRef CAS.
- K. Sun, Q. Lyu, X. Zheng, R. Liu, C. Y. Tang and M. Zhao,
et al., Enhanced water treatment performance of ceramic-based forward osmosis membranes via MOF interlayer, Water Res., 2024, 254, 121395 CrossRef CAS PubMed.
- Y. Wibisono, V. Noviani, A. T. Ramadhani, L. A. Devianto and A. A. Sulianto, Eco-friendly forward osmosis membrane manufacturing using dihydrolevoglucosenone, Results Eng., 2022, 16, 100712 CrossRef CAS.
- W. Zeng, M. Yu, J. Lin, L. Huang, J. Li and S. Lin,
et al., Electrospun chitosan nanofiber constructing superhigh-water-flux forward osmosis membrane, Int. J. Biol. Macromol., 2023, 226, 833–839 CrossRef CAS PubMed.
- B. E. Khan, A. Mahmood, M. Zaman and K.-H. Lee, Zinc sulfate as a draw solute in forward osmosis and its regeneration by reagent precipitation, Desalination, 2024, 571, 117087 CrossRef CAS.
- X. Li, S. Chou, R. Wang, L. Shi, W. Fang and G. Chaitra,
et al., Nature gives the best solution for desalination: Aquaporin-based hollow fiber composite membrane with superior performance, J. Membr. Sci., 2015, 494, 68–77 CrossRef CAS.
- X. Bao, Q. Wu, J. Tian, W. Shi, W. Wang and Z. Zhang,
et al., Fouling mechanism of forward osmosis membrane in domestic wastewater concentration: Role of substrate structures, Chem. Eng. J., 2019, 370, 262–273 CrossRef CAS.
- D. Zhao, S. Chen, C. X. Guo, Q. Zhao and X. Lu, Multi-functional forward osmosis draw solutes for seawater desalination, Chin. J. Chem. Eng., 2016, 24(1), 23–30 CrossRef CAS.
- S. Subramani, R. C. Panda and B. Panda, Studies on performances of membrane, draw solute and modeling of forward osmosis process in desalination—A review, Desalin. Water Treat., 2017, 70, 46–63 CrossRef CAS.
- N. M. Mazlan, D. Peshev and A. G. Livingston, Energy consumption for desalination—A comparison of forward osmosis with reverse osmosis, and the potential for perfect membranes, Desalination, 2016, 377, 138–151 CrossRef CAS.
- B. Bolto, M. Hoang and T. Tran, Less commonly used membrane desalination technologies, Arch. Environ. Prot., 2008, 5–16 CAS.
- F. E. Ahmed, R. Hashaikeh and N. Hilal, Hybrid technologies: The future of energy efficient desalination–A review, Desalination, 2020, 495, 114659 CrossRef CAS.
- Y. Xu, Y. Zhu, Z. Chen, J. Zhu and G. Chen, A comprehensive review on forward osmosis water treatment: Recent advances and prospects of membranes and draw solutes, Int. J. Environ. Res. Public Health, 2022, 19(13), 8215 CrossRef CAS PubMed.
- J. R. McCutcheon, R. L. McGinnis and M. Elimelech, A novel ammonia—carbon dioxide forward (direct) osmosis desalination process, Desalination, 2005, 174(1), 1–11 CrossRef CAS.
- J. R. McCutcheon, R. L. McGinnis and M. Elimelech, Desalination by ammonia–carbon dioxide forward osmosis: influence of draw and feed solution concentrations on process performance, J. Membr. Sci., 2006, 278(1–2), 114–123 CrossRef CAS.
- R. L. McGinnis and M. Elimelech, Energy requirements of ammonia–carbon dioxide forward osmosis desalination, Desalination, 2007, 207(1–3), 370–382 CrossRef CAS.
- V. Yangali-Quintanilla, Z. Li, R. Valladares, Q. Li and G. Amy, Indirect desalination of Red Sea water with forward osmosis and low pressure reverse osmosis for water reuse, Desalination, 2011, 280(1–3), 160–166 CrossRef CAS.
- M. Salamanca, M. Peña, A. Hernandez, P. Prádanos and L. Palacio, Forward Osmosis Application for the Removal of Emerging Contaminants from Municipal Wastewater: A Review, Membranes, 2023, 13(7), 655 CrossRef CAS PubMed.
- S. K. Singh, C. Sharma and A. Maiti, A comprehensive review of standalone and hybrid forward osmosis for water treatment: Membranes and recovery strategies of draw solutions, J. Environ. Chem. Eng., 2021, 9(4), 105473 CrossRef CAS.
- C. XianáGuo, Na+-functionalized carbon quantum dots: a new draw solute in forward osmosis for seawater desalination, Chem. Commun., 2014, 50(55), 7318–7321 RSC.
- C. F. Wan and T.-S. Chung, Techno-economic evaluation of various RO+ PRO and RO+ FO integrated processes, Appl. Energy, 2018, 212, 1038–1050 CrossRef CAS.
- L. Luo, Z. Zhou, T.-S. Chung, M. Weber, C. Staudt and C. Maletzko, Experiments and modeling of boric acid permeation through double-skinned forward osmosis membranes, Environ. Sci. Technol., 2016, 50(14), 7696–7705 CrossRef CAS PubMed.
- E. Klein, Affinity membranes: a 10-year review, J. Membr. Sci., 2000, 179(1–2), 1–27 CrossRef CAS.
