DOI:
10.1039/C6QM00149A
(Research Article)
Mater. Chem. Front., 2017,
1, 893-899
Metal-deposited bismuth oxyiodide nanonetworks with tunable enzyme-like activity: sensing of mercury and lead ions†
Received
29th July 2016
, Accepted 13th November 2016
First published on 7th December 2016
Abstract
In this study, we demonstrate that the enzyme-like activity of bismuth oxyiodide (BiOI) nanonetworks can be regulated through homogeneous deposition of metal atoms/ions or nanoparticles. Bismuth oxyhalide (BiOX; X = Cl, Br or I) nanostructures were prepared from a simple mixture of bismuth ions (Bi3+) and halide ions (X−) in aqueous solution. The BiOI nanonetworks exhibited much stronger (>25-fold) peroxidase-like activity than BiOCl or BiOBr nanosheets. In situ formation and deposition of gold nanoparticles (Au NPs) onto BiOI nanonetworks greatly enhanced the oxidase-like activity of the nanocomposites. The deposition of Ni, Zn or Mn on the BiOI nanonetworks boosted their peroxidase-like activity by at least 3-fold. Moreover, the catalase-like activity of the BiOI nanonetworks was elevated after deposition of MnO2 or ZnO nanoparticles. The enzyme-like activity of BiOI regulated by the deposition of metals was mainly due to the changes in the electronic and band structures of the BiOX nanonetworks, and the existence of surface metal atoms/ions in various oxidation states. We used the Au NPs/BiOI nanocomposites and NiO NPs/BiOI nanocomposites for the detection of Hg2+ and Pb2+ heavy metal ions, respectively, based on the suppression of the enzyme-like activity of the nanocomposite after deposition of these metal ions. These BiOI nanocomposite-based probes allow the selective detection of Hg2+ and Pb2+ down to nanomolar quantities. The practicality of these two nanozyme probes was validated by analysis of Hg2+ and Pb2+ ions in environmental water samples (tap water, river water, lake water, and sea water).
Introduction
Bismuth oxyhalides (BiOX, X = Cl, Br, and I) with unique structural, electrical and optical properties, as well as excellent photocatalytic activities, have become attractive nanomaterials.1 The reactions between bismuth and halides (X−) in the presence of a stabilizer (e.g., surfactant, polymer) through hydrothermal, microwave or solvothermal processes can result in the formation of stable BiOX nano- and microstructures, including particles, rods/wires, and plates/sheets in the micro-/nano-range, and hierarchical architectures.2 BiOX materials are mostly crystallized as tetragonal matlockites, which are characterized by their layered composition of [Bi2O2] slabs interleaved with double halogen atom slabs along the [001] direction.1 Many BiOX nano- and micro-structures have been used in photocatalytic applications, but most of them can only work in the UV region.3 To improve their photocatalytic efficiency and separate the photogenerated electron–hole pairs in the visible light regime, different compositions, facets, morphologies, metal doping, heterojunction4 and hybridized BiOX materials have been synthesized, especially for photocatalytic applications such as the degradation of dyes,4,5 disinfection of pathogens,6 photofuel cells,7 and H2 evolution.8 However, the enzyme-like activity of BiOX materials has rarely been studied.9
Recently, we have demonstrated that bismuth oxychloride (BiOCl) nanosheets immobilized with aptamer-modified gold nanoparticles (Apt–Au NPs) exhibit intrinsic peroxidase (POX)-like activity.9 These Apt–Au NPs/BiOCl nanocomposites can be used for the detection of vascular endothelial growth factor-A165 (VEGF-A165), which specifically interacts with the aptamer units on the nanocomposite surface and thereby suppress the nanocomposites' catalytic activity. In the present study, we systematically examined the enzyme-like activity [cf. peroxidase (POX), oxidase (OX), and catalase (CAT)] of BiOX (X = Cl, Br, and I) nanostructures. Furthermore, we demonstrated that the enzyme-like activity of BiOI network-like nanostructures (nanonetworks) can be modulated by hybridization with heavy metals (e.g., Au, Pt, Mn, Ni, Zn). For example, the enzyme-like activity of BiOI nanonetworks was switched from POX to OX by in situ formation and deposition of gold nanoparticles (Au NPs), and the POX-like activity was boosted by up to 4-fold by deposition of NiO NPs. Furthermore, we utilized the Au NP- and NiO NP-deposited BiOI nanocomposites for the selective detection of Hg2+ and Pb2+ ions, respectively, based on the fact that Hg and Pb ions tend to deposit onto the surfaces of nanocomposites as a result of strong metallophilic and Hg–I/Pb–I interactions, subsequently inducing inhibition of the nanocomposite's enzymatic activity (Scheme 1).
