DOI:
10.1039/C7RA05544G
(Paper)
RSC Adv., 2017,
7, 29271-29274
Subphthalocyanine-based porous organic polymers†
Received
16th May 2017
, Accepted 31st May 2017
First published on 5th June 2017
Abstract
Subphthalocyanines (SubPcs) are bowl-shaped chromophores that possess optical properties desirable for organic electronics. Herein, we present the synthesis and characterization of two SubPc-based porous organic polymers (POPs). The SubPc-POPs exhibit respectable surface areas, and Q-bands that are significantly red-shifted in the solid-state.
Porous organic polymers (POPs) are a diverse group of materials that have garnered interest for their lightweight, highly porous architectures covering a wide range of applications including catalysis,1–3 sensing,4–7 and separations.8–11 Within the broader umbrella of POPs12 exist many subgroups, including but not limited to porous aromatic frameworks (PAFs),13 porous polymer networks (PPNs),14–16 and conjugated microporous polymers (CMPs).17 The incorporation of π-conjugated monomers allows for tuning of the bulk properties of the polymer and increases their potential utility in devices. Inclusion of chromophores with extended π-systems, such as boron-dipyrromethenes (BODIPYs), porphyrins and phthalocyanines (Pcs), into porous polymers has allowed for the creation of materials amenable to optoelectronic applications, such as photocatalysis,18,19 organic light emitting diodes (OLEDs),20,21 and photovoltaic materials.22–24 Despite the incorporation of Pcs into linear polymers25–27 and two-dimensional (2D) porous polymers,28–36 no porous material to date has incorporated subphthalocyanine (SubPc) monomers.
SubPcs, which are smaller homologues of Pcs, contain three N-fused 1,3-diiminoisoindole units that are chelated to a boron atom with a protruding axial ligand. Our inspiration to pursue the C3-symmetric bowl-shaped SubPc monomer evolved from its non-planar 14 π-electron conjugated structure which could provide access to unique porous polymers with small Stokes shifts and low reorganizational energies. These features have enabled SubPcs to achieve respectable power conversion efficiencies as both donor and acceptor materials in small molecule-based organic photovoltaic devices (OPVs).37–40 However, 2D polymeric materials based on SubPcs are essentially unknown, with the exception of their inclusion into self-assembled SubPc films.41–43 While these thin layer 2D systems could be useful for information storage or liquid crystalline technologies, only slow progress has been made in this direction. Polymers successfully incorporating SubPc monomers are rare and only a few examples of styrene copolymers with pendant SubPc groups have been reported.44,45 However, this post polymerization strategy was problematic, and did not afford a high incorporation of SubPc into the polymeric material. It also lacks the added functionality of porosity as a way to interact with guest molecules. Our strategy opted for a mild co-condensation reaction to form a boronate ester linkage, which can be constructed without an external catalyst at moderate temperatures, while simultaneously incorporating the SubPc monomeric units into a covalently linked polymeric network. Such nonplanar polymeric systems incorporating SubPcs have yet to be reported.
Herein, we present the synthesis and characterization of SubPc-POP 1 and 2 (Scheme 1). The POPs were constructed by reacting SubPcs 2b and 3b (Fig. 1) with 1,4-benzene diboronic acid (BDBA) in a 1
:
1 (v/v) mixture of dioxane and mesitylene to yield dark purple solids. These optimal reaction conditions were obtained by thoroughly screening solvent ratios and reaction temperatures (Table 1, ESI†). It should be noted that our initial polymer design aimed to utilize 1b to form a 2D POP with axial chloride ligands (Fig. 1). However, compound 1b and the TIPS-protected 1a were highly sensitive to acidic or basic environments and ambient light, which made them difficult to isolate in large quantities. We believe that this rapid degradation is mostly due to the relatively labile B–Cl bond,46 and the strongly electron-donating nature of the peripheral hydroxyl groups. As a consequence, we exchanged the axial chlorine atom for a phenoxy substituent in a one-pot synthetic procedure (pg. S4, ESI†). Interestingly, compounds 2a and 2b proved to be more chemically stable than the 1b counterpart. In an effort to investigate the effects of axial substitution on the solid-state packing of the bulk material, we also synthesized 3a and 3b by replacing the chlorine atom with a pentafluorophenoxy substituent.