- W. Sun, J. Shi, C. Chen, N. Li, Z. Xu and J. Li,
et al., A review on organic–inorganic hybrid nanocomposite membranes: A versatile tool to overcome the barriers of forward osmosis, RSC Adv., 2018, 8(18), 10040–10056 RSC.
- T. Wu, B. Zhou, T. Zhu, J. Shi, Z. Xu and C. Hu,
et al., Facile and low-cost approach towards a PVDF ultrafiltration membrane with enhanced hydrophilicity and antifouling performance via graphene oxide/water-bath coagulation, RSC Adv., 2015, 5(11), 7880–7889 RSC.
- X. Song, L. Wang, C. Y. Tang, Z. Wang and C. Gao, Fabrication of carbon nanotubes incorporated double-skinned thin film nanocomposite membranes for enhanced separation performance and antifouling capability in forward osmosis process, Desalination, 2015, 369, 1–9 CrossRef CAS.
- M. Elma, D. R. Mujiyanti, N. M. Ismail, M. R. Bilad, A. Rahma and S. K. Rahman,
et al., Development of hybrid and templated silica-P123 membranes for brackish water desalination, Polymers, 2020, 12(11), 2644 CrossRef CAS PubMed.
- S. Wang, D. K. Wang, K. S. Jack, S. Smart and J. C. D. da Costa, Improved hydrothermal stability of silica materials prepared from ethyl silicate 40, RSC Adv., 2015, 5(8), 6092–6099 RSC.
- A. I. Schäfer and B. S. Richards, Testing of a hybrid membrane system for groundwater desalination in an Australian national park, Desalination, 2005, 183(1–3), 55–62 CrossRef.
- M. A. Al-Obaidi, R. H. Zubo, F. L. Rashid, H. J. Dakkama, R. Abd-Alhameed and I. M. Mujtaba, Evaluation of solar energy powered seawater desalination processes: a review, Energies, 2022, 15(18), 6562 CrossRef CAS.
- M. Al-Obaidi, A. A. Alsarayreh, F. L. Rashid, M. T. Sowgath, S. Alsadaie and A. Ruiz-García,
et al., Hybrid membrane and thermal seawater desalination processes powered by fossil fuels: A comprehensive review, future challenges and prospects, Desalination, 2024, 117694 CrossRef CAS.
- Y. Dong, H. Wu, F. Yang and S. Gray, Cost and efficiency perspectives of ceramic membranes for water treatment, Water Res., 2022, 220, 118629 CrossRef CAS PubMed.
- S. Mestre, A. Gozalbo, M. Lorente-Ayza and E. Sánchez, Low-cost ceramic membranes: A research opportunity for industrial application, J. Eur. Ceram. Soc., 2019, 39(12), 3392–3407 CrossRef CAS.
- B. Nandi, R. Uppaluri and M. Purkait, Identification of optimal membrane morphological parameters during microfiltration of mosambi juice using low cost ceramic membranes, LWT Food Sci. Technol., 2011, 44(1), 214–223 CrossRef CAS.
- P. Monash and G. Pugazhenthi, Development of ceramic supports derived from low-cost raw materials for membrane applications and its optimization based on sintering temperature, Int. J. Appl. Ceram. Technol., 2011, 8(1), 227–238 CrossRef CAS.
- D. Vasanth, R. Uppaluri and G. Pugazhenthi, Influence of sintering temperature on the properties of porous ceramic support prepared by uniaxial dry compaction method using low-cost raw materials for membrane applications, Sep. Sci. Technol., 2011, 46(8), 1241–1249 CrossRef CAS.
- A. Abdullayev, M. F. Bekheet, D. A. Hanaor and A. Gurlo, Materials and applications for low-cost ceramic membranes, Membranes, 2019, 9(9), 105 CrossRef CAS PubMed.
- L. Qiao, S. Liu, M. Fang, M. Yang and X. Ma, A Composite Membrane with High Stability and Low Cost Specifically for Iron–Chromium Flow Battery, Polymers, 2022, 14(11), 2245 CrossRef CAS PubMed.
- A. Alsalme, Organic-Inorganic hybrid membrane for simultaneous heavy metal removal at drinking water pollution concentration levels, Microchem. J., 2023, 195, 109447 CrossRef CAS.
- D. Unlu, Water Desalination by Pervaporation Using MIL-101 (Cr) and MIL-101 (Cr)@ GODoped PVA Hybrid Membranes, Water, Air, Soil Pollut., 2023, 234(2), 96 CrossRef CAS.
- B. Sun, M. Wu, H. Zhen, Y. Jia, P. Li and Z. Yuan,
et al., Tailored PVDF membrane with coordinated interfacial nano/micro-structure for enhanced membrane distillation, Desalination, 2024, 573, 117177 CrossRef CAS.
- S. Tang, J. Yang, B. Wu, J. Zhang, J. Li and B. He,
et al., Fabrication of hollow fiber nanofiltration membrane with high permselectivity based on “Co-deposition, biomineralization and dual cross-linking”
process, J. Membr. Sci., 2023, 670, 121388 CrossRef CAS.
- R. Xu, S. Cheng, X. Cheng, L. Qi, J. Zhong and G. Liu,
et al., In situ carboxyl functionalization of hybrid organosilica reverse osmosis membranes for water desalination, Adv. Compos. Hybrid Mater., 2023, 6(4), 153 CrossRef CAS.
