DOI:
10.1039/D3RA05020C
(Review Article)
RSC Adv., 2023,
13, 27333-27358
Recent theranostic applications of hydrogen peroxide-responsive nanomaterials for multiple diseases
Received
25th July 2023
, Accepted 31st August 2023
First published on 12th September 2023
Abstract
It is well established that hydrogen peroxide (H2O2) is associated with the initiation and progression of many diseases. With the rapid development of nanotechnology, the diagnosis and treatment of those diseases could be realized through a variety of H2O2-responsive nanomaterials. In order to broaden the application prospects of H2O2-responsive nanomaterials and promote their development, understanding and summarizing the design and application fields of such materials has attracted much attention. This review provides a comprehensive summary of the types of H2O2-responsive nanomaterials including organic, inorganic and organic-inorganic hybrids in recent years, and focused on their specific design and applications. Based on the type of disease, such as tumors, bacteria, dental diseases, inflammation, cardiovascular diseases, bone injury and so on, key examples for above disease imaging diagnosis and therapy strategies are introduced. In addition, current challenges and the outlook of H2O2-responsive nanomaterials are also discussed. This review aims to stimulate the potential of H2O2-responsive nanomaterials and provide new application ideas for various functional nanomaterials related to H2O2.
1 Introduction
As a biomolecule, hydrogen peroxide (H2O2) is commonly generated from specifically triggered enzymatic systems and cellular metabolism, and has been linked to the development and progression of multiple diseases.1–6 Overexpression of H2O2 is a typical feature of the microenvironment in numerous diseases.7,8 It can be catalyzed to produce significant substances, including oxygen (O2) and hydroxyl radicals (˙OH). The O2 not only alleviates the hypoxic environment of some diseases such as tumors and inflammation, but also can be converted into singlet oxygen to kill bad cells.9–11 The ˙OH as a type of reactive oxygen species (ROS) can cause the oxidative stress of cells, which could be beneficial in treating tumors and bacterial infections.10 However, the ability of H2O2 also is a double-edged sword and poses a risk in certain conditions.12,13 Excessive H2O2 induced DNA damage and protein denaturation, resulting in mutations and genetic instability.11 It is unfavorable to the treatment of diseases, such as inflammation, bone injury, cardiovascular diseases, and so on. By observing the changes of H2O2 concentration in the lesion, the corresponding physiological and pathological state of the lesion could be detected.14 Therefore, there should be different choices for catalyzing or consuming H2O2 based on different disease types.
With the development of nanotechnology, a growing number of H2O2-responsive nanomaterials have been designed and investigated for biomedical applications. H2O2-responsive nanomaterials can be simply classified as organic, inorganic, and hybrid nanomaterials. In particular, the core components of H2O2-responsive organic nanomaterials mainly are organic molecules and enzymes.15–18 For example, some organic molecules react with H2O2 causing the changes of their absorption or emission characteristics, such as 2,2′-azino-bis(3-ethylbenzothiazoline-6-sulfonic acid) (ABTS).3,19 Some enzymes like catalase (CAT) were applied for decomposing H2O2, producing O2 and water.16–18,20,21 Compared to organic nanomaterials, inorganic H2O2-responsive nanomaterials have greater stability, more functionalities, and a broader range of applications. There inorganic nanomaterials with enzyme-like properties, such as metal oxides and noble metal nanoparticles, were utilized to react with H2O2.22,23 According to the composition of inorganic nanomaterials, the reaction products can be broadly classified as metal ions, O2 or ROS, etc. And some hybrid nanomaterials formed by the combination of organic and inorganic materials combine their respective advantages and play an important role in H2O2-related applications.24,25
For therapeutic applications, H2O2-responsive nanomaterials provided a variety of treatment methods, mainly including producing ROS and consuming H2O2.10,26–30 These nanomaterials employ their characteristics to participate in the treatment of many diseases, including tumors, bacteria, inflammation, cardiovascular diseases, bones, neurologic diseases and ophthalmic diseases.26,27,31,32 For tumors and bacterial infections, decomposing or catalyzing H2O2 often generates other ROS to enhance the treatment effect, while for other diseases such as inflammation, it's crucial to eliminate the oxidative stress of H2O2 to foster injury repair and maintain normal physiological state.10,26–30,33 The research focused on the elimination of H2O2 has also been applied for the treatment of diseases such as inflammation, uveitis, and ischemic stroke.34–36 Furthermore, H2O2-related diagnosis based on H2O2-responsive nanomaterials holds promise for the diagnosis of multiple diseases via medical imaging technology like fluorescent imaging (FLI), photoacoustic imaging (PAI), ultrasound (US) imaging, and magnetic resonance imaging (MRI).37–40 For example, the catalytic decomposition of H2O2 produces O2 bubbles that reflect ultrasound signals in US imaging.41 Another example, the conversion of ABTS into oxidized ABTS in the presence of H2O2 leads to strong near-infrared (NIR) absorbance, thus used for PA imaging.42 The combination of H2O2-responsive nanomaterials and imaging technology provides a new avenue for disease diagnosis. As more diseases are found to be closely related to H2O2, understanding the working principle of H2O2-responsive nanomaterials and developing novel and efficient theranostic reagents will unlock additional application channels of H2O2-responsive nanomaterials and help address various diseases.
This review summarizes recent advancements in the treatment and imaging of partial diseases associated with H2O2, including the design of H2O2-responsive nanomaterials and application strategies for different diseases (Fig. 1). The mechanisms and functions of various types of H2O2-responsive nanomaterials in the treatment of different diseases are also discussed and summarized. Furthermore, therapeutic applications utilizing H2O2-responsive nanomaterials are categorized into two different groups: (1) producing ROS and (2) consuming H2O2. The former included tumors, bacterium, dental diseases. The latter included inflammations, cardiovascular diseases, bones, neurologic diseases and ophthalmic diseases. The diagnosis agents that are based on H2O2-responsive nanomaterials are utilized for diagnosis purposes in four categories: fluorescence imaging, photoacoustic imaging, ultrasonic imaging, and magnetic resonance imaging. The purpose of this review is to integrate recent findings on H2O2-related diseases and H2O2-responsive nanomaterials, and to advance the field of H2O2-related biomedicine. These strategies and studies allow for better understanding of the functions and mechanisms of H2O2-responsive nanomaterials and also open up more possibilities for other biomedical application scenarios.
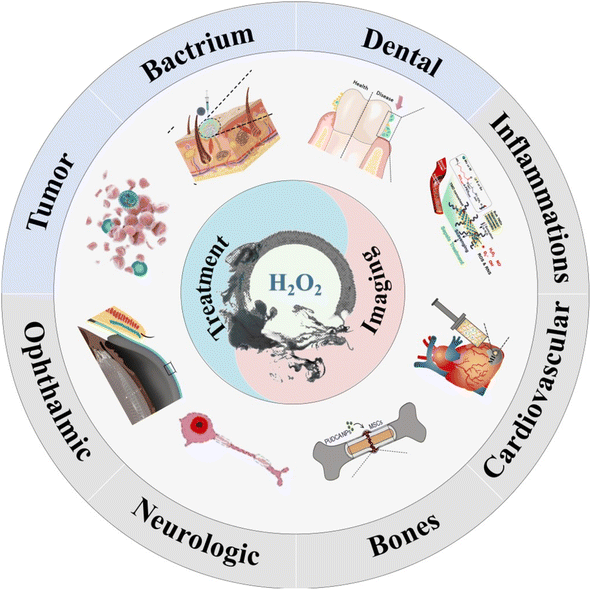 |
| Fig. 1 Theranostic applications of H2O2-responsive nanomaterials, including tumors, bacterium, dental diseases, inflammations, cardiovascular diseases, bones, neurologic diseases, and ophthalmic diseases. | |
2 H2O2-responsive nanomaterials
In this section, the H2O2-responsive nanomaterials are introduced that came in three forms (Table 1): organic nanomaterials, inorganic nanomaterials, and hybrid nanomaterials.
Table 1 Categorization of H2O2-responsive nanomaterials in recent years
Category |
|
Material |
Mechanism |
Mode |
Ref. |
Organic nanomaterials |
Small molecules |
BSA-MBPB |
Boron-carbon link |
Releasing drug |
Zeng et al.47 |
BODIPY-based photosensitizer |
Arylboronate group |
Recovering fluorescence |
Wang et al.48 |
PF1 |
Boric acid |
Detecting H2O2 |
Chang et al.49 |
Diselenide based cross-linker nanomaterial |
Diselenide bridges |
Releasing DOX |
Jo et al.51 |
HKPerox-1 and HKPerox-2 |
Payne/Dakin reaction |
Molecular recognition of H2O2 |
Ye et al.54 |
DPPEA-HC |
Reaction of triphenylphosphine |
Detecting H2O2 |
Soh et al.56 |
ABTS |
Oxidation reaction |
Photoacoustic imaging |
Ding et al.57 |
Enzymes |
HA-CAT@aCe6 |
Catalase |
Producing O2 |
Phua et al.63 |
PLGA-R837@Cat |
Catalase |
Relieving the tumor hypoxia |
Chen et al.64 |
Organic polymer nanoparticles |
PVAX polymer nanoparticles |
Peroxalate ester linkages |
Decreasing H2O2 and releasing drug |
Park et al.66 |
POD-PEG NPs |
Oxalate ester bond |
Releasing drug |
Ou et al.67 |
Block copolymer NPs |
Change of particle size |
Releasing drug |
Phan et al.65 |
SCNPs |
Heavy-atom effect |
Detecting H2O2 |
Lu et al.68 |
Metalloporphyrin-based porous organic polymer |
POD-like activity |
Detecting H2O2 |
Liu et al.69 |
Hydrogels |
Hydrogel based on the thiazolidinone group |
Gel–sol phase transition |
Releasing drug |
Ren et al.71 |
L012@PAni-PAAm hydrogels |
Biosensors |
Monitoring H2O2 |
Guo et al.72 |
Inorganic nanomaterials |
Metal oxides |
MnO2@PtCo nanoflowers |
Catalase mimic |
Relieving hypoxic condition |
Wang et al.81 |
IR780-sMnO2-PCM NPs |
Catalase mimic |
Producing O2 |
Zhang et al.82 |
Co3O4 NPs |
Oxidase mimic and peroxidase mimic |
Detecting H2O2 |
Zhang et al.85 |
Metal ions |
H–MnO2@TPyP@Bro |
Generating ˙OH by Mn2+ |
Chemodynamic therapy |
Zhu et al.90 |
Fe(III)@WS2-PVP NPs |
Fenton reation |
Chemodynamic therapy |
Wu et al.91 |
Single-atom enzyme |
Fe-SANzyme nanozymes |
Catalase-like activity |
Producing O2 |
Zhang et al.96 |
Pd-based SAzyme |
POD mimic |
Generation of ˙OH |
Zhu et al.97 |
Noble metal nanoparticles |
SiO2–Pt |
CAT mimic |
Producing O2 |
Lyn et al.104 |
Ru nanoframes |
POD mimic |
Generation of ˙OH |
Ye et al.107 |
Hybrid nanomaterials |
Metal–organic frameworks |
ICG@Mn/Cu/Zn-MOF@MnO2 |
Producing ˙OH by Cu+ and Mn2+ |
CDT |
Cheng et al.24 |
GOx@ZIF@MPN |
Producing ˙OH by Fe2+ |
CDT |
Zhang et al.113 |
Quantum dots and their capping agents or coating |
MPA capped CdTe QDs |
Fluorescence signal change |
Detecting H2O2 |
Castro et al.115 |
Si–CdTe hybrid QDs |
Cd–S bond |
Detecting H2O2 |
Zhou et al.116 |
|
Cy5-labeled HRP–QDs |
Fluorescence change |
Detecting H2O2 |
Huang et al.118 |
|
Noble-metal nanoclusters and organic protective agents |
AgNCs protecting BSA |
Dual-emission fluorescent |
Detecting H2O2 |
Chen et al.120 |
|
AgNCs protecting [C4py]124 |
Coordination bond between AsO33− and π molecular orbitals |
Detecting H2O2 |
Wang et al.121 |
2.1 H2O2-responsive organic nanomaterials
Organic nanomaterials possess unique advantages, such as biosafety and easy synthesis. We summarize some H2O2-responsive organic nanomaterials, including small molecules, enzymes, organic polymer nanoparticles and hydrogels.
2.1.1 Small molecules.
2.1.1.1 Boronate-based small molecules. Recently, boronate-based molecules have been reported as H2O2-responsive organic molecules, which can be selectively hydrolyzed by H2O2.15 Its reaction with H2O2 was mainly based on the cleavage of the formed boron-carbon link.43 Boronate-based organic nanoparticles were widely used in the biomedical field, including H2O2-responsive drug delivery and detection.44–46For example, Zeng et al. designed a pro-photosensitizer MBPB based on boron-carbon link, which could be hydrolyzed by H2O2 and achieved responsive release of methylene blue (MB) and quinone methide (QM) in Fig. 2a.47 After being ingested by tumor cells, the MBPB under the activation of H2O2 would release MB to produce 1O2 and QM to enhance oxidative stress, and finally play a role in enhancing photodynamic therapy. Moreover, Wang et al. designed a H2O2-responsive photosensitizer contained a diiodo distyryl boron dipyrromethene (BODIPY) core and an arylboronate group, which quenched the fluorescence signal of BODIPY by photoinduced electron transfer (PET).48 The arylboronate group did not break without H2O2, and the fluorescence of PET was still inhibited. However, in the presence of H2O2, the strong fluorescence emission would be produced by BODIPY. Herein, the combination of arylboronate group and BODIPY as H2O2-responsive fluorescence nanomaterials had enormous potential. Chang and coworkers reported a peroxyfluor-1 (PF1) molecule which could be used for detecting H2O2 in living cells via fluorescence signals change (Fig. 2b).49 PF1 used the boric acid deprotection mechanism to detect H2O2 in aqueous solutions of reactive oxygen species, providing an unprecedented selectivity and optical dynamic range.
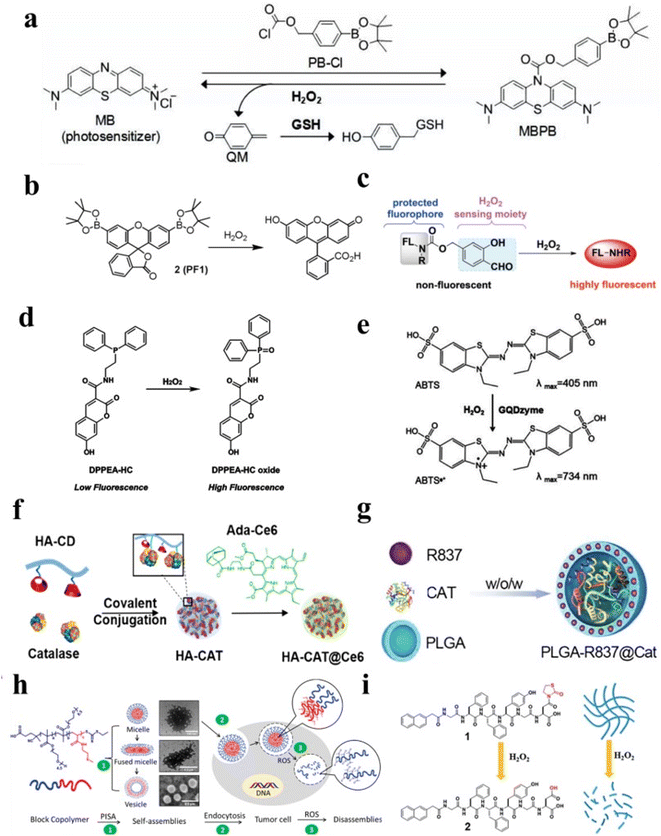 |
| Fig. 2 (a) A schematic diagram illustrating of MBPB to deliver methylene blue. Reproduced from ref. 47 copyright 2019, Elsevier Ltd. (b) A schematic diagram illustrating H2O2-related fluorescent conversion of PF1. Reproduced from ref. 49 copyright 2004, American Chemical Society. (c) Schematic of a tandem Payne/Dakin reaction. Reproduced from ref. 54 copyright 2018, Wiley-VCH Verlag GmbH & Co. KGaA, Weinheim. (d) A schematic diagram illustrating the fluorescence intensity of DPPEA-HC enhanced. Reproduced from ref. 56 copyright 2004, Elsevier Ltd. (e) A schematic diagram illustrating the conversion of ABTS in the presence of H2O2. Reproduced from ref. 57 copyright 2019, American Chemical Society. (f) The preparation process of HA-CAT@Ce6 by the conjugation of HA with CAT. Reproduced from ref. 63 copyright 2019, American Chemical Society. (g) Schematic of PLGA to encapsulate CAT. Reproduced from ref. 64 copyright 2019, WILEY-VCH Verlag GmbH & Co. KGaA, Weinheim. (h) Schematic of the evolution of particle size and morphology of H2O2-sensitive block copolymer NPs. Reproduced from ref. 65 copyright 2022, American Chemical Society. (i) Schematic of releasing drugs via H2O2-responsive hydrogels. Reproduced under terms of the CC-BY license.71 Copyright 2017, Chunhua Ren, published by RSC Advances. | |
2.1.1.2 H2O2-responsive diselenide bridges. Similar to the B–C link, the diselenide bridge could be also cleaved by H2O2.50 Based on this, H2O2-responsive diselenide bridges were often used for controllable drug delivery. For example, Jo et al. designed H2O2-stimuli responsive nanomaterials based on diselenide bridges, which showed a rapid release of doxorubicin (DOX) in the presence of H2O2.51 The diselenide bridge could be cleaved and converted to seleninic acid, providing a good direction for biomedical research.52 Not only that, some selenide-based nanoparticles can produce fluorescence via the oxidation of H2O2.53
2.1.1.3 Other small molecules. In addition, some molecules can also serve as probes to achieve H2O2 detection by reacting with H2O2.15 For example, Ye et al. reported novel fluorescent probes HKPerox-1 and HKPerox-2 based on the H2O2-responsive group and tandem Payne/Dakin reaction to molecular recognition of H2O2 (Fig. 2c).54 A benzopyrylium-coumarin (BC) probe has been reported to detect H2O2.55 And a fluorescent probe 7-hydroxy-2-oxo-N-(2-(diphenylphosphino)ethyl)-2H-chromene-3-carboxamide (DPPEA-HC) consisting of a 7-hydroxycoumarin moiety and a diphenylphosphine moiety have been designed to detect H2O2 (Fig. 2d).56 Not only that, ABTS could be converted into oxidized ABTS in the presence of H2O2.57 As depicted in Fig. 2e, oxidized ABTS possessed strong NIR absorbance, which could be used for PA imaging. Therefore, this implies that organic molecules-based nanomaterial may be an effective nanoplatform for H2O2-related diseases treatment and diagnosis.