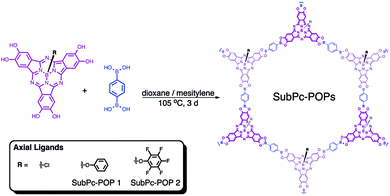 |
| Scheme 1 Synthesis of the SubPc-POPs. | |
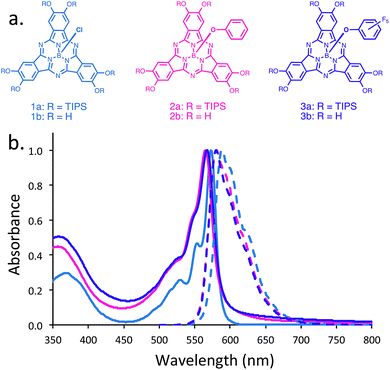 |
| Fig. 1 (a) Structures of TIPS- and hexahydroxyl-substituted SubPc monomers. (b) UV-Vis (solid line) and fluorescence spectra (dotted line) of hexahydroxyl-substituted SubPcs 1b (blue), 2b (pink), and 3b (purple) in acetone. | |
The SubPc-POPs were characterized by Fourier transform infrared (FT-IR) and 13C cross-polarization magic angle spinning (CP-MAS) spectroscopies. The FT-IR spectra of SubPc-POP 1 and 2 both displayed the characteristic C
N stretching mode of the SubPc monomer at 1465 and 1468 cm−1, and the B–O stretch from the axial ligands at 1046 and 1039 cm−1, respectively (Fig. S7 & S8, ESI†). The B–O stretching modes at 1347 and 1352 cm−1 are indicative of the boronate ester linkages for SubPc-POP 1 and 2, respectively. The connectivity of the SubPc-POPs was verified by solid-state 13C CP-MAS NMR, which contained all of the expected resonances for the materials (Fig. S11 & S12, ESI†). In addition, the incorporation of the SubPc 2b and 3b monomers was further confirmed by 1H NMR digestion experiments (Fig. S26 & S27, ESI†). Thermogravimetric analysis (TGA) revealed that SubPc-POP 1 maintains ∼85% of its weight up to 400 °C (Fig. S13, ESI†). In contrast, SubPc-POP 2 failed to give conclusive TGA data at high temperatures. Scanning electron microscopy (SEM) revealed two different bulk phase morphologies for both SubPc-POPs (Fig. 2).
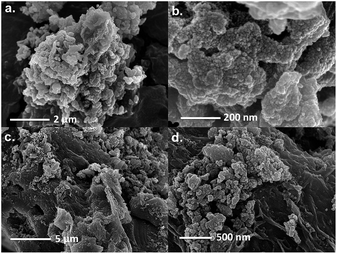 |
| Fig. 2 SEM Images of SubPc-POP 1 (a and b) and SubPc-POP 2 (c and d) at different magnifications. | |
The permanent porosities of the SubPc-POPs were evaluated by nitrogen gas adsorption isotherms at 77 K (Fig. 3). SubPc-POP 1 and 2 both exhibit reversible type I isotherms with a small but noticeable hysteresis. Application of the Brunauer–Emmett–Teller (BET) model over the low-pressure region (0.001–0.005 < P/P0 < 0.13–0.20) provided surface areas of 231 and 93 m2 g−1 for SubPc-POP 1 and 2, respectively. Nonlocal density functional theory (NLDFT) was used to estimate the pore size distributions of SubPc-POP 1 and 2 yielding values of 1.7 and 1.5 nm, respectively, which is indicative of the microporosity of the materials. The total pore volumes were calculated from the single point value of P/P0 = 0.90 to provide values of 0.131 and 0.087 cm3 g−1 for SubPc-POP 1 and 2, respectively.