- Y. Wan, L. Yao and P. Cui, Graphene quantum dots doped poly (vinyl alcohol) hybrid membranes for Desalination via Pervaporation, Chin. J. Chem. Eng., 2023, 63, 226–234 CrossRef CAS.
- S. Zeng, Q. He, Q. Wang, J. Si and Z. Cui, Phase-transitioned lysozyme-mediated fabrication of zeolitic imidazole framework-8 hybrid membrane with enhanced separation and antifouling properties, J. Environ. Chem. Eng., 2023, 11(5), 110838 CrossRef CAS.
- Y. Lai, J. He, Y. Li, S. Zhou, W. Yan and Y. Zhou,
et al., Preparation of hydrophilic modified ZIF-8 and its application in the preparation of nanocomposite matrix reverse osmosis membrane with improved permeation performance, J. Coat. Technol. Res., 2024, 21(2), 683–692 CrossRef CAS.
- J. García-Chirino, A. D. Jiménez and B. Van der Bruggen, Hybrid Na-A zeolite/oxycut residue thin film composite nanofiltration membrane for Cr (III) removal, J. Environ. Chem. Eng., 2023, 11(2), 109351 CrossRef.
- D. Jiang, N. Zhang, G. He and A. Wang, Sandwich-structured covalent organic framework membranes for selective sodium ion transport, Desalination, 2023, 567, 116988 CrossRef CAS.
- C. Mendes-Felipe, A. Veloso-Fernández, J. L. Vilas-Vilela and L. Ruiz-Rubio, Hybrid organic–inorganic membranes for photocatalytic water remediation, Catalysts, 2022, 12(2), 180 CrossRef CAS.
- S. Mbakop, L. N. Nthunya and M. S. Onyango, Recent advances in the synthesis of nanocellulose functionalized–hybrid membranes and application in water quality improvement, Processes, 2021, 9(4), 611 CrossRef CAS.
- A. Khosla, Sonu, H. T. A. Awan, K. Singh, Gaurav and R. Walvekar,
et al., Emergence of MXene and MXene–polymer hybrid membranes as future-environmental remediation strategies, Adv. Sci., 2022, 9(36), 2203527 CrossRef CAS PubMed.
- V. Chaudhary, N. Ashraf, M. Khalid, R. Walvekar, Y. Yang and A. Kaushik,
et al., Emergence of MXene–polymer hybrid nanocomposites as high-performance next-generation chemiresistors for efficient air quality monitoring, Adv. Funct. Mater., 2022, 32(33), 2112913 CrossRef CAS.
- C. Ma, M. G. Ma, C. Si, X. X. Ji and P. Wan, Flexible MXene-based composites for wearable devices, Adv. Funct. Mater., 2021, 31(22), 2009524 CrossRef CAS.
- M. Liu, J. Wang, P. Song, J. Ji and Q. Wang, Metal-organic frameworks-derived In2O3 microtubes/Ti3C2Tx MXene composites for NH3 detection at room temperature, Sens. Actuators, B, 2022, 361, 131755 CrossRef CAS.
- A. W. Carpenter, C.-F. de Lannoy and M. R. Wiesner, Cellulose nanomaterials in water treatment technologies, Environ. Sci. Technol., 2015, 49(9), 5277–5287 CrossRef CAS PubMed.
-
M. Börjesson and G. Westman, Crystalline nanocellulose—preparation, modification, and properties, Cellulose-Fundamental Aspects and Current Trends, 2015, vol. 7 Search PubMed.
- K. Maleski, V. N. Mochalin and Y. Gogotsi, Dispersions of two-dimensional titanium carbide MXene in organic solvents, Chem. Mater., 2017, 29(4), 1632–1640 CrossRef CAS.
- Z. Wu, W. Fu, H. Xu, R. Zheng, F. Han and Z. Liang,
et al., A simple preparation method of in situ oxidized titanium carbide MXene for photocatalytic degradation of catechol, New J. Chem., 2022, 46(19), 9364–9371 RSC.
- S. M. Alardhi, N. S. Ali, N. M. C. Saady, S. Zendehboudi, I. K. Salih and J. M. Alrubaye,
et al., Separation techniques in different configurations of hybrid systems via synergetic adsorption
and membrane processes for water treatment: a review, J. Ind. Eng. Chem., 2024, 130, 91–104 CrossRef CAS.
- S. Jamaly, A. Giwa and S. W. Hasan, Recent improvements in oily wastewater treatment: Progress, challenges, and future opportunities, J. Environ. Sci., 2015, 37, 15–30 CrossRef CAS PubMed.
- M. Xie, L. D. Nghiem, W. E. Price and M. Elimelech, Comparison of the removal of hydrophobic trace organic contaminants by forward osmosis and reverse osmosis, Water Res., 2012, 46(8), 2683–2692 CrossRef CAS PubMed.
- A. Giwa, S. Hasan, A. Yousuf, S. Chakraborty, D. Johnson and N. Hilal, Biomimetic membranes: A critical review of recent progress, Desalination, 2017, 420, 403–424 CrossRef CAS.
- V. Sharma, G. Borkute and S. P. Gumfekar, Biomimetic nanofiltration membranes: Critical review of materials, structures, and applications to water purification, Chem. Eng. J., 2022, 433, 133823 CrossRef CAS.