2.1.2 Enzymes. There are amounts of enzymes that can react with H2O2, such as CAT, superoxide dismutase (SOD), horseradish peroxidase (HRP), peroxidase (POD) and so on.58–60 For example, CAT, a H2O2-metabolizing enzyme, can catalyze H2O2 to generate O2, which is an important pathway for H2O2 metabolism.61,62 Then, the generation of O2 can be used to alleviate hypoxia tumor microenvironment (TME) or convert to ROS. The involvement of CAT in tumor therapy is a major trend in biomedical fields. However, as a natural enzyme, it is easy to leak into the normal tissue before entering the tumor area, thus affecting the activity of normal cells. Similarly, its activity during the transportation is affected by the surrounding environment. To prevent organic molecules or enzymes' premature leakage and improve their delivery, nanocarriers were usually used to load them into disease regions. Organic carriers loading H2O2-related enzymes have the advantages, of high loading efficiency and good biocompatibility.63 For instance, hyaluronic acid (HA) served as a biodegradable carrier to load CAT by covalent conjugation, which had tumor-targeting ability (Fig. 2f).63 The encapsulated CAT could decompose endogenous H2O2 to produce oxygen and relieve hypoxia in situ. In other study, Chen et al. synthesized a core–shell nanoparticle-based poly(lactic-co-glycolic) acid (PLGA) to encapsulate CAT in order to protect CAT in Fig. 2g.64
2.1.3 Organic polymer nanoparticles. It is becoming increasingly apparent that organic polymer nanoparticles are widely used in H2O2-responsive biomedical fields because of their stability advantage.65 For example, Park et al. synthesized polyoxalate containing vanillyl alcohol (PVAX) polymer nanoparticles for H2O2 scavenging and drug release.66 Ou et al. designed prodrug-delivery nanoplatforms based on podophyllotoxin (POD) prodrug linking with poly(ethylene glycol)(n)monomethacrylate.67 In the presence of H2O2, POD drugs could be released by a self-sacrificing pathway. In another study, Phan et al. reported the evolution of particle size and morphology of H2O2-sensitive block copolymer NPs from spherical micelles, fused micelles, to vesicles over time (Fig. 2h).65 Not only that, polymers could also be used to detect H2O2.68 Fluorescent single-chain polymer nanoparticles (SCNPs) with aggregation-induced emission (AIE) were used for H2O2 detection through intermolecular heavy-atom effect. Liu and coworkers designed a metalloporphyrin-based porous organic polymer which exhibited excellent peroxidase-like activity for detecting H2O2.69 The polymer possessed a wide linear range of 50–1800
μM and a relative lower limit of detection (LOD) of 26.70
μM for H2O2. Therefore, the polymer could accurately detect the change of H2O2 under physiological and pathological conditions.
2.1.4 Hydrogels. As a safe material, hydrogels have many advantages, such as high porosity, which is conducive to substance exchange and drug loading.70 At the same time, hydrogels are sensitive to external stimuli, such as pH, redox, and so on.71 Therefore, these advantages can be utilized to design hydrogels with H2O2-response for drug delivery or H2O2 detection. For example, Ren et al. designed a novel H2O2-responsive hydrogel based on the thiazolidinone group.71 With the addition of H2O2, hydrogels underwent gel–sol phase transition, which was used for drug release (Fig. 2i). In other studies, hydrogels also had great applications in the field of biosensing.72–74 Guo et al. designed L012@PAni-PAAm hydrogels as biosensors to monitor H2O2 produced from cardiomyocytes.72In conclusion, organic nanomaterials play a very important role in H2O2-related biomedical applications. Reasonable design of organic nanomaterials with higher safety, better therapeutic efficiency and more functionality is the focus of future research.
2.2 H2O2-responsive inorganic nanomaterials
Owing to the advancement of nanotechnology, many inorganic materials now have possessed enzyme-mimic catalytic effects, mainly including oxidase-like, peroxidase-like, CAT-like, and superoxide dismutase-like.75,76 Compared with natural enzymes, inorganic materials have amounts of advantages, such as facile fabrication, low cost, and robust stability against severe conditions.77 In this part, we list four types of H2O2-responsive inorganic nanomaterials: metal oxides, metal ions, single-atom nanozymes, and noble metal nanoparticles.
2.2.1 Metal oxides. Currently, various metal oxide inorganic materials, which served as enzyme mimics have been reported that could directly or indirectly react with H2O2, such as manganese (Mn), cobalt (Co), cerium (Ce), and ruthenium (Ru).78–80 For example, H2O2 would be catalyzed to O2 by manganese dioxide (MnO2).81 Then, the increasing concentration of O2 could be used to enhance the therapeutic effect of various oxygen-dependent therapeutic methods. As shown in Fig. 3a, Zhang et al. loaded MnO2 and IR780 into a thermal-responsive phase-change material (IR780-sMnO2-PCM NPs) for enhancing the photodynamic therapy effect.82 In addition to Mn, Co-based metal oxides have been proven that possessed SOD-like, CAT-like, and POD-like catalytic activities.83,84 Based on those, cobaltosic oxide (Co3O4) nanoparticles have been designed as biosensing platforms to detect H2O2.85 In another study, Ce-based metal oxides have been reported that the ratio of Ce3+/Ce4+ determined the enzyme-like properties, such as CAT mimic.86 In addition, vanadium (V)-based and titanium (Ti)-based metal oxides exhibited POD-like activity that has been reported.87,88
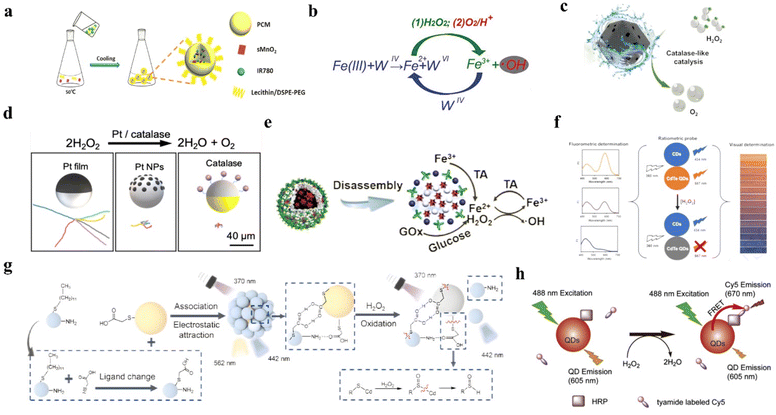 |
| Fig. 3 (a) The synthetic process of IR780-sMnO2-PCM NPs. Reproduced from ref. 82 copyright 2019, WILEY-VCH Verlag GmbH & Co. KGaA, Weinheim. (b) Schematic of Fe(III)@WS2-PVP nanocapsules to generate Fe2+ and trigger a Fenton reaction. Reproduced from ref. 91 copyright 2018, American Chemical Society. (c) Schematic of The Fe-SAzyme exhibited CAT-like capacity to decompose H2O2 to O2. Reproduced from ref. 96 copyright 2022, Wiley-VCH GmbH. (d) Schematic of Pt NPs catalyzed H2O2 and the synthetic process of Pt NPs. Reproduced from ref. 104 copyright 2021, American Chemical Society. (e) Schematic of TA reducing Fe(III) to Fe(II). Reproduced from ref. 113 copyright 2018, American Chemical Society. (f) The schematic of MPA-CdTe QDs to detect H2O2. Reproduced from ref. 115 copyright 2019, Elsevier B.V. (g) Schematic of Si–CdTe hybrid QDs to detect H2O2. Reproduced from ref. 116 copyright 2022, Wiley-VCH GmbH. (h) Schematic of QDs labeled Cy5 to detect H2O2. Reproduced from ref. 118 copyright 2012, Elsevier B.V. | |
2.2.2 Metal ions. As with metal oxides, metal ions can also react with H2O2, such as Mn ions (Mn2+), Fe ions (Fe2+), cuprum ions (Cu2+), and so on.89 The main mechanism of the reaction with H2O2 is Fenton or Fenton-like reaction. For example, Zhu et al. synthesized a nanoplatform that could release Mn2+ in the presence of overexpressed glutathione (GSH).90 Subsequently, Mn2+ was oxidized by H2O2 and generated toxic ˙OH. Besides Mn ions, Fe(III)@WS2-PVP nanocapsules to generate Fe2+ and trigger a Fenton reaction have been designed (Fig. 3b).91 In the nanocapsule, the Fe(III) was reduced to form Fe2+ by WS2. Then, the continuously generated Fe2+ reacted with H2O2 to produce ˙OH in tumor cell. The same as irons, Cu2+ and Co2+ have been proven to trigger the H2O2 to generate ˙OH.92,93
2.2.3 Single-atom nanozymes. Recently, a single-atom enzyme (SAzyme) has been reported to apply for developing H2O2-related biomedicines due to its unique catalytic properties.94 For example, SAzyme possesses POD-like activity which catalyzed H2O2 into ˙OH in the TME.95,96 Zhang et al. designed an Fe-based SAzyme by edge-site engineering, which exhibited CAT-like capacity to decompose H2O2 to O2, and the edge-site engineering would enhance the CAT-like activity (Fig. 3c).96 The Fe-SANzyme significantly removed ROS and reduced oxidative stress through CAT-like catalysis, thereby eliminating pathological angiogenesis in animal models of retinal angiopathies without affecting normal vascular repair. And a novel palladium (Pd)-based SAzyme, which could generate ˙OH in the presence of H2O2 has been synthesized.97 At present, the research on H2O2-related SAzymes is still in its infancy, and it will be a hot spot in future research.
2.2.4 Noble metal nanoparticles. Like metal oxides, some noble metal nanoparticles can produce or consume H2O2, such as aurum (Au), platinum (Pt), ruthenium, and palladium.83,94,98–100 For example, Pt, a common noble metal, is often used to catalyze H2O2 due to its CAT-like ability, electrochemical production, etc.101–103 As depicted in Fig. 3d, silicon dioxide (SiO2)–Pt NPs have been designed, which could decompose H2O2 to O2 as like CAT.104 In other research, a motor that combined Au and Pt moved along a stable direction by the catalytic decomposition of H2O2.105 And, Ru nanoparticles and Pd-based nanozymes have been proven that exhibited HRP, CAT, or POD-like activities.100,106 Ye et al. reported Ru nanoframes with an octahedral shape that were three times more active than natural POD.107 Furthermore, noble metal alloy nanoparticles, such as iridium (Ir)/Ru and Pt/Cu alloy nanoparticles, also exhibited enzyme-like activities.108,109 Thus, the applications of noble metal in H2O2-responsive nanomaterials are showing great potential for the theranostic of diseases.
2.3 Hybrid nanomaterials
Most hybrid nanomaterials are composed of close integration of organic components, inorganic components, or dual types of ingredients.110 Hybrid materials can combine the advantages of organic and inorganic materials for more efficient biological applications.
2.3.1 Metal–organic frameworks. Metal–organic frameworks (MOFs), a type of hybrid material, are consisted of metal ions (or clusters) and organic groups, and their role in nanomaterials is becoming central.111 Recently, many researches have shown that MOF could serve as a nanozyme, which would react with H2O2.112 For example, Cheng et al. reported a nanoplatform ICG@Mn/Cu/Zn-MOF@MnO2 that could be used for synergistic tumor treatment.24 The nanoplatform could release Cu+ and Mn2+ to catalyze H2O2 into ˙OH in tumor cells. More than that, the organic components of MOF could assist inorganic components for H2O2-related applications. For instance, tannic acid (TA) could quickly reduce Fe(III) to Fe(II), and then Fe(II) catalyzed H2O2 to ˙OH (Fig. 3e).113
2.3.2 Quantum dots and their capping agents or coating. Because of their unique optical properties, quantum dots (QDs) were widely used for fluorescence-based sensing.114 H2O2-sensitive QDs have been designed to detect H2O2 via coating organic molecules.114 Castro et al. designed a 3-mercaptopropionic acid (MPA) capped cadmium telluride (CdTe) QDs with orange-emitting and combined with a blue-emitting carbon dot (CD).115 When H2O2 was not present, MPA-CdTe QDs and CDs could produce fluorescence signals of different wavelengths, respectively (Fig. 3f). With the addition of H2O2, the emitting fluorescence of MPA-CdTe QDs was decreased while the emitting fluorescence of CDs remained constant. The change of fluorescence signal was linearly correlated with H2O2. Using this special fluorescence signal change, it could be used as a method to determine H2O2. In another study, water-soluble Si–CdTe hybrid QDs have been designed to detect H2O2.116 In the presence of H2O2, the fluorescence of CdTe QDs was extinguished due to the breakage of the Cd–S bond (Fig. 3g). Furthermore, Te-doped CDs have been reported to scavenge H2O2 for protecting normal cells.117 In addition to fluorescence attenuation, QDs-related fluorescence enhancement could also be used to detect H2O2. For example, Huang et al. reported a new strategy of fluorescence resonance energy transfer (FRET) for enhanced fluorescence in the HRP-catalyzed oxidation with H2O2.118 In the presence of H2O2, the QDs served as a fluorescent donor, and the tyramide labeled Cy5 served as an acceptor to generate a fluorescent wavelength of 670 nm (Fig. 3h).
2.3.3 Noble-metal nanoclusters and organic protective agents. Noble-metal nanoclusters can be served as biomedical probes to detect H2O2 due to their excellent fluorescence properties.119 Chen et al. utilized bovine serum albumin (BSA) as a stabilizer to synthesize argentum nanoclusters (AgNCs), which could be applied for selectively H2O2 detection.120 Wang et al. prepared a fluorescent probe AgNC which was collaboratively composed of pyridinium-based ionic liquid.121 The original fluorescence could be quenched by the coordination bond between AsO33− and π molecular orbitals. With the addition of H2O2, AsO33− could be oxidized to AsO43− and the origin fluorescence would recover. In addition to Ag, Au nanoclusters (HRP-AuNCs) have also been used to investigate H2O2 secretion at the single-cell level.122 Not only that, organic agents-stabilized Cu nanoclusters have also served as H2O2-responsive fluorescent probes in biomedical applications.123To summarize, an increasing number of H2O2-responsive nanomaterials, such as organic, inorganic, and hybrid nanomaterials, are being employed in a variety of biological applications. Future research will also concentrate on the discovery novel nanomaterials as well as the advancement of existing nanomaterials. In the subsequent sections, we summarize and review the various biomedical applications of novel nanomaterials, including disease therapy and imaging.
3 The mechanism of H2O2-responsive nanomaterials for biomedical applications
The mechanism of H2O2 responsive nanomaterials can be broadly divided into three categories: (1) utilize H2O2 to change the state of organic molecules; (2) eliminate H2O2 and produce oxygen; (3) catalyze H2O2 to produce hydroxyl radical. For the former (1), the H2O2 can directly affect the nanomaterials composed of ABTS molecules, aromatic boronates-based molecules, or fluorescent molecules, causing the change of their characteristic absorption or emission peak, or the cleavage of molecules. For the latter (2) and (3), the by-products such as O2, ˙OH, and metal ions, generated by those nanomaterials and H2O2 reactions played central role in their biological applications. For example, the generation of ˙OH by catalyzing H2O2 can cause oxidative stress, which can then induce tumor cells or bacterial death. The generation of O2 by decomposed H2O2 can alleviate hypoxia, or be converted into 1O2, or be used as US contrast agents for enhancing the effect of disease treatment. Another example, a lot of ions can react with H2O2 through a Fenton reaction or a Fenton-like reaction and can be monitored by magnetic resonance imaging. Here, we listed the main usage mechanisms of H2O2 (Fig. 4).
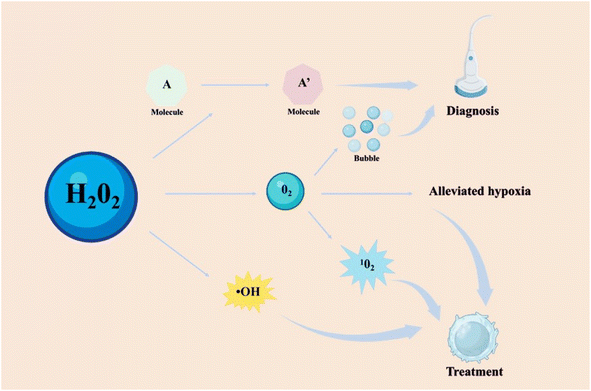 |
| Fig. 4 The typical mechanism of H2O2-responsive nanomaterials in the biomedical applications. | |
4 Biomedical applications of H2O2-responsive nanomaterials
The occurrence and progression of many diseases are closely related to H2O2 levels. Reasonable selection of H2O2 treatment methods is of great help into the treatment and diagnosis of diseases. H2O2-related diseases treatment mainly relies on oxidative stress from H2O2 generated ROS, increasing oxygen content for various therapy methods and removing H2O2. Thus, the H2O2-responsive nanomaterials are categorized into two different groups: (1) producing ROS and (2) reducing oxidative stress. The former included tumors, bacterium, dental diseases. The latter included inflammations, cardiovascular diseases, bones, neurologic diseases and ophthalmic diseases. H2O2-related imaging diagnosis are based on the release product of nanomaterials after interacting with H2O2, such as gas bubbles, metal ions, and fluorescence signal changes. Herein, we provide an overview of current researches on H2O2-related various therapy and imaging detection applications (Table 2).