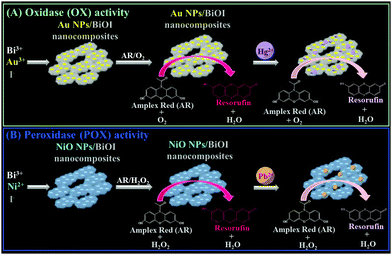 |
| Scheme 1 Schematic representation of the preparation of (A) Au-deposited BiOI nanonetworks and (B) Ni-deposited BiOI nanonetworks that exhibit (A) oxidase-like and (B) peroxidase-like activities, and their application for the determination of (A) Hg2+ and (B) Pb2+ ions based on inhibition of enzymatic activities. | |
Results and discussion
Synthesis and characterization of BiOX nanostructures
BiOX nanostructures were synthesized from Bi3+ ions and halide ions (X−) in aqueous solution (Bi3+ + X− + H2O → BiOX(s) + 2H+) at room temperature.10 Transmission electron microscopy (TEM; Fig. 1A), atomic force microscopy (AFM; Fig. 1B) and scanning electron microscopy (SEM; Fig. S1, ESI†) images reveal that BiOCl and BiOBr both have nanosheet-like morphologies, each with an average size of ∼100 nm and a thickness of around 15 nm. Surprisingly, BiOI has a nanonetwork-like structure that is formed through the connection of high-density small nanoplates (∼20 nm) with thickness around ∼5 nm. The slow growth rate of BiOI and the hydrolysis of iodide result in the formation of BiOI having a nanonetwork structure.11 The smaller size and thin sheet structure of BiOI increases the surface area ratio and reaction area. The yields of these three nanostructures were all almost the same (∼60%). The X-ray diffraction (XRD) patterns shown in Fig. S2 (ESI†) reveal that the BiOX nanostructures have typical tetragonal crystal structures and are predominantly exposed along the {001} facet.12 Quantitative analysis using X-ray photoelectron spectroscopy (XPS; Fig. S3, ESI†), inductively coupled plasma optical emission spectrometry (ICP-OES) or inductively coupled plasma mass spectrometry (ICP-MS) (Table S1, ESI†) reveals that the ratios of Bi to X (Cl, Br or I) in the BiOX nanostructures are all higher than 1. This finding is consistent with the results of XRD (Fig. S2, ESI†), which shows that Bi2O3 is present in the BiOX nanostructures.
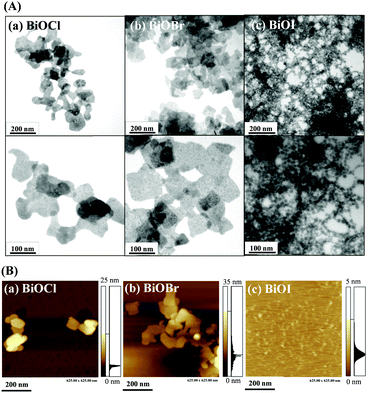 |
| Fig. 1 (A) TEM and (B) AFM images of as-prepared (a) BiOCl nanosheets, (b) BiOBr nanosheets, and (c) BiOI nanonetworks. The TEM images in the upper panels and lower panels are low and high magnified views, respectively. | |
Enzyme-like activity of the BiOX nanostructures
Among the as-prepared BiOX nanostructures, the BiOI nanonetworks possess the greatest POX-like activity (>25-fold higher than that of the BiOCl and BiOBr nanosheets) for the H2O2-mediated oxidation of Amplex Red (AR) to resorufin (7-hydroxy-3H-phenoxazin-3-one; curve d in Fig. 2). The BiOX nanostructures likely catalyzed a one-electron oxidation of nonfluorescent AR to form a nonfluorescent AR radical in the presence of H2O2, which acts as an electron acceptor.13 The AR radicals subsequently underwent enzyme-independent dismutation to yield highly colored and fluorescent resorufin (absorption coefficient: 5.4 × 104 cm−1 M−1 at 570 nm; quantum yield: 0.83).13 In the control experiments, we used Bi3+ or halide ions to catalyze the H2O2-mediated AR reaction, which showed negligible catalytic activity (data not shown). In the kinetic catalysis study (Table 1), we demonstrated that BiOI nanonetworks exhibited a much higher catalytic efficiency (Kcat/KM; KM and Kcat are the Michaelis constant and turnover number, respectively) than that of BiOBr and BiOCl. The higher POX-like activity of the BiOI nanonetworks presumably reflects their smaller band gap (1.77 eV),14 higher number of active sites, and greater surface energy in the junctions of the heterogeneous crystal faces (Fig. 1). Heterogeneous crystal structures disturb the static internal electric field in the layered structure of the BiOI nanonetworks, thereby facilitating the formation of ˙OH radicals from H2O2.15 These ˙OH radicals can further oxidize the AR substrate to form fluorescent resorufin. Moreover, I− ions on the surface of the BiOI nanonetworks may be catalytically converted to iodine oxide (IO) radicals by H2O2,15 which facilitates the catalysis of AR to resorufin.
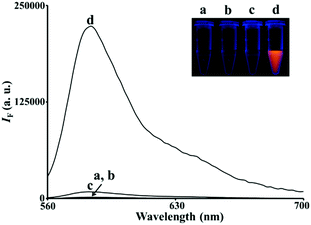 |
| Fig. 2 Fluorescence spectra of AR (5.0 μM) reacted with H2O2 (100 μM) in 5 mM Tris–borate solution (pH 7.0) in the (a) absence and (b–d) presence of (b) BiOCl nanosheets (20 μg mL−1), (c) BiOBr nanosheets (20 μg mL−1), and (d) BiOI nanonetworks (20 μg mL−1). The excitation wavelength was set at 540 nm. Inset: Photograph of the corresponding solutions upon excitation under a hand-held UV lamp (365 nm). The fluorescence intensity (IF) is plotted in arbitrary units (a.u.). | |
Table 1 Comparison of the apparent Michaelis constants (KM), maximal velocities (vmax), turnover numbers (Kcat), and catalytic efficiencies (Kcat/KM) of BiOX nanostructures
Catalysta |
Substrate |
K
M (μM) |
v
max (μM S−1) |
K
cat
(μM S−1 mL μg−1) |
K
cat/KM (S−1 mL μg−1) |
Kinetic catalysis parameters were determined according to the Lineweaver–Burk plot using the Michaelis–Menten kinetic equation [1/v = KM/vmax·(1/[S] + 1/KM)], where v is the reaction velocity, [S] is the substrate concentration, KM is the Michaelis constant, and vmax is the maximal velocity.