- A. Ismail, M. Padaki, N. Hilal, T. Matsuura and W. Lau, Thin film composite membrane—Recent development and future potential, Desalination, 2015, 356, 140–148 CrossRef CAS.
- J. Li, M. Wei and Y. Wang, Substrate matters: The influences of substrate layers on the performances of thin-film composite reverse osmosis membranes, Chin. J. Chem. Eng., 2017, 25(11), 1676–1684 CrossRef.
- M. R. Esfahani, S. A. Aktij, Z. Dabaghian, M. D. Firouzjaei, A. Rahimpour and J. Eke,
et al., Nanocomposite membranes for water separation and purification: Fabrication, modification, and applications, Sep. Purif. Technol., 2019, 213, 465–499 CrossRef CAS.
- A. Sotto, A. Boromand, R. Zhang, P. Luis, J. M. Arsuaga and J. Kim,
et al., Effect of nanoparticle aggregation at low concentrations of TiO2 on the hydrophilicity, morphology, and fouling resistance of PES–TiO2 membranes, J. Colloid Interface Sci., 2011, 363(2), 540–550 CrossRef CAS PubMed.
- Y. M. Mojtahedi, M. R. Mehrnia and M. Homayoonfal, Fabrication of Al2O3/PSf nanocomposite membranes: efficiency comparison of coating and blending methods in modification of filtration performance, Desalin. Water Treat., 2013, 51(34–36), 6736–6742 CrossRef CAS.
- M. Homayoonfal, M. R. Mehrnia, Y. M. Mojtahedi and A. F. Ismail, Effect of metal and metal oxide nanoparticle impregnation route on structure and liquid filtration performance of polymeric nanocomposite membranes: a comprehensive review, Desalin. Water Treat., 2013, 51(16–18), 3295–3316 CrossRef CAS.
- J. Yin, G. Huang, H. Xiao, N. Chen, C. An and T.-C. Chao,
et al., Bioinspired and dual-functional nanocellulose aerogels for water disinfection and heavy metal removal, Nano Today, 2023, 51, 101918 CrossRef CAS.
- B. Bhide and S. Stern, A new evaluation of membrane processes for the oxygen-enrichment of air. I. Identification of optimum operating conditions and process configuration, J. Membr. Sci., 1991, 62(1), 13–35 CrossRef CAS.
- V. Singh, N. Meena, A. Golder and C. Das, Fabrication of cost effective iron ore slime ceramic membrane for the recovery of organic solvent used in coke production, Int. J. Coal Sci. Technol., 2016, 3, 226–234 CrossRef CAS.
-
M. Lee, Z. Wu and K. Li, Advances in ceramic membranes for water treatment, Advances in Membrane Technologies for Water Treatment, Elsevier, 2015, pp. 43–82 Search PubMed.
- P. Jarvis, I. Carra, M. Jafari and S. J. Judd, Ceramic vs polymeric membrane implementation for potable water treatment, Water Res., 2022, 215, 118269 CrossRef CAS PubMed.
- B. S. Al-Anzi and O. C. Siang, Recent developments of carbon based nanomaterials and membranes for oily wastewater treatment, RSC Adv., 2017, 7(34), 20981–20994 RSC.
- M. Kamali, D. Suhas, M. E. Costa, I. Capela and T. M. Aminabhavi, Sustainability considerations in membrane-based technologies for industrial effluents treatment, Chem. Eng. J., 2019, 368, 474–494 CrossRef CAS.
- S. M. Samaei, S. Gato-Trinidad and A. Altaee, The application of pressure-driven ceramic membrane technology for the treatment of industrial wastewaters–A review, Sep. Purif. Technol., 2018, 200, 198–220 CrossRef CAS.
- D. Cohen-Tanugi, R. K. McGovern, S. H. Dave, J. H. Lienhard and J. C. Grossman, Quantifying the potential of ultra-permeable membranes for water desalination, Energy Environ. Sci., 2014, 7(3), 1134–1141 RSC.
- M. Bodzek, K. Konieczny and A. Kwiecinska-Mydlak, Application of nanotechnology and nanomaterials in water and wastewater treatment: membranes, photocatalysis and disinfection, Desalin. Water Treat., 2020, 186, 88–106 CrossRef CAS.
- J. H. Low, J. Zhang, W. P. Li, T. Yang, C. F. Wan and F. Esa,
et al., Industrial scale thin-film composite membrane modules for salinity-gradient energy harvesting through pressure retarded osmosis, Desalination, 2023, 548, 116217 CrossRef CAS.
- S. E. Skilhagen, J. E. Dugstad and R. J. Aaberg, Osmotic power—power production based on the osmotic pressure difference between waters with varying salt gradients, Desalination, 2008, 220(1–3), 476–482 CrossRef CAS.
- C. F. Wan, B. Li, T. Yang and T.-S. Chung, Design and fabrication of inner-selective thin-film composite (TFC) hollow fiber modules for pressure retarded osmosis (PRO), Sep. Purif. Technol., 2017, 172, 32–42 CrossRef CAS.
- P. Goh, A. Ismail and N. Hilal, Nano-enabled membranes technology: sustainable and revolutionary solutions for membrane desalination?, Desalination, 2016, 380, 100–104 CrossRef CAS.
- M. B. Asif and Z. Zhang, Ceramic membrane technology for water and wastewater treatment: A critical review of performance, full-scale applications, membrane fouling and prospects, Chem. Eng. J., 2021, 418, 129481 CrossRef CAS.
-
K. A. Khalil, Advanced sintering of nano-ceramic materials, Ceramic Materials-Progress in Modern Ceramics, 2012, pp. 65–82 Search PubMed.
- S. Huang, R. H. Ras and X. Tian, Antifouling membranes for oily wastewater treatment: Interplay between wetting and membrane fouling, Curr. Opin. Colloid Interface Sci., 2018, 36, 90–109 CrossRef CAS.
- M. Qasim, M. Badrelzaman, N. N. Darwish, N. A. Darwish and N. Hilal, Reverse osmosis desalination: A state-of-the-art review, Desalination, 2019, 459, 59–104 CrossRef CAS.
- R. Sirohi, Y. Kumar, A. Madhavan, N. A. Sagar, R. Sindhu and B. Bharathiraja,
et al., Engineered nanomaterials for water desalination: Trends and challenges, Environ. Technol. Innovation, 2023, 30, 103108 CrossRef CAS.
- A. Abdelrasoul, H. Doan, A. Lohi and C.-H. Cheng, Aquaporin-based biomimetic and bioinspired membranes for new frontiers in sustainable water treatment technology: approaches and challenges, Polym. Sci., Ser. A, 2018, 60, 429–450 CrossRef CAS.
- M. Tian, H. Xu, L. Yao and R. Wang, A biomimetic antimicrobial surface for membrane fouling control in reverse osmosis for seawater desalination, Desalination, 2021, 503, 114954 CrossRef CAS.
- Y. Wang, Z. Fang, S. Zhao, D. Ng, J. Zhang and Z. Xie, Dopamine incorporating forward osmosis membranes with enhanced selectivity and antifouling properties, RSC Adv., 2018, 8(40), 22469–22481 RSC.
- R. Alfahel, R. S. Azzam, M. Hafiz, A. H. Hawari, R. P. Pandey and K. A. Mahmoud,
et al., Fabrication of fouling resistant Ti3C2Tx (MXene)/cellulose acetate nanocomposite membrane for forward osmosis application, J. Water Process Eng., 2020, 38, 101551 CrossRef.
- M. Bagherzadeh, M. Nikkhoo, M. M. Ahadian and M. Amini, Novel thin-film nanocomposite forward osmosis membranes modified with WS2/CuAl LDH nanocomposite to enhance desalination and anti-fouling performance, J. Inorg. Organomet. Polym. Mater., 2023, 33(4), 956–968 CrossRef CAS.
- S. F. Seyedpour, A. Rahimpour, A. A. Shamsabadi and M. Soroush, Improved performance and antifouling properties of thin-film composite polyamide membranes modified with nano-sized bactericidal graphene quantum dots for forward
osmosis, Chem. Eng. Res. Des., 2018, 139, 321–334 CrossRef CAS.
- H. Salehi, M. Rastgar and A. Shakeri, Anti-fouling and high water permeable forward osmosis membrane fabricated via layer by layer assembly of chitosan/graphene oxide, Appl. Surf. Sci., 2017, 413, 99–108 CrossRef CAS.
- V. Pesarakloo, V. Alipour and A. H. Javid, Technical and Economic of Various Pretreatment Methods for Desalination of Seawater Using Reverse Osmosis, Int. J. Environ. Sci. Technol., 2024, 1–16 Search PubMed.
- F. Galiano, S. A. Schmidt, X. Ye, R. Kumar, R. Mancuso and E. Curcio,
et al., UV-LED induced bicontinuous microemulsions polymerisation for surface modification of commercial membranes–Enhancing the antifouling properties, Sep. Purif. Technol., 2018, 194, 149–160 CrossRef CAS.
- D. Rana and T. Matsuura, Surface modifications for antifouling membranes, Chem. Rev., 2010, 110(4), 2448–2471 CrossRef CAS PubMed.
-
Y. Fang, Z. K. Xu and J. Wu, Surface modification of membranes. Encyclopedia of Membrane Science and Technology, 2013, pp. 1–15 Search PubMed.
-
B. Ladewig and M. N. Z. Al-Shaeli, Fundamentals of membrane bioreactors, Springer Transactions in Civil and Environmental Engineering, Springer, 2017, vol. 2363, p. 150 Search PubMed.
- G. S. Ajmani, D. Goodwin, K. Marsh, D. H. Fairbrother, K. J. Schwab and J. G. Jacangelo,
et al., Modification of low pressure membranes with carbon nanotube layers for fouling control, Water Res., 2012, 46(17), 5645–5654 CrossRef CAS PubMed.
- H. M. Hegab, Y. Wimalasiri, M. Ginic-Markovic and L. Zou, Improving the fouling resistance of brackish water membranes via surface modification with graphene oxide functionalized chitosan, Desalination, 2015, 365, 99–107 CrossRef CAS.
- D. I. Petukhov and D. J. Johnson, Membrane modification with carbon nanomaterials for fouling mitigation: A review, Adv. Colloid Interface Sci., 2024, 103140 CrossRef CAS PubMed.
- N. Wang, R. Zhang, K. Liu, Y. Zhang, X. Shi and W. Sand,
et al., Application of nanomaterials in antifouling: A review, Nano Mater. Sci., 2024, 672–700 CrossRef CAS.