Table 2 Categorization of H2O2-responsive nanomaterials in recent years
Research field |
Method |
Nanomaterial |
Mechanism |
Application |
Ref. |
Tumors |
Treatment |
Mn-CDs |
Produce O2 |
Enhanced PDT |
Jia et al.7 |
Ce6-DNAzyme NPs |
Convert H2O2 to ˙OH for type I PDT |
Combined type I and II PDT |
Liu et al.27 |
CSI@Ex-A |
Produce O2 via CAT |
Enhanced SDT |
Wu et al.138 |
HMONs-MnOx |
Produce O2 via Mn-based nanozymes |
Enhanced SDT |
Zhu et al.140 |
GDY–CeO2–miR181a–PEG–iRGD |
Produce O2 via CeO2 |
Enhanced RT |
Zhou et al.143 |
AVPt@HP@M |
Produce O2 via Pt NPs |
Enhanced RT |
Gong et al.141 |
PLGA-R837@CAT |
Produce O2 via CAT |
Enhanced immunotherapy |
Chen et al.64 |
AFe NPs |
Generate ˙OH via Fe2+ |
Enhanced CDT |
Chen et al.158 |
Imaging |
MS@MnO2 NPs |
Deplete GSH and produce ˙OH |
Enhanced CDT |
Lin et al.159 |
Cu2MoS4@GOx |
Enhance multiple enzyme effect |
Synergistic therapy |
Chang et al.21 |
ACD |
Turn on the fluorescence |
H2O2-responsive FLI |
Zhao et al.171 |
Ag/Ag2S NPs |
Turn on the fluorescence |
FLI of liver injury and tumor |
Zhang et al.172 |
GQDzyme/ABTS NPs |
Convert ABTS into oxidized ABTS |
PAI of nasopharyngeal carcinoma in vivo |
Ding et al.57 |
LOD/CAT-loaded nanogels |
Generate abundant O2 gas |
Efficient diagnostic nanoprobes of US imaging |
Wu et al.180 |
AuNCs@mSiO2@MnO2 |
Release Mn2+ |
Enhanced MRI signal intensity |
Yin et al.185 |
MnPcE4 |
Achieve trimodal imaging |
Achieved multimodal imaging |
Wang et al.187 |
Bacterium |
Treatment and imaging |
CoIITBPP(bpy) nanozymes |
Enhance CAT-like activity |
Enhanced PDT |
Hu et al.8 |
Pd@Pt-T790 NPs |
Produce O2 via Pt NPs |
Enhanced PDT and combined PAI and MRI |
Sun et al.191 |
Cu2O NPs |
Catalyze H2O2 to ˙OH |
Combined CDT and PAI |
Yang et al.193 |
Ce6@Arg-ADP NPs |
Produce NO via H2O2 |
NO therapy |
Zhu et al.33 |
Dental diseases |
Treatment and imaging |
TiO2/Pt tubular robots |
Generate bubbles and ˙OH |
Oral biofilm disruption |
Villa et al.196 |
Dex-IONP-GOx NPs |
Generate ˙OH |
Prevent dental caries |
Huang et al.13 |
CuO/BP nanosheets |
Conversion from H2O2 to O2 is related valence change of Cu |
Assessment of disease progression and treatment effectiveness |
Wang et al.197 |
Inflammations |
Treatment |
2D-TMD nanosheets |
Scavenge intracellular H2O2 |
Anti-inflammation |
Yim et al.32 |
|
Hollow microspheres |
Release drugs and CO2 gas by H2O2 |
Anti-inflammation |
Chung et al.200 |
Imaging |
TMSN@PM |
Reaction with H2O2 and degrades itself to release Mn2+ |
Switch of MRI signal |
Li et al.203 |
Au–Pd@Ag nanorods |
Variate absorption via H2O2 |
Therapeutic effect evaluation by PAI |
Ye et al.34 |
CUR-PVAX NPs |
Degrade peroxalate ester to generate CO2 bubbles |
Potential US agents of ischemic diseases |
Jung et al.204 |
Co complexes |
Utilizing the large change in chemical shift shown at 19F |
Therapeutic effect evaluation by MRI |
Yu et al.205 |
Cardiovascular diseases |
Treatment |
Hydrogels contained CAT |
Scavenge H2O2 |
MI repair |
Ding et al.209 |
nCe-decorated nanofibers |
Scavenge H2O2 |
Cardiac hypertrophy inhibition |
Jain et al.210 |
Fu-sBR nanoassemblies |
Scavenge H2O2 |
Thrombosis treatment |
Jung et al.211 |
Imaging |
BSA-Cy-Mito nanoprobes |
Produce PAI signal |
Atherosclerotic plaque vulnerability assessment and precise treatment |
Gao et al.212 |
Bone injury |
Treatment and imaging |
Pt/CeO2 nanozymes |
Multiple enzyme effects scavenge uric acid and H2O2 |
Treatment of MSU-induced acute gout |
Lin et al.230 |
PUDCA NPs |
Scavenge H2O2 and release UDCA |
The osteogenic differentiation of MSCs |
Arai et al.5 |
Fe3O4@GO/BMP2 |
Reduce H2O2 and capture ˙OH |
Bone tissue regeneration |
Zhang et al.232 |
hMNP/GelMA composite hydrogels |
Scavenge H2O2 and release Mn2+ for promoting osteogenesis |
Bone tissue regeneration |
Li et al.234 |
Neurologic diseases |
Treatment |
Ma@(MnO2 + FTY) NPs |
Convert H2O2 to O2 |
Protect damaged neurons |
Li et al.238 |
Imaging |
PCAB |
Detect H2O2 |
Therapeutic effect evaluation by FLI |
Wang et al.240 |
CRANAD-88 |
Amplifying NIR fluorescence signals |
Monitoring the changes of H2O2 concentrations before and after treatment |
Yang et al.241 |
Ophthalmic diseases |
Treatment |
CeNP-CL |
Scavenge H2O2 |
Treatment of ophthalmic diseases |
Choi et al.245 |
4.1 Enhanced tumor therapy effect by producing ROS and correlated imaging
As a major threat to human health, it has always been the goal of tumor nanomedicine to develop effective treatments.125 In this section, we will provide an overview of current researches on H2O2-involving tumor therapy and diagnosis.
4.1.1 Enhanced tumor therapy by enhancing 1O2 production and alleviating hypoxia. Photodynamic therapy (PDT), sonodynamic therapy (SDT), radiotherapy (RT), radiodynamic therapy (RDT) and immunotherapy have emerged as novel tumor treatment strategies as a result of the advancement of nanotechnology.64,126–129 The main mechanism of PDT, SDT, and RDT is the transfer of electrons or energies from sensitizers to adjacent substrates, under light, US, or X-ray irradiation, followed by the production of ROS.9 While the main mechanism of enhanced RT and immunotherapy is changing the microenvironment of RT and the immune by alleviating hypoxia. The concentration of O2 plays a key role in those therapies, which are positively correlated with the therapeutic effect. However, due to the rapid proliferation of tumor cells and insufficient blood supply, hypoxia becomes an important characteristic of tumors.130 If the oxygen tension falls below 10 mmHg, tissue is generally classified as hypoxic.131 Hypoxia-inducing factor 1 (HIF-1) is considered to be the main regulatory factor for cell adaptation to hypoxia, which is closely related to tumor progression and therapeutic effect.132 It can make cells and tissues produce a series of reactions to adapt to hypoxia environment, promote tumor angiogenesis, and increase the tumor's own invasiveness and resistance to treatments.4,26,29,133 Strategies for O2 generation have been reported include exogenous delivery of O2 to tumor region, or inhibition of mitochondrial respiration in tumor cells.9 However, these methods all encountered the problem of insufficient oxygen loading effect.9 At present, the decomposition of overexpressed H2O2 into O2 by nanomaterials become an important method to alleviate hypoxia.9
4.1.1.1 Enhanced photodynamic therapy. PDT mainly uses photosensitizer to generate energy level transition under the excitation of light and enter the excited state of higher energy level.134 Then, as the photosensitizer returns to its ground state, it needs to release a charge or energy to its surroundings. The substrate molecules around the photosensitizer gain this charge or energy to form ROS. PDT are divided into type I and type II by obtaining differences in charge or energy. PDT could be divided into type II (enhancing treatment by H2O2) and type I (direct treatment with H2O2) as shown in Fig. 5a.135 The mechanism of type II was that converted O2 from the decomposition of H2O2 into 1O2. For instance, Jia and coworkers synthesized a nanoplatform (Mn-CDs) to enhance PDT.7 Mn-CDs served as a CAT-like nanozyme to produce O2 by H2O2, then as a photosensitizer to convert O2 to 1O2. The amount of 1O2 produced was closely correlated with the concentration of O2. The mechanism of type I was that the excited photosensitizer under light irradiation could react with adjacent substrates by electron transfer to produce ROS.136 A GSH-responsive-release nanoplatform for H2O2-related type I PDT under 808 nm laser irradiation have been designed (Fig. 5b).27 The nanosheet could not only convert H2O2 to ˙OH under 808 nm laser irradiation for type I PDT, but also convert O2 to 1O2 under 660 nm laser irradiation for type II PDT. As shown in Fig. 5c and d, through DCFH-DA fluorescence in cells it was not difficult to find that the nanomaterial could produce ROS through type I and type II PDT under laser irradiation of different wavelengths. Moreover, cell viability in Fig. 5e and live/dead staining imaging in Fig. 5f showed that the killing ability of this nanomaterial against MCF-7 cells.
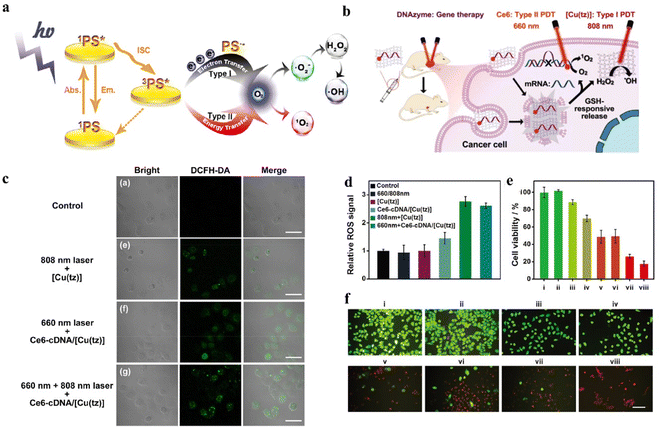 |
| Fig. 5 (a) Schematic of the difference between type I and type II. Reproduced from ref. 135 copyright 2021, Wiley-VCH GmbH. (b) Schematic of the nanoplatform to convert H2O2 to ˙OH for tumor treatment. Reproduced from ref. 27 copyright 2021, Wiley-VCH GmbH. (c) Confocal images of intracellular ROS generation. Reproduced from ref. 27 copyright 2021, Wiley-VCH GmbH. (d) ROS fluorescence signals generated during different treatments. Reproduced from ref. 27 copyright 2021, Wiley-VCH GmbH. (e) Cell viability of MCF-7 cells with different treatments. Reproduced from ref. 27 copyright 2021, Wiley-VCH GmbH. (f) Live/dead staining images of MCF-7 cells with different treatments. Reproduced from ref. 27 copyright 2021, Wiley-VCH GmbH. PBS (i), 660 and 808 nm laser irradiation (ii), Ce6-cDNA/[Cu(tz)] (iii), Ce6-DNAzyme/[Cu(tz)] (iv), Ce6-cDNA/[Cu(tz)] under 660 nm laser irradiation (v), [Cu(tz)] under 808 nm laser irradiation (vi), Ce6-DNAzyme/[Cu(tz)] under 660 nm laser irradiation (vii), and Ce6-DNAzyme/[Cu(tz)] under 660 and 808 nm laser irradiation (viii). | |
4.1.1.2 Enhanced sonodynamic therapy. SDT was also an excellent method of tumor therapies, which could generate ROS and possess maximum tissue-penetration depth.28,137 Thus, the increasing concentration of O2 could also enhance the effect of SDT. Inspired by the fact that H2O2 produced oxygen by CAT, Wu et al. designed a nanoplatform CSI@Ex-A for enhancing SDT (Fig. 6a).138 The generated O2 from the decomposition of H2O2 by CAT would be converted to 1O2 by indocyanine green (ICG) under US irradiation. According to the HIF-1ɑ fluorescence pattern analysis in Fig. 6b, it could be seen that CAT decomposed H2O2 to produce O2, which could degrade HIF-1a to enhance the efficacy of tumor therapy. As shown in Fig. 6c, the DCFH-DA fluorescence image showed that the nanomaterial had the ability of SDT to produce ROS, and CAT could enhance the SDT effect. Meanwhile, the use of ultrasonic excitation compensated for the lack of penetration of light. In addition to CAT, Mn-based nanomaterials could also serve as a H2O2-responsive nanozyme for SDT.139 For example, Zhu et al. designed a protoporphyrin-based nanoplatform that loaded into the hollow mesoporous organosilica nanoparticles (HMONs)-MnOx to generate O2 for enhancing SDT.140
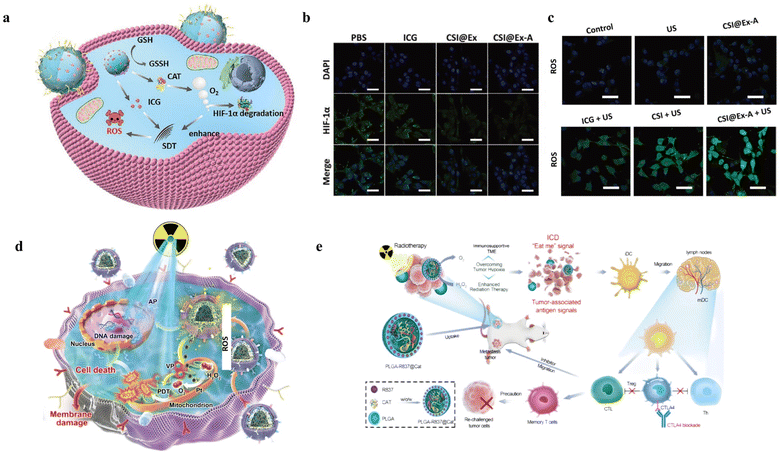 |
| Fig. 6 (a) Schematic illustration of CSI@Ex-A nanoplatforms based on CAT and ICG for SDT. Reproduced from ref. 138 copyright 2022, Wiley-VCH GmbH. (b) HIF-1α fluorescence signals generated during different treatments. Reproduced from ref. 138 copyright 2022, Wiley-VCH GmbH. (c) ROS fluorescence signals generated during different treatments. Reproduced from ref. 138 copyright 2022, Wiley-VCH GmbH. (d) Schematic illustration of the synthetic process of Pt and apoptin-based nanoplatform and its mechanism of alleviating hypoxia and enhancing RT. Reproduced from ref. 141 copyright 2022, Wiley-VCH GmbH. (e) Schematic of PLGA-R837@CAT NPs to trigger ICD and anti-CTLA4 checkpoint blockade. Reproduced from ref. 64 copyright 2019, WILEY-VCH Verlag GmbH & Co. KGaA, Weinheim. | |
4.1.1.3 Enhanced radiotherapy and radiodynamic therapy. After X-ray irradiation, oxygen molecules can inhibit DNA repair and HIF-1 expression enhancing the effect of Radiotherapy.129 Hypoxia can mitigate DNA damage as well as reduce the absorption of radiation, which makes the resistance of the tumor to RT.142 Hence, the rational design of nanomaterials for relieving hypoxia and enhancing tumor RT has been a hot area of research for several years. Zhou et al. reported a high-Z CeO2-based nanoplatform for decomposing H2O2 to O2 and enhancing RT.143 The nanoplatform exhibited CAT-mimic activity, induced DNA damage and inhibited tumor growth. Similar nanoplatforms based on Pt NPs as CAT-like nanozymes have been designed for enhancing RT (Fig. 6d).141 This nanoplatform possessed pH-responsive property, worked as radiosensitizer and had CAT-like activity to improve radiotherapy sensitivity. Compared with no oxygen supply, this strategy highly enhanced the effect of RT. Not only that, Sun et al. presented the RDT mechanism based on the X-ray excitation that transformed O2 to 1O2, combining the advantages of RT and PDT.144
4.1.1.4 Enhanced immunotherapy. For immunotherapy of cancer, alleviated hypoxia environment can also be used to enhance their effect.145 Some studies have been reported that the therapy effect could be weakened by hypoxic-mediated immune suppression.146 Therefore, alleviating hypoxia to enhance immunotherapy is a feasible tumor treatment strategy. Chen et al. designed poly PLGA-R837@CAT nanoparticles to produce O2 for increasing immunogenic cell death (ICD) and then combined with anti-cytotoxic T-lymphocyte-associated antigen 4 (CTLA4) checkpoint blockade (Fig. 6e).64 This nanoparticle was based on the core–shell PLGA nanoparticle to load CAT for synergistic oncotherapy. The PLGA-R837@CAT nanoparticle could destruct primary cancers under X-ray radiation and trigger the immunogenic cell death. This study was mainly based on oxygen relieving immunosuppressive tumor microenvironment. Moreover, hypoxia has been reported to facilitate immune-supportive M1-type tumor-associated macrophages (TAM) to immune-suppressive M2-type TAM, which was a disadvantage for immunotherapy.147 Therefore, the production of O2 by decomposed H2O2 had also attracted tremendous attention for immunotherapy.
4.1.2 Enhanced tumor therapy by ˙OH production. In addition to producing oxygen, H2O2 can be used to produce ˙OH for tumor treatment. Chemodynamic therapy (CDT) is an emerging, minimally invasive, and effective cancer treatment, which is based on the H2O2 induce ˙OH formation via the Fenton or Fenton-like reaction.10,148 Hydroxyl radical possesses efficient oxidative stress capacity for killing tumor cells.149,150 Many nanomaterials have been reported that could be used for CDT, such as Fe-based, Mn-based, and other-based materials.151–156 Fe-based materials have been widely used in the biological field because of their high biosafety.148 The production of ˙OH could be induced by the reaction between Fe2+ and H2O2 in acidic environments.157 Then, the ˙OH as a type of ROS could cause lipid peroxidation (LPO).10 Chen et al. designed unique amorphous iron nanoparticles (AFe NPs) which loaded with carbonic anhydrase IX inhibitor (CAI) for self-enhanced CDT based on the Fenton reaction (Fig. 7a).158 The CAI could inhibit the expression of carbonic anhydrase IX, caused acidic ions to accumulate in the cell. The accumulated acidic ions could lower pH in cells and had an enhanced effect on CDT (Fig. 7b). Meanwhile, researchers found that Mn2+ and Cu2+ also have Fenton-like reaction.92 Inspired by this, Lin et al. synthesized MnO2-coated mesoporous silica (MS) NPs to deplete GSH and produce ˙OH for CDT.159 As depicted in Fig. 7c, the MnO2 layer would release Mn2+, then the production of Mn2+ could react with H2O2 to generate ˙OH. As shown in Fig. 7d, the DCF fluorescence in tumor cells by MS@MnO2 NPs was higher than MnCl2 at the same Mn concentration. This proved that MS@MnO2 NPs could produce stronger oxidative stress effects in the process of CDT. Hence, Fenton or Fenton-like reaction-based CDT has shown the great potential for cancer treatment.