K
cat = vmax/[E]total, where [E]total is the concentration (μg mL−1) of BiOX nanostructures.
|
BiOCl |
AR |
5.88 |
6.66 × 10−6 |
3.33 × 10−7 |
5.66 × 10−8 |
BiOBr |
AR |
6.23 |
8.45 × 10−6 |
4.23 × 10−7 |
6.79 × 10−8 |
BiOI |
AR |
31.3 |
6.10 × 10−2 |
3.05 × 10−3 |
9.74 × 10−5 |
BiOCl |
H2O2 |
0.583 |
2.46 × 10−6 |
1.23 × 10−7 |
2.11 × 10−7 |
BiOBr |
H2O2 |
0.408 |
2.49 × 10−6 |
1.25 × 10−7 |
3.06 × 10−7 |
BiOI |
H2O2 |
32.9 |
2.76 × 10−2 |
1.38 × 10−3 |
4.19 × 10−5 |
Enzyme-like activity of the metal/BiOI nanocomposites
We further prepared a series of metal/BiOI nanocomposites (see details of their synthesis in the Experimental section) to evaluate their POX-, OX-, and CAT-like activities (Scheme S1, ESI†) towards solutions of AR and H2O2, AR and O2, and H2O2, respectively. The hybrid metal/BiOI nanocomposites were probably formed through co-hydrolysis (Mn+ + Bi3+ + I− + 2H2O → MOBiOI(s) + 4H+), ion exchange-coprecipitation (nBiOI + M → MIn + BiO+; MIn + BiOI → MIn–BiOI(s)), or in situ deposition of reduced metallic nanoparticles onto the BiOI nanonetworks.16 The electronic and band structures of BiOX can be tuned by hybridization of metals or metal oxides, leading to various activities of the as-formed hybrid metal/BiOI nanocomposites.16 In addition, metal/BiOX or metal oxide/BiOX nanonetworks possess defective structures between different crystal planes and vacancies (e.g., oxygen vacancy, metal atom vacancy), resulting in the variation of the electronic structure, charge transport, and surface properties, and thereby varying their activities.17Fig. 3A reveals that the OX-like activity of both the Au NPs/BiOI and Bi2Pt/BiOI nanocomposites is >10-fold higher than that of the BiOI nanonetworks. Au NPs/BiOI and Bi2Pt/BiOI nanocomposites have metallic nanoparticles embedded in the BiOI nanonetworks (Fig. 4 and 5), wherein the heterojunction increases the OX-like activity. Scanning transmission electron microscopy/energy dispersive X-ray (STEM-EDS) mapping shows that the Au NPs (diameter ∼ 3 nm) or Bi2Pt NPs (diameter ∼ 4 nm) and Bi, O, and I coexist and are well-dispersed in the nanonetwork structures. The POX-like activity of the MnO2/BiOI, NiO/BiOI, and ZnO/BiOI nanocomposites is, respectively, 3.2-fold, 4.3-fold and 5.1-fold higher than that of the BiOI nanonetworks in the H2O2-mediated oxidation of the AR reaction (Fig. 3B). On the other hand, the CAT-like catalytic enhancement of the metal–BiOI nanonetworks induced by Mn or Ni was greatly enhanced relative to the other tested metal ions (Fig. 3C). The NiO/BiOI, ZnO/BiOI and MnO2/BiOI nanocomposites show very similar sizes and morphologies to the BiOI nanonetworks (Fig. S4–S6A, ESI†). Fig. S4–S6B (ESI†) show that the d-spacing values of BiOI (110), Bi2O3 (111), NiO (200), ZnO (102), and MnO2 (400) are 0.27, 0.32, 0.21, 0.19, and 0.24 nm, respectively. High-resolution TEM (HRTEM) images reveal that the sizes of the NiO NPs, ZnO NPs and MnO2 NPs are all smaller than 3 nm (Fig. S4–S6B, ESI†). STEM-EDS analysis indicates that the metal (Ni, Zn or Mn) and Bi, O, and I all coexist well in the nanonetworks (Fig. S4–S6C, ESI†).
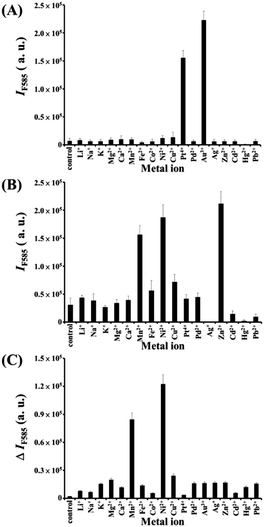 |
| Fig. 3 (A) OX-like activity assays; fluorescence intensity at 585 nm (IF585) of AR (5.0 μM) after reaction with dissolved oxygen in the presence of metal–BiOI nanocomposites (2.0 μg mL−1) for 1 h. (B) POX-like activity assays; IF585 of AR (5.0 μM) after reaction with H2O2 (100 μM) in the presence of metal–BiOI nanocomposites (2.0 μg mL−1) for 2 h. (C) CAT-like activity assays; fluorescence intensity difference at 585 nm (ΔIF585 = IF0 − IF) of AR (5.0 μM) after reaction with the remaining H2O2 in the presence of horseradish peroxidase (HRP) (1.32 U mL−1) for 1 h in solutions containing H2O2 that had been reacted in the absence/presence of metal–BiOI nanocomposites for 1 h. IF0 and IF in (C) represent the fluorescence intensities of the solutions at 585 nm in the absence and presence of metal–BiOI nanocomposites, respectively. Tris–borate solution (5.0 mM, pH 7.0) was used in this study. Error bars represent standard deviations from three repeated experiments. Other conditions were the same as those described in Fig. 2. | |
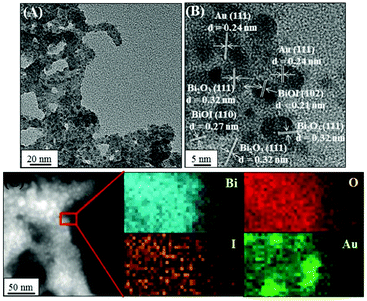 |
| Fig. 4 (A) TEM image, (B) HRTEM image, and (C) high-angle annular dark-field scanning TEM mapping images of Au NPs/BiOI nanocomposites. | |