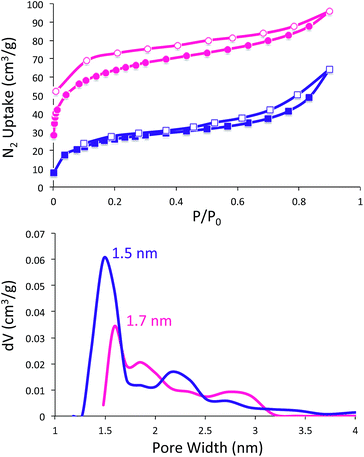 |
| Fig. 3 Nitrogen adsorption/desorption isotherms (top), and NLDFT pore size distributions (bottom) for SubPc-POP 1 (pink) and SubPc-POP 2 (purple). | |
Powder X-ray diffraction (PXRD) analysis of the SubPc-POPs revealed amorphous materials with no apparent long-range order (Fig. S9 & S10, ESI†). Initially, we anticipated that a SubPc-POP substituted with chlorine atoms in the axial position would exhibit a concave-to-ligand solid-state packing motif similar to the trans binuclear SubPc systems previously reported by Kobayashi and Durfee.47 However, these polymeric systems could not be constructed due to the chemical instability of 1b. As a consequence, we believe the larger axial phenoxy and pentafluorophenoxy substitutents of 2b and 3b prevent the monomers from forming the concave-to-ligand stacking interactions, and instead lead to the formation of disordered POPs.
The 14-π electron core of SubPcs is capable of absorbing and emitting radiation in the visible spectrum. Normalized UV-Vis and fluorescence spectra of dilute solutions (2 × 10−5 M) of 1b, 2b, and 3b in acetone are shown in Fig. 1b. The absorption maxima range from 566 to 574 nm and are attributed to the typical Q-band absorption peaks for SubPcs. Each absorption spectrum contains distinct shoulder peaks ranging from 547 to 553 nm. The weak high energy absorption bands at ∼368 nm are possibly attributed to an n–π* transition between the lone pair of the oxygen atoms and the peripheral aromatic ring.48 The fluorescence spectra of 1b, 2b and 3b revealed emission peaks at λmax 587, 581 and 580 nm, respectively. In addition, broad shoulders ranging from 616 to 624 nm, which reflected their corresponding absorption spectra, accompanied each emission spectrum.
In contrast to the monomers in solution, SubPc-POPs 1 and 2 did not exhibit any unique fluorescent properties, and were fully quenched in the solid-state. We believe that the disordered packing of the material leads to aggregation-caused quenching (ACQ) due to the rapid thermal decay of the photoexcited state.49 However, diffuse reflectance spectra of SubPc-POP 1 and 2 revealed that both materials display peak absorption bands at ∼583 and 581 nm, respectively, along with weak bands that extend into the near infrared part of the spectrum (Fig. 4). It should be noted that these absorption maxima are considerably red-shifted by ∼50–60 nm compared to the powders of 2b (λmax = 530 nm) and 3b (λmax = 520 nm) (Fig. S24 & S25, ESI†), which suggest the formation of disordered J-aggregates in the solid-state.
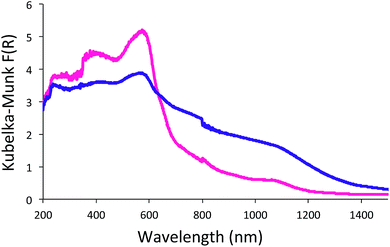 |
| Fig. 4 Diffuse reflectance of SubPc-POP 1 (pink) and SubPc-POP 2 (purple). | |
Conclusions
In conclusion, we have demonstrated that hexahydroxyl-substituted SubPc monomers can be used to construct nonplanar POPs with respectable pore sizes and surface areas. The diffuse reflectance spectra show that the absorption properties of the SubPc-POPs are significantly red-shifted compared to the monomeric SubPc units. Future studies could involve utilizing axial-substitution to tune the bulk properties of the material in the solid-state.50,51 Such investigations could yield functional nonplanar SubPc-based porous polymers with unique optoelectronic properties.