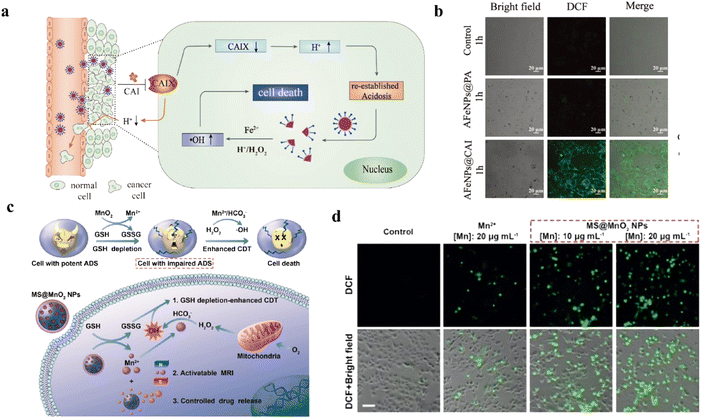 |
| Fig. 7 (a) Schematic illustration of AFe NPs for self-enhanced CDT. Reproduced from ref. 158 copyright 2019, WILEY-VCH Verlag GmbH & Co. KGaA, Weinheim. (b) The fluorescence images of DCFH-DA with different treatments. Reproduced from ref. 158 copyright 2019, WILEY-VCH Verlag GmbH & Co. KGaA, Weinheim. (c) Schematic illustration of MS@MnO2 NPs to deplete GSH and produce ˙OH for CDT. Reproduced from ref. 159 copyright 2018, WILEY-VCH Verlag GmbH & Co. KGaA, Weinheim. (d) The fluorescence images of DCFH-DA with different treatments. Reproduced from ref. 159 copyright 2018, WILEY-VCH Verlag GmbH & Co. KGaA, Weinheim. | |
In addition, H2O2-related synergistic therapy based on glucose oxidase (GOx) or GOx-like nanomaterials that exhibited excellent properties, which often combined with other treatment methods for enhancing tumor therapy effect.160 Chang et al. have designed a nanoplatform Cu2MoS4 for synergistic starvation therapy and CDT.21 Starvation treatment can continue to produce H2O2 to replace the H2O2 consumed by CDT. In other studies, H2O2-related gas therapy has also been reported.161 Therefore, H2O2-related therapy strategies play important roles in the research of tumor therapy.
4.1.3 Enhanced tumor therapy by H2O2-related imaging guidance. Currently, H2O2-related tumor imaging methods commonly used mainly include fluorescence imaging (FLI), magnetic resonance imaging (MRI), ultrasound imaging (USI), photoacoustic imaging (PAI), etc. Each of imaging strategies has its own advantage. For example, FLI is an emerging tumor molecular imaging method, which possesses the advantages of high spatial and temporal resolution.162 Overexpression of H2O2, a characteristic of TME, is widely believed to be involved in regulating the proliferation and malignant characteristics of cancer cells.3 Therefore, qualitative and quantitative research on H2O2 is conducive to understanding the progression of tumor development and judging the effect of related treatment. For instance, Yu et al. designed a lapachone-loaded H2O2-responsive nanoplatform to detect tumor by the H2O2-related chemiluminescence.163
4.1.3.1 H2O2-related fluorescence imaging of tumor. In the field of biosensing, FLI can be used to detect the disorder of H2O2 in tumor.164–170 The mechanism of H2O2-related FLI is mainly based on the fluorescence signal changes with the concentration of H2O2. For instance, Zhao et al. synthesized Cu ion-doped zeolitic imidazolate framework-8 (ZIF-8) loaded with AuNCs and DOX for FL imaging-guided therapy.171 When the pH decreased and H2O2 existed, DOX and Cu ions would be released, then fluorescence signals of DOX and AuNCs could be recovered. In other studies, a Janus nanoparticle Ag/Ag2S Janus NP has been designed for the diagnosis of disease and detection of H2O2 by H2O2-activated NIR-fluorescence imaging.172 Due to the plasmonic electron transfer, the Janus NP possessed the ability of fluorescence quenching. The H2O2 served as a key to turn on the fluorescence signals under NIR irradiation in Fig. 8a. As shown in Fig. 8b, compared with the group without H2O2, the group with H2O2 showed strong fluorescence signals. Meanwhile, the fluorescence intensity increased with the increase of the contact time between nanomaterials and H2O2. Undoubtedly, the further exploration of FLI in biomedical applications will be readily pushed forward by these new probes for H2O2.
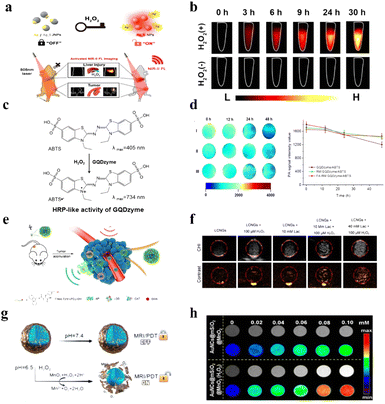 |
| Fig. 8 (a) Schematic of Ag/Ag2S Janus NPs for H2O2-activated NIR-fluorescence imaging. Reproduced from ref. 172 copyright 2021, American Chemical Society. (b) The change of fluorescence intensity under different conditions. Reproduced from ref. 172 copyright 2021, American Chemical Society. (c) Schematic illustration of ABTS for photoacoustic imaging. Reproduced from ref. 57 copyright 2019, American Chemical Society. (d) The change of PAI signal intensity. Reproduced from ref. 57 copyright 2019, American Chemical Society. (e) Schematic of nanoplatforms to continuously release oxygen bubbles for US imaging. Reproduced from ref. 180 copyright 2021, American Chemical Society. (f) The change of US intensity of in the tumor region. Reproduced from ref. 180 copyright 2021, American Chemical Society. (g) Schematic of AuNCs@mSiO2@MnO2 nanozymes for MRI. Reproduced from ref. 185 copyright 2021, American Chemical Society. (h) The change of MRI intensity of AuNCs@mSiO2@MnO2 nanozymes. Reproduced from ref. 185 copyright 2021, American Chemical Society. | |
4.1.3.2 H2O2-related photoacoustic imaging of tumor. Photoacoustic imaging (PAI) is also a promising diagnostic strategy that combines the advantages of traditional optical imaging and ultrasound imaging.173 Briefly, a material undergoes thermoelastic expansion that is based on photothermal conversion under light irradiation, which is then converted to a US wave.174 Some H2O2-responsive sensitizers possessed excellent properties for PA imaging, such as ABTS.42 Ding et al. designed a nanoplatform to load ABTS for PAI, which was based on the strong NIR absorbance of ABTS.57 The mechanism was based on the HRP-catalyzed colorless ABTS substrate into its green oxidized form in the presence of H2O2 (Fig. 8c and d). According to the change of PA signals, the fluctuation in H2O2 concentration could be analyzed. Hence, PA imaging can also become an important method for disease diagnosis and H2O2 detection in the biomedical application.
4.1.3.3 H2O2-related ultrasound imaging of tumor. Ultrasound imaging is an important tool in biomedical imaging fields due to its high resolution, non-invasive method, and real-time modality.175,176 Recently, many researches have reported to focus on contrast agents based on nanomaterials to produce gas bubbles in the tumor regions.177,178 The generating bubbles could effectively reflect ultrasound signals, which were used for USI.179 Wu et al. designed a nanosystem, which incorporated CAT and lactate oxidase (LOX), for enhancing US signals.180 As shown in Fig. 8e, this nanoplatform could continuously release oxygen bubbles for USI by cascade catalysis of LOX and CAT. The continuously releasing oxygen bubbles could accumulate in the tumor region, which enhanced the specificity and continuity of USI (Fig. 8f). Thus, the generation of O2 and the alleviation of hypoxia could be monitored. Besides, owing to its strong acoustic impedance, H2O2-induced CO bubbles could also serve as a contrast agent for USI.181
4.1.3.4 H2O2-related magnetic resonance imaging of tumor. Magnetic resonance imaging, a commonly used diagnostic tool, has the characteristics of high resolution, security, signal-to-noise ratio, and so on.182 MnO2 has been found that it could decompose H2O2 into water and oxygen, and release Mn2+ ions by itself.183 The non-lanthanide metal ion Mn2+ could short the longitudinal relaxation time (T1) and transverse relaxation time (T2) of water protons, thus enhancing T1-magnetic resonance imaging contrast and weakening T2-magnetic resonance imaging contrast.184 As shown in Fig. 8g, Yin and coworkers used this feature to design a H2O2-responsive nanozyme (AuNCs@mSiO2@MnO2) for enhancing MR signals.185 Herein, according to the change of MR signals, the concentration of H2O2 could be analyzed in Fig. 8h.
4.1.3.5 H2O2-related multimodal imaging of tumor. H2O2-related multimodal imaging can combine the advantages of different imaging modalities.186 For instance, Wang et al. designed a new type of phthalocyanine manganese (MnPcE4) photosensitizer to achieve trimodal imaging (in vivo FL/CT/MRI).187 Fluorescence imaging showed that the nanomaterial was able to rapidly accumulate in the tumor area. Furthermore, in the presence of H2O2, the Mn2+ could release as well as be used for MR imaging. The complementary advantages of different imaging models greatly improved the application of H2O2-related imaging.
4.2 Bacterial infections therapy by producing ROS and correlated imaging
It has been demonstrated that H2O2 is also overexpressed in the microenvironment of bacterial infection regions.8 Infections and unsuccessful wound healing caused by bacteria are among the serious health problems.188 Utilizing H2O2 for producing ROS is an excellent antibacterial therapy.31 This is because ROS destroy the bacterial membrane and damaged DNA, leading to bacterial death.189
As with tumor treatment, H2O2-related antibacterial therapy mainly includes PDT, SDT, and CDT.190–192 As described in Fig. 9a, Hu et al. designed a phenylboronic acid-based nanozyme for antibacterial PDT.8 Sun et al. synthesized a Pd@Pt-T790 therapeutic nanoplatform with organic sonosensitizer meso-tetra(4-carboxyphenyl)porphine (T790) for bacterial therapy (Fig. 9b).191 The nanoplatform catalyzed H2O2 to produce O2 and converted it into ROS under US irradiation. As shown in Fig. 9c, the results of colony counting showed that Pd@Pt-T790 nanoplatforms had good antibacterial effect of SDT. Moreover, H2O2 could be also used to directly generate ˙OH for antibacterial therapy.192 For example, Yang et al. designed Cu2O nanoparticles, which catalyzed H2O2 to ˙OH by a Fenton-like reaction (Fig. 9d).193 The oxidative stress caused by ˙OH could induce bacterial death. Then, Cu2O nanoparticles could be transformed to Cu9S8 NPs in bacterial environment, which as photoacoustic agents accurately distinguished between bacterial infections and normal tissues (Fig. 9e). After injection of Cu2O NPs, photoacoustic signal in normal tissue did not change significantly. However, Cu2O NPs could be vulcanized to form Cu9S8 NPs in the infected tissue, the PA signal intensity reached the maximum after in situ injection at 12 h.
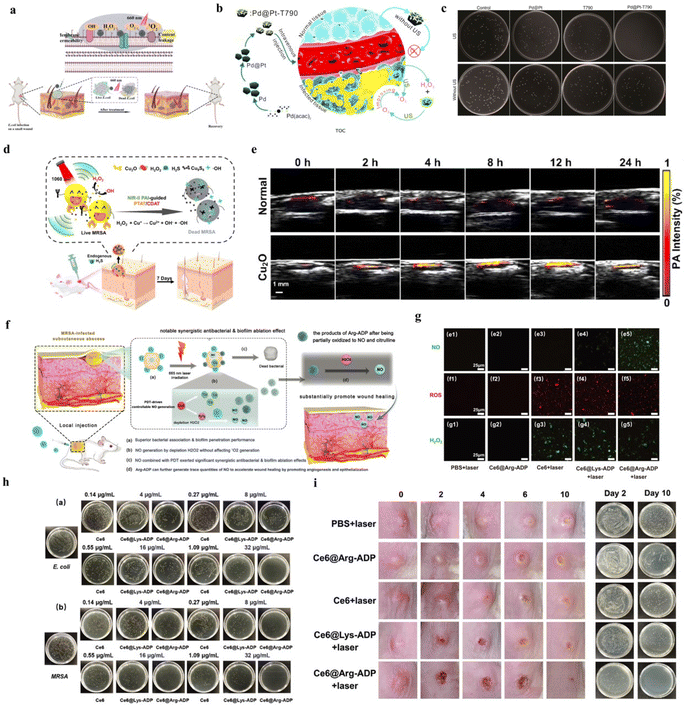 |
| Fig. 9 (a) Schematic illustration of a phenylboronic acid-based nanozyme for synergistic antibacterial therapy. Reproduced from ref. 8 copyright 2021, Elsevier B.V. (b) Schematic illustration of a Pd@Pt-T790 nanotherapeutic platform for SDT. Reproduced from ref. 191 copyright 2020, American Chemical Society. (c) MRSA colony pictures after different treatments. Reproduced from ref. 191 copyright 2020, American Chemical Society. (d) Schematic illustration of Cu2O nanoparticles for PA imaging-guided therapy. Reproduced from ref. 193 copyright 2021, Elsevier Ltd. (e) The PAI images of Cu2O NPs in vivo at different time. Reproduced from ref. 193 copyright 2021, Elsevier Ltd. (f) Schematic illustration of Ce6@Arg-ADP for antibacterial therapy. Reproduced from ref. 33 copyright 2021, Wiley-VCH GmbH. (g) CLSM images of NO, ROS, and H2O2 generation in MRSA after different treatments. Reproduced from ref. 33 copyright 2021, Wiley-VCH GmbH. (h) Representative images of plate samples of E. coli and MRSA after different treatments. Reproduced from ref. 33 copyright 2021, Wiley-VCH GmbH. (i) Wound healing and colony change in vivo. Reproduced from ref. 33 copyright 2021, Wiley-VCH GmbH. | |
Similar to ˙OH, nitric oxide (NO) as a broad-spectrum antibacterial molecule could also be used for antibacterial therapy.194 In the presence of H2O2, L-arginine could be oxidized to produce NO.194 Zhu et al. designed a nanoplatform Ce6@Arg-ADP, which had rich L-arginine, to deal with bacteria.33 The PDT could control generation of NO in situ and substantially promoted wound healing (Fig. 9f). As shown in Fig. 9g, there was almost no NO produced in PBS + laser-, Ce6@Arg-ADP-, Ce6 + laser-, Ce6@Lys-ADP + laser-treated bacteria, but large amount of NO was observed in Ce6@Arg-ADP + laser-treated bacteria. As displayed in Fig. 9h, at all tested concentrations, the antibacterial effect of Ce6@Arg-ADP + laser was significantly higher than that of Ce6 + laser and Ce6@Lys-ADP + laser. In the treatment of abscess, it was necessary to control infection, promote the rapid healing of disfigured wound, shorten the time of wound care, and avoid secondary infection. The result in Fig. 9i, Ce6@Arg-ADP + laser could inhibit bacterial infection and promote wound healing. Consequently, H2O2-based antibacterial treatment and imaging strategies provide an excellent method for further biomedical application.
4.3 Dental diseases therapy by producing ROS and correlated diagnosis
Dental microbial biofilms, which can embed microorganisms, are the causative factor of dental diseases, such as dental caries and periodontitis.195,196 Because the entire biofilm is protected by a homegrown organic polymer matrix, it is more resistant to treatment than free bacteria.196 Previously, ˙OH generation from H2O2 could disrupt dental microbial biofilms were reported.196 Therefore, H2O2-related dental disease treatment and diagnosis receive more attention. For instance, Villa et al. synthesized self-propelled tubular microrobots to disrupt biofilms by the generation of bubbles and ˙OH (Fig. 10a).196 As shown in Fig. 10b, the motion mechanism was the decomposition of H2O2 into O2 catalyzed by Pt NPs on the inner surface. Then, this caused O2 exit from one end of the tube, which triggers its motion in the opposite direction. At the same time, it was not difficult to find from Fig. 10c that microrobots motion velocity was increased with the increase of H2O2 concentration. Thus, the self-propelled tubular microrobot could destroy biofilms in the presence of H2O2. Huang et al. designed Dex-IONP-GOx nanoplatforms to generate ˙OH from H2O2 for disrupting biofilms.13 As shown in Fig. 10d, this nanoplatform had cascade catalysis activity to continuously produce H2O2 and ˙OH for the prevention of dental caries. Furthermore, this study found that the Dex-IONP-GOx nanoplatform had specific binding to S. mutans, which has destructive effect on biofilm (Fig. 10e). Therapeutic effect evaluation of dental disease has also been developed recently.197 Wang et al. designed copper oxide nanoparticles anchoring onto black phosphorus nanosheets for assessment of disease progression and treatment effectiveness.197 This nanoplatform possessed superior selectivity, it could utilize human gingival crevicular fluid and saliva samples for the diagnosis of periodontitis.