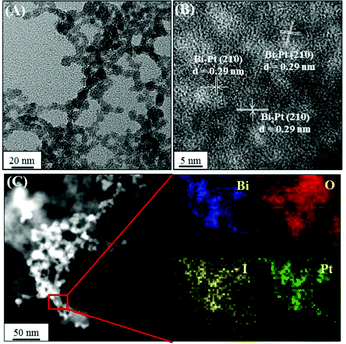 |
| Fig. 5 (A) TEM image, (B) HRTEM image, and (C) high-angle annular dark-field scanning TEM mapping images of Bi2Pt NPs/BiOI nanocomposites. | |
Nanozyme-based probes for sensing Hg2+ and Pb2+
We further utilized the as-prepared M/BiOI nanocomposites in the sensing of metal ions. By taking advantage of the intrinsic enzyme-like activities (POX, OX or CAT) of the M/BiOI nanonetworks (M = Au, Bi2Pt, ZnO, NiO or MnO2), a series of experiments were conducted for sensing metal ions using the as-prepared catalytic M/BiOI nanocomposites. Fig. 6a shows that the Au NPs/BiOI nanonetworks can be utilized for the selective detection of Hg2+ down to the nanomolar regime based on the Hg2+ induced suppression of the OX-like activity of the Au NPs/BiOI nanocomposites. Generally, the d10–d10 interactions between Au+ ions and closed-shell atoms/ions, such as Ag, Pt, and Cu, are weak (ca. 25 kJ mol−1).18 On the other hand, the two complementary forces (charge-induced dipole and dispersion interactions) involved in the aurophilic interaction between Au and Hg result in strong (>150 kJ mol−1) closed-shell d10–s2 interactions.19 As a result, the Au NPs/BiOI nanonetworks exhibit superior selectivity towards Hg2+ ions relative to other metal ions. However, inhibition of the OX-like activity of the Au NPs/BiOI nanocomposites by Hg2+ through the formation of stable HgI2 (Hg2+ + 2I− → HgI2(s)K ∼ 3 × 1028 M−2) on the nanocomposites' surfaces cannot be excluded.20 Furthermore, NiO NPs/BiOI nanocomposites coupled with H2O2/AR can be used as a probe for the selective detection of Pb2+ down to the nanomolar regime based on its induced inhibition of their POX-like activity (Fig. 6b). The catalytic activity of the NiO NPs/BiOI nanonetworks declined in the presence of Pb2+, probably due to the reaction of Pb2+ with surface I− to form PbIn(n−2)− (K > 1012),21 which then blocked the active sites on the surfaces of the nanonetworks.
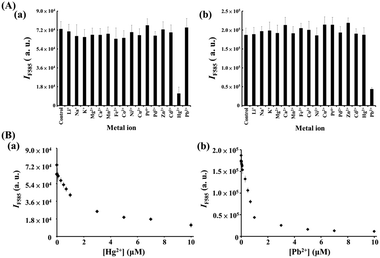 |
| Fig. 6 (A) Selectivity of the (a) O2/AR–Au NPs/BiOI nanozyme probe and (b) H2O2/AR–NiO NPs/BiOI nanozyme probe towards (a) Hg2+ and (b) Pb2+ ions. The concentrations of both tested metal ions were 10 μM. (B) Fluorescence response (IF585) of the (a) O2/AR–Au NPs/BiOI probe and (b) H2O2/AR–NiO NPs/BiOI probe to various concentrations of (a) Hg2+ and (b) Pb2+ ions. Error bars are the standard deviations of three repeated experiments. Other conditions were the same as those described in Fig. 3. | |
Detection of Hg2+ and Pb2+ in real samples
To validate the practicality of the proposed sensing strategy for environmental sample analysis, O2/AR–Au NPs/BiOI and H2O2/AR–NiO NPs/BiOI nanozyme sensing systems were utilized to determine the concentrations of spiked Hg2+ and Pb2+ (0–500 nM), respectively, in tap-, river-, lake-, and sea-water samples. As shown in Table S2 (ESI†), our probes show good accuracy (relative errors <10%) and recovery (94–112%) for the detection of Hg2+- and Pb2+-spiked real water samples. Neither the probes nor the ICP-MS system detected the presence of Pb2+ and Hg2+ in the four unadulterated water samples (without spiking), which were used as the controls. Thus, the proposed methods are applicable for practical analysis of Pb2+ and Hg2+ in environmental water samples.
Conclusions
In summary, we have demonstrated that BiOX nanostructures are a promising platform for the development of diverse nanozymes mimicking the activity of natural enzymes. The BiOI nanonetworks exhibited superior POX-like activities relative to BiOCl or BiOBr nanosheets. Compared to other nanocomposites having peroxidase-like activities towards H2O2-mediated reactions, the preparation of our metal/BiOI nanocomposites is relatively straightforward.22–24 Simple in situ deposition of metal or metal oxide NPs results in the tunable and switchable enzyme-like activities of the BiOI nanonetworks. We have also demonstrated O2/AR–Au NPs/BiOI and H2O2/AR–NiO NPs/BiOI probes as selective sensing systems for Pb2+ and Hg2+, respectively, in environmental water samples. The metal/BiOI and metal oxide/BiOI nanocomposites have great potential to be further applied to photocatalytic reactions due to their unique structure, and surface and electronic characteristics.