Acknowledgements
P. L. M. acknowledges the National Science Foundation (NSF) and Georgia Tech Facilitating Academic Careers in Engineering and Science (GT-FACES) for a Career Initiation Grant, and funding from The Ohio State University.
Notes and references
- Q. Q. Dang, Y. F. Zhan, X. M. Wang and X. M. Zhang, ACS Appl. Mater. Interfaces, 2015, 7, 28452–28458 CAS
. - Y. Peng, T. Ben, Y. Jia, D. Yang, H. Zhao, S. Qiu and X. Yao, J. Phys. Chem. C, 2012, 116, 25694–25700 CAS
. - S. N. Talapaneni, O. Buyukcakir, S. H. Je, S. Srinivasan, Y. Seo, K. Polychronopoulou and A. Coskun, Chem. Mater., 2015, 27, 6818–6826 CrossRef CAS
. - X. Liu, Y. Xu and D. Jiang, J. Am. Chem. Soc., 2012, 134, 8738–8741 CrossRef CAS PubMed
. - J. L. Novotney and W. R. Dichtel, ACS Macro Lett., 2013, 2, 423–426 CrossRef CAS
. - A. T. E. Vilian, P. Puthiaraj, C. H. Kwak, S. K. Hwang, Y. S. Huh, W. S. Ahn and Y. K. Han, ACS Appl. Mater. Interfaces, 2016, 8, 12740–12747 CAS
. - J. S. Yang and T. M. Swager, J. Am. Chem. Soc., 1998, 120, 11864–11873 CrossRef CAS
. - A. Ahmed, Z. Xie, K. Konstas, R. Babarao, B. D. Todd, M. R. Hill and A. W. Thornton, Langmuir, 2014, 30, 14621–14630 CrossRef CAS PubMed
. - C. H. Lau, K. Konstas, A. W. Thornton, A. C. Y. Liu, S. Mudie, D. F. Kennedy, S. C. Howard, A. J. Hill and M. R. Hill, Angew. Chem., Int. Ed., 2015, 54, 2669–2673 CrossRef CAS PubMed
. - B. Li, Y. Zhang, R. Krishna, K. Yao, Y. Han, Z. Wu, D. Ma, Z. Shi, T. Pham, B. Space, J. Liu, P. K. Thallapally, J. Liu, M. Chrzanowski and S. Ma, J. Am. Chem. Soc., 2014, 136, 8654–8660 CrossRef CAS PubMed
. - X. Zhu, C. Tian, S. M. Mahurin, S.-H. Chai, C. Wang, S. Brown, G. M. Veith, H. Luo, H. Liu and S. Dai, J. Am. Chem. Soc., 2012, 134, 10478–10484 CrossRef CAS PubMed
. - Q. Chen, M. Luo, T. Wang, J. X. Wang, D. Zhou, Y. Han, C. S. Zhang, C. G. Yan and B. H. Han, Macromolecules, 2011, 44, 5573–5577 CrossRef CAS
. - L. Li, K. Cai, P. Wang, H. Ren and G. Zhu, ACS Appl. Mater. Interfaces, 2015, 7, 201–208 CAS
. - P. Kuhn, A. Thomas and M. Antonietti, Macromolecules, 2009, 42, 319–326 CrossRef CAS
. - W. Lu, D. Yuan, J. Sculley, D. Zhao, R. Krishna and H.-C. Zhou, J. Am. Chem. Soc., 2011, 133, 18126–18129 CrossRef CAS PubMed
. - W. Lu, D. Yuan, D. Zhao, C. I. Schilling, O. Plietzsch, T. Muller, S. Bräse, J. Guenther, J. Blümel, R. Krishna, Z. Li and H. C. Zhou, Chem. Mater., 2010, 22, 5964–5972 CrossRef CAS
. - L. Chen, Y. Honsho, S. Seki and D. Jiang, J. Am. Chem. Soc., 2010, 132, 6742–6748 CrossRef CAS PubMed
. - M. Liras, M. Iglesias and F. Sánchez, Macromolecules, 2016, 49, 1666–1673 CrossRef CAS
. - J. Luo, X. Zhang and J. Zhang, ACS Catal., 2015, 5, 2250–2254 CrossRef CAS
. - B. Bonillo, R. S. Sprick and A. I. Cooper, Chem. Mater., 2016, 28, 3469–3480 CrossRef CAS
. - J. Liu, K. K. Yee, K. K. W. Lo, K. Y. Zhang, W. P. To, C. M. Che and Z. Xu, J. Am. Chem. Soc., 2014, 136, 2818–2824 CrossRef CAS PubMed
. - J. Guo, Y. Xu, S. Jin, L. Chen, T. Kaji, Y. Honsho, M. A. Addicoat, J. Kim, A. Saeki, H. Ihee, S. Seki, S. Irle, M. Hiramoto, J. Gao and D. Jiang, Nat. Commun., 2013, 4, 2736–2744 Search PubMed
. - M. Dogru, M. Handloser, F. Auras, T. Kunz, D. Medina, A. Hartschuh, P. Knochel and T. Bein, Angew. Chem., Int. Ed., 2013, 52, 2920–2924 CrossRef CAS PubMed
. - C. Gu, N. Huang, Y. Chen, L. Qin, H. Xu, S. Zhang, F. Li, Y. Ma and D. Jiang, Angew. Chem., Int. Ed., 2015, 54, 13594–13598 CrossRef CAS PubMed
. - B. N. Achar, G. M. Fohlen and J. A. Parker, J. Polym. Sci., Polym. Chem. Ed., 1982, 20, 2773–2780 CrossRef CAS
. - G. C. Bryant, M. J. Cook, S. D. Haslam, R. M. Richardson, T. G. Ryan and A. J. Thornec, J. Mater. Chem., 1994, 4, 209–216 RSC
. - M. Kimura, K. Wada, K. Ohta, K. Hanabusa, H. Shirai and N. Kobayashi, Macromolecules, 2001, 34, 4706–4711 CrossRef CAS
. - E. L. Spitler and W. R. Dichtel, Nat. Chem., 2010, 2, 672–677 CrossRef CAS PubMed
. - X. Ding, L. Chen, Y. Honsho, X. Feng, O. Saengsawang, J. Guo, A. Saeki, S. Seki, S. Irle, S. Nagase, V. Parasuk and D. Jiang, J. Am. Chem. Soc., 2011, 133, 14510–14513 CrossRef CAS PubMed
. - X. Ding, J. Guo, X. Feng, Y. Honsho, J. Guo, S. Seki, P. Maitarad, A. Saeki, S. Nagase and D. Jiang, Angew. Chem., Int. Ed., 2011, 50, 1289–1293 CrossRef CAS PubMed
. - X. Ding, X. Feng, A. Saeki, S. Seki, A. Nagai and D. Jiang, Chem. Commun., 2012, 48, 8952–8954 RSC
. - S. Jin, X. Ding, X. Feng, M. Supur, K. Furukawa, S. Takahashi, M. Addicoat, M. E. El-Khouly, T. Nakamura, S. Irle, S. Fukuzumi, A. Nagai and D. Jiang, Angew. Chem., Int. Ed., 2013, 52, 2017–2021 CrossRef CAS PubMed
. - V. S. P. K. Neti, X. Wu, M. Hosseini, R. A. Bernal, S. Deng and L. Echegoyen, CrystEngComm, 2013, 15, 7157–7160 RSC
. - L. Chen, K. Furukawa, A. Nagai, T. Nakamura, Y. Dong and D. Jiang, J. Am. Chem. Soc., 2014, 9806–9809 CrossRef CAS PubMed
. - X. Ding and B. Han, Angew. Chem., Int. Ed., 2015, 54, 6536–6539 CrossRef CAS PubMed
. - X. Feng, X. Ding and D. Jiang, Chem. Soc. Rev., 2012, 41, 6010–6022 RSC
. - K. L. Mutolo, E. I. Mayo, B. P. Rand, S. R. Forrest and M. E. Thompson, J. Am. Chem. Soc., 2006, 128, 8108–8109 CrossRef CAS PubMed
. - B. Verreet, B. P. Rand, D. Cheyns, A. Hadipour, T. Aernouts, P. Heremans, A. Medina, C. G. Claessens and T. Torres, Adv. Energy Mater., 2011, 1, 565–568 CrossRef CAS
. - G. E. Morse and T. P. Bender, ACS Appl. Mater. Interfaces, 2012, 4, 5055–5068 CAS
. - K. Cnops, G. Zango, J. Genoe, P. Heremans, M. V. Martínez-Díaz, T. Torres and D. Cheyns, J. Am. Chem. Soc., 2015, 137, 8991–8997 CrossRef CAS PubMed
. - M. Trelka, A. Medina, D. Ecija, C. Urban, O. Gröning, R. Fasel, J. M. Gallego, C. G. Claessens, R. Otero, T. Torres and R. Miranda, Chem. Commun., 2011, 47, 9986–9988 RSC
. - X. Liang, T. Chen, Y.-S. Jung, Y. Miyamoto, G. Han, S. Cabrini, B. Ma and D. L. Olynick, ACS Nano, 2010, 4, 2627–2634 CrossRef CAS PubMed
. - H. Yanagi, K. Ikuta, H. Mukai and T. Shibutani, Nano Lett., 2002, 2, 951–955 CrossRef CAS
. - B. H. Lessard and T. P. Bender, Macromol. Rapid Commun., 2013, 34, 568–573 CrossRef CAS PubMed
. - B. H. Lessard, K. L. Sampson, T. Plint and T. P. Bender, J. Polym. Sci., Part A: Polym. Chem., 2015, 53, 1996–2006 CrossRef CAS
. - S. Remiro-Buenamañana, A. Díaz-Moscoso, D. L. Hughes, M. Bochmann, G. J. Tizzard, S. J. Coles and A. N. Cammidge, Angew. Chem., Int. Ed., 2015, 54, 7510–7514 CrossRef PubMed
. - T. Fukuda, J. R. Stork, R. J. Potucek, M. M. Olmstead, B. C. Noll, N. Kobayashi and W. S. Durfee, Angew. Chem. Int. Ed., 2002, 41, 2565–2568 CrossRef CAS PubMed
. - I. Sánchez-Molina, C. G. Claessens, B. Grimm, D. M. Guldi and T. Torres, Chem. Sci., 2013, 4, 1338–1344 RSC
. - S. Dalapati, E. Jin, M. Addicoat, T. Heine and D. Jiang, J. Am. Chem. Soc., 2016, 138, 5797–5800 CrossRef CAS PubMed
. - J. Guilleme, D. González-Rodríguez and T. Torres, Angew. Chem., Int. Ed., 2011, 50, 3506–3509 CrossRef CAS PubMed
. - J. D. Virdo, Y. H. Kawar, A. J. Lough and T. P. Bender, CrystEngComm, 2013, 15, 3187–3199 RSC
.
Footnote |
† Electronic supplementary information (ESI) available. See DOI: 10.1039/c7ra05544g |
|
This journal is © The Royal Society of Chemistry 2017 |
Click here to see how this site uses Cookies. View our privacy policy here.