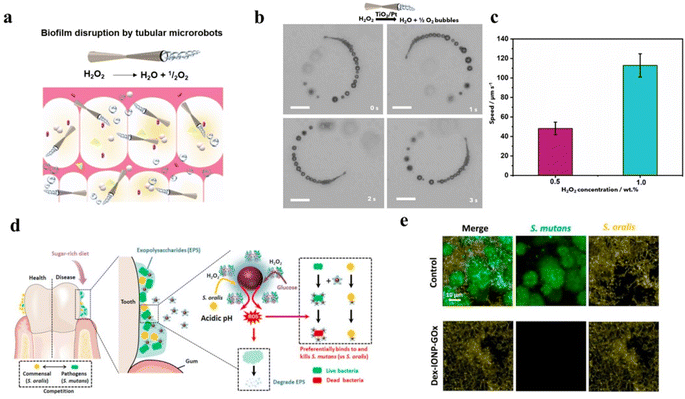 |
| Fig. 10 (a) Schematic illustration of self-propelled tubular microrobots to disrupt biofilms. Reproduced from ref. 196 copyright 2020, Elsevier. (b) Snapshot images of TiO2/Pt microrobots moving in different solution. Reproduced from ref. 196 copyright 2020, Elsevier. (c) Speed of the TiO2/Pt microrobots at different concentrations of H2O2. Reproduced from ref. 196 copyright 2020, Elsevier. (d) Schematic illustration of Dex-IONP-GOx nanoplatforms for prevention of dental caries. Reproduced from ref. 13 copyright 2021, Elsevier Ltd. (e) Fluorescence images of survival of different bacteria. Reproduced from ref. 13 copyright 2021, Elsevier Ltd. | |
4.4 Enhanced therapy and imaging by direct consumption of H2O2
4.4.1 Inflammations therapy and correlated imaging. Inflammation plays a pathological basis role in multiple chronic diseases.34 Previous studies have shown that H2O2 can be produced in inflammation areas and kill infiltrating pathogens.34 However, oxidative stress caused by excess H2O2 will exacerbate the inflammatory response and impede the repair of tissues and organs.198 Oxidative stress of H2O2 damages endothelial barrier, promotes migration and infiltration of inflammatory cells, and aggravates tissue damage.199 Therefore, the reduction of H2O2 is one of the effective ways for anti-inflammatory treatment. For example, Yim et al. designed two-dimensional (2D) transition-metal dichalcogenides (2D-TMD), which could scavenge intracellular H2O2 for inflammatory treatment (Fig. 11a).32 As described in Fig. 11b, the nanomaterial had a good ability to clear ROS and reactive nitrogen species (RNS), which could alleviate the inflammatory response to a large extent. Additionally, a H2O2-responsive nanoplatforms which released drugs and CO2 gas treatment for inflammation inhibition have been reported.200
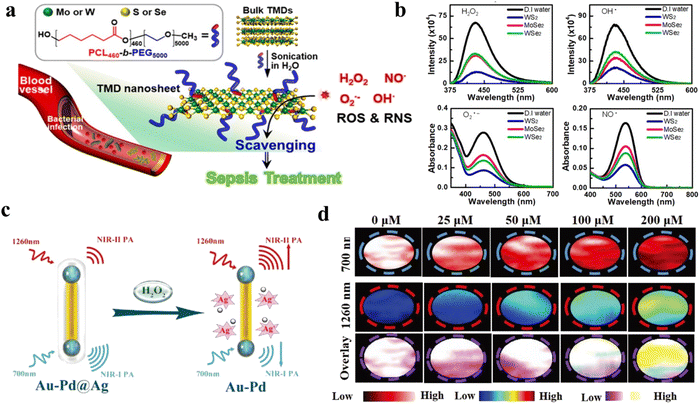 |
| Fig. 11 (a) Schematic illustration of two-dimensional transition-metal dichalcogenides for inflammatory treatment. Reproduced from ref. 32 copyright 2020, American Chemical Society. (b) The clearing of ROS and RNS by 2D-TMD. Reproduced from ref. 32 copyright 2020, American Chemical Society. (c) Schematic illustration of Au–Pd@Ag nanorods for detection of H2O2 by PA imaging. Reproduced from ref. 34 copyright 2020, WILEY-VCH Verlag GmbH & Co. KGaA, Weinheim. (d) PA intensity of 700 nm and 1260 nm at different hydrogen peroxide concentrations. Reproduced from ref. 34 copyright 2020, WILEY-VCH Verlag GmbH & Co. KGaA, Weinheim. | |
More than that, imaging can be used to assess changes in H2O2 concentration in the inflamed area, enabling monitoring of therapeutic effects and inflammatory progression.201,202 Li et al. designed a novel nanotheranostic agent (TMSN@PM) to scavenge the excess ROS for alleviating inflammation and release Mn2+ ions.203 The number of Mn2+ ions released was correlated with the ROS level, which could be monitored by MRI. Moreover, Ye et al. designed a H2O2-responsive nanomaterial (Au–Pd@Ag nanorod) to detect H2O2 by PAI (Fig. 11c).34 The PAI signal at 1260 nm and 700 nm could be used accurately differentiate the inflammation region and normal tissue in Fig. 11d. In another study, a nanoparticle that could be responsive to H2O2 to produce CO2 bubbles for USI has been developed.204 And a Co complex has been designed for detection of H2O2 production by MRI.205 Utilizing the large change in chemical shift shown at 19F when oxidizing from Co(II) to Co(III), this was done using a chemical shift selective pulse train. Furthermore, H2O2-responsive nanomaterials have also been shown to be useful in the treatment and diagnosis of other inflammatory diseases, including inflammation of musculoskeletal systems, interstitial cystitis, ulcerative colitis, lupus nephritis, etc.176,206–208 In a word, H2O2-responsive nanomaterials offer an alternative strategy for inflammation therapy and diagnosis.
4.4.2 Cardiovascular diseases therapy and correlated imaging. In the cardiovascular system, H2O2-responsive nanomaterials can be used for the treatment and imaging of many diseases, such as thrombosis.213 Myocardial infarction (MI), a cardiac disease, has a high mortality rate in the world.214 A hypoxic microenvironment in MI tissues will induce aberrant production of H2O2.215 Unfortunately, excessive H2O2 will lead to myocardial damage, which is adverse to cardiac repair after MI.209 Therefore, scavenging of H2O2 and production of O2 means a potential way for MI repair. Ding et al. synthesized a hydrogel, that contained CAT, to turn H2O2 into O2.209 As depicted in Fig. 12a, this hydrogel possessed excellent properties to scavenge H2O2 for MI repair. Experimental results showed that removing H2O2 was beneficial to MI repair. Moreover, cardiac hypertrophy is also associated with H2O2, which is caused by MI, leading to cardiac failure.216,217 Inspired by this, a nanofiber has been developed to scavenge H2O2 for cardiac hypertrophy inhibition as shown in Fig. 12b.210 In addition, H2O2-related nanotechnology could also be used for early cardiac disease diagnosis, such as early acute myocardial infarction.218
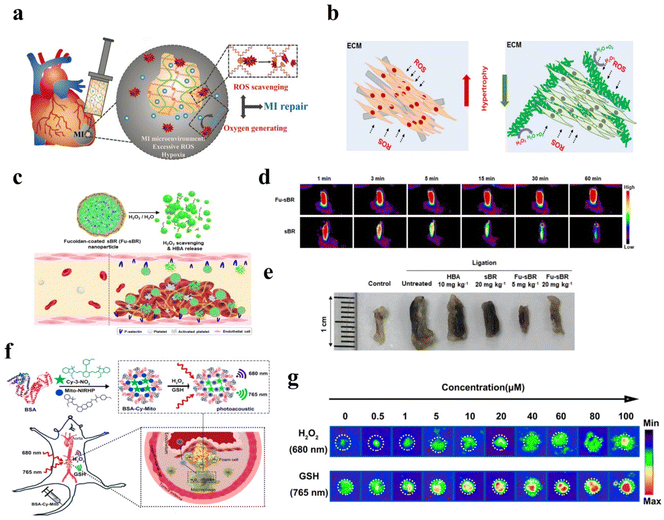 |
| Fig. 12 (a) Schematic illustration of hydrogel for H2O2-scavenging and O2 generating. Reproduced from ref. 209 copyright 2020, Wiley-VCH GmbH. (b) Schematic illustration of nanofiber for mitigating H2O2 generated after myocardial infarction. Reproduced from ref. 210 copyright 2020, Elsevier B.V. (c) Schematic illustration of Fu-sBR nanoassemblies for antithrombotic treatment. Reproduced from ref. 211 copyright 2021, Wiley-VCH GmbH. (d) Fluorescence images of thrombosed IVC excised at 60 min after injection. Reproduced from ref. 211 copyright 2021, Wiley-VCH GmbH. (e) Photographs of thrombosed IVC excised at 48 h after the release of ligation. Reproduced from ref. 211 copyright 2021, Wiley-VCH GmbH. (f) Schematic illustration of nanoprobe to detect H2O2 for atherosclerotic plaque vulnerability assessment. Reproduced from ref. 212 copyright 2019, American Chemical Society. (g):PA imaging of BSA-Cy-Mito nanoprobe with different concentrations of H2O2 and GSH. Reproduced from ref. 212 copyright 2019, American Chemical Society. | |
Similarly, H2O2-related nanotechnology has been widely used in vascular systems, such as thrombus and angiogenesis.219,220 It has been reported that the pathophysiological process of thrombus is associated with H2O2.221,222 The generation of H2O2 was associated with activated platelets and injured endothelium.223 Platelet-dependent thrombus formation could be progressed by high levels of H2O2.224 Herein, the removal of overproduced H2O2 was an effective method for thrombosis treatment. Jung et al. synthesized H2O2-responsive Fu-sBR nano assemblies to scavenge H2O2 (Fig. 12c).211 This nanomaterial possessed the ability for targeted on-demand treatment of thrombus. As shown in Fig. 12d and e, due to the H2O2-responsive effect of Fu-sBR, there was no attenuation of the fluorescence signal within 60 min. However, sBR was not H2O2-responsive effect, and the fluorescence signal continued to decay. Photographs of thrombosed excised at 48 h, Fu-sBR had a good effect on the treatment of thrombus. In another study, Gao et al. designed a nanoprobe to detect H2O2 for atherosclerotic plaque vulnerability assessment by PAI and precise treatment in Fig. 12f.212 As described in Fig. 12g, the PAI signal of nanoprobe increased with the increase of H2O2 concentration. Furthermore, the H2O2-responsive nanoplatform has been proven to release drugs in the blood vessels.223,225–227 Therefore, these H2O2-related nanoplatforms offered excellent opportunities for future cardiovascular disease applications.
4.4.3 Bone injury therapy and correlated imaging. High ROS levels, a character of bone tissue injury, can promote osteoclast generation.228 H2O2, as a representative type of ROS, can induce oxidative stress, which is detrimental to bone injury repair.229 Therefore, nanotechnology to remove H2O2 provides a potential method for treatment of bone injury.Lin et al. synthesized a Pt/CeO2 nanozyme for the treatment of monosodium urate (MSU)-induced acute gout.230 As shown in Fig. 13a, nanosized Pt as a uricase and CAT mimic could scavenge uric acid and H2O2. The Pt/CeO2 nanozyme catalyzed uric acid and O2 to H2O2 and water-soluble allantoin, which reduced the production of the tophus. Then, the CAT mimic could provide a constant supply of oxygen, which creates a cycle of uric acid removal. Thus, the nanozyme alleviated joint pain and tissue injury. According to previous researches, peroxalate ester linkage was reported that it could also to react with H2O2.231 Inspired by this, peroxalate ester-based PUDCA NPs have been synthesized to scavenge H2O2 and release ursodeoxycholic acid (UDCA) for promoting the osteogenic differentiation of mesenchymal stem cells (MSCs).5 As described in Fig. 13b, UDCA could be released in the presence of H2O2, followed by it scavenged H2O2 for bone tissue regeneration. This research also proved that reducing levels of H2O2 could decrease the adipogenic differentiation of MSCs, and then reduce the inhibition of bone regeneration. By micro-computed tomography analysis, PUDCA NP-treated group had the best effect on bone injury repair in Fig. 13c. In a similar study, Zhang et al. focused on improving the activity of MSCs and osteogenic differentiation.232 Fe3O4@GO/BMP2 magnetic nanocomposites could reduce H2O2 by Fenton reaction and capture ˙OH for bone tissue regeneration under magnetic regulation.
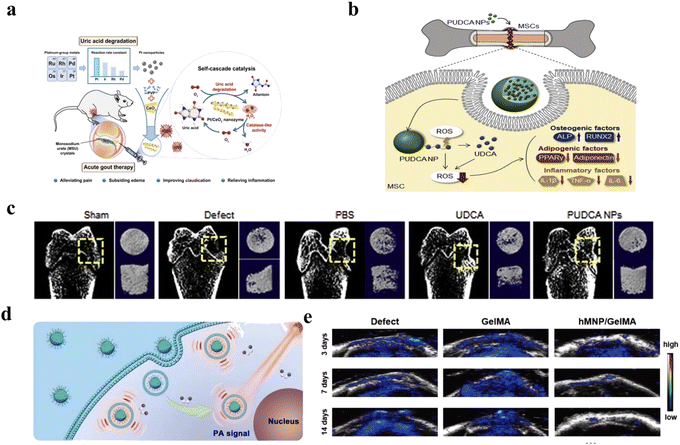 |
| Fig. 13 (a) Schematic illustration of synthesis and nanozyme activities of Pt/CeO2 for treatment of acute gout. Reproduced from ref. 230 copyright 2022, American Chemical Society. (b) Schematic illustration of PUDCA NPs for bone tissue regeneration. Reproduced from ref. 5 copyright 2020, Elsevier B.V. (c) CT images after different treatments. Reproduced from ref. 5 copyright 2020, Elsevier B.V. (d) Schematic illustration of Lipo@HRP&ABTS nanoprobes for PAI. Reproduced from ref. 234 copyright 2021, Wiley-VCH GmbH. (e) The PA signal of Lipo@HRP&ABTS nanoprobes to detect H2O2. Reproduced from ref. 234 copyright 2021, Wiley-VCH GmbH. | |
More than that, H2O2-responsive nanomaterials have been proven to promote osteogenesis.233 Because of this, Li et al. designed hollow manganese dioxide nanoparticles (hMNPs)/gelatin methacryloyl (GelMA) composite hydrogels to clear H2O2 and release Mn2+ for promoting bone regeneration.234 MnO2 NPs released Mn2+ in response to H2O2, and then Mn2+ promoted cell growth and osteogenic differentiation. In order to further study the concentration of H2O2 in the bone injury area, Lipo@HRP&ABTS nanoprobe was introduced in this experiment (Fig. 13d). In the presence of H2O2, Lipo@HRP&ABTS nanoprobe had strong NIR absorption, which could be converted into PA signal, realizing real-time imaging monitoring of H2O2 (Fig. 13e). In a word, H2O2-related nanoparticles had marvelous prospects in the treatment and diagnosis of bone injury.
4.4.4 Neurologic diseases therapy and correlated imaging. H2O2-induced cellular oxidation was not conducive to neurologic injury repair caused by diseases, such as neuroinflammation, traumatic brain injury, Parkinson's disease, and ischemic stroke.30,36,235,236 The production of H2O2 was related to reperfusion after ischemia stroke, and the oxidative stress generated by H2O2 would further aggravate the severity of ischemia stroke.237 According to previous researches, scavenging excessive H2O2 could effectively retard the progression of neuronal death caused by an ischemic stroke.237 Inspired by this, Li et al. designed a macrophage-disguised fingolimod (FTY)-loaded MnO2 nanoparticles (Ma@(MnO2 + FTY)) nanoparticle to convert H2O2 to O2 in the ischemic brain.238 As depicted in Fig. 14a, the nanoparticle could polarity M1 type microglia (proinflammatory type) to M2 type (anti-inflammatory), then cooperated with decomposing H2O2 and generating O2 to protect damaged neurons. As described in Fig. 14b and c, Ma@(MnO2 + FTY) NPs could remove H2O2 while producing O2, which was important for the protection of nerve cells. And oxygen-glucose deprivation/reoxidation (OGD/R) mimicked neuronal ischemia-reperfusion, demonstrating that ROS could be removed as a major source of nerve damage (Fig. 14d). Subsequently, [(Ru(dpp)3)]Cl2 probe was used to study the intracellular O2 concentration, which proved that Ma@(MnO2 + FTY) NPs could also produce a large amount of O2 in the cell (Fig. 14e). Immunofluorescence results showed that OGD/R increased the expression of M1 marker CD16/32, and FTY and Ma@MnO2 nanoparticles could decrease the expression of CD16/32 and increase the expression of M2 marker CD206 (Fig. 14f). Because it was a significant molecule in neurologic injury repair, change in H2O2 levels was closely related to the progression of diseases.239 Wang et al. designed a nanoprobe based on a PCAB for detecting H2O2 in vivo.240 A fluorescent imaging probe CRANAD-88 has been created to investigate H2O2 in Alzheimer's disease (AD) brains.241 Qiao et al. have also done some research on H2O2-responsive nanomaterials for the treatment of AD.242 Therefore, H2O2-related treatment and diagnosis have shown potential methods for neurologic diseases.
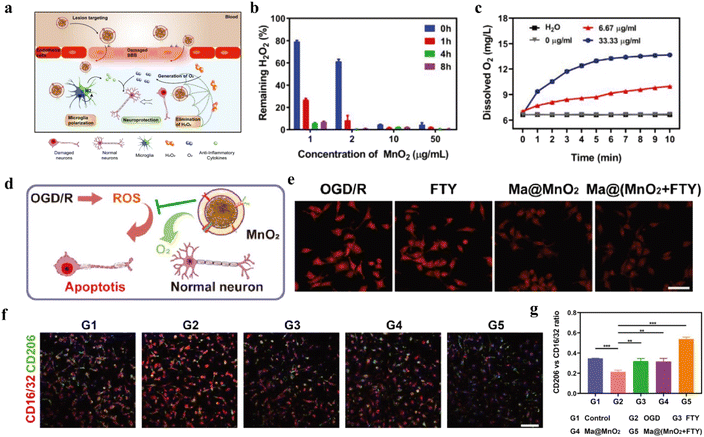 |
| Fig. 14 (a) Schematic illustration of Ma@(MnO2 + FTY) nanoparticles to protect damaged neurons. (b) The concentration of H2O2 with different concentration of MnO2. (c) The concentration of O2 with different concentration of MnO2. (d) Scheme of the mechanism of neuroprotection by Ma@MnO2 nanospheres on OGD/R. (e) Fluorescence images of intracellular oxygen concentration after different treatments. (f) Immunostained images of microglia with CD16/32 and CD206. (g) Quantification of the relative fluorescence intensity of CD206 versus CD16/32. (a–g) Reproduced under terms of the CC-BY license.238 Copyright 2021, C. Li et al., Published by Wiley-VCH GmbH. | |
4.4.5 Ophthalmic diseases therapy. It has been proved that some ophthalmic diseases were related to the increasing levels of H2O2.243 In ocular diseases, the overexpress production of H2O2 was based the prooxidative/antioxidative cellular imbalance.243 The overexpress production of H2O2 could disrupt retinal homeostasis, which is one of the cause of uveitis.35 Not only that, the human eye is more susceptible to oxidative damage, especially to the cornea, because of its exposure to environment.244 As the ocular surface composition, antioxidative damage of cornea is an essential content for the treatment of ophthalmic diseases.244 Therefore, Choi and coworkers designed a ROS-scavenging ceria nanoparticle-embedded contact lens (CeNP-CLs) for scavenging the ROS on the cornea.245 As reflected in Fig. 15a, CeNP-CLs possessed CAT-like ability and SOD-mimetic activities, which could remove H2O2 and other ROS. With the increase of Ce concentration, the concentration of H2O2 continued to decrease (Fig. 15b). Corneal endothelial damage caused by H2O2 infiltration will lead to corneal opacity. As described in Fig. 15c, obvious corneal opacity could be found in the group with 0 mM CeNP-CLs after 3 days of H2O2 exposure, compared with before H2O2 exposure. According to the tissue sections, the degree of corneal epithelial injury in the group wearing 0 mM CeNP-CLs (Fig. 15d) was greater than that in the group wearing 50 mM CeNP-CLs (Fig. 15e). These findings suggested that adequate clearance of H2O2 in the presence of CeNPs-CLs can reduce damage to corneal epithelium and endothelial cells. To sum up, H2O2-related nanoparticles have marvelous prospects and potential in the treatment and diagnosis of many diseases.