Experimental section
Materials
Boric acid, potassium bromide, potassium chloride, potassium iodide, bismuth nitrate pentahydrate, tris(hydroxymethyl)aminomethane (Tris), and the other metal salts used were purchased from Mallinckrodt Baker (Phillipsburg, NJ, USA). Hydrogen peroxide was purchased from SHOWA (Tokyo, Japan). Amplex Red (AR, 10-acetyl-3,7-dihydroxyphenoxazine) was purchased from Invitrogen (Carlsbad, CA, USA). Hydrogen tetrachloroaurate(III) trihydrate (HAuCl4·3H2O) was purchased from Acros (Geel, Belgium). Milli-Q ultrapure water (Millipore, Billerica, MA, USA) was used throughout the experiments. The buffer used in this study was a solution of Tris–borate (50 mM, pH 7.0) adjusted with boric acid.
Preparation of BiOI nanostructures and metal/BiOI nanocomposites
For the preparation of BiOX nanostructures, 50 mM Tris–borate (pH 7.0, 1 mL), Bi(NO3)2 (10 mM, 50 μL), and potassium halides (10 mM, 50 μL) were sequentially added to DI water (3.9 mL) under continuous stirring, and subsequently reacted at ambient temperature for 2 h in the dark. For the preparation of metal–BiOI nanocomposites, 50 mM Tris–borate (pH 7.0, 1 mL), Bi(NO3)2 (10 mM, 50 μL), metal salt solution (10 mM, 50 μL), and KI (10 mM, 50 μL) were sequentially added to DI water (3.85 mL) under continuous stirring, and subsequently reacted at ambient temperature for 2 h in the dark.
Characterization of BiOX nanostructures
Transmission electron microscopy (TEM) images of the BiOX nanonetworks were obtained using a Tecnai 20 G2 S-twin transmission electron microscope (Philips/FEI, Hillsboro, OR, USA). Fluorescence spectra for the monitoring of enzymatic reactions were recorded using a monochromatic microplate spectrophotometer (Synergy 4 Multi-Mode; Biotek Instruments, Winooski, VT, USA). Samples for X-ray diffraction (XRD) were prepared by depositing the BiOX nanostructure solution on glass slides. XRD measurements were carried out at room temperature using a Rigaku (Tokyo, Japan) 18 kW rotating anode source X-ray diffractometer (operated at 50 kV and 100 mA) with a Cu Ka1 line (λ = 1.54 Å). Samples for scanning electron microscopy (SEM) were prepared by depositing the BiOX nanostructure solution on a Si(100) wafer prior to measurement with a field emission SEM (JSM-6500F, JEOL, Ltd, Tokyo, Japan). For determination of the concentrations of metal ions using ICP-MS (Agilent 7700 Series, Agilent Technologies, Santa Clara, CA, USA), the BiOX nanostructure samples were prepared in 2% HNO3. X-ray photoelectron spectroscopy (XPS) was performed using a VG ESCA Scientific theta probe spectrometer (Uppsala, Sweden) in a constant-analyzer energy mode (pass energy: 28 eV) with Al Ka (1486.6 eV) radiation as the excitation source.
Enzyme kinetic analysis
Kinetic measurements were conducted in a black 96-well microplate using a microplate reader (Synergy 4). The AR/H2O2 substrate solution, in 180 μL of Tris–borate solution (pH 7.0), was added to each well of a 96-well microtiter plate, and aliquots (20 μL) of enzyme-like BiOX nanostructure solutions were then added to the plate. The reaction progress was monitored every 30 s for 2 h by recording the fluorescence of the reaction product, resorufin, at 585 nm with an excitation wavelength of 540 nm. Variable concentrations (1–100 μM) of AR with a constant H2O2 concentration (100 μM) were investigated in the catalytic reactions. In addition, variable H2O2 concentrations (1–100 μM) with a constant AR concentration (5 μM) were investigated.
Au NPs/BiOI nanozyme probe for Hg2+ sensing
A series of mixtures (400 μL) containing Hg2+ ions (0–12.5 μM) and Au NPs/BiOI nanocomposites (2.5 μg mL−1) in Tris–borate buffer solution (5.0 mM, pH 7.0) were equilibrated at room temperature for 30 min. Then, Tris–borate solution (100 μL, 5.0 mM, pH 7.0) containing AR (25 μM) was added to each of the mixtures and left for 1 h prior to fluorescence measurements using a Synergy 4 fluorescence microplate reader, with excitation at a wavelength of 540 nm.
NiO NPs/BiOI nanocomposite probe for Pb2+ sensing
A series of aliquots (400 μL) of Tris–borate solutions (5.0 mM, pH 7.0) containing NiO NPs/BiOI nanocomposites (2.5 μg mL−1) and Pb2+ ions (0–12.5 μM) were equilibrated at ambient temperature for 30 min. Then, Tris–borate solution (100 μL, 5.0 mM, pH 7.0) containing AR (25 μM) and H2O2 (500 μM) was added to each of the mixtures and left for 1 h prior to fluorescence measurements with excitation at 540 nm (Synergy 4 spectrophotometer).
Analysis of Hg2+ and Pb2+ ions in real samples
10-fold diluted real samples [including tap water and lake water from the National Taiwan University campus and river water on, and coastal sea water near, the campus of National Taiwan Ocean University] in Tris–borate (pH 7.0, 5 mM) solutions were spiked with Hg2+ (0–500 nM) or Pb2+ ions (0–500 nM), and were then analyzed using the described probe following processes similar to those used for the standard solution.