- M. A. Kivi, H. Alinia, Y. Jafarzadeh and R. Yegani, High-density polyethylene membranes embedded with carboxylated and polyethylene glycol-grafted nanodiamond to be used in membrane bioreactors, J. Appl. Polym. Sci., 2019, 136(35), 47914 CrossRef.
- S. Hoseinpour, Y. Jafarzadeh, R. Yegani and S. Masoumi, Embedding neat and carboxylated nanodiamonds into polypropylene membranes to enhance antifouling properties, Polyolefins J., 2019, 6(1), 63–74 CAS.
- D. Georgouvelas, B. Jalvo, L. Valencia, W. Papawassiliou, A. J. Pell and U. Edlund,
et al., Residual lignin and zwitterionic polymer grafts on cellulose nanocrystals for antifouling and antibacterial applications, ACS Appl. Polym. Mater., 2020, 2(8), 3060–3071 CrossRef CAS.
- D. Xia, M. Zhang, C. Tong, Z. Wang, H. Liu and L. Zhu, In-situ incorporating zwitterionic nanocellulose into polyamide nanofiltration membrane towards excellent perm-selectivity and antifouling performances, Desalination, 2022, 521, 115397 CrossRef CAS.
- B. R. Knowles, P. Wagner, S. Maclaughlin, M. J. Higgins and P. J. Molino, Silica nanoparticles functionalized with zwitterionic sulfobetaine siloxane for application as a versatile antifouling coating system, ACS Appl. Mater. Interfaces, 2017, 9(22), 18584–18594 CrossRef CAS PubMed.
- T. Sullivan and I. O'Callaghan, Recent developments in biomimetic antifouling materials: A review, Biomimetics, 2020, 5(4), 58 CrossRef PubMed.
- J. Zhao, X. Zhao, Z. Jiang, Z. Li, X. Fan and J. Zhu,
et al., Biomimetic and bioinspired membranes: Preparation and application, Prog. Polym. Sci., 2014, 39(9), 1668–1720 CrossRef CAS.
- B. Mustafa, T. Mehmood, Z. Wang, A. G. Chofreh, A. Shen and B. Yang,
et al., Next-generation graphene oxide additives composite membranes for emerging organic micropollutants removal: Separation, adsorption and degradation, Chemosphere, 2022, 308, 136333 CrossRef CAS PubMed.
- Z. C. Ng, W. J. Lau, G. S. Lai, J. Meng, H. Gao and A. F. Ismail, Facile fabrication of polyethyleneimine interlayer-assisted graphene oxide incorporated reverse osmosis membranes for water desalination, Desalination, 2022, 526, 115502 CrossRef CAS.
- A. Inurria, P. Cay-Durgun, D. Rice, H. Zhang, D.-K. Seo and M. L. Lind,
et al., Polyamide thin-film nanocomposite membranes with graphene oxide nanosheets: Balancing membrane performance and fouling propensity, Desalination, 2019, 451, 139–147 CrossRef CAS.
- G. Lai, W. Lau, P. Goh, Y. Tan, B. Ng and A. Ismail, A novel interfacial polymerization approach towards synthesis of graphene oxide-incorporated thin film nanocomposite membrane with improved surface properties, Arabian J. Chem., 2019, 12(1), 75–87 CrossRef CAS.
- V. Vatanpour, S. S. Madaeni, R. Moradian, S. Zinadini and B. Astinchap, Novel antibifouling nanofiltration polyethersulfone membrane fabricated from embedding TiO2 coated multiwalled carbon nanotubes, Sep. Purif. Technol., 2012, 90, 69–82 CrossRef CAS.
- Z. W. Heng, W. C. Chong, Y. L. Pang and C. H. Koo, Self-assembling of NCQDs-TiO2 nanocomposite on poly (acrylic acid)-grafted polyethersulfone membrane for photocatalytic removal and membrane filtration, Mater. Today: Proc., 2021, 46, 1901–1907 CAS.
- D. Jirak, J. Svoboda, M. Filipová, O. Pop-Georgievski and O. Sedlacek, Antifouling fluoropolymer-coated nanomaterials for 19 F MRI, Chem. Commun., 2021, 57(38), 4718–4721 RSC.
- M. Mahmoudpour, A. Jouyban, J. Soleymani and M. Rahimi, Rational design of smart nano-platforms based on antifouling-nanomaterials toward multifunctional bioanalysis, Adv. Colloid Interface Sci., 2022, 302, 102637 CrossRef CAS PubMed.
- A. Zirehpour and A. Rahimpour, Membranes for wastewater treatment, Nanostruct. Polym. Membr., 2016, 2, 159–207 Search PubMed.
- N. K. Zaman, J. Y. Law, P. V. Chai, R. Rohani and A. W. Mohammad, Recovery of Organic Acids from Fermentation Broth Using Nanofiltration Technologies: A Review, J. Phys. Sci., 2017, 28, 85–109 CrossRef CAS.
- K. J. Howe and M. M. Clark, Fouling of microfiltration and ultrafiltration membranes by natural waters, Environ. Sci. Technol., 2002, 36(16), 3571–6 CrossRef CAS PubMed.
- S. Zhao, L. Zou, C. Y. Tang and D. Mulcahy, Recent developments in forward osmosis: Opportunities and challenges, J. Membr. Sci., 2012, 396, 1–21 CrossRef CAS.
-
M. Mulder, Basic Principles of Membrane Technology, Springer science & business media, 2012 Search PubMed.
- G. M. Von Medeazza, “Direct” and socially-induced environmental impacts of desalination, Desalination, 2005, 185(1–3), 57–70 CrossRef.
- R. A. Tufa, Perspectives on environmental ethics in sustainability of membrane based technologies for water and energy production, Environ. Technol. Innovation, 2015, 4, 182–193 CrossRef.
- M. Zubair, M. Yasir, D. Ponnamma, H. Mazhar, V. Sedlarik and A. H. Hawari,
et al., Recent advances in nanocellulose-based two-dimensional nanostructured membranes for sustainable water purification: A review, Carbohydr. Polym., 2024, 121775 CrossRef CAS PubMed.
- M. Issaoui, S. Jellali, A. A. Zorpas and P. Dutournie, Membrane technology for sustainable water resources management: Challenges and future projections, Sustainable Chem. Pharm., 2022, 25, 100590 CrossRef CAS.
- P. H. Wolf, S. Siverns and S. Monti, UF membranes for RO desalination pretreatment, Desalination, 2005, 182(1–3), 293–300 CrossRef CAS.
- W. L. Ang, A. W. Mohammad, N. Hilal and C. P. Leo, A review on the applicability of integrated/hybrid membrane
processes in water treatment and desalination plants, Desalination, 2015, 363, 2–18 CrossRef CAS.
- I. A. Shah, M. Bilal, I. Ihsanullah, S. Ali and M. Yaqub, Revolutionizing water purification: unleashing graphene oxide (GO) membranes, J. Environ. Chem. Eng., 2023, 111450 CrossRef CAS.
- A. Olabi, T. Wilberforce, E. T. Sayed, K. Elsaid, H. Rezk and M. A. Abdelkareem, Recent progress of graphene based nanomaterials in bioelectrochemical systems, Sci. Total Environ., 2020, 749, 141225 CrossRef CAS PubMed.
- E. T. Sayed, N. Shehata, M. A. Abdelkareem and M. A. Atieh, Recent progress in environmentally friendly bio-electrochemical devices for simultaneous water desalination and wastewater treatment, Sci. Total Environ., 2020, 748, 141046 CrossRef CAS PubMed.
- R. Einav and F. Lokiec, Environmental aspects of a desalination plant in Ashkelon, Desalination, 2003, 156(1–3), 79–85 CrossRef CAS.
- S. Lattemann and T. Höpner, Environmental impact and impact assessment of seawater desalination, Desalination, 2008, 220(1–3), 1–15 CrossRef CAS.
- S. Sarkar and A. K. SenGupta, A new hybrid ion exchange-nanofiltration (HIX-NF) separation process for energy-efficient desalination: process concept and laboratory evaluation, J. Membr. Sci., 2008, 324(1–2), 76–84 CrossRef CAS.
- Y. Ghalavand, M. S. Hatamipour and A. Rahimi, A review on energy consumption of desalination processes, Desalin. Water Treat., 2015, 54(6), 1526–1541 CrossRef CAS.
- L. Feng, Y. Gao, Y. Xu, H. Dan, Y. Qi and S. Wang,
et al., A dual-functional layer modified GO@ SiO2 membrane with excellent anti-fouling performance for continuous separation of oil-in-water emulsion, J. Hazard. Mater., 2021, 420, 126681 CrossRef CAS PubMed.
- S. Li, Y. Wan, S. Guo and J. Luo, Ferric ions mediated defects narrowing of graphene oxide nanofiltration membrane for robust removal of organic micropollutants, Chem. Eng. J., 2021, 411, 128587 CrossRef CAS.
- W. Liu, D. Wang, R. A. Soomro, F. Fu, N. Qiao and Y. Yu,
et al., Ceramic supported attapulgite-graphene oxide composite membrane for efficient removal of heavy metal contamination, J. Membr. Sci., 2019, 591, 117323 CrossRef CAS.
- S. Mahmood, N. S. Jiran, M. Z. M. Saman and M. Y. Noordin, Determination of Parameters for Sustainability Assessment of Hollow Fiber Membrane Module Life Cycle, Adv. Mater. Res., 2014, 845, 724–729 Search PubMed.
- A. I. Osman, M. Nasr, M. Farghali, S. S. Bakr, A. S. Eltaweil and A. K. Rashwan,
et al., Machine learning for membrane design in energy production, gas separation, and water treatment: a review, Environ. Chem. Lett., 2024, 22(2), 505–560 CrossRef CAS.
- S. Safeer, R. P. Pandey, B. Rehman, T. Safdar, I. Ahmad and S. W. Hasan,
et al., A review of artificial intelligence in water purification and wastewater treatment: Recent advancements, J. Water Process Eng., 2022, 49, 102974 CrossRef.
- M. Jafari, C. Tzirtzipi and B. Castro-Dominguez, Applications of artificial intelligence for membrane separation: A review, J. Water Process Eng., 2024, 68, 106532 CrossRef.
- M. T. Gaudio, G. Coppola, L. Zangari, S. Curcio, S. Greco and S. Chakraborty, Artificial intelligence-based optimization of industrial membrane processes, Earth Syst. Environ., 2021, 5(2), 385–398 CrossRef.
- M. Bagheri, A. Akbari and S. A. Mirbagheri, Advanced control of membrane fouling in filtration systems using artificial intelligence and machine learning techniques: A critical review, Process Saf. Environ. Prot., 2019, 123, 229–252 CrossRef CAS.
- M. Asghari, A. Dashti, M. Rezakazemi, E. Jokar and H. Halakoei, Application of neural networks in membrane separation, Rev. Chem. Eng., 2020, 36(2), 265–310 CrossRef.
- N. D. Viet, D. Jang, Y. Yoon and A. Jang, Enhancement of membrane system performance using artificial intelligence technologies for sustainable water and wastewater treatment: A critical review, Crit. Rev. Environ. Sci. Technol., 2022, 52(20), 3689–3719 CrossRef.
- C. Niu, X. Li, R. Dai and Z. Wang, Artificial intelligence-incorporated membrane fouling prediction for membrane-based processes in the past 20 years: A critical review, Water Res., 2022, 216, 118299 CrossRef CAS PubMed.
- C. M. Kim and M. Parnichkun, MLP, ANFIS, and GRNN based real-time coagulant dosage determination and accuracy comparison using full-scale data of a water treatment plant, J. Water Supply Res. Technol. AQUA, 2017, 66(1), 49–61 CrossRef.
- S. Yuan, H. Ajam, Z. A. B. Sinnah, F. M. Altalbawy, S. A. A. Ameer and A. Husain,
et al., The roles of artificial intelligence techniques for increasing the prediction performance of important parameters and their optimization in membrane processes: A systematic review, Ecotoxicol. Environ. Saf., 2023, 260, 115066 CrossRef CAS PubMed.
- H. Yin, M. Xu, Z. Luo, X. Bi, J. Li and S. Zhang,
et al., Machine learning for membrane design and discovery, Green Energy Environ., 2024, 9(1), 54–70 CrossRef CAS.
- A. Abushawish, I. Bouaziz, I. W. Almanassra, M. M. AL-Rajabi, L. Jaber and A. K. Khalil,
et al., Desalination pretreatment technologies: current status and future developments, Water, 2023, 15(8), 1572 CrossRef CAS.
- A. Karunakaran, A. A. Mungray, S. Agarwal and M. C. Garg, Optimization of the forward-osmosis performance with a low-concentration draw solution using response surface modeling, Chem. Eng. Technol., 2021, 44(7), 1278–1286 CrossRef CAS.
- H. Jain, R. Dhupper, A. K. Verma and M. C. Garg, Development of titanium dioxide incorporated ultrathin cellulose acetate membrane for enhanced forward osmosis performance, Nanotechnol. Environ. Eng., 2021, 6, 1–8 CrossRef.
- G. Arthanareeswaran and P. Thanikaivelan, Fabrication of cellulose acetate–zirconia hybrid membranes for ultrafiltration applications: Performance, structure and fouling analysis, Sep. Purif. Technol., 2010, 74(2), 230–235 CrossRef CAS.
- L. Valencia and H. N. Abdelhamid, Nanocellulose leaf-like zeolitic imidazolate framework (ZIF-L) foams for selective capture of carbon dioxide, Carbohydr. Polym., 2019, 213, 338–345 CrossRef CAS PubMed.
- A. Karunakaran, A. A. Mungray and M. C. Garg, Effects of temperature, pH, feed, and fertilizer draw solution concentrations on the performance of forward osmosis process for textile wastewater treatment, Water Environ. Res., 2021, 93(10), 2329–2340 CrossRef CAS PubMed.
- Z. Naghdali, S. Sahebi, M. Mousazadeh and H. A. Jamali, Optimization of the forward osmosis process using aquaporin membranes in chromium removal, Chem. Eng. Technol., 2020, 43(2), 298–306 CrossRef CAS.
- F. Zaviska and L. Zou, Using modelling approach to validate a bench scale forward osmosis pre-treatment process for desalination, Desalination, 2014, 350, 1–13 CrossRef CAS.
- M. Khayet, J. Sanmartino, M. Essalhi, M. García-Payo and N. Hilal, Modeling and optimization of a solar forward osmosis pilot plant by response surface methodology, Sol. Energy, 2016, 137, 290–302 CrossRef.
- S. P. Nunes, P. Z. Culfaz-Emecen, G. Z. Ramon, T. Visser, G. H. Koops and W. Jin,
et al., Thinking the future of membranes: Perspectives for advanced and new membrane materials and manufacturing processes, J. Membr. Sci., 2020, 598, 117761 CrossRef CAS.
- B. Sutisna, V. Musteata, B. Pulido, T. Puspasari, D.-M. Smilgies and N. Hadjichristidis,
et al., High flux membranes, based on self-assembled and H-bond linked triblock copolymer nanospheres, J. Membr. Sci., 2019, 585, 10–18 CrossRef CAS.
- P. Tyagi, A. Deratani, D. Bouyer, D. Cot, V. Gence and M. Barboiu,
et al., Dynamic interactive membranes with pressure-driven tunable porosity and self-healing ability, Angew. Chem., 2012, 124(29), 7278 CrossRef.
- J. E. Gu, S. Lee, C. M. Stafford, J. S. Lee, W. Choi and B. Y. Kim,
et al., Molecular layer-by-layer assembled thin-film composite membranes for water desalination, Adv. Mater., 2013, 25(34), 4778–4782 CrossRef CAS PubMed.
|
This journal is © The Royal Society of Chemistry 2025 |
Click here to see how this site uses Cookies. View our privacy policy here.