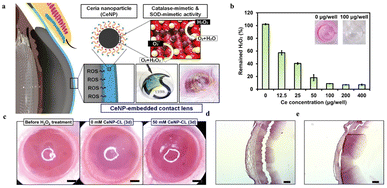 |
| Fig. 15 (a) Schematic illustration of CeNP-CL for scavenging the ROS on the cornea (b) changes of H2O2 at different Ce concentrations. (c) Representative photographs of the ocular surface for different groups. (d) Histologic photographs for the group wearing 0 mM CeNP-CLs. (e) Histologic photographs for the group wearing 50 mM CeNP-CLs. Reproduced from ref. 245 copyright 2020, American Chemical Society. | |
5 Conclusion and perspective
H2O2-responsive nanomaterials with their applications have been proven as interesting and meaningful methods for disease diagnosis and therapy. On the one hand, under external light or US excitation, the O2 generated from the decomposition of H2O2 by the H2O2-responsive nanomaterials is converted into ROS for disease treatment. Hypoxia could also be alleviated by the generation of O2, which enhanced the effectiveness of therapy. On the other hand, H2O2-responsive nanomaterials can catalyze H2O2 into ˙OH by Fenton or Fenton-like reaction. Furthermore, the combination of multiple treatments has also become a hot research topic. Treatment is often used in conjunction with other treatment modalities for increasing the effect, which possessed a “1 + 1 > 2” treatment effect. More importantly, imaging-guided therapy has been reported to could monitor the effect of H2O2-related treatments in real-time. This is mainly based on the fact that nanomaterials can react with H2O2 and enhance imaging signal. In this review, we summarized recent advances in H2O2-responsive nanomaterials and their applications in treatment and diagnosis of multiple types of diseases, including tumors, bacterium, dental diseases, inflammations, cardiovascular diseases, bones, neurologic diseases and ophthalmic diseases. According to the mechanism of therapy, they can be divided into two different groups: (1) producing ROS and (2) consuming H2O2.
Despite some advantages of H2O2-responsive nanomaterials and their related diagnosis and treatment approaches, many challenges are still needed to be addressed. (1) Most H2O2-responsive sensitizers or nanomaterials possess potential toxicity; (2) the synthesis process of H2O2-responsive nanomaterials is tedious and complex; (3) the researches on H2O2-related nontumor diseases therapy are not in-depth and comprehensive enough; (4) the effectiveness of some nanoplatforms still needs further improvement.
As a novel and versatile theranostic modality, H2O2-responsive nanomaterials have shown their high specificity and therapeutic performances in various biomedical applications, opening up new avenues for the treatment and diagnosis of H2O2 overexpression diseases. Provided the above challenges are adequately addressed, this unique H2O2-responsive nanomaterial is expected to enter the clinical stage in the near future to bring benefit to personalized biomedicine. Therefore, we hope that this review can contribute to providing new design inspirations and application ideas for various functional nanomaterials related to H2O2.
Conflicts of interest
There are no conflicts to declare.
Acknowledgements
We gratefully acknowledge support from the National Natural Science Foundation of China (No. 82102056) and the Natural Science Foundation of Fujian Province of China (No. 2022J01280).
Notes and references
- A. Adrian, K. Schoppmann, J. Sromicki, S. Brungs, M. von der Wiesche, B. Hock, W. Kolanus, R. Hemmersbach and O. Ullrich, Cell Commun. Signal., 2013, 11, 98 CrossRef PubMed
. - H. Sies and D. P. Jones, Nat. Rev. Mol. Cell Biol., 2020, 21, 363–383 CrossRef CAS PubMed
. - P. Wang, W. Yang, S. Shen, C. Wu, L. Wen, Q. Cheng, B. Zhang and X. Wang, ACS Nano, 2019, 13, 11168–11180 CrossRef CAS PubMed
. - L. Meng, Y. Cheng, X. Tong, S. Gan, Y. Ding, Y. Zhang, C. Wang, L. Xu, Y. Zhu, J. Wu, Y. Hu and A. Yuan, ACS Nano, 2018, 12, 8308–8322 CrossRef CAS PubMed
. - Y. Arai, H. Park, S. Park, D. Kim, I. Baek, L. Jeong, B. J. Kim, K. Park, D. Lee and S. H. Lee, J. Control Release., 2020, 328, 596–607 CrossRef CAS PubMed
. - Q. Zeng, R. Zhang, T. Zhang and D. Xing, Biomaterials, 2019, 207, 39–48 CrossRef CAS PubMed
. - Q. Jia, J. Ge, W. Liu, X. Zheng, S. Chen, Y. Wen, H. Zhang and P. Wang, Adv. Mater., 2018, 30, e1706090 CrossRef PubMed
. - Y. Hu, W. Wang, S. Huang, J. Li, Y. Zhang, Y. Gao, Y. Cheng, Z. Wu and X. Zhang, Chem. Eng. J., 2022, 431, 133704 CrossRef CAS
. - S. Wang, R. Tian, X. Zhang, G. Cheng, P. Yu, J. Chang and X. Chen, Adv. Mater., 2021, 33, e2007488 CrossRef PubMed
. - H. Ranji-Burachaloo, P. A. Gurr, D. E. Dunstan and G. G. Qiao, ACS Nano, 2018, 12, 11819–11837 CrossRef CAS PubMed
. - M. Lopez-Lazaro, Cancer Lett., 2007, 252, 1–8 CrossRef CAS PubMed
. - X. Hou, H. Zeng, X. Chi and X. Hu, Nano Lett., 2021, 21, 9966–9975 CrossRef CAS PubMed
. - Y. Huang, Y. Liu, S. Shah, D. Kim, A. Simon-Soro, T. Ito, M. Hajfathalian, Y. Li, J. C. Hsu, L. M. Nieves, F. Alawi, P. C. Naha, D. P. Cormode and H. Koo, Biomaterials, 2021, 268, 120581 CrossRef CAS PubMed
. - P. Niu, J. Liu, F. Xu, L. Yang, Y. Li, A. Sun, L. Wei, X. Liu and X. Song, ACS Appl. Bio Mater., 2022, 5, 1683–1691 CrossRef CAS PubMed
. - K. Zamojc, M. Zdrowowicz, D. Jacewicz, D. Wyrzykowski and L. Chmurzynski, Crit. Rev. Anal. Chem., 2016, 46, 171–200 CrossRef CAS PubMed
. - L. H. Fu, C. Qi, J. Lin and P. Huang, Chem. Soc. Rev., 2018, 47, 6454–6472 RSC
. - M. Alfonso-Prieto, X. Biarnes, P. Vidossich and C. Rovira, J. Am. Chem. Soc., 2009, 131, 11751–11761 CrossRef CAS PubMed
. - Y. Sheng, I. A. Abreu, D. E. Cabelli, M. J. Maroney, A. F. Miller, M. Teixeira and J. S. Valentine, Chem. Rev., 2014, 114, 3854–3918 CrossRef CAS PubMed
. - W. Yang, X. Shi, Y. Shi, D. Yao, S. Chen, X. Zhou and B. Zhang, ACS Nano, 2018, 12, 12169–12180 CrossRef CAS PubMed
. - D. He, L. Hai, X. He, X. Yang and H.-W. Li, Adv. Funct. Mater., 2017, 27, 1704089 CrossRef
. - M. Chang, M. Wang, M. Wang, M. Shu, B. Ding, C. Li, M. Pang, S. Cui, Z. Hou and J. Lin, Adv. Mater., 2019, 31, e1905271 CrossRef PubMed
. - X. Wang, T. Xiong, M. Cui, X. Guan, J. Yuan, Z. Wang, R. Li, H. Zhang, S. Duan and F. Wei, Appl. Mater. Today, 2020, 21, 100827 CrossRef
. - D. Wang, H. Wu, S. Z. F. Phua, G. Yang, W. Qi Lim, L. Gu, C. Qian, H. Wang, Z. Guo, H. Chen and Y. Zhao, Nat. Commun., 2020, 11, 357 CrossRef CAS PubMed
. - Y. Cheng, C. Wen, Y. Q. Sun, H. Yu and X. B. Yin, Adv. Funct. Mater., 2021, 31, 2104378 CrossRef CAS
. - F. Nan, Q. Jia, X. Xue, S. Wang, W. Liu, J. Wang, J. Ge and P. Wang, Biomaterials, 2022, 284, 121495 CrossRef CAS PubMed
. - J. Ding, G. Lu, W. Nie, L. L. Huang, Y. Zhang, W. Fan, G. Wu, H. Liu and H. Y. Xie, Adv. Mater., 2021, 33, e2005562 CrossRef PubMed
. - S. Y. Liu, Y. Xu, H. Yang, L. Liu, M. Zhao, W. Yin, Y. T. Xu, Y. Huang, C. Tan, Z. Dai, H. Zhang, J. P. Zhang and X. M. Chen, Adv. Mater., 2021, 33, e2100849 CrossRef PubMed
. - S. Fu, R. Yang, J. Ren, J. Liu, L. Zhang, Z. Xu, Y. Kang and P. Xue, ACS Nano, 2021, 15, 11953–11969 CrossRef CAS PubMed
. - J. Wang, J. Huang, W. Zhou, J. Zhao, Q. Peng, L. Zhang, Z. Wang, P. Li and R. Li, J. Nanobiotechnol., 2021, 19, 87 CrossRef CAS PubMed
. - S. K. Rajendrakumar, V. Revuri, M. Samidurai, A. Mohapatra, J. H. Lee, P. Ganesan, J. Jo, Y.-K. Lee and I.-K. Park, Nano Lett., 2018, 18, 6417–6426 CrossRef CAS PubMed
. - D. Mao, F. Hu, Kenry, S. Ji, W. Wu, D. Ding, D. Kong and B. Liu, Adv. Mater., 2018, 30, e1706831 CrossRef PubMed
. - D. Yim, D. E. Lee, Y. So, C. Choi, W. Son, K. Jang, C. S. Yang and J. H. Kim, ACS Nano, 2020, 14, 10324–10336 CrossRef CAS PubMed
. - J. Zhu, J. Tian, C. Yang, J. Chen, L. Wu, M. Fan and X. Cai, Small, 2021, 17, e2101495 CrossRef PubMed
. - J. Ye, Z. Li, Q. Fu, Q. Li, X. Zhang, L. Su, H. Yang and J. Song, Adv. Funct. Mater., 2020, 30, 2001771 CrossRef CAS
. - G. Wang, X. Li, N. Li, X. Wang, S. He, W. Li, W. Fan, R. Li, J. Liu and S. Hou, Redox Biol., 2022, 52, 102297 CrossRef CAS PubMed
. - Y. Liu, K. Ai, X. Ji, D. Askhatova, R. Du, L. Lu and J. Shi, J. Am. Chem. Soc., 2017, 139, 856–862 CrossRef CAS PubMed
. - S. Gao, G. Wang, Z. Qin, X. Wang, G. Zhao, Q. Ma and L. Zhu, Biomaterials, 2017, 112, 324–335 CrossRef CAS PubMed
. - Y. Tian, H. Zhou, Q. Cheng, H. Dang, H. Qian, C. Teng, K. Xie and L. Yan, J. Mater. Chem. B, 2022, 10, 707–716 RSC
. - W. Liu, S. Y. Yin, Y. Hu, T. Deng and J. Li, ACS Appl. Mater. Interfaces, 2022, 14, 2629–2637 CrossRef CAS PubMed
. - X. Chen, X. Ren, L. Zhang, Z. Liu and Z. Hai, Anal. Chem., 2020, 92, 14244–14250 CrossRef CAS PubMed
. - T. Liu, N. Zhang, Z. Wang, M. Wu, Y. Chen, M. Ma, H. Chen and J. Shi, ACS Nano, 2017, 11, 9093–9102 CrossRef CAS PubMed
. - Q. Chen, C. Liang, X. Sun, J. Chen, Z. Yang, H. Zhao, L. Feng and Z. Liu, Proc. Natl. Acad. Sci. U. S. A., 2017, 114, 5343–5348 CrossRef CAS PubMed
. - C. Cheng, Y. Gao, W. Song, Q. Zhao, H. Zhang and H. Zhang, Chem. Eng. J., 2020, 380, 140049 Search PubMed
. - Q. Pan, B. Zhang, X. Peng, S. Wan, K. Luo, W. Gao, Y. Pu and B. He, Chem. Commun., 2019, 55, 13896–13899 RSC
. - C. Yik-Sham Chung, G. A. Timblin, K. Saijo and C. J. Chang, J. Am. Chem. Soc., 2018, 140, 6109–6121 CrossRef CAS PubMed
. - J. F. Mukerabigwi, W. Yin, Z. Zha, W. Ke, Y. Wang, W. Chen, A. Japir, Y. Wang and Z. Ge, J. Control Release., 2019, 303, 209–222 CrossRef CAS PubMed
. - Q. Zeng, R. Zhang, T. Zhang and D. Xing, Biomaterials, 2019, 207, 39–48 CrossRef CAS PubMed
. - Z. W. Wang, D. Su, X. Q. Li, J. J. Cao, D. C. Yang and J. Y. Liu, Molecules, 2018, 24, 32 CrossRef PubMed
. - M. C. Chang, A. Pralle, E. Y. Isacoff and C. J. Chang, J. Am. Chem. Soc., 2004, 126, 15392–15393 CrossRef CAS PubMed
. - J. Wang, J. Liu, D. Q. Lu, L. Chen, R. Yang, D. Liu and B. Zhang, Carbohydr. Polym., 2022, 292, 119699 CrossRef CAS PubMed
. - Y. J. Jo, M. Gulfam, S. H. Jo, Y. S. Gal, C. W. Oh, S. H. Park and K. T. Lim, Carbohydr. Polym., 2022, 286, 119303 CrossRef CAS PubMed
. - J. Yang, S. Pan, S. Gao, T. Li and H. Xu, Biomaterials, 2021, 271, 120721 CrossRef CAS PubMed
. - D. Pham, U. Basu, I. Pohorilets, C. M. St Croix, S. C. Watkins and K. Koide, Angew Chem. Int. Ed. Engl., 2020, 59, 17435–17441 CrossRef CAS PubMed
. - S. Ye, J. J. Hu and D. Yang, Angew Chem. Int. Ed. Engl., 2018, 57, 10173–10177 CrossRef CAS PubMed
. - B. Dong, X. Song, X. Kong, C. Wang, Y. Tang, Y. Liu and W. Lin, Adv. Mater., 2016, 28, 8755–8759 CrossRef CAS PubMed
. - N. Soh, O. Sakawaki, K. Makihara, Y. Odo, T. Fukaminato, T. Kawai, M. Irie and T. Imato, Bioorg. Med. Chem., 2005, 13, 1131–1139 CrossRef CAS PubMed
. - H. Ding, Y. Cai, L. Gao, M. Liang, B. Miao, H. Wu, Y. Liu, N. Xie, A. Tang, K. Fan, X. Yan and G. Nie, Nano Lett., 2019, 19, 203–209 CrossRef CAS PubMed
. - P. Campomanes, U. Rothlisberger, M. Alfonso-Prieto and C. Rovira, J. Am. Chem. Soc., 2015, 137, 11170–11178 CrossRef CAS PubMed
. - X. Qin, C. Wu, D. Niu, L. Qin, X. Wang, Q. Wang and Y. Li, Nat. Commun., 2021, 12, 5243 CrossRef CAS PubMed
. - F. P. Chang, Y. P. Chen and C. Y. Mou, Small, 2014, 10, 4785–4795 CrossRef CAS PubMed
. - Y. Fujiki and M. C. Bassik, Trends Cell Biol., 2021, 31, 148–151 CrossRef CAS PubMed
. - B. K. Vainshtein, W. R. Melik-Adamyan, V. V. Barynin, A. A. Vagin and A. I. Grebenko, Nature, 1981, 293, 411–412 CrossRef CAS PubMed
. - S. Z. F. Phua, G. Yang, W. Q. Lim, A. Verma, H. Chen, T. Thanabalu and Y. Zhao, ACS Nano, 2019, 13, 4742–4751 CrossRef CAS PubMed
. - Q. Chen, J. Chen, Z. Yang, J. Xu, L. Xu, C. Liang, X. Han and Z. Liu, Adv. Mater., 2019, 31, e1802228 CrossRef PubMed
. - H. Phan, R. Cavanagh, D. Destouches, F. Vacherot, B. Brissault, V. Taresco, J. Penelle and B. Couturaud, ACS Appl. Polym. Mater., 2022, 4, 7778–7789 CrossRef CAS
. - S. Park, J. Yoon, S. Bae, M. Park, C. Kang, Q. Ke, D. Lee and P. M. Kang, Biomaterials, 2014, 35, 5944–5953 CrossRef CAS PubMed
. - K. Ou, Y. Kang, L. Chen, X. Zhang, X. Chen, Y. Zheng, J. Wu and S. Guan, Biomater. Sci., 2019, 7, 2491–2498 RSC
. - Z. Lu, J. Zhang, W. Yin, C. Guo and M. Lang, Macromol. Rapid Commun., 2022, 43, e2200156 CrossRef PubMed
. - T. Liu, J. Tian, L. Cui, Q. Liu, L. Wu and X. Zhang, Colloids Surf. B Biointerfaces, 2019, 178, 137–145 CrossRef CAS PubMed
. - Z. Wang, L. Zhang and Y. Tian, Analyst, 2015, 140, 3788–3793 RSC
. - C. Ren, L. Chu, F. Huang, L. Yang, H. Fan, J. Liu and C. Yang, RSC Adv., 2017, 7, 1313–1317 RSC
. - X. Guo, Y. Li, Y. Li, Z. Ye, J. Zhang, T. Zhu and F. Li, Electrochim. Acta, 2020, 354, 136763 CrossRef CAS
. - Z. Qu, D. Zhang, C. Wang, S. Tian, Y. Deng, D. Qin and H. Duan, Polymers, 2022, 14, 3005 CrossRef CAS PubMed
. - X. Shang, S. He, Z. Xu, W. Lu and W. Zhang, Electroanalysis, 2021, 33, 1088–1095 CrossRef CAS
. - W. Wu, L. Huang, E. Wang and S. Dong, Chem. Sci., 2020, 11, 9741–9756 RSC
. - Y. Fan, S. Liu, Y. Yi, H. Rong and J. Zhang, ACS Nano, 2021, 15, 2005–2037 CrossRef CAS PubMed
. - S. Gao, H. Lin, H. Zhang, H. Yao, Y. Chen and J. Shi, Adv. Sci., 2019, 6, 1801733 CrossRef PubMed
. - S. Wang, Q. You, J. Wang, Y. Song, Y. Cheng, Y. Wang, S. Yang, L. Yang, P. Li, Q. Lu, M. Yu and N. Li, Nanoscale, 2019, 11, 6270–6284 RSC
. - J. Yu, F. Zhao, W. Gao, X. Yang, Y. Ju, L. Zhao, W. Guo, J. Xie, X. J. Liang, X. Tao, J. Li, Y. Ying, W. Li, J. Zheng, L. Qiao, S. Xiong, X. Mou, S. Che and Y. Hou, ACS Nano, 2019, 13, 10002–10014 CrossRef CAS PubMed
. - C. Wu, X. Han, W. Feng, Z. Liu, L. Chen, B. Zhou, Y. Chen and J. Shi, Chem. Eng. J., 2021, 411, 128543 CrossRef CAS
. - Z. Wang, Y. Zhang, E. Ju, Z. Liu, F. Cao, Z. Chen, J. Ren and X. Qu, Nat. Commun., 2018, 9, 3334 CrossRef PubMed
. - S. Zhang, Q. Li, N. Yang, Y. Shi, W. Ge, W. Wang, W. Huang, X. Song and X. Dong, Adv. Funct. Mater., 2019, 29, 1906805 CrossRef CAS
. - Q. You, K. Zhang, J. Liu, C. Liu, H. Wang, M. Wang, S. Ye, H. Gao, L. Lv, C. Wang, L. Zhu and Y. Yang, Adv. Sci., 2020, 7, 1903341 CrossRef CAS PubMed
. - M. Mao, X. Guan, F. Wu and L. Ma, Nanomaterials, 2022, 12, 639 CrossRef PubMed
. - X. Zhang, Y. Lu, Q. Chen and Y. Huang, J. Mater. Chem. B, 2020, 8, 6459–6468 RSC
. - S. Liu, L. Fang, H. Ding, Y. Zhang, W. Li, B. Liu, S. Dong, B. Tian, L. Feng and P. Yang, ACS Nano, 2022, 16, 20805–20819 CrossRef CAS PubMed
. - R. André, F. Natálio, M. Humanes, J. Leppin, K. Heinze, R. Wever, H. C. Schröder, W. E. G. Müller and W. Tremel, Adv. Funct. Mater., 2011, 21, 501–509 CrossRef
. - A. Y. Zhang, T. Lin, Y. Y. He and Y. X. Mou, J. Hazard. Mater., 2016, 311, 81–90 CrossRef CAS PubMed
. - S. Guan, X. Liu, Y. Fu, C. Li, J. Wang, Q. Mei, G. Deng, W. Zheng, Z. Wan and J. Lu, J. Colloid Interface Sci., 2022, 608, 344–354 CrossRef CAS PubMed
. - X. Zhu, M. Wang, H. Wang, Y. Ding, Y. Liu, Z. Fu, D. Lin, C. Lu and X. Tu, Small, 2022, 18, 2204951 CrossRef CAS PubMed
. - C. Wu, S. Wang, J. Zhao, Y. Liu, Y. Zheng, Y. Luo, C. Ye, M. Huang and H. Chen, Adv. Funct. Mater., 2019, 29, 1901722 CrossRef
. - L. H. Fu, Y. Wan, C. Qi, J. He, C. Li, C. Yang, H. Xu, J. Lin and P. Huang, Adv. Mater., 2021, 33, e2006892 CrossRef PubMed
. - S. Gao, Y. Jin, K. Ge, Z. Li, H. Liu, X. Dai, Y. Zhang, S. Chen, X. Liang and J. Zhang, Adv. Sci., 2019, 6, 1902137 CrossRef CAS PubMed
. - X. Lu, S. Gao, H. Lin and J. Shi, Small, 2021, 17, e2004467 CrossRef PubMed
. - M. Chang, Z. Hou, M. Wang, C. Yang, R. Wang, F. Li, D. Liu, T. Peng, C. Li and J. Lin, Angew Chem. Int. Ed. Engl., 2021, 60, 12971–12979 CrossRef CAS PubMed
. - R. Zhang, B. Xue, Y. Tao, H. Zhao, Z. Zhang, X. Wang, X. Zhou, B. Jiang, Z. Yang, X. Yan and K. Fan, Adv. Mater., 2022, 34, e2205324 CrossRef PubMed
. - D. Zhu, H. Chen, C. Huang, G. Li, X. Wang, W. Jiang and K. Fan, Adv. Funct. Mater., 2022, 32, 2110268 CrossRef CAS
. - Q. Zhang, S. Chen and H. Wang, Green Chem., 2018, 20, 4067–4074 RSC
. - F. Cao, Y. Sang, C. Liu, F. Bai, L. Zheng, J. Ren and X. Qu, ACS Nano, 2022, 16, 855–868 CrossRef CAS PubMed
. - D. Zhu, H. Chen, C. Huang, G. Li, X. Wang, W. Jiang and K. Fan, Adv. Funct. Mater., 2022, 32, 2110268 CrossRef CAS
. - Y. Bao, J. Chen, H. Qiu, C. Zhang, P. Huang, Z. Mao and W. Tong, ACS Appl. Mater. Interfaces, 2021, 13, 24532–24542 CrossRef CAS PubMed
. - Y. Zhang, F. Wang, C. Liu, Z. Wang, L. Kang, Y. Huang, K. Dong, J. Ren and X. Qu, ACS Nano, 2018, 12, 651–661 CrossRef CAS PubMed
. - M. Melchionna and P. Fornasiero, Chem, 2019, 5, 1927–1928 CAS
. - X. Lyu, X. Liu, C. Zhou, S. Duan, P. Xu, J. Dai, X. Chen, Y. Peng, D. Cui, J. Tang, X. Ma and W. Wang, J. Am. Chem. Soc., 2021, 143, 12154–12164 CrossRef CAS PubMed
. - Y. Ji, X. Lin, H. Zhang, Y. Wu, J. Li and Q. He, Angew Chem. Int. Ed. Engl., 2019, 58, 4184–4188 CrossRef CAS PubMed
. - G.-J. Cao, X. Jiang, H. Zhang, T. R. Croley and J.-J. Yin, RSC Adv., 2017, 7, 52210–52217 RSC
. - H. Ye, J. Mohar, Q. Wang, M. Catalano, M. J. Kim and X. Xia, Sci. Bull., 2016, 61, 1739–1745 CrossRef CAS
. - C. Wei, Y. Liu, X. Zhu, X. Chen, Y. Zhou, G. Yuan, Y. Gong and J. Liu, Biomaterials, 2020, 238, 119848 CrossRef CAS PubMed
. - T. Chen, G. Han and X. Li, Bioact. Mater., 2022, 12, 143–152 CrossRef CAS PubMed
. - J. M. Garcia-Martinez and E. P. Collar, Polymers, 2020, 13, 86 CrossRef PubMed
. - H. C. Zhou, J. R. Long and O. M. Yaghi, Chem. Rev., 2012, 112, 673–674 CrossRef CAS PubMed
. - Y. Wang, Y. Zhu, A. Binyam, M. Liu, Y. Wu and F. Li, Biosens. Bioelectron., 2016, 86, 432–438 CrossRef CAS PubMed
. - L. Zhang, S. S. Wan, C. X. Li, L. Xu, H. Cheng and X. Z. Zhang, Nano Lett., 2018, 18, 7609–7618 CrossRef CAS PubMed
. - O. Adegoke and P. B. Forbes, Anal. Chim. Acta, 2015, 862, 1–13 CrossRef CAS PubMed
. - R. C. Castro, J. X. Soares, D. S. M. Ribeiro and J. L. M. Santos, Sens. Actuators, B, 2019, 296, 126665 CrossRef CAS
. - J. Zhou, R. Zhao, Y. Du, S. Liu, W. Li, S. Gai, F. He, L. Feng and P. Yang, Adv. Funct. Mater., 2022, 32, 2112083 CrossRef CAS
. - H. Chen, K. Wen, J. Chen, W. Xing, X. Wu, Q. Shi, A. Peng and H. Huang, Sci. Bull., 2020, 65, 1580–1586 CrossRef CAS PubMed
. - X. Huang, J. Wang, H. Liu, T. Lan and J. Ren, Talanta, 2013, 106, 79–84 CrossRef CAS PubMed
. - Z. Zhou, L. Yang, L. Huang, Y. Liao, Y. Liu and Q. Xiao, Anal. Chim. Acta, 2020, 1106, 176–182 CrossRef CAS PubMed
. - X. Chen, T. Wang, W. Le, X. Huang, M. Gao, Q. Chen, S. Xu, D. Yin, Q. Fu, C. Shao, B. Chen and D. Shi, Theranostics, 2020, 10, 3430–3450 CrossRef CAS PubMed
. - S. Wang, E. Zhou, X. Wei, R. Liu, C. Li, L. Pan, Y. Zheng and N. Xing, ACS Omega, 2022, 7, 20241–20249 CrossRef CAS PubMed
. - R. Shen, P. Liu, Y. Zhang, Z. Yu, X. Chen, L. Zhou, B. Nie, A. Zaczek, J. Chen and J. Liu, Anal. Chem., 2018, 90, 4478–4484 CrossRef CAS PubMed
. - T. Zhou, Q. Yao, T. Zhao and X. Chen, Talanta, 2015, 141, 80–85 CrossRef CAS PubMed
. - Q. Raas, F. E. Saih, C. Gondcaille, D. Trompier, Y. Hamon, V. Leoni, C. Caccia, B. Nasser, M. Jadot, F. Menetrier, G. Lizard, M. Cherkaoui-Malki, P. Andreoletti and S. Savary, Biochim. Biophys. Acta, Mol. Cell Biol. Lipids, 2019, 1864, 567–576 CrossRef CAS PubMed
. - K. Zhang, C. Qi and K. Cai, Adv. Mater., 2022, 35, 2205409 CrossRef PubMed
. - F. Wu, Q. Zhang, B. Sun, X. Chu, M. Zhang, Z. She, Z. Li, N. Zhou, J. Wang and A. Li, J. Control Release., 2021, 338, 46–55 CrossRef CAS PubMed
. - S. Bai, N. Yang, X. Wang, F. Gong, Z. Dong, Y. Gong, Z. Liu and L. Cheng, ACS Nano, 2020, 14, 15119–15130 CrossRef CAS PubMed
. - X. Zhong, X. Wang, G. Zhan, Y. Tang, Y. Yao, Z. Dong, L. Hou, H. Zhao, S. Zeng, J. Hu, L. Cheng and X. Yang, Nano Lett., 2019, 19, 8234–8244 CrossRef CAS PubMed
. - G. Song, Y. Chen, C. Liang, X. Yi, J. Liu, X. Sun, S. Shen, K. Yang and Z. Liu, Adv. Mater., 2016, 28, 7143–7148 CrossRef CAS PubMed
. - X. Jing, F. Yang, C. Shao, K. Wei, M. Xie, H. Shen and Y. Shu, Mol. Cancer, 2019, 18, 157 CrossRef PubMed
. - Y. Li, L. Zhao and X. F. Li, Technol. Cancer Res. Treat., 2021, 20, 15330338211036304 CAS
. - G. L. Wang, B. H. Jiang, E. A. Rue and G. L. Semenza, Proc. Natl. Acad. Sci. U. S. A., 1995, 92, 5510–5514 CrossRef CAS PubMed
. - Q. Chen, L. Feng, J. Liu, W. Zhu, Z. Dong, Y. Wu and Z. Liu, Adv. Mater., 2016, 28, 7129–7136 CrossRef CAS PubMed
. - N. Yang, W. Xiao, X. Song, W. Wang and X. Dong, Nano-Micro Lett., 2020, 12, 15 CrossRef CAS PubMed
. - Y. Wang, Y. Li, Z. Zhang, L. Wang, D. Wang and B. Z. Tang, Adv. Mater., 2021, 33, e2103748 CrossRef PubMed
. - C. Yao, W. Wang, P. Wang, M. Zhao, X. Li and F. Zhang, Adv. Mater., 2018, 30, 1704833 CrossRef PubMed
. - X. Wang, M. Wu, H. Li, J. Jiang, S. Zhou, W. Chen, C. Xie, X. Zhen and X. Jiang, Advanced Science, 2022, 9, 2104125 CrossRef CAS PubMed
. - T. Wu, Y. Liu, Y. Cao and Z. Liu, Adv. Mater., 2022, 34, e2110364 CrossRef PubMed
. - Q. Liu, L. Shi, Y. Liao, X. Cao, X. Liu, Y. Yu, Z. Wang, X. Lu and J. Wang, Adv. Sci., 2022, 9, e2200005 CrossRef PubMed
. - P. Zhu, Y. Chen and J. Shi, ACS Nano, 2018, 12, 3780–3795 CrossRef CAS PubMed
. - L. Gong, Y. Zhang, J. Zhao, Y. Zhang, K. Tu, L. Jiao, Q. Xu, M. Zhang and S. Han, Small, 2022, 18, e2107656 CrossRef PubMed
. - Z. Yang, Y. Luo, H. Yu, K. Liang, M. Wang, Q. Wang, B. Yin and H. Chen, Adv. Mater., 2022, 34, e2108908 CrossRef PubMed
. - X. Zhou, M. You, F. Wang, Z. Wang, X. Gao, C. Jing, J. Liu, M. Guo, J. Li, A. Luo, H. Liu, Z. Liu and C. Chen, Adv. Mater., 2021, 33, e2100556 CrossRef PubMed
. - W. Sun, Z. Zhou, G. Pratx, X. Chen and H. Chen, Theranostics, 2020, 10, 1296–1318 CrossRef CAS PubMed
. - X. Song, J. Xu, C. Liang, Y. Chao, Q. Jin, C. Wang, M. Chen and Z. Liu, Nano Lett., 2018, 18, 6360–6368 CrossRef CAS PubMed
. - W. Zai, L. Kang, T. Dong, H. Wang, L. Yin, S. Gan, W. Lai, Y. Ding, Y. Hu and J. Wu, ACS Nano, 2021, 15, 15381–15394 CrossRef CAS PubMed
. - T. Chanmee, P. Ontong, K. Konno and N. Itano, Cancers, 2014, 6, 1670–1690 CrossRef PubMed
. - Y. Wang, F. Gao, X. Li, G. Niu, Y. Yang, H. Li and Y. Jiang, J. Nanobiotechnol., 2022, 20, 69 CrossRef CAS PubMed
. - T. J. Zhou, Y. Xu, L. Xing, Y. Wang and H. L. Jiang, Adv. Mater., 2021, 33, e2100114 CrossRef PubMed
. - P. Yang, J. Tao, F. Chen, Y. Chen, J. He, K. Shen, P. Zhao and Y. Li, Small, 2021, 17, e2005865 CrossRef PubMed
. - Z. Liu, T. Li, F. Han, Y. Wang, Y. Gan, J. Shi, T. Wang, M. L. Akhtar and Y. Li, Biomater. Sci., 2019, 7, 3683–3692 RSC
. - D. Wang, J. Zhou, R. Chen, R. Shi, G. Xia, S. Zhou, Z. Liu, N. Zhang, H. Wang, Z. Guo and Q. Chen, Biomaterials, 2016, 107, 88–101 CrossRef CAS PubMed
. - L. F. Yang, C. C. Ren, M. Xu, Y. L. Song, Q. L. Lu, Y. L. Wang, Y. Zhu, X. X. Wang and N. Li, Nano Res., 2020, 13, 2246–2258 CrossRef CAS
. - M. D. Liu, D. K. Guo, R. Y. Zeng, J. J. Ye, S. B. Wang, C. X. Li, Y. X. Sun, S. X. Cheng and X. Z. Zhang, Adv. Funct. Mater., 2020, 30, 2006098 CrossRef CAS
. - H. Z. Deng, Z. Yang, X. Y. Pang, C. Y. Zhao, J. Tian, Z. L. Wang and X. Y. Chen, Nano Today, 2022, 42, 101337 CrossRef CAS
. - X. Y. Lu, S. S. Gao, H. Lin, L. D. Yu, Y. H. Han, P. A. Zhu, W. C. Bao, H. L. Yao, Y. Chen and J. L. Shi, Adv. Mater., 2020, 32, 2002246 CrossRef CAS PubMed
. - Z. Tang, P. Zhao, H. Wang, Y. Liu and W. Bu, Chem. Rev., 2021, 121, 1981–2019 CrossRef CAS PubMed
. - X. Chen, H. Zhang, M. Zhang, P. Zhao, R. Song, T. Gong, Y. Liu, X. He, K. Zhao and W. Bu, Adv. Funct. Mater., 2019, 30, 1908365 CrossRef
. - L. S. Lin, J. B. Song, L. Song, K. M. Ke, Y. J. Liu, Z. J. Zhou, Z. Y. Shen, J. Li, Z. Yang, W. Tang, G. Niu, H. H. Yang and X. Y. Chen, Angew. Chem., Int. Ed., 2018, 57, 4902–4906 CrossRef CAS PubMed
. - L. H. Fu, C. Qi, Y. R. Hu, J. Lin and P. Huang, Adv. Mater., 2019, 31, e1808325 CrossRef PubMed
. - B. Chen, K. Guo, X. Zhao, Z. Liu, C. Xu, N. Zhao and F. J. Xu, Exploration, 2023, 20220140 CrossRef
. - A. L. Antaris, H. Chen, K. Cheng, Y. Sun, G. Hong, C. Qu, S. Diao, Z. Deng, X. Hu, B. Zhang, X. Zhang, O. K. Yaghi, Z. R. Alamparambil, X. Hong, Z. Cheng and H. Dai, Nat. Mater., 2016, 15, 235–242 CrossRef CAS PubMed
. - Y. Yu, B.-R. Xie, X.-H. Liu, J.-J. Ye, H. Cheng, Z. Zhong and X.-Z. Zhang, J. Mater. Chem. B, 2022, 10, 1634–1640 RSC
. - M. Ren, D. Dong, Q. Xu, J. Yin, S. Wang and F. Kong, Talanta, 2021, 234, 122684 CrossRef CAS PubMed
. - M. Li, X. Huang and J. Ren, Anal. Chem., 2021, 93, 3042–3051 CrossRef CAS PubMed
. - J. Cao, B. Qiao, Y. Luo, C. Cheng, A. Yang, M. Wang, X. Yuan, K. Fan, M. Li and Z. Wang, Biomater. Sci., 2020, 8, 6561–6578 RSC
. - W. Zhen, Y. Liu, W. Wang, M. Zhang, W. Hu, X. Jia, C. Wang and X. Jiang, Angew Chem.
Int. Ed. Engl., 2020, 59, 9491–9497 CrossRef CAS PubMed
. - Q. Guan, L. Shi, C. Li, X. Gao, K. Wang, X. Liang, P. Li and X. Zhu, ACS Biomater. Sci. Eng., 2019, 5, 1023–1033 CrossRef CAS PubMed
. - K. Liu, H. Shang, X. Kong, M. Ren, J. Y. Wang, Y. Liu and W. Lin, Biomaterials, 2016, 100, 162–171 CrossRef CAS PubMed
. - Y. Wang, S. Li, L. Feng, C. Nie, L. Liu, F. Lv and S. Wang, ACS Appl. Mater. Interfaces, 2015, 7, 24110–24118 CrossRef CAS PubMed
. - D. H. Zhao, C. Q. Li, X. L. Hou, X. T. Xie, B. Zhang, G. Y. Wu, F. Jin, Y. D. Zhao and B. Liu, ACS Appl. Mater. Interfaces, 2021, 13, 55780–55789 CrossRef CAS PubMed
. - X. Zhang, W. Wang, L. Su, X. Ge, J. Ye, C. Zhao, Y. He, H. Yang, J. Song and H. Duan, Nano Lett., 2021, 21, 2625–2633 CrossRef CAS PubMed
. - J. Weber, P. C. Beard and S. E. Bohndiek, Nat. Methods, 2016, 13, 639–650 CrossRef CAS PubMed
. - Q. Fu, R. Zhu, J. Song, H. Yang and X. Chen, Adv. Mater., 2019, 31, e1805875 Search PubMed
. - E. Jung, J. Noh, C. Kang, D. Yoo, C. Song and D. Lee, Biomaterials, 2018, 179, 175–185 CrossRef CAS PubMed
. - G. W. Kim, C. Kang, Y. B. Oh, M. H. Ko, J. H. Seo and D. Lee, Theranostics, 2017, 7, 2463–2476 CrossRef CAS PubMed
. - T. Iwashita, S. Uemura, M. Shimizu, F. Hyodo, H. Tomita, R. Iwasaki, M. Takasu, T. Mori, H. Tanaka and M. Matsuo, Ultrasound Med. Biol., 2019, 45, 579–585 CrossRef PubMed
. - E. Jung, C. Kang, J. Lee, D. Yoo, D. W. Hwang, D. Kim, S. C. Park, S. K. Lim, C. Song and D. Lee, ACS Nano, 2018, 12, 392–401 CrossRef CAS PubMed
. - H. Chen, D. Zheng, W. Pan, X. Li, B. Lv, W. Gu, J. O. Machuki, J. Chen, W. Liang, K. Qin, J. Greven, F. Hildebrand, Z. Yu, X. Zhang and K. Guo, ACS Appl. Mater. Interfaces, 2021, 13, 19710–19725 CrossRef CAS PubMed
. - Q. Wu, Q. Zhang, T. Yu, X. Wang, C. Jia, Z. Zhao and J. Zhao, ACS Appl. Bio Mater., 2021, 4, 4244–4253 CrossRef CAS PubMed
. - Y. Wang, J. Zhang, X. Lv, L. Wang, Z. Zhong, D. P. Yang, W. Si, T. Zhang and X. Dong, Biomaterials, 2020, 252, 120111 CrossRef CAS PubMed
. - X. Li, H. Zhou, Z. Niu, K. Zheng, D. Niu, W. Zhao, X. Liu, W. Si, C. Li, P. Wang, J. Cao, Y. Li and G. Wen, ACS Appl. Mater. Interfaces, 2020, 12, 24644–24654 CrossRef CAS PubMed
. - M. Tian, J. Sun, Y. Tang, B. Dong and W. Lin, Anal. Chem., 2018, 90, 998–1005 CrossRef CAS PubMed
. - D. Pan, A. H. Schmieder, S. A. Wickline and G. M. Lanza, Tetrahedron, 2011, 67, 8431–8444 CrossRef CAS PubMed
. - Z. Yin, Q. Ji, D. Wu, Z. Li, M. Fan, H. Zhang, X. Zhao, A. Wu, L. Cheng and L. Zeng, ACS Appl. Mater. Interfaces, 2021, 13, 14928–14937 CrossRef CAS PubMed
. - Y. Cheng, X. Tan, J. Wang, Y. Wang, Y. Song, Q. You, Q. Sun, L. Liu, S. Wang, F. Tan, J. Li and N. Li, J. Control Release., 2018, 277, 77–88 CrossRef CAS PubMed
. - Z. Wang, T. Jia, Q. Sun, Y. Kuang, B. Liu, M. Xu, H. Zhu, F. He, S. Gai and P. Yang, Biomaterials, 2020, 228, 119569 CrossRef CAS PubMed
. - G. Taubes, Science, 2008, 321, 356–361 CrossRef CAS PubMed
. - Y. Yang, X. Wu, L. Ma, C. He, S. Cao, Y. Long, J. Huang, R. D. Rodriguez, C. Cheng, C. Zhao and L. Qiu, Adv. Mater., 2021, 33, e2005477 CrossRef PubMed
. - X. Zhou, Z. Wang, Y. K. Chan, Y. Yang, Z. Jiao, L. Li, J. Li, K. Liang and Y. Deng, Adv. Funct. Mater., 2021, 32, 2109469 CrossRef
. - D. Sun, X. Pang, Y. Cheng, J. Ming, S. Xiang, C. Zhang, P. Lv, C. Chu, X. Chen, G. Liu and N. Zheng, ACS Nano, 2020, 14, 2063–2076 CrossRef CAS PubMed
. - L. Lai, W. Zou, Y. Zhang, Y. Tu, S. Li, T. Xin, T. Zhou, S. Xu, P. Zheng, Q. Pan and W. Zhu, Chem. Eng. J., 2022, 435, 135084 CrossRef CAS
. - N. Yang, H. Guo, C. Cao, X. Wang, X. Song, W. Wang, D. Yang, L. Xi, X. Mou and X. Dong, Biomaterials, 2021, 275, 120918 CrossRef CAS PubMed
. - Y. Shi, Y. Cao, J. Cheng, W. Yu, M. Liu, J. Yin, C. Huang, X. Liang, H. Zhou, H. Liu, Z. Yang, Y. Fang, H. Wei and G. Zhao, Adv. Funct. Mater., 2022, 32, 2111148 CrossRef CAS
. - L. Gao, Y. Liu, D. Kim, Y. Li, G. Hwang, P. C. Naha, D. P. Cormode and H. Koo, Biomaterials, 2016, 101, 272–284 CrossRef CAS PubMed
. - K. Villa, J. Viktorova, J. Plutnar, T. Ruml, L. Hoang and M. Pumera, Cell Rep. Phys. Sci., 2020, 1, 100181 CrossRef
. - K. Wang, Y. Sun, W. Xu, W. Zhang, F. Zhang, Y. Qi, Y. Zhang, Q. Zhou, B. Dong, C. Li, L. Wang and L. Xu, Sens. Actuators, B, 2022, 355, 131298 CrossRef CAS
. - K. Wu, X. Wu, M. Chen, H. Wu, Y. Jiao and C. Zhou, Chem. Eng. J., 2020, 387, 124127 CrossRef CAS
. - J. Liu, L. Shi, Y. Wang, M. Li, C. Zhou, L. Zhang, C. Yao, Y. Yuan, D. Fu, Y. Deng, M. Liu, G. Wang, L. Wang and Z. Wang, Nano Today, 2022, 47, 101627 CrossRef CAS
. - M. F. Chung, W. T. Chia, W. L. Wan, Y. J. Lin and H. W. Sung, J. Am. Chem. Soc., 2015, 137, 12462–12465 CrossRef CAS PubMed
. - Y. Cheng, J. Dai, C. Sun, R. Liu, T. Zhai, X. Lou and F. Xia, Angew Chem. Int. Ed. Engl., 2018, 57, 3123–3127 CrossRef CAS PubMed
. - R. Etzioni, N. Urban, S. Ramsey, M. McIntosh, S. Schwartz, B. Reid, J. Radich, G. Anderson and L. Hartwell, Nat. Rev. Cancer, 2003, 3, 243–252 CrossRef CAS PubMed
. - X. Li, Y. Liu, X. Qi, S. Xiao, Z. Xu, Z. Yuan, Q. Liu, H. Li, S. Ma, T. Liu, Y. Huang, X. Zhang, X. Zhang, Z. Mao, G. Luo and J. Deng, Adv. Mater., 2022, 34, 2109004 CrossRef CAS PubMed
. - E. Jung, J. Noh, C. Kang, D. Yoo, C. Song and D. Lee, Biomaterials, 2018, 179, 175–185 CrossRef CAS PubMed
. - M. Yu, B. S. Bouley, D. Xie, J. S. Enriquez and E. L. Que, J. Am. Chem. Soc., 2018, 140, 10546–10552 CrossRef CAS PubMed
. - L. Sun, J. Ouyang, F. Zeng and S. Wu, Biomaterials, 2022, 283, 121468 CrossRef CAS PubMed
. - J. Chen, L. Chen, Y. Wu, Y. Fang, F. Zeng, S. Wu and Y. Zhao, Nat. Commun., 2021, 12, 6870 CrossRef CAS PubMed
. - M. Li, Y. Wang, X. Han, Y. Liu, M. Ma and L. Zhang, Pharmaceutics, 2022, 14, 1988 CrossRef CAS PubMed
. - J. Ding, Y. Yao, J. Li, Y. Duan, J. R. Nakkala, X. Feng, W. Cao, Y. Wang, L. Hong, L. Shen, Z. Mao, Y. Zhu and C. Gao, Small, 2020, 16, e2005038 CrossRef PubMed
. - A. Jain, M. Behera, C. Mahapatra, N. R. Sundaresan and K. Chatterjee, Mater. Sci. Eng. C, 2021, 118, 111416 CrossRef CAS PubMed
. - E. Jung, T. Kim, S. Bae, P. M. Kang and D. Lee, Adv. Ther., 2021, 4, 2000273 CrossRef CAS
. - W. Gao, X. Li, Z. Liu, W. Fu, Y. Sun, W. Cao, L. Tong and B. Tang, Anal.
Chem., 2019, 91, 1150–1156 CrossRef CAS PubMed
. - C. Li, P. Wu, Y. Dou, Q. Li and J. Zhang, View, 2022, 3, 20200137 CrossRef CAS
. - S. S. Virani, A. Alonso, H. J. Aparicio, E. J. Benjamin, M. S. Bittencourt, C. W. Callaway, A. P. Carson, A. M. Chamberlain, S. Cheng, F. N. Delling, M. S. V. Elkind, K. R. Evenson, J. F. Ferguson, D. K. Gupta, S. S. Khan, B. M. Kissela, K. L. Knutson, C. D. Lee, T. T. Lewis, J. Liu, M. S. Loop, P. L. Lutsey, J. Ma, J. Mackey, S. S. Martin, D. B. Matchar, M. E. Mussolino, S. D. Navaneethan, A. M. Perak, G. A. Roth, Z. Samad, G. M. Satou, E. B. Schroeder, S. H. Shah, C. M. Shay, A. Stokes, L. B. VanWagner, N. Y. Wang, C. W. Tsao, E. American Heart Association Council on, C. Prevention Statistics and S. Stroke Statistics, Circulation, 2021, 143, e254–e743 CrossRef PubMed
. - M. S. Oliveira, L. Y. Tanaka, E. L. Antonio, L. I. Brandizzi, A. J. Serra, L. Dos Santos, J. E. Krieger, F. R. M. Laurindo and P. J. F. Tucci, Mol. Med. Rep., 2020, 21, 1431–1438 CAS
. - K. S. Cunningham, D. A. Spears and M. Care, Forensic Sci. Res., 2019, 4, 223–240 CrossRef PubMed
. - R. Aikawa, T. Nagai, M. Tanaka, Y. Zou, T. Ishihara, H. Takano, H. Hasegawa, H. Akazawa, M. Mizukami, R. Nagai and I. Komuro, Biochem. Biophys. Res. Commun., 2001, 289, 901–907 CrossRef CAS PubMed
. - Z. Wang, H. Zhao, K. Chen, H. Li and M. Lan, Anal. Chim. Acta, 2021, 1188, 339202 CrossRef CAS PubMed
. - J. Lee, L. Jeong, E. Jung, C. Ko, S. Seon, J. Noh and D. Lee, J. Control Release., 2019, 304, 164–172 CrossRef CAS PubMed
. - E. Jung, J. Lee, L. Jeong, S. Park, M. Lee, C. Song and D. Lee, Biomaterials, 2019, 192, 282–291 CrossRef CAS PubMed
. - T. Y. Lee, W. J. Lu, C. A. Changou, Y. C. Hsiung, N. T. T. Trang, C. Y. Lee, T. H. Chang, T. Jayakumar, C. Y. Hsieh, C. H. Yang, C. C. Chang, R. J. Chen, J. R. Sheu and K. H. Lin, Autophagy, 2021, 17, 4141–4158 CrossRef CAS PubMed
. - C. Cui, Z. Yang, X. Hu, J. Wu, K. Shou, H. Ma, C. Jian, Y. Zhao, B. Qi, X. Hu, A. Yu and Q. Fan, ACS Nano, 2017, 11, 3298–3310 CrossRef CAS PubMed
. - Y. Zhao, R. Xie, N. Yodsanit, M. Ye, Y. Wang and S. Gong, Nano Today, 2020, 35, 100986 CrossRef CAS PubMed
. - J. E. Freedman, Arterioscler. Thromb. Vasc. Biol., 2008, 28, s11–s16 CrossRef CAS PubMed
. - W. Gao, X. Li, Z. Liu, W. Fu, Y. Sun, W. Cao, L. Tong and B. Tang, Anal. Chem., 2018, 91, 1150–1156 CrossRef PubMed
. - Y. Chen, Z. Chen, J. Duan, L. Gui, H. Li, X. Liang, X. Tian, K. Liu, Y. Li and J. Yang, J. Nanobiotechnol., 2022, 20, 145 CrossRef CAS PubMed
. - M. Zuo, W. Qian, Z. Xu, W. Shao, X. Y. Hu, D. Zhang, J. Jiang, X. Sun and L. Wang, Small, 2018, 14, e1801942 CrossRef PubMed
. - Q. Chen, J. Li, F. Han, Q. Meng, H. Wang, Q. Wei, Z. Li, F. Li, E. Xie, X. Qin, S. Chen, W. Wang, C. Liu, B. Li and F. Han, Adv. Funct. Mater., 2022, 32, 2201067 CrossRef CAS
. - G. Yang, M. Fan, J. Zhu, C. Ling, L. Wu, X. Zhang, M. Zhang, J. Li, Q. Yao, Z. Gu and X. Cai, Biomaterials, 2020, 255, 120155 CrossRef CAS PubMed
. - A. Lin, Z. Sun, X. Xu, S. Zhao, J. Li, H. Sun, Q. Wang, Q. Jiang, H. Wei and D. Shi, Nano Lett., 2022, 22, 508–516 CrossRef CAS PubMed
. - D. Yoo, K. Guk, H. Kim, G. Khang, D. Wu and D. Lee, Int. J. Pharm., 2013, 450, 87–94 CrossRef CAS PubMed
. - H. Zhang, S. Li, Y. Liu, Y. Yu, S. Lin, Q. Wang, L. Miao, H. Wei and W. Sun, Biomater. Sci., 2020, 8, 5984–5993 RSC
. - Y. Li, Q. Pan, J. Xu, X. He, H. A. Li, D. A. Oldridge, G. Li and L. Qin, J. Orthop. Translat., 2021, 27, 110–118 CrossRef PubMed
. - J. Li, F. Han, J. Ma, H. Wang, J. Pan, G. Yang, H. Zhao, J. Zhao, J. Liu, Z. Liu and B. Li, Adv. Funct. Mater., 2021, 32, 2111208 CrossRef
. - X. Mu, H. He, J. Wang, W. Long, Q. Li, H. Liu, Y. Gao, L. Ouyang, Q. Ren, S. Sun, J. Wang, J. Yang, Q. Liu, Y. Sun, C. Liu, X. D. Zhang and W. Hu, Nano Lett., 2019, 19, 4527–4534 CrossRef CAS PubMed
. - L. Lei, Q. Tu, L. Jiao, S. Xiang, L. Wang, X. Ran, B. Xiao, G. Feng, J. Feng and C. Zhang, Chem. Eng. J., 2022, 432, 134356 CrossRef CAS
. - L. Wu, X. Xiong, X. Wu, Y. Ye, Z. Jian, Z. Zhi and L. Gu, Front. Mol. Neurosci., 2020, 13, 28 CrossRef CAS PubMed
. - C. Li, Z. Zhao, Y. Luo, T. Ning, P. Liu, Q. Chen, Y. Chu, Q. Guo, Y. Zhang, W. Zhou, H. Chen, Z. Zhou, Y. Wang, B. Su, H. You, T. Zhang, X. Li, H. Song, C. Li, T. Sun and C. Jiang, Adv. Sci., 2021, 8, e2101526 CrossRef PubMed
. - T. Kalogeris, C. P. Baines, M. Krenz and R. J. Korthuis, Compr. Physiol., 2016, 7, 113–170 Search PubMed
. - J. Wang, Y. Zhu, L. Yang, H. Liu, T. Zhou, F. Xu, P. Xu, L. Yuan and L. Liang, ACS Sens., 2021, 6, 1330–1338 CrossRef CAS PubMed
. - J. Yang, J. Yang, S. H. Liang, Y. Xu, A. Moore and C. Ran, Sci. Rep., 2016, 6, 35613 CrossRef CAS PubMed
. - L. Qiao, Y. Shen, S. Zhang, M. Wang, G. Lv, Q. Dou and C. Li, BMEMat, 2023, 1, 12011 CrossRef
. - M. Nita and A. Grzybowski, Oxid. Med. Cell. Longev., 2016, 2016, 3164734 Search PubMed
. - Y. Chen, G. Mehta and V. Vasiliou, Ocul. Surf., 2009, 7, 176–185 CrossRef PubMed
. - S. W. Choi, B. G. Cha and J. Kim, ACS Nano, 2020, 14, 2483–2496 CrossRef CAS PubMed
.
|
This journal is © The Royal Society of Chemistry 2023 |
Click here to see how this site uses Cookies. View our privacy policy here.