Acknowledgements
This study was supported by the Ministry of Science and Technology of Taiwan under contracts 104-2113-M-002-008-MY3, 104-2628-M-019-001-MY3, 103-2314-B-182-013, and 103-2627-M-007-002-MY3. We thank Ms Ya-Yun Yang and Ms Ching-Yen Lin from the Instrument Center of National Taiwan University (NTU) for assistance with the TEM and SEM measurements.
References
-
(a) J. Li, Y. Yu and L. Zhang, Nanoscale, 2014, 6, 8473–8488 RSC
;
(b) H. Cheng, B. Huang and Y. Dai, Nanoscale, 2014, 6, 2009–2026 RSC
.
-
(a) D. S. Bhachu, S. J. A. Moniz, S. Sathasivam, D. O. Scanlon, A. Walsh, S. M. Bawaked, M. Mokhtar, A. Y. Obaid, I. P. Parkin, J. Tang and C. J. Carmalt, Chem. Sci., 2016, 7, 4832–4841 RSC
;
(b) A. M. Ganose, M. Cuff, K. T. Butler, A. Walsh and D. O. Scanlon, Chem. Mater., 2016, 28, 1980–1984 CrossRef CAS PubMed
;
(c) S.-L. Wang, L.-L. Wang, W.-H. Ma, D. M. Johnson, Y.-F. Fang, M.-K. Jia and Y.-P. Huang, Chem. Eng. J., 2015, 259, 410–416 CrossRef CAS
;
(d) I. D. Sharma, G. K. Tripathi, V. K. Shamra, S. N. Tripathi, R. Kurchania, C. Kant, A. K. Sharma and K. K. Saini, Cogent Chem., 2015, 1, 1076371 Search PubMed
.
-
(a) H. Z. An, Y. Du, T. M. Wang, C. Wang, W. C. Hao and J. Y. Zhang, Rare Met., 2008, 27, 243–250 CrossRef CAS
;
(b) L. Q. Ye, Y. R. Su, X. L. Jin, H. Q. Xie and C. Zhang, Environ. Sci.: Nano, 2014, 1, 90–112 RSC
.
-
(a) Y.-R. Jiang, H.-P. Lin, W.-H. Chung, Y.-M. Dai, W.-Y. Lin and C.-C. Chen, J. Hazard. Mater., 2015, 283, 787–805 CrossRef CAS PubMed
;
(b) H.-P. Lin, W. W. Lee, S.-T. Huang, L.-W. Chen, T.-W. Yeh, J.-Y. Fu and C.-C. Chen, J. Mol. Catal. A: Chem., 2016, 417, 168–183 CrossRef CAS
;
(c) W. W. Lee, C.-S. Lu, C.-W. Chuang, Y.-J. Chen, J.-Y. Fu, C.-W. Siao and C.-C. Chen, RSC Adv., 2015, 5, 23450–23463 RSC
;
(d) S.-T. Huang, Y.-R. Jiang, S.-Y. Chou, Y.-M. Dai and C.-C. Chen, J. Mol. Catal. A: Chem., 2014, 391, 105–120 CrossRef CAS
;
(e) S.-Y. Chou, C.-C. Chen, Y.-M. Dai, J.-H. Lin and W. W. Lee, RSC Adv., 2016, 6, 33478–33491 RSC
;
(f) C. L. Yu, J. C. Chen, W. Q. Zhou, L. F. Wei and Q. Z. Fan, Mater. Res. Innovations, 2015, 19, 54–59 CrossRef CAS
.
-
(a) C.-T. Yang, W. W. Lee, H.-P. Lin, Y.-M. Dai, H.-T. Chi and C.-C. Chen, RSC Adv., 2016, 6, 40664–40675 RSC
;
(b) S.-Y. Chou, W.-H. Chung, L.-W. Chen, Y.-M. Dai, W.-Y. Lin, J.-H. Lin and C.-C. Chen, RSC Adv., 2016, 6, 82743–82758 RSC
;
(c) J. Di, J. X. Xia, M. X. Ji, L. Xu, S. Yin, Z. G. Chen and H. M. Li, J. Mater. Chem. A, 2016, 4, 5051–5061 RSC
;
(d) K. Natarajan, H. C. Bajaj and R. J. Tayade, J. Ind. Eng. Chem., 2016, 34, 146–156 CrossRef CAS
;
(e) F. Chang, Y. C. Xie, J. Zhang, J. Chen, C. L. Li, J. Wang, J. R. Luo, B. Q. Deng and X. F. Hu, RSC Adv., 2014, 4, 28519–28528 RSC
;
(f) R. A. He, S. W. Cao, J. G. Yu and Y. C. Yang, Catal. Today, 2016, 264, 221–228 CrossRef CAS
;
(g) Y.-R. Jiang, S.-Y. Chou, J.-L. Chang, S.-T. Huang, H.-P. Lin and C.-C. Chen, RSC Adv., 2015, 5, 30851–30860 RSC
;
(h) X. Xiao, R. Hao, M. Liang, X. X. Zuo, J. M. Nan, L. S. Li and W. D. Zhang, J. Hazard. Mater., 2012, 233, 122–130 CrossRef PubMed
;
(i) H.-P. Lin, C.-C. Chen, W. W. Lee, Y.-Y. Lai, J.-Y. Chen, Y.-Q. Chen and J.-Y. Fu, RSC Adv., 2016, 6, 2323–2336 RSC
;
(j) C. L. Yu, C. F. Fan, J. C. Yu, W. Q. Zhou and K. Yang, Mater. Res. Bull., 2011, 46, 140–146 CrossRef CAS
;
(k) W. Fang, C. L. Yu, J. D. Li, W. Q. Zhou and L. H. Zhu, J. Mater. Res., 2015, 30, 3125–3133 CrossRef CAS
.
-
(a) T. S. Jamil, E. S. Mansor and M. A. El-Liethy, J. Environ. Chem. Eng., 2015, 3, 2463–2471 CrossRef CAS
;
(b) Z. Fang, J. Yang, Y. Cao, L. Zhu, Q. Zhang, D. Shu and C. He, Procedia Environ. Sci., 2013, 18, 503–508 CrossRef CAS
.
-
(a) Y. Fujishima, S. Okamoto, M. Yoshiba, T. Itoi, S. Kawamura, Y. Yoshida, Y. Oqura and Y. Izumi, J. Mater. Chem. A, 2015, 3, 8389–8404 RSC
;
(b) K. Li, Y. L. Xu, Y. He, C. Yang, Y. L. Wang and J. P. Jia, Environ. Sci. Technol., 2013, 47, 3490–3497 CAS
.
- Y. Bai, T. Chen, P. Q. Wang, L. Wang and L. Q. Ye, Chem. Eng. J., 2016, 304, 454–460 CrossRef CAS
.
- C.-L. Hsu, C.-W. Lien, C.-W. Wang, S. G. Harroum, C.-C. Huang and H.-T. Chang, Biosens. Bioelectron., 2016, 75, 181–187 CrossRef CAS PubMed
.
-
(a) L. Chen, R. Huang, M. Xiong, Q. Yuan, J. He, J. Jia, M.-Y. Yao, S.-L. Luo, C.-T. Au and S.-F. Yin, Inorg. Chem., 2013, 52, 11118–11125 CrossRef CAS PubMed
;
(b) L. Chen, S.-F. Yin, R. Huang, Y. Zhou, S.-L. Luo and C.-T. Au, Catal. Commun., 2012, 23, 54–57 CrossRef CAS
;
(c) M. Wang, J. T. Zai, X. Wei, W. L. Chen, N. Liang, M. Xu, R. R. Qi and X. F. Qian, CrystEngComm, 2015, 17, 4019–4025 RSC
.
-
(a) C. F. Guo, J. M. Zhang, Y. Tian and Q. Liu, ACS Nano, 2012, 6, 8746–8752 CrossRef CAS PubMed
;
(b) J. M. Gong, X. Q. Wang, X. Li and K. W. Wang, Biosens. Bioelectron., 2012, 38, 43–49 CrossRef CAS PubMed
;
(c) M. E. Kazyrevich, M. V. Malashchonak, A. V. Mazanik, E. A. Streltsov, A. L. Kulak and C. Bhattacharya, Electrochim. Acta, 2016, 190, 612–619 CrossRef CAS
.
-
(a) D. Zhang, J. Li, Q. G. Wang and Q. S. Wu, J. Mater. Chem. A, 2013, 1, 8622–8629 RSC
;
(b) F. Duan, X. F. Wang, T. T. Tan and M. Q. Chen, Phys. Chem. Chem. Phys., 2016, 18, 6113–6121 RSC
.
- T. V. Votyakova and I. J. Reynolds, Arch. Biochem. Biophys., 2004, 431, 138–144 CrossRef CAS PubMed
.
-
(a) W. D. Zhang, Q. Zhang and F. Dong, Ind. Eng. Chem. Res., 2013, 52, 6740–6746 CrossRef CAS
;
(b) H. W. Huang, X. W. Li, X. Han, N. Tian, Y. H. Zhang and T. R. Zhang, Phys. Chem. Chem. Phys., 2015, 17, 3673–3679 RSC
;
(c) J. Cao, B. Y. Xu, H. L. Lin, B. D. Luo and S. F. Chen, Dalton Trans., 2012, 41, 11482–11490 RSC
;
(d) T. S. Jamil, E. S. Mansor and R. A. Nasr, Desalin. Water Treat., 2016, 57, 14750–14761 CrossRef CAS
;
(e) W. L. Huang and Q. S. Zhu, Comput. Mater. Sci., 2008, 43, 1101–1108 CrossRef CAS
.
-
(a) M. K. Jia, X. L. Hu, S. L. Wang, Y. P. Huang and L. R. Song, J. Environ. Sci., 2015, 35, 172–180 CrossRef PubMed
;
(b) W. D. Wang, F. Q. Huang, X. P. Lin and J. H. Yang, Catal. Commun., 2008, 9, 8–12 CrossRef CAS
;
(c) X. F. Chang, J. Huang, C. Cheng, Q. Sui, W. Sha, G. B. Ji, S. B. Deng and G. Yu, Catal. Commun., 2010, 11, 460–464 CrossRef CAS
;
(d) Y. Y. Li, J. S. Wang, H. C. Yao, L. Y. Dang and Z. J. Li, J. Mol. Catal. A: Chem., 2011, 334, 116–122 CrossRef CAS
.
-
(a) C. L. Yu, F. F. Cao, G. Li, R. F. Wei, J. C. Yu, R. C. Jin, Q. Z. Fan and C. Y. Wang, Sep. Purif. Technol., 2013, 120, 110–122 CrossRef CAS
;
(b) X. Zhang and L. Z. Zhang, J. Phys. Chem. C, 2010, 114, 18198–18206 CrossRef CAS
;
(c) C. J. Bi, J. Cao, H. L. Lina, Y. J. Wang and S. F. Chen, Appl. Catal., B, 2016, 195, 132–140 CrossRef CAS
;
(d) A. Dash, S. Sarkar, V. N. K. B. Adusumalli and V. Mahalingam, Langmuir, 2014, 30, 1401–1409 CrossRef CAS PubMed
;
(e) W. Guo, Q. Qin, L. Geng, D. Wang, Y. H. Guo and Y. X. Yang, J. Hazard. Mater., 2016, 308, 374–385 CrossRef CAS PubMed
.
-
(a) Y. C. Huang, H. B. Li, M.-S. Balogun, W. Y. Liu, Y. X. Tong, X. H. Lu and H. B. Ji, ACS Appl. Mater. Interfaces, 2014, 6, 22920–22927 CrossRef CAS PubMed
;
(b) L. Q. Ye, H. Wang, X. L. Jin, Y. R. Su, D. Q. Wang, H. Q. Xie, X. D. Liu and X. X. Liu, Sol. Energy Mater. Sol. Cells, 2016, 144, 732–739 CrossRef CAS
;
(c) M. C. Long, P. D. Hu, H. D. Wu, Y. Y. Chen, B. H. Tan and W. M. Cai, J. Mater. Chem. A, 2015, 3, 5592–5598 RSC
;
(d) H. Li, J. Shang, Z. H. Ai and L. Z. Zhang, J. Am. Chem. Soc., 2015, 137, 6393–6399 CrossRef CAS PubMed
.
-
(a) P. Pyykkö, Chem. Rev., 1997, 97, 597–636 CrossRef
;
(b) S. Sculfort and P. Braunstein, Chem. Soc. Rev., 2011, 40, 2741–2760 RSC
.
-
(a) R. Wesendrup and P. Schwerdtfeger, Angew. Chem., Int. Ed., 2000, 39, 907–910 CrossRef CAS
;
(b) J. M. López-de-Luzuriaga, M. Monge, M. E. Olmos, D. Pascual and T. Lasanta, Chem. Commun., 2011, 47, 6795–6797 RSC
;
(c) A. Zaitsevskii, Chem. Phys. Lett., 2010, 495, 141–145 CrossRef CAS
;
(d) J. M. López-De-Luzuriaga, M. Monge, M. E. Olmos and D. Pascual, Organometallics, 2015, 34, 3029–3038 CrossRef
.
-
(a) S. Y. Lee, J. J. Lee, K. H. Bok, J. A. Kim, S. Kim and C. Kim, Inorg. Chem. Commun., 2016, 70, 147–152 CrossRef CAS
;
(b) S. W. Chen, P. P. Wang, C. M. Jia, Q. Lin and W. B. Yuan, Spectrochim. Acta, Part A, 2014, 133, 223–228 CrossRef CAS PubMed
;
(c) X. Wu, J. Chen and J. X. Zhao, Analyst, 2013, 138, 5281–5287 RSC
;
(d) S. Hussain, S. De and P. K. Iyer, ACS Appl. Mater. Interfaces, 2013, 5, 2234–2240 CrossRef CAS PubMed
.
-
(a) A. A. Ramadan, H. Mandil and L. Anadani, Int. J. Pharm. Pharm. Sci., 2014, 6, 203–207 Search PubMed
;
(b) A. A. Ramadan, H. Mandil and M. Maktabi, Asian J. Chem., 2010, 22, 3260–3266 CAS
.
-
(a) N. Wang, J. C. Sun, L. J. Chen, H. Fan and S. Y. Ai, Microchim. Acta, 2015, 182, 1733–1738 CrossRef CAS
;
(b) F. M. Qiao, Q. Q. Qi, Z. Z. Wang, K. Xu and S. Y. Ai, Sens. Actuators, B, 2016, 229, 379–386 CrossRef CAS
;
(c) L. Han, L. X. Zeng, M. D. Wei, C. M. Li and A. H. Liu, Nanoscale, 2015, 7, 11678–11685 RSC
;
(d) Y. Y. Huang, Z. Liu, C. Q. Liu, E. G. Ju, Y. Zhang, J. S. Ren and X. G. Qu, Angew. Chem., Int. Ed., 2016, 55, 6646–6650 CrossRef CAS PubMed
.
-
(a) L. Wu, W. M. Yin, K. Tang, K. Shao, Q. Li, P. Wang, Y. P. Zuo, X. M. Lei, Z. C. Lu and H. Y. Han, Biosens. Bioelectron., 2016, 82, 177–184 CrossRef CAS PubMed
;
(b) L. Q. Yang, X. Y. Liu, Q. J. Lu, N. Huang, M. L. Liu, Y. Y. Zhang and S. Z. Yao, Anal. Chim. Acta, 2016, 930, 23–30 CrossRef CAS PubMed
;
(c) Z. Q. Gao, M. D. Xu, M. H. Lu, G. N. Chen and D. P. Tang, Biosens. Bioelectron., 2015, 70, 194–201 CrossRef CAS PubMed
;
(d) L. Y. Chau, Q. J. He, A. L. Qin, S. P. Yip and T. M. H. Lee, J. Mater. Chem. B, 2016, 4, 4076–4083 RSC
;
(e) H. Zhao, Y. M. Dong, P. P. Jiang, G. L. Wang and J. J. Zhang, ACS Appl. Mater. Interfaces, 2015, 7, 6451–6461 CrossRef CAS PubMed
.
-
(a) V. Sharma and S. M. Mobin, Sens. Actuators, B, 2017, 240, 338–348 CrossRef CAS
;
(b) Z. H. Wang, M. Chen, J. X. Shu and Y. Li, J. Alloys Compd., 2016, 682, 432–440 CrossRef CAS
;
(c) P. Singh, P. Nath, R. K. Arun, S. Mandala and N. Chanda, RSC Adv., 2016, 6, 92729–92738 RSC
;
(d) Y. Z. Jiang, Y. Gu, G. D. Nie, M. Q. Chi, Z. Z. Yang, C. Wang, Y. Wei and X. F. Lu, Part. Part. Syst. Charact., 2016 DOI:10.1002/ppsc.201600233
.
Footnote |
† Electronic supplementary information (ESI) available. See DOI: 10.1039/c6qm00149a |
|
This journal is © the Partner Organisations 2017 |
Click here to see how this site uses Cookies. View our privacy policy here.