DOI:
10.1039/D4DT02714K
(Paper)
Dalton Trans., 2024,
53, 19153-19165
Rhenium(I) and technetium(I) complexes with megazol derivatives: towards the development of a theranostic platform for Chagas disease†
Received
25th September 2024
, Accepted 31st October 2024
First published on 6th November 2024
Abstract
The diagnosis and treatment of Chagas disease (CD) in the chronic phase remains a challenge. With that in mind, a potential theranostic device based on the trypanocidal agent known as megazol and the fac-M(CO)3+ (M = Re or 99mTc) fragment is proposed in the present work. The peripheral structure of megazol (LH,H) was modified to obtain the compounds LR1,R2 (R1 = H, R2 = Me and R1 = R2 = Me), which were used in the syntheses of complexes of composition [ReBr(CO)3LR1,R2]. These compounds were studied by elemental analysis, FTIR, UV-vis, NMR (1H and 13C), HR-ESI-MS, HPLC/UPLC and single-crystal XRD. The trypanocidal activity of the rhenium complexes was evaluated in vitro against the intracellular form of Trypanosoma cruzi. With exception of the [ReBr(CO)3LMe,Me] complex, all compounds are more active than the standard drug, benznidazole (Bzn), while [ReBr(CO)3LH,H] also exhibited a much higher selectivity index. In addition, the interaction of megazol and its ReI complex was evaluated with the T. cruzi Old Yellow Enzyme (TcOYE) by both experimental and computational methods. The data showed that megazol as well as its metal complex exhibited a higher affinity for TcOYE compared to Bzn. Finally, the labelling of megazol with 99mTc was successfully carried out. However, the results indicated that the Re complexes used as standards were not homologous with the 99mTc complexes. Despite this discrepancy, this research suggests that the investigation into Re and 99mTc complexes with megazol could lead to the development of a theranostic device for CD in a near future.
Introduction
Neglected Tropical Diseases (NTDs) pose significant health challenges, particularly for the most vulnerable populations residing in remote rural areas, urban slums, and conflict zones within tropical and sub-tropical regions.1–4 Among these diseases, American trypanosomiasis, commonly known as Chagas disease (CD), stands out as one of the most lethal parasitic diseases in Latin America, second only to malaria, claiming thousands of lives annually.1,2 The World Health Organization (WHO) estimates that approximately 30
000–40
000 new infections occur worldwide each year.1 Diagnosis and treatment, especially during the chronic phase of the disease, remain challenging due to the absence of symptoms and low levels of parasitemia.5,6 As a result, there is a critical need for the development of both new trypanocidal agents and more effective diagnostic techniques.6 A device combining both functions, diagnosis and treatment, would be particularly valuable in combating CD effectively.
Recently, an extension of definition of the “theranostic pair” Tc/Re was described considering the combination of an organometallic complex containing the γ-emitter isotope 99mTc with its non-radioactive rhenium homologue.7 In this scenario, the rhenium complex is designed to possess therapeutic activity, while the 99mTc homologue enables the visualization through radioimaging, allowing sensitive detection of biological processes within the body (in vivo). Complexes of these two elements in oxidation state I, d6 electronic configuration, are expected to be isostructural and physiologically robust. Therefore, by combining the multimodality of the fragment M(CO)3+ with the theranostic properties of the pair 99mTc/Re, a multifunctional system can be developed. This system enables simultaneous diagnosis (via radioimaging) and therapy,7 offering new opportunities for the application of technetium and rhenium in medicine.
99mTc is extensively used in medicine, presenting various clinical applications.8 Particularly considering the frequent occurrence of heart complications in CD infected patients, the use of 99mTc radiotracers for targeting the myocardium, such as Sestamibi (cardiolite) and Tetrofosmin,9 promise as an alternative for diagnosing and monitoring the progression of CD. This approach is supported by previous findings indicating that the biodistribution of 99mTcO4− is influenced by parasitemia with Trypanosoma cruzi.10 Furthermore, given the chemical similarities between of Re and Tc,11 as well as those between the metabolism of cancer cells and parasites,12 non-radioactive Re complexes can be used as models for comparison with technetium analogues and additionally can potentially be explored for chemotherapy. However, to develop therapeutically active rhenium complexes, organic molecules with antiparasitic activity must be utilized as ligands.
The treatment of CD relies on nitroheterocyclic compounds, which exert their biological effects through the bioactivation of the nitro group via enzymatic reduction and the generation of reactive oxygen species.13,14 Nitrofuran and nitroimidazole, for example, are found in the structures of nifurtimox (Fig. 1a) and benznidazole (Fig. 1b), respectively, two drugs used in the chemotherapy of CD. Then, the use of such compounds as ligands appear to be an attractive prospect. However, to stabilize the metal center effectively, chelating ligands are required what precludes the direct use of compounds like benznidazole. Consequently, it's necessary for the compound to have donor atoms in specific positions for coordination, in addition to possessing the desired biological properties. Megazol (Fig. 1c) emerges as a promising option. It consists of a 5-nitroimidazole with a thiadiazole ring, featuring donor atoms in suitable positions for chelation. Moreover, it demonstrates in vivo activity against T. cruzi and T. brucei strains by inactivating enzymes within the parasites.15,16 This dual functionality makes megazol a compelling candidate for the development of metal complexes aimed at treating CD.
 |
| Fig. 1 Chemical structure of drugs used in the chemotherapy of Chagas disease (a) nifurtimox, (b) benznidazole and (c) megazol. | |
Considering the promising possibilities of the combination of rhenium and 99mTc complexes for the development of a theranostic device capable of both therapy and diagnosis, in the present study, we focused on investigating the reactions of the antichagasic agent megazol and derivaties with a fac-Re(CO)3+ containing precursor. The primary objectives included evaluation of the antiproliferative effects of these compounds on T. cruzi amastigotes, as well as assessing their cytotoxic activity on normal cell lines in order to get information about the selectivity. Aiming to gain a preliminary comprehension into the mechanism of action of these compounds, T. cruzi Old Yellow Enzyme (TcOYE) was investigated as molecular target of the compounds, employing both experimental and theoretical approaches. Finally, radiolabeling experiments with the megazol derivatives and 99mTc have were performed in an attempt to provide the first step towards the development of a theranostic platform for CD.
Experimental
Materials
The compound (Et4N)2[ReBr3(CO)3] was synthesized according to a literature procedure.17 The chemicals, (1-methyl-5-nitro-1H-2-imidazole)methanol, thiosemicarbazide, 4-methyl-3-thiosemicarbazide, 4,4-dimethyl-3-thiosemicarbazide, Activated Manganese Oxide, Ammoniacal Iron(III) Sulfate Dodecahydrate, Sodium Boranecarbonate, Disodium Tartrate Dihydrate, Disodium Tetraborate Decahydrate, and all solvents used were obtained commercially and used without additional purification, with the exception of the toluene that was previously treated according to a standard procedure.18 [99mTcO4]− was eluted from a 99Mo/99mTc Ultratechnekow FM generator provided by the pharmaceutical company Mallinckrodt Schweiz AG. [99mTc(H2O)3(CO)3]+ was prepared according to ref. 19.
Instruments
The IR spectra were obtained on a PerkinElmer Spectrum Two spectrometer using a Specac Golden Gate™ ATR (attenuated total reflection) or on a BRUKER ALPHA II using a Platinum ATR QuickSnap™ accessory, both with resolutions of 4 cm−1 and 32 scans. The stretching and bending vibrations are represented by the symbols “υ” and “δ”, respectively. The intensities of the bands are symbolized as strong (s), medium (m), weak (w). The 1H and 13C spectra were obtained in deuterated solvents and collected using a Bruker DRX 400 (1H: 400 MHz, 13C: 100.6 MHz) or DRX 500 equipment (1H: 400 MHz, 13C: 125.8 MHz). Chemical shifts (δ) are in ppm (parts per million) relative to the residual peak of the solvent. The abbreviations for the splitting of the peaks are as follows: s (single), d (double), t (triple), q (quarter), m (multiplet). The software used for processing NMR data was the MestreNova version 6.0.2–5475. The electronic spectra were acquired on a SHIMADZU-2018 spectrometer on the DMSO solvent, with 1 cm optical-path quartz cuvette at room temperature. The microwave-assisted reactions were carried out using the Biotage Initiator+ Robot Eight and Anton Paar (AP) Monowave 200 instruments. The ESI-MS high-resolution (HR) analyses were carried out on a Thermo DFS double-focusing spectrometer system. (ThermoFisher Scientific, Germany). The samples were prepared with 1 mg of compound and dissolved in MeOH. The mass spectral data is presented with the mass/charge ratio (m/z) assignment. The UPLC-ESI-MS measurements were performed using a UPLC Waters Acquity coupled to a Bruker HCTTM, with a column Acquity UPLC BEH C18 1.7 μm (2.1 × 50 mm) and wavelength 200–800 nm, considering the m/z values for the most intense signal (Details are given in the ESI†).
Preparation of the compounds
All organic reactions and Re complexes were followed by UPLC and UPLC-ESI-MS, being discontinued immediately after consumption of the reagents.
Synthesis of the ligands
Preparation of 5-(1-methyl-5-nitro-1H-imidazole-2-il)-1,3,4-thiadiazol-2-amine (LR1,R2) Derivatives.
1 mmol of the desired thiosemicarbazone TscR,R derivative (see the synthesis in the ESI†) and 4 equivalents of ferric ammonium sulfate dodecahydrate were added to a 20 mL biotage bottle containing 8 mL of distilled H2O. The suspension was heated under microwave radiation at 80 °C for 90 minutes. Upon cooling to room temperature, the yellow precipitates formed were immediately filtered and washed with 100 mL of H2O. The products were recrystallized from acetone affording yellow crystals.
LH,H (R1 = H; R2 = H) – UPLC retention time: 1.88 minutes. Yield: 68.3% (154.5 mg). Elemental Analysis Calculated for C6H6N6O2S (226.21 g mol−1): C: 31.9; H: 2.7; N: 37.2; Found: C: 31.7; H: 2.7, N: 37.1 IR (cm−1): 3428w and 3280w υ(N−H), 3072w υ(C−H), 1632m δ(C−N) + υ(C
N), 1518m δ(C
N) + υ (C
N), 1494s υ(N−O)ass, 1365s υ(N−O)s, 1336s υ(C−S). 1H NMR (DMSO-d6, 500 MHz), δ/ppm: 8.50 (s, 1H, CH), 7.59 (s, 2H, NH2), 4.90 (s, 3H, CH3). 1H NMR (Acetone-d6, 500 MHz), δ/ppm: 8.05 (s, 1H, CH), 7.13 (s, 2H, NH2), 4.45 (s, 3H, CH3). 13C NMR (DMSO-d6, 125.8 MHz), δ/ppm: 170.05 (C–S), 148.30 (C–NH2), 141.48 (C–NO2), 140.24 (C–Nimidazole), 133.18 (CH) and 35.07 (CH3). 13C NMR (Acetone-d6, 125.8 MHz), δ/ppm: 170.76 (C–S), 150.73 (C–NH2), 142.77 (C–NO2), 141.49 (C–Nimidazole), 133.45 (CH) and 35.71 (CH3). HR-ESI+-MS (m/z, assignment): 227.03453, [M + H]+ (calcd. 227.03512).
LH,Me (R1 = H; R2 = Me) – UPLC retention time: 2.24 minutes. Yield: 75.0% (180.2 mg) – Elemental Analysis Calculated for C7H8N6O2S (240.24 g mol−1): C: 35.0; H: 3.4; N: 35.0. Found: C: 34.8; H: 3.4; N: 35.1. IR (cm−1): 3193w υ(N−H), 3125w υ(C−H), 1583m δ(C−N) + υ(C
N), 1536s υ(C
N) + (C
C), 1523s υ(N−O)ass, 1362s υ(N−O)s, 1332s υ(C−S). 1H NMR (DMSO-d6, 500 MHz), δ/ppm: 8.30 (m, 1H, NH), 8.21 (s, 1H, CH), 4.33 (s, 3H, N–CH3imidazole) and 2.97 (d, J = 5 Hz, 3H, CH3). 13C NMR (DMSO-d6, 125.8 MHz), δ/ppm: 170.49 (C–S), 147.85 (C–NH), 141.42 (C–NO2), 140.30 (C–Nimidazole), 133.21 (CH), 35.06 (CH3imidazole) and 31.47 (NH-CH3). HR-ESI+-MS (m/z, assignment): 241.05023, [M + H]+ (calcd. 241.05022); 263.03217, [M + Na]+ (calcd. 263.03271).
LMe,Me (R1 = Me; R2 = Me) – UPLC retention time: 2.33 minutes. Yield: 50.4% (128.2 mg) - Elemental Analysis Calculated for C8H10N6O2S (254.27 g mol−1): C: 37.8; H: 4.0; N: 33.0. Found: C: 37.6; H: 4.1; N: 32.8. IR (cm−1): 3148w υ(C–H), 1555s υ(C
N) + (C
C), 1513s υ(N−O)as, 1397s υ(N−O)ass, 1362s υ(C−S). 1H NMR (DMSO-d6, 500 MHz), δ/ppm: 8.22 (s, 1H, CH), 4.33 (s, 3H, CH3) and 3.19 (s, 6H, N(CH3)2). 13C NMR (DMSO-d6, 125.8 MHz), δ/ppm: 172.54 (C–S), 148.82 (C–N(Me)2), 141.80 (C–NO2), 140.79 (C
Nimidazole), 133.71 (CH), 41.73 (N-(CH3)2) and 35.52 (CH3imidazole). HR-ESI+-MS (m/z, assignment): 255.06541, [M + H]+ (calcd. 255.06541).
Synthesis of the [ReBr(CO)3(LR1,R2)] complexes.
In a 25 mL reaction flask containing 4 mL of MeOH, 0.11 mmol (84.0 mg) of (Et4N)2[ReBr3(CO)3] and 0.1 mmol of the desired LR1,R2 ligand were added in sequence. The resulting solutions were kept under reflux. A reaction control was carried out by UPLC and the reaction was discontinued after full consumption of the ligands. After that, the solution was left at 0 °C for 72 hours. The red solids which precipitated were filtered, washed with n-hexane and dried under vacuum. The complex 1 was recrystallized from acetone and the complex 3 precipitated directly from the reaction mixture in the crystalline form.
[ReBr(CO)3(LH,H)]·C3H6O (R1 = H; R2 = H) 1·C3H6O – Reaction time 1 hour. Yield: 74.0% (46.9 mg). Elemental Analysis Calculated for C9H6BrN6O5ReS·C3H6O (634.44 g mol−1): C: 22.7; H: 1.9; N: 13.3; Found: C: 22.5; H: 1.9; N: 13.4. IR (cm−1): 3407m and 3236m υ(N−H), 2031s, 1955s, 1906s υ(C
O), 1597 δ(C−N) + (C
N), 1528s υ(C
N) + (C
C), 1497s υ(N−O)as, 1384s υ(N−O)ass, 1282s υ(C−S). 1H NMR (Acetone-d6, 500 MHz) δ/ppm: 8.63 (s, 1H, CH), 7.99 (s, 2H, NH2), 4.45 (s, 3H, CH3). 13C NMR (Acetone-d6, 500 MHz), δ/ppm: 197.35 (CO), 188.46 (CO), 172.20 (CO), 172.14 (CS), 147.96 (C–NH2), 147.61 (C–NO2), 141.05 (C–Nimidazole), 133.98 (CH), 36.39 (CH3). HR-ESI+-MS (m/z, assignment): 496.96803, [M − Br]+ (calcd. 496.96724); 514.97822, [(M–Br) + H2O]+ (calcd. 514.97781); 598.87403, [M + Na]+ (calcd. 598.87535).
[ReBr(CO)3(LH,Me)] (R1 = H; R2 = Me) 2 – Reaction time 1 hour and 30 minutes. Yield: 76.3% (47.5 mg). Elemental Analysis Calculated for C10H8BrN6O5ReS (590.39 g mol−1): C: 20.2; H: 1.4; N: 14.2. Found: C: 20.4; H: 1.5; N: 14.2. IR (cm−1): 3216m υ(N−H), 2033s, 1931s and 1911s υ(C
O), 1556s δ(C−N) + (C
N), 1529s υ(C
N) + (C
C), 1503s υ(N−O)as, 1372s υ(N−O)ass, 1280s υ(C−S). 1H NMR (Acetone-d6, 500 MHz) δ/ppm: 8.63 (s, 1H, CH), 8.23 (s, 1H, NH), 4.45 (s, 3H, CH3imidazole), 3.24 (s, 3H, CH3thiadiazole). 13C NMR (Acetone-d6, 500 MHz), δ/ppm: 197.09 (CO), 188.44 (CO), 172.92 (CS), 147.88 (C–NH), 146.31 (C–NO2), 140.91 (C–Nimidazole), 133.89 (CH), 36.24 (CH3imidazole) and 32.84 (CH3thiadiazole). HR-ESI+-MS (m/z, assignment): 510.98239, [M − Br]+ (calcd. 510.98289); 528.99249, [(M–Br) + H2O]+ (calcd. 528.99346); 612.88849, [M + Na]+ (calcd. 612.89100).
[ReBr(CO)3(LMe,Me)]·H2O (R1 = Me; R2 = Me) 3·H2O – Reaction time 3 hours. Yield: 83.2% (51.8 mg). Elemental Analysis Calculated for C11H10BrN6O5ReS·H2O (622.42 g mol−1): C: 21.2; H: 1.9; N: 13.5. Found: C: 21.4; H: 1.9; N: 13.6. IR (cm−1): 2024s, 1922s and 1886s υ(C
O), 1578s υ(C
N) + (C
C), 1531s υ(N–O)as, 1374s υ(N–O)ass, 1309s υ(C–S). 1H NMR (Acetone-d6, 500 MHz) δ/ppm: 8.62 (s, 1H, CH), 4.47 (s, 3H, CH3imidazole), 3.40 (s, 6H, N(CH3)2). 13C NMR (Acetone-d6, 500 MHz), δ/ppm: 197.13 (CO), 188.42 (CO), 173.79 (CS), 147.90 (C–Nthiadiazole), 146.00 (C–NO2), 140.89 (C–Nimidazole), 133.91 (CH), 41.88 (N(CH3)2), 36.22 (CH3imidazole). HR-ESI+-MS (m/z, assignment): 524.99858, [M − Br]+ (calcd. 524.99854); 626.90403, [M + Na]+ (calcd. 626.90665).
Radiolabeling with 99mTc
First, the solution of [99mTc(H2O)3(CO)3]+ had its pH adjusted using a 1 M solution of HCl (for reactions with the ligands LH,H and LH,Me the pH was adjusted to 4 and with the ligand LMe,Me to 2). Subsequently, 0.9 mL of [99mTc(H2O)3(CO)3]+ and 0.6 mL of 1 mM LR1,R2 ethanolic solutions were added to a 1–2 mL sealed biotage flask. The resulting solutions were heated in microwave radiation at 100 °C for 1 hour and then analyzed by radio-HPLC. The retention times for [99mTc(H2O)(CO)3(LH,H)]+ (4), [99mTc(H2O)(CO)3(LH,Me)]+ (5) and [99mTc(H2O)(CO)3(LMe,Me)]+ (6) were 19.20, 19.83 and 21.03 minutes, respectively.
99mTc experiments.
Caution! 99mTc is a γ-emitter (E = 140 keV, t1/2 = 6.02 h) which should only be handled by trained personnel in a licensed and appropriately shielded facility.
Crystal structure determinations
Single-crystals adequate for the crystallographic study were obtained for TscH,Me, TscMe,Me, LH,H, LMe,Me, [ReBr(CO)3(LH,H)]·C3H6O and [ReBr(CO)3(LMe,Me)]. The single crystals were collected at 160(1) K on the following diffractometers: (I) Rigaku OD XtaLAB Synergy, Dualflex, Pilatus 200 K for LH,H, LH,Me and [ReBr(CO)3(LH,H)]·C3H6O; (II) Rigaku OD SuperNova/Atlas for the compound TscH,Me; and (III) RIGAKU OD Xcalibur (Ruby CCD detector) for TscMe,Me and [ReBr(CO)3(LMe,Me)], using Cu Kα radiation (λ = 1.54184 Å) or Mo Kα radiation (λ = 0.71073 Å) from a micro-focus sealed X-ray tube and Oxford liquid-nitrogen Cryostream cooling system.20 The selected single crystals were mounted using polybutene oil into a flexible loop fixed on a goniometer head and immediately transferred to the diffractometer. The pre-experiment, data collection, data reduction and analytical absorption correction21 were carried out using the program suite CrysAlisPro.22 Using Olex2,23 the structures were resolved with the “small molecule structure solution” program SHELXT24 and refined with the SHELXL2018/325 program package by full-matrix least-squares minimization on F2. PLATON26 was used to check the results of X-ray analyses. More details on data collection and refining parameters can be found in Table S1.† Additional information on the structure determinations has been deposited with the Cambridge Crystallographic Data Centre. The ellipsoid representations were prepared with DIAMOND27 and the H-bond plots with Mercury.28
Biological studies
Evaluation of the cytotoxicity on LLC-MK2 cells.
The MTT (3-(4,5-Dimethylthiazol-2-yl)-2,5-Diphenyltetrazolium Bromide) test was used to evaluate the cytotoxic effects of the compounds.29 Mammalian cells (LLC-MK2) were grown in RPMI-1640 media. The amount of 1.0 × 105 cells per well were added to the 96-well microplate in the absence or presence of compounds or benznidazole, diluted in series at base 2 (500 to 1.95 μM) and incubated at 37 °C with 5% CO2 for 72 hours. At the end of the incubation period, 50 μL of MTT (Sigma-Aldrich Corp. St Louis, MO) at 5 mg mL−1 was added, and after 3 hours of incubation 50 μL of DMSO at the percentage of 0.5% was added to dissolve the crystals of formazan blue. The absorbance at 570 nm was acquired using microplate spectrophotometer Stat Fax 2100 (Awareness Technology, Palm City, FL, USA). The percentage of cell viability was determined from the following formula: Cell viability (%) = (treatment absorption)/(negative control absorption) × 100. The value of IC50 was determined by the dose response curve using the statistical program GraphPad Prism 5 for Windows (GraphPad Software, San Diego, CA, USA).
In vitro Evaluation of the trypanocidal activity on amastigote form.
The tests were performed as previously described.30 The trypomastigote and amastigote forms of the Tulahuen LacZ strain were obtained according to the procedure of Buckner and collaborators (1996).31 On a 96-wells plate, 80 μL of the LLC-MK2 cells at a concentration of 5 × 104 cells per mL were added. The trypomastigote forms of the Tulahuen LacZ strain were added at a concentration of 5 × 105 cells per mL (20 μL) and incubated at 37 °C at 5% CO2 for 24 hours. Subsequently, the trypomastigote forms present in the supernatant were removed by successive washing and only the amastigote forms remained. The compounds were dissolved in DMSO (maximum percentage of 5%) and added at different concentrations (250 to 0.093 μM- serial dilutions) and incubated in culture for 72 hours. At the end the CPRG substrate (red chlorophenol β-D-galactopyranoside, 400 μM at 0.3% Triton X-100, pH 7.4) was added. After 4 hours at 37 °C, the absorbance at 570 nm was acquired. Benznidazole at the same concentrations as the evaluated the positive control and the solvent used to solubilize the compounds the negative control.
Enzymatic assays
Expression and purification of TcOYE.
The recombinant Old Yellow Enzyme of T. cruzi (TcOYE) was produced using the methodology described by Murakami et al.32 The protein was expressed in the strain Escherichia coli BL21(DE3) and the pET28a::TcOYE was used as the vector and IPTG as inducer. The enzyme was purified by nickel affinity. After affinity purification, the polyhistidine tag was removed by overnight incubation with thrombin and a second stage of purifying was carried out by preparatory size exclusion chromatography (SEC) using a 25 mmol L−1 Tris–HCl buffer (pH 8.0) containing 100 mmol L−1 NaCl as eluent.
Suppression of the intrinsic fluorescence of tryptophan.
Quenching of fluorescence emission experiments were carried out by following the decay of the maximum intensity of the fluorescence spectra of TcOYE (1 μmol L−1) by the presence of increasing concentration of the compounds benznidazole (Bzn), megazol (LH,H) and [ReBr(CO)3(LH,H)] as previously reported.30,33 The spectra were collected using a Hitach F-4500 spectrofluorometer with λex = 295 nm and emission and excitation slit bandwidth of 5.0 nm. The experiments were performed at 25 mmol L−1 Tris–HCl buffer (pH 8.0) containing 100 mmol L−1 NaCl. The stock solutions of ligands were prepared in DMSO, with final concentration of 2.0% in all samples. The binding parameters were determined by the Hill plot (eqn (1)),34 obtaining the dissociation constant (KD) by non-linear fitting of the saturation curve to experimental data and with n = 1. KD was also obtained by double-log equation using the relation 1/KB (eqn (2)).35 The double-log plot was used to determine the binding constant (KB) and the stoichiometric number (n). It also allows the calculation of the KD from 1/KB. The fluorescence quenching data were analyzed by the Stern–Volmer plot at different temperatures (eqn (3));36 where, F0 is fluorescence in the absence of any ligand; [L] is the ligand concentration; [P0] is protein concentration. | 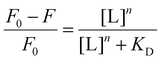 | (1) |
|  | (2) |
| 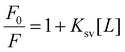 | (3) |
The methodology used for the determination of KD values assumes the formation of the protein-binding complex which results in the suppression of fluorescence when compared to the protein in the absence of the ligand. When the ligand presents absorption bands that overlap with the fluorescent emission or excitation wavelengths, or both, it may have an impact on the fluorescence suppression.37 This interference is called the inner filter effect, which can be minimized using the procedure described by eqn (4),37 where Fobs and Fcor are the fluorescence intensities at 336 nm measured and corrected, respectively, and l represents the optical step where the absorption and emission of light occurs and C0 is the concentration of the compound. For the correction of the internal filter effect, the values of the molar absorption coefficient determined by the technique of molecular absorption spectroscopy in the region of the ultraviolet and visible (UV-vis) at the excitation (295 nm) and emission (336 nm) wavelengths of Benznidazole (εex = 1855 M−1 cm−1 and εem = 6319 M−1 cm−1), Megazol (LH,H) (εex = 15
335 M−1 cm−1 and εem = 8207 M−1 cm−1) and [ReBr(CO)3(LH,H)] (εex = 13
250 M−1 cm−1 and εem = 6803 M−1 cm−1).
| Fcor = Fobse−2.303(εex+εem)IC0 | (4) |
Theoretical study of molecular docking at TcOYE.
Molecular docking simulations of the compounds were carried out to explore the binding modes in the enzyme TcOYE. The crystallographic X-ray diffraction data of the TcOYE were extracted from the Protein Data Bank (PDB, code 4E2B).32,38 The molecular structures of megazol (LH,H) and [ReBr(CO)3LH,H] and benznidazole (CCDC number: 680687), determined by crystallographic methods, were used in docking studies. Docking simulations of the compounds were carried out with GOLD (Genetic Optimization for Ligand Docking) package version 5.5 on the active site of the enzyme. Interactions with the compounds were verified with the Mercury software of the GOLD package. Pre- and post-docking visualization and interactive docking configuration were carried out with the Hermes software of the GOLD package. All water molecules and heteroatoms were removed from the PDB input file. 6.0 Å around the native inhibitor was used as the cavity site of the compounds and the FMN prosthetic group inside the native structure. Another set of simulations with a cut point of 10.0 Å was executed to verify convergence. Genetic algorithm (GA) was used to perform the calculations,39 total flexibility was allowed to compound and GA executions were carried out with a maximum of 100
000 GA operations with a population size of 100 individuals. Various solutions were generated, the corners of the rings were able to rotate, various conformations were explored and no restriction was applied in coupling simulations. Simulations of redocking of the native FMN prosthetic group of PDB code 4E2B were executed on the active site of the enzyme with all the GOLD score functions available and the best score (the lowest rmsd relative to the crystalline structure conformation obtained by DRX) was found with the GoldScore suitability function.39 Thus, the Goldscore matching function was chosen to predict the mode of binding the compound in study with the TcOYE crystallographic structure. The GoldScore suitability rating function was evaluated and the three best poses, best-rated structures, of the compounds were selected for the interaction analysis. The three-dimensional figures of the complex were prepared with PyMOL (Molecular Graphics System, version 1.8 Schrödinger, LLC) and the two-dimensional analyses were submitted to the PoseView web server,40 and to the LigPlot software.41
Results and discussion
Synthesis of megazol its derivatives
Megazol, initially synthesized by Berkelhammer and Asato,42 has undergone various synthesis methodologies since its discovery in the 1960s.43–45 Chauvière et al. (2003) were the first to revisit the synthesis of megazol, comparing the conventional method with a new synthesis approach.43 In the conventional method, the first step involves oxidizing the corresponding alcohol into an aldehyde, which is then utilized in the preparation of the thiosemicarbazone. Megazol is ultimately obtained through an oxidative cyclization reaction in the presence of ferric ions, achieving a yield of 40%.43
In contrast, the new methodology proposed by Chauvière et al. employs the corresponding carbonitrile compound as a precursor instead of the aldehyde. Finally, a condensation with thiosemicarbazide in trifluoroacetic acid, further cyclization followed by loss of ammonia, and isomerization lead to megazol. This alternative approach offers a yield of 50% at this stage. Herein, we propose to use the Microwave Assisted Organic Synthesis (MAOS) to improve the synthesis of megazol, aiming to reduce reaction time and increase the yield.46,47 To optimize the synthesis, we varied both the reaction time and temperature, ultimately identifying the optimal conditions as 1 hour and 40 minutes at 80 °C (Scheme 1). Following the reaction, a yellow raw product was isolated by filtration and promptly dissolved in acetone for recrystallization. This optimization process resulted in a significant increase in the reaction yield, achieving 70% of the desired product. Additionally, to investigate the structure/activity relationship, we introduced structural modifications to the amine group attached to the 1,3,4-thiadiazole ring using different thiosemicarbazides. These modifications led to the synthesis of the intended products consistently in good yields. The TscR1,R2 precursors and the LR1,R2 compounds were analysed by UPLC, 1H NMR and HR-ESI-MS; was additional proof for the identity of the LR1,R2 products was given by 13C NMR and FTIR (see ESI† for details).
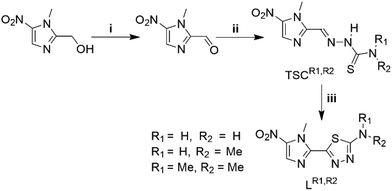 |
| Scheme 1 Synthesis of megazol (LH,H) and its derivatives (LR1,R2: R1 = H, R2 = Me and R1 = R2 = Me). (i) method 1: toluene, MnO2, reflux, 4 h; method 2: DCM, DMP, 0 °C, 20 h; (ii) EtOH, thiosemicarbazide, HCl, m.w. 80 °C, 40 min; (iii) H2O, NH4Fe(SO4)2·12H2O (4 equiv.), m.w. 80 °C, 90 min. | |
The 1H NMR spectra of the TscR1,R2 and LR1,R2 compounds show the signals in the expected regions (see ESI†). Table S2† contains the NMR data for the obtained organic compounds. Two characteristic signals are given by methyl group and by the hydrogen atom attached to the imidazole ring which appear as singlet peaks in the 4.15–4.90 ppm and 8.1–8.5 ppm regions, respectively. The signals of the two NH protons, observed respectively at 11.8, 11.9 and 11.3 ppm for TscH,H, TscH,Me and TscMe,Me, are expectedly not observed in the 1H NMR spectra of LR1,R2. Furthermore, the signals related to the NH2 bonded to the thioamide portion of LH,H is not observed. This fact is consistent with the effect of the quadrupolar moment of the nitrogen isotope (14N: I = 1) which affects the intermolecular exchange rate of the protons attached to the nitrogen atom in solution.48,49 The 1H NMR spectra of TscH,Me and LH,Me present two broad signals around 3.0 ppm and 8.3 ppm assigned to the –CH3 and –NH end groups, respectively. This enlargement occurs due to the lower exchange rate compared to the NH2 in TscH,H and LH,H. The 13C NMR spectra (ESI†) show the expected signals in the appropriate regions. The DEPT 90 experiment was applied in order to confirm the CH and methyl signals at approximately 133 and 35 ppm. The quaternary carbon atoms of the thiadiazole ring of LR1,R2 resonances at 170 and 148 ppm. Table S3† shows the attribution of the other peaks.
The IR spectra of the LH,H and LH,Me compounds (see ESI†) reveal broad, strong absorptions in the range between 3428 and 3193 cm−1 of the NH stretches, which is expectedly not present in the spectrum of LMe,Me. Medium intensity absorptions in the region between 1632 and 1555 cm−1, assigned to the C
N vibrations, are also found in the spectra of the LR1,R2 compounds. The presence of the −NO2 group is characterized by the νass(N–O) and νsym(N–O) bands at around 1500 cm−1 and 1365 cm−1, respectively. Table S4† summarizes the main absorption bands in the infrared region for the LR1,R2 compounds. Furthermore, the ESI + MS spectra of the organic compounds show exclusively the peaks of the [M + H]+, [M + Na]+ or [M + K]+ molecular ions (see ESI†).
TscH,Me, TscMe,Me, LH,H and LMe,Me were studied by X-ray analysis. Fig. 2 illustrates the molecular structures of the organic compounds. Selected bond lengths and angles are shown in Table S5.† The bonding arrangement of the TscH,Me and TscMe,Me skeleton is consistent with C5–N4 bond lengths of 1.288/1.291 Å, which can be assigned to a C
N double bond, and C6–N5 distances of 1.372/1.379 Å, which is typical for C–N single bonds. The C6–S1 bond lengths has a double bond character (1.681/1.683 Å) in the TscH,Me and TscMe,Me and a single bond character upon formation of the 1,3,4-thiadiazole ring of LH,H and LMe,Me (1.734/1.740 Å). The N1–O1 and N1–O2 bond lengths for all structurally determined compounds are around 1.23 Å. The protonation of the nitrogen atom N5 in the molecular structure of TscH,Me and TscMe,Me is experimentally justified by the detection of peaks of electron density in the Fourier map, which can be assigned to corresponding hydrogen atoms, and the fact that it is involved into H-bonds (see Fig. S4.1–4.4† for hydrogen bonding plots), whereas it is expectedly not observed in the crystal structures of LH,H and LMe,Me.
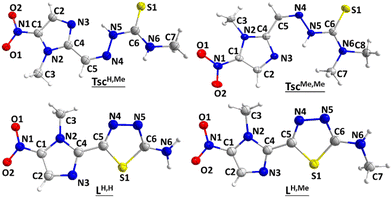 |
| Fig. 2 ORTEP representation of the molecular structures of TscH,Me, TscMe,Me, LH,H (megazol) and LH,Me (thermal ellipsoids with a 50% probability). | |
Rhenium(I) tricarbonyl complexes
Reactions of (Et4N)2[ReBr3(CO)3] with LR1,R2 in methanol under reflux (Scheme 2) affords red solids of general composition [ReBr(CO)3(LR1,R2)]. 1.1 equivalent of the metal precursor was used in order to ensure the complete consumption of the ligands. The reaction was followed by analytical UPLC and stopped at the time that the entire ligand was consumed. The complexes are soluble in methanol, ethanol, acetone, acetonitrile, dichloromethane, DMSO and DMF, and are poorly soluble in water.
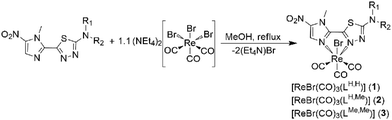 |
| Scheme 2 Synthesis of the rhenium(I) complexes [ReBr(CO)3(LR1,R2)]. | |
The chemical composition of the 1–3 complexes was determined by both CHN elemental analysis and HR-ESI-MS mass spectrometry. Both techniques have certified the previously proposed structures. The chemical element Re has two natural isotopes (abundances: 62.6% for 187Re and 37.4% for 185Re), consequently, the peaks related to the rhenium containing species in the mass spectra of the complexes are characterized by the abundances of the metal isotopes. The mass spectra (check the ESI†) present the of the molecular ion peaks as an adduct with Na+, [M + Na]+ with high intensity. In addition to the expected molecular ion peak, two other peaks were observed. The first related to the replacement of the bromido ligand by a water molecule [M − Br + H2O]+, and the second is just the peak of the ion without the bromido [M − Br]+.
The IR spectra of the 1–3 compounds (Fig. S2.4–2.6†) reveal very strong absorptions in the region between 1597 and 1530 cm−1, which can be assigned to the C
N vibrations. The spectra of the complexes 1 and 2 also present broad medium absorptions in the range between 3407 and 3216 cm−1 of the NH stretches, which are not observed for 3. The υas(N−O) and υs(N—O) bands do not undergo significant shifts when compared to the same bonds in the free ligands. The presence of the fac-Re(CO)3 moiety is confirmed by the observation of three bands in the range from 2030 to 1900 cm−1, characterizing the three CO facial ligands (Table S4†). In the metal precursor spectrum (Et4N)2[ReBr3(CO)3] the stretching band υ(CO) is observed between 1996 and 1847 cm−1.
The 1H NMR spectra (see ESI and Table S6†) of the rhenium complexes recorded in acetone solutions show the expected signals for the LR1,R2 ligands coordinated to the Re(CO)3+ fragment. The signals related to the CH and to the methyl group of the imidazole ring are observed around 8.63 and 4.45 ppm, respectively. The signals for the NH protons are found at 7.99 ppm for the complex 1 and at 8.23 ppm for the complex 2. The methyl groups bounded to the secondary and tertiary amines are observed at 3.24 ppm for 2 and at 3.40 ppm for 3. The 13C NMR spectra was also measured (see ESI†). The DEPT 90 and 135 techniques were applied in order to confirm the signals unambiguously (see Table S7†). The carbon signals related to the LR1,R2 ligands are found in two regions of the spectra, being the first in the 30–40 ppm range and the second between 130 and 170 ppm. The presence of the – ReICO3 fragment is evident due to the presence of signals between 190 and 200 ppm attributed to the carbonyl ligands which is in accordance with similar compounds found in the literature.50,51
The complexes 1 and 3 have been studied crystallographically. Selected bond lengths and angles are compared in Table S8.† The complexes 1 and 3 crystallized in orthorhombic crystalline system and spatial groups Pca21 (5) and Pbca (6), respectively. The representations of their molecular structures are shown in Fig. 3. The coordination around the metal center is described as distorted octahedral with the three carbonyl ligands occupying one face of the octahedron and the second one occupied by the bromido ligand and the N3 and N4 atoms of the LR1,R2 ligands. The octahedron distortion is evidenced by the C13–Re1–Br, C11–Re1–N3 and C12–Re1–N4 angles, with a deviation from the ideal angle of 180° in between 4 and 10°. This distortion is caused by the restriction caused by the formation of the five-member chelate ring upon coordination with the megazol-derived ligands. The Re1–Br1 distance is around 2.60 Å, which can be considered as a bond with the potential to be cleaved during reactions for substitution of the bromido ligand. The megazol derivatives in the neutral NN-bidentate mode, bonding situation slightly affected by the coordination to ReI in comparison with the free ligands. The CN bond lengths, in the range of 1.30 and 1.37 Å, are consistent with the double bonds even for the C6–N6 bond which is not inside the rings. The CO bond lengths are in the 1.10–1.14 Å range, with the CO trans to the bromido ligand having the shortest bond. The shortening of the C13–O13 bond compared to the other C
O ligands was well as the elongation of the Re1–C(13) coordinating trans to the chlorido ligand (the π-donor) does not reflect the expected π-backbonding effect. This fact has been observed for similar tricarbonyl rhenium(I) complexes.52,53
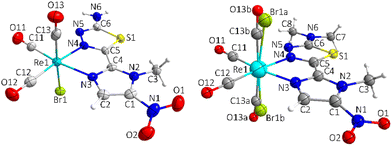 |
| Fig. 3 Ellipsoid representations of the complexes 1 (left) and 3 (right). The complex 1 has an acetone molecule in its asymmetric unit. The Br1, C13 and O13 atoms in the structure of 3 are disordered over two sets of positions (Part A with occupation factor of 83.6% and part B with occupation factor of 16.4%). | |
Biological assays
The antiparasitic activity of on nitroheterocyclic compounds is well-known. Particularly, the biological activity of megazol against T. cruzi parasites has been confirmed previously.43,54 However, its development as a drug was ceased due to genotoxic and mutagenic effects.55,56 In order to assess whether the modifications in the peripheral structure of megazol and its coordination to the Re(CO)3+ fragment have effects on the parasites we decided to investigate the compounds of the present work using the modified T. cruzi Tulahuen β-galactosidase strain. As described in the experimental part, the activity was evaluated on the amastigote form of T. cruzi inside the LLC-MK2 cells. Complementary and simultaneously to the trypanocidal assay, the cytotoxicity on LLC-MK2 cells was also performed in order to determine the selectivity of the compounds. A dose–response curve was established to determine IC50 of the compounds on the parasites and the CC50 on the LLC-MK2 cells by serial dilutions in the 250 μM – 0.093 μM range (see Fig. S5.1 and S5.2†). The results obtained are presented in Table 1.
Table 1 IC50 against Tulahuen β-galactosidase amastigotes, CC50 on LLCMK2 cells and SI obtained for the compounds, LH,H (megazol), LH,Me, LMe,Me, [ReBr(CO)3(LH,H)] (1), [ReBr(CO)3(LH,Me)] (2) and [ReBr(CO)3(LMe,Me)] (3). Bzn was used as reference drug
Compounds |
IC50 amastigotes (μM) |
CC50 (LLCMK2 cells) (μM) |
SI |
Bzn |
3.53 ± 0.02 |
376.5 ± 14.63 |
106.6 |
[ReBr3(CO)3]2− |
153 ± 3.32 |
179.3 ± 0.21 |
1.17 |
LH,H |
1.04 ± 0.08 |
117.9 ± 5.65 |
113.4 |
LH,Me |
1.25 ± 0.08 |
215.5 ± 28 |
172.4 |
LMe,Me |
1.8 ± 0.26 |
89.1 ± 5.89 |
49.5 |
1
|
0.35 ± 0.055 |
150.8 ± 14.9 |
430.8 |
2
|
1.24 ± 0.13 |
114.4 ± 7.84 |
92.2 |
3
|
5.61 ± 0.12 |
127.3 ± 10 |
22.7 |
In general, all megazol derivatives presented a higher trypanocidal activity than Bzn (IC50 = 3.53 μM). As expected, the (Et4N)2[ReBr3(CO)3] metal precursor was not effective in eliminating amastigote forms of T. cruzi (IC50 = 153 μM). LH,H (megazol) and its derivatives LH,Me and LMe,Me presented similar IC50 values. On the other hand, the mono-substituted compound LH,Me showed a lower cytotoxicity compared to LH,H, resulting in a better SI. Furthermore, the coordination of megazol to ReI and formation of the [ReBr(CO)3LH,H] complex increased the trypanocidal activity by approximately 3 times. Surprisingly, the cytotoxicity of the complex was lower than that of free LH,H, leading to a very high selectivity index (SI ≈ 431). The complex 2 exhibited a similar activity compared to its free ligand, while the complex 3 showed a lower activity. Therefore, lipophilicity was a determining factor for the activities of complexes, since the complex 3, the most ineffective, is the most hydrophobic compound in the series studied. Probably, the complex 1 can cross the cell membrane of the host cells to reach intracellular parasites more easily.
The international DNDi program uses the SI as a parameter for evaluating new drugs for the treatment of Chagas disease: a lead candidate for development of a trypanocidal drug present SI ≥ 100.57,58 Therefore, not only megazol but its derivative LH,Me and the rhenium complex 1 may be considered as lead candidates for trypanocidal drugs.
Interaction with TcOYE
Experimental studies.
The comprehension of the interaction of the compounds with biomolecules that play a role in the cell cycle is important for the rational development of drugs and new drug formulations.59 The identification of several biomolecules that are needed for survival of the etiological organisms responsible for the illnesses might be the start points for understanding the mechanism of action of new drugs. The Old Yellow Enzymes (OYEs), for example, are found in organisms such as bacteria, fungi, plants and protozoa. They have the flavin mononucleotide (FMN) as their prosthetic group which gives to the protein the yellow color and the name of this family of proteins. The OYEs mediates the transfer two electrons from reductants NAD(P)H to the final acceptors (substrates) such as cyclohexanone, duroquinone and menadione.60 The TcOYE consists of a NAD(P)H oxidoreductase that acts on the pathway of prostaglandin synthesis by converting H2 (PGH2) to F2α (PGF2α). The prosthetic group of TcOYE, FMN, is reduced to FMNH2 by the reducing agent NAD(P)H, turn into FMNH2, promotes the reduction of PGH2 to PGF2α by electron transfer. Prostaglandins are potent mediators of physiological and pathological responses, some of them observed in Chagas disease, but their function in T. cruzi is not well explained.61,62 Meah and Massey showed that OYE catalyzes the reduction of the nitro group of unsaturated nitroolefins and acts as an acid/base catalyst.63 In addition, TcOYE has been shown to be an important enzyme in the metabolism of drugs used in the treatment of Chagas disease as studies with different strains of T. cruzi show that their overexpression or absence is determining the susceptibility or resistance to benznidazole. This study verified that when copies of the TcOYE gene were deleted, the strains were resistant to the drug.64 For this reason, the interaction of the compounds Bzn, LH,H (megazol) and [ReBr(CO)3(LH,H)] with the TcOYE was studied in the present work.
The stock solutions of Bzn, LH,H and [ReBr(CO)3LH,H] (1) were prepared in DMSO. The final concentration of DMSO for all the compounds in the experiment was kept in 2.0%. The enzyme TcOYE was prepared in Tris–HCl buffer pH 8.0, 100 mmol L−1 of NaCl. The Fig. 4, S6.1 and S6.2† reports the results obtained from the fluorescence emission spectra of the intrinsic tryptophan probe (Fig. 4A, S6.1A and S6.2A†) in the presence of the compounds Bzn (Fig. S6.1†), LH,H (Fig. S6.2†) and the metal complex [ReBr(CO)3(LH,H)] (Fig. 4). All tested compounds showed saturation profiles with increased concentrations of Bzn, LH,H and [ReBr(CO)3LH,H], which allowed adjustment to the Hill equation for determining the KD, Fig. 4B, S6.1B and S6.2B.† The Stern–Volmer ratio allows the kinetic study of the photophysical deactivation of the fluorophore to be carried out. The nature of this deactivation can be static or dynamic.35 Static deactivation occurs when the interaction results in the formation of the protein–ligand complex which is reflected in decreased fluorescence intensities. Fluorescence suppression can also be the result of deactivation by the shock between the molecules and this phenomenon is called dynamic suppression, which can occur along with static supposition when in the presence of high concentrations of the free compound and is favored by the increase in temperature.35Fig. 4D, S6.1D and S6.2D† shows that the increase in temperature does not result in an increase in the suppression rate suggesting that nature of the interaction between the tested compounds and the TcOYE being static.34
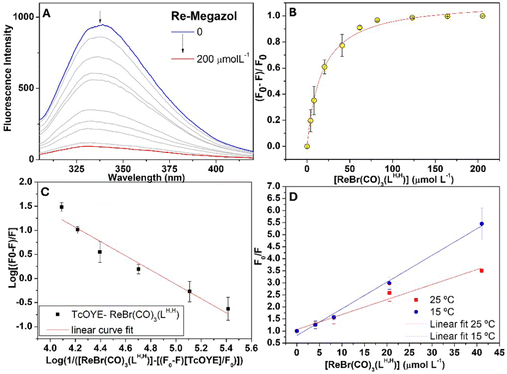 |
| Fig. 4 (A) Emission fluorescence spectra of TcOYE (1 μmol L−1) in the presence of [ReBr(CO)3(LH,H)] (0–200 μmol L−1), (B) Hill plot with fixed n = 1 at 25 °C. (C) Double-log plot relation and linear fit indicating n of about 1. (D) Stern–Volmer plot at 15 °C and 25 °C indicating a static quenching mechanism. The experiments were performed in Tris–HCl buffer pH 8.0, 100 mmol L−1 of NaCl and 2.0% de DMSO. | |
Table 2 shows that the compounds presented KD in the μM order. Megazol as well as its rhenium complex showed greater affinity for TcOYE when compared to Bzn. The interaction of the megazol containing metal complex showed similar KD values which indicates that the presence of the metal did not significantly affect the ability of the molecule to interact with the protein.
Table 2 Dissociation constant (KD), number of binding sites (n), association constant (KB), and Stern–Volmer constant (Ksv) for the interaction of TcOYE with Bzn, megazol (LH,H) and [ReBr(CO)3 (LH,H)]. Experiments were carried out in Tris–HCl buffer pH 8.0, NaCl 100 mM
Parameters |
Compounds |
LH,H |
1
|
Bzn |
Hill Plot (n = 1), eqn (1).
Stern–Volmer, eqn (2).
Double log, eqn (3), where KDc = 1/KBc.
|
n
|
1.2 ± 0.1 |
1.4 ± 0.2 |
1.1 ± 0.1 |
K
D
(μmol L−1) |
18 ± 2 |
17 ± 1 |
26 ± 3 |
K
B
× 104 (L mol−1) |
8.6 ± 0.5 |
8.3 ± 0.8 |
5.0 ± 0.6 |
K
D
(μmol L−1) |
12 ± 2 |
12 ± 1 |
21 ± 2 |
K
sv
× 104 (L mol−1) at 25 °C |
8.6 ± 0.6 |
1.1 ± 0.5 |
2.7 ± 0.2 |
K
sv
× 104 (L mol−1) at 15 °C |
9.7 ± 0.6 |
6.2 ± 0.5 |
4.3 ± 0.1 |
Theoretical studies.
As discussed above, the experimental assays with TcOYE have shown that the coordination of LH,H (megazol) to the ReI center does not significantly alter the interaction with this protein since both present similar KD values. In this context, the molecular docking of these compounds was carried out in order to better understand binding mode with TcOYE. Additionally, for comparison, docking was also performed with the standard drug, benznidazole. The best poses, obtained by the GOLD package ranking function, are shown in Fig. 5 and S6.3.† It is possible to see that megazol and its complex, as well as benznidazole, fit well on top of the FMN prosthetic group (Fig. 5). It is interesting to mention that the cavity of TcOYE is large and that the compounds studied here are comparatively small, which should bring the possibility of accommodation anywhere in the cavity. However, the compounds ended up bonding well at the bottom of the cavity funnel near the prosthetic group, suggesting that they might have an affinity with the enzymatic site of TcOYE. All compounds interacted with a large number of hydrophobic interactions (Fig. S6.3A†) and formed a π–π stacking bond with Phe71 (Fig. S6.3B†). Furthermore, megazol and the rhenium complex were well buried in the binding site with the -NO2 group facing the cavity, however, only megazol formed a hydrogen bond with His195. It's worth to highlight that the His195 residue has importance in hydride transfer reactions with α,β-unsaturated enones and in the reduction of PGH2 to PGF2α of prostaglandins.62,65
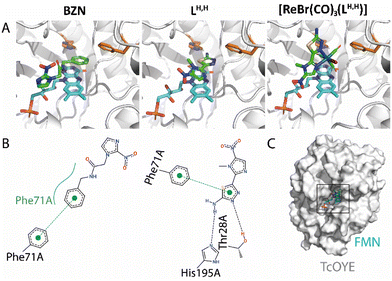 |
| Fig. 5 Molecular interactions of the ternary complex TcOYE/FMN/Compound. (A) and (C) Three-dimensional representation of the first-ranked structural poses of each compound (green) bound in the TcOYE cavity (grey cartoon) containing the prosthetic group FMN (cyan). (B) Two-dimensional projection of the compounds interacting with TcOYE/FMN. Hydrogen bonds are shown in dashed lines, hydrophobic interactions are represented in green contours and π-π interactions are displayed in green circle-dashed lines. Interactions of [ReBr(CO)3(LH,H)] with the enzyme are not shown. | |
In previous works of our research group,30,66 docking simulations showed that FMN N5 nitrogen atom and His195 remained about 3 Å apart from the inhibitors [AuCl(L1)] (L1 = diacetylmonooxime-derived thiosemicarbazone) and HFedtc (S-benzyl-N-(ferrocenyl)methylenodithiocarbazate), a distance suitable for hydride transfer, as presented by the compounds studied here with the hydrogen bond interactions. However, the mechanisms of inhibition for these two previously published compounds seems to be different, while [AuCl(L1)] is processed by TcOYE by receiving electrons from it, HFedtc supposedly acts as a competitive inhibitor by preventing menadione (a classic inhibitor of TcOYE) from binding to the active site of the enzyme. For the compounds studied in this work, it's possible to affirm that both mechanisms are plausible since the compounds were docked close to FMN within an interaction distance favouring hydride transfer.65,67 Moreover, both theoretical and experimental studies involving the enzymatic activity of TcOYE are necessary to better understand the inhibitory mechanism of megazol and its rhenium-derived complexes.
Radiolabeling with 99mTc
The structural elucidation of the ligands and their Re complexes was essential for the radiolabeling of the megazol derivatives with 99mTc since Re complexes have been employed as standards in the characterization of 99mTc complexes. Once the characterization of Re complexes were successfully carried out, the next step was the radiolabeling of megazol derivatives with 99mTc. The first stage of all reactions with 99mTc is the preparation of the precursor complex, in this case, [99mTc(H2O)3(CO)3]+. The kit is prepared in a single step, starting from [TcO4]−, in which boranocarbonate acts both as a reducing agent and as a source of carbon monoxide in a physiological environment.19 The next step of the reactions was the radiolabeling with the 99mTc(CO)3+ fragment. The reactions were carried out by varying the temperature, reaction time, concentration of the ligand and the pH of the reaction solutions.
The first reactions were carried out in a 2 mL reaction flask containing 0.9 mL of the [99mTc(H2O)3(CO)3]+ solution, to which 0.5 mL of each LR1,R2 ligand (1 mM in EtOH) was added and the solution and heated under microwave at 100 °C for 1 hour. The reactions were characterized by radio-HPLC, however, the formation of various products was observed in the chromatograms at basic pH (Fig. S7.1†). Subsequently, new reactions changing the pH of the solution were performed. Despite these details, these reactions have shown that megazol and its derivatives can be labeled.
The solutions of [99mTc(H2O)3(CO)3]+ were then neutralized using concentrated hydrochloric acid until the excess boranocarbonate was consumed to reach the optimum pH for the reaction with the LR1,R2 ligands. The optimal conditions for the reactions were then obtained as follows: 0.9 mL of the complex [99mTc(H2O)3(CO)3]+ and 0.5 mL of the ligand solution (1 mM in EtOH) at 100 °C for 1 hour (Scheme 3). Heating and microwave radiation did not result in different products for all cases. However, due to the sealing facility of the biotage type bottles as well as the stability in the volume of the solutions, the microwave radiation heating was employed in these studies. Differently from the rhenium derivatives, cationic complexes of composition [99mTc(H2O)(CO)3(LR1,R2)]+ were expected to be obtained (Scheme 3).
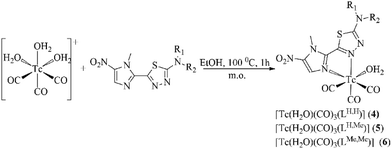 |
| Scheme 3 Megazol and derivatives radiolabeling reactions. | |
The reaction chromatograms showed the importance of the pH of the solution for the formation of a single product (Fig. S7.2 and 7.3†). Probably, the N(R1R2) group of LR1,R2 is protonated in acid medium, blocking the coordination of the amino group to the – Tc(CO)3+ fragment and favoring the formation of a single product. At basic pH, the electron pair of the nitrogen group N(R1R2) is available and might be able to coordinate with Tc by replacing the H2O molecule, for example. The yields of the reactions with LH,H, LH,Me and LMe,Me according to radio-HPLC were 45, 48 and 14%, respectively. The lower yields with LMe,Me is explained by the low solubility of the compound in water (r.t. 22.07 minutes, Fig. S7.4†) compared to LH,H (r.t.18.10 minutes, Fig. S7.5†).
For the full characterization, LH,H and its respective complex have been chosen as models. The unconventional chemistry of 99mTc complexes involves co-injection technique. For radio-HPLC, the method used in these studies, the non-radioactive compound of Na[ReO4] mixed with the saline solution of Na [99mTcO4] (Fig. S7.6†) was used as reference. The solution is injected into the radio-HPLC and, after the solution passes through the equipment column, its mobile phase passes then through the UV detector and finally through the γ detector. The difference in retention times between the cold and hot compounds was used as a reference to characterize the 99mTc complexes.
The chromatogram generated by the γ presented a peak with a retention time of 18.93 minutes attributed to the reaction product, Fig. 6. The chromatogram generated by the UV detector showed a peak from the LH,H and the reference cold product, [ReBr(CO)3(LH,H)], with retention times of 18.03 minutes and 19.20 minutes, respectively (Fig. 6B). However, the difference in the retention time of the radioactive complex and the cold reference complex was 0.27 minutes, stating that the rhenium complex used as a reference is not homologous to the new 99mTc complex. However, this fact was already expected due to the charge difference between the Re and 99mTc complexes, leading to a different lipophilicity and retention times. Finally, it is possible to assert that the megazol labeling with 99mTc was successfully.
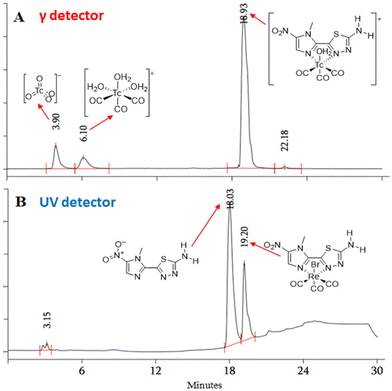 |
| Fig. 6 Co-injection chromatograms for (A) ([99mTc(H2O)(CO)3(LH,H)]+) and (B) non-radioactive (LH,H and [ReBr(CO)3(LH,H)]). | |
Conclusions
The synthesis of megazol has been explored by different methodologies through which it was also possible to perform modifications in its peripheral structure. The advantage of the new synthetic route was the increased yield together with a lower reaction time surpassing problems reported for other methods. Furthermore, to our knowledge, this is the first work that explores megazol in coordination chemistry. Various spectroscopic and spectrometric techniques have been used in the characterization of the compounds. Additionally, the crystal structure of most of them was determined. It was possible to verify that megazol and its derivatives formed octahedral complexes of composition [ReBr(CO)3(LR1,R2)], confirming their coordination mode as a neutral bidentate ligands.
With regard to biological activity, all compounds exhibited high selectivity for the Chagas disease parasite, with some compounds demonstrating selectivity indexes surpassing that of the reference drug, benznidazole. Experimental and molecular docking interaction studies with the TcOYE enzyme revealed better interactions for megazol and its Re(I) complex compared to benznidazole, indicating this enzyme as a potential target for these compounds. Furthermore, the megazol derivatives were successfully radiolabeled with 99mTc, although a time difference was noted between the rhenium standards and the 99mTc compounds. Although there is a need for improvements in the chelation ability of the megazol ligands, this study suggests that the ReI and 99mTcI complexes with megazol represent a concrete possibility for the development of a theranostic device for simultaneous treatment and diagnosis of Chagas disease. In vivo biological studies with both rhenium and 99mTc compounds will also help to better understand the potential of these compounds.
Author contributions
Conceptualization, J.C.B., R.J.O., S.A., C.D.L., R.A., and P.I.S.M.; methodology, A.C.R.G., S.H.L., R.J.O., C.G., O.B., C.D.L., R.A. and P.I.S.M.; validation, A.C.R.G., S.H.L., R.J.O., C.G., O.B. and C.D.L.; formal analysis, J.C.B., R.J.O., S.A., C.D.L., R.A., and P.I.S.M.; investigation A.C.R.G., S.H.L., R.J.O., C.G., O.B. and C.D.L.; resources, J.C.B., R.J.O., S.A., C.D.L., R.A. and P.I.S.M.; data curation, A.C.R.G., S.H.L., S.A., C.D.L., R.A., and P.I.S.M.; writing – original draft preparation, A.C.R.G. and P.I.S.M.; writing – review and editing, A.C.R.G., S.H.L., J.C.B., R.J.O., O.B., S.A., C.D.L., R.A. and P.I.S.M.; visualization, P.I.S.M.; supervision, J.C.B., S.A., R.A. and P.I.S.M.; project administration, P.I.S.M.; funding acquisition, J.C.B., S.A., C.D.L., R.A., P.I.S.M. All authors have read and agreed to the published version of the manuscript.
Data availability
The data supporting this article have been included as part of the ESI.† Crystallographic data for TscH,Me, TscMe,Me, LH,H, LH,Me, 1·C3H6O and 3 has been deposited at the CCDC under 2366616–2366621 and can be obtained from https://www.ccdc.cam.ac.uk.
Conflicts of interest
There are no conflicts to declare.
Acknowledgements
This research was funded by the CNPq: (grants: 408926/2021-0, 311747/2023-0, 402495/2022-5, 307992/2022-5, 310927/2021-8 and 309145/2020-1), FAPESP (grants: 2018/05576-1 and 2017/07335-9) and FAPEMIG (Grant: APQ-00834-24). Ana C. R. Gonçalves would like to thank Coordenação de Aperfeiçoamento de Pessoal de Nível Superior (CAPES) PDSE Nº 47 /2017 (Grant: 88881.188912/2018-01) for the PhD scholarship. We acknowledge the Núcleo de Computação Científica da Universidade do Estado de São Paulo (NCC/GridUnesp) and the Centro Nacional de Processamento de Alto Desempenho in São Paulo (CENAPAD-SP) for the Computational resources. The authors are also thankful to the Cancer Theranostics Innovation Center (CancerThera, funded by FAPESP process 2021/10265-8) and to the Rede Mineira de Materiais Inorgânicos (RM2I), a research group supported by FAPEMIG (RED-00116-23).
References
-
World Health Organization (WHO)
. World Chagas Disease Day. 2024. Available online: https://www.who.int/campaigns/world-chagas-disease-day/2024 (accessed July 2024).
-
Pan American Health Organization
. Factsheet: Chagas Disease in the Americas for Public Health Workers-PAHO/WHO. 2022. Available online: https://www.paho.org/en/documents/factsheet-chagas-disease-americas-public-health-workers (accessed July 2024).
-
E. R. Choffnes and D. A. Relman, IOM (Institute of Medicine). The Causes and Impacts of Neglected Tropical and Zoonotic Diseases: Opportunities for Integrated Intervention Strategies, The National Academies Press, Washington, DC, 2011 Search PubMed.
- A. Yajima, Z. Lin, A. J. Mohamed, A. P. Dash and S. Rijal, Lancet Reg. Health Southeast Asia, 2023, 18, 100302 CrossRef PubMed.
- J. A. Pérez-Molina and I. Molina, Lancet, 2018, 391(10115), 82 CrossRef PubMed.
- J. Durães-Oliveira, J. Palma-Marques, C. Moreno, A. Rodrigues, M. Monteiro, G. Alexandre-Pires, I. P. da Fonseca and G. Santos-Gomes, Int. J. Mol. Sci., 2024, 25, 3840 CrossRef PubMed.
- G. Meola, H. Braband, S. Jordi, T. Fox, O. Blacque, B. Spingler and R. Alberto, Dalton Trans., 2017, 46(42), 14631 RSC.
-
F. A. Mettler and M. J. Guiberteau, Essentials of Nuclear Medicine Imaging, Sixth edn, Elsevier Inc., 2012 Search PubMed.
- R. Klein, E. Celiker-Guler, B. H. Rotstein and R. A. de Kemp, Semin. Nucl. Med., 2020, 50(3), 208 CrossRef PubMed.
- V. S. A. Barbosa, C. M. C. X. Holanda, A. C. J. Câmara, R. P. Silva, D. P. Oliveira, J. A. Moreira and A. C. Medeiros, Exp. Parasitol., 2009, 123(4), 309 CrossRef CAS PubMed.
-
R. Alberto and H. Braband, SPECT/PET Imaging with Technetium, Gallium, Copper, and Other Metallic Radionuclides, in Comprehensive Inorganic Chemistry II, ed. J. Reedijk and K. Poeppelmeier, Elsevier (org.), Oxford, 2nd edn, 2013 Search PubMed.
- M. Navarro, C. Gabbiani, L. Messori and D. Gambino, Drug Discovery Today, 2010, 15(23–24), 1070 CrossRef CAS PubMed.
- R. Docampo and A. O. Stoppani, Arch. Biochem. Biophys., 1979, 197(1), 317 CrossRef CAS PubMed.
- S. Patterson and S. Wyllie, Trends Parasitol., 2014, 30(6), 289 CrossRef CAS PubMed.
- A. S. Carvalho, K. Salomão, S. L. Castro, T. R. Conde, H. P. S. Zamith, E. R. Caffarena, B. S. Hall, S. R. Wilkinson and N. Boechat, Mem. Inst. Oswaldo Cruz, 2014, 109(3), 315 CrossRef PubMed.
- P. Poli, M. A. Mello, A. Buschini, R. A. Mortara, C. N. de Albuquerque, S. Silva, C. Rossi and T. M. A. D. Zucchi, Biochem. Pharmacol., 2002, 64(11), 1617 CrossRef CAS PubMed.
- R. Alberto, A. Egli, U. Abram, K. Hegetschweiler, V. Gramlich and P. A. Schubiger, Dalton Trans., 1994, 2815 RSC.
-
J. A. Riddick, W. B. Bunger and T. K. Sakano, Organic Solvents Physical Properties and Methods of Purification (Techniques of Chemistry), Wiley, New York, NY, USA, 4th edn, 1986, vol II Search PubMed.
- R. Alberto, K. Ortner, N. Wheatley, R. Schibli and A. P. Schubiger, J. Am. Chem. Soc., 2001, 123, 3135 CrossRef CAS PubMed.
- P. L. Magueres, M. DelCampo, K. Saito, J. D. Ferrara, J. Wojciechowski, A. Jones, D. Kucharczyk and M. Meyer, Acta Crystallogr., Sect. A: Found. Adv., 2019, A75, a163 Search PubMed.
- R. C. Clark and J. S. Reid, Acta Crystallogr., Sect. A: Found. Crystallogr., 1995, 51, 887 CrossRef.
-
Agilent Technologies UK Ltd. CrysAlisPRO, Oxford Diffraction Ltd, Yarnton, England, 2009 Search PubMed.
- O. V. Dolomanov, L. J. Bourhis, R. J. Gildea, J. A. K. Howard and H. Puschmann, OLEX2: a complete structure solution, refinement and analysis program, J. Appl. Crystallogr., 2009, 42, 339 CrossRef CAS.
- G. M. Sheldrick, SHELXT – Integrated space-group and crystal-structure determination, Acta Crystallogr., Sect. A: Found. Adv., 2015, 71, 3 CrossRef PubMed.
- G. M. Sheldrick, Crystal structure refinement with SHELXL, Acta Crystallogr., Sect. C: Struct. Chem., 2015, 71, 3 Search PubMed.
- A. L. Spek, Structure validation in chemical crystallography, Acta Crystallogr., Sect. D: Biol. Crystallogr., 2009, 65, 148 CrossRef CAS PubMed.
-
K. Brandenburg, Diamond Crystal and Molecular Structure Visualization (Version 4.0.2), Crystal Impact GbR, Bonn, Germany, 2018 Search PubMed.
- C. F. Macrae, I. Sovago, S. J. Cottrell, P. T. A. Galek, P. McCabe, E. Pidcock, M. Platings, G. P. Shields, J. S. Stevens, M. Towler and P. A. Wood, Mercury 4.0: From visualization to analysis, design and prediction, J. Appl. Crystallogr., 2020, 53, 226 CrossRef CAS PubMed.
- R. Viotti, B. Alarcón de Noya, T. Araujo-Jorge, M. J. Grijalva, F. Guhl, M. C. López, J. M. Ramsey, I. Ribeiro, A. G. Schijman, S. Sosa-Estani, F. Torrico and J. Gascon, Antimicrob. Agents Chemother., 2014, 58(2), 635 CrossRef CAS PubMed.
- A. C. R. Gonçalves, Z. A. Carneiro, C. G. Oliveira, A. Danuello, W. Guerra, R. J. Oliveira, F. B. Ferreira, L. L. W. Veloso-Silva, F. A. H. Batista, J. C. Borges, S. de Albuquerque, V. M. Deflon and P. I. S. Maia, Eur. J. Med. Chem., 2017, 141, 615 CrossRef PubMed.
- F. S. Buckner, C. L. Verlinde, A. C. La Flamme and W. C. Van Voorhis, Antimicrob. Agents Chemother., 1996, 40, 2592 CrossRef CAS PubMed.
- M. T. Murakami, N. C. Rodrigues, L. M. Gava, R. V. Honorato, F. Canduri, L. R. S. Barbosa, G. Oliva and J. C. Borges, Biophys. Chem., 2013, 184, 44 CrossRef CAS PubMed.
- A. P. Borges, M. M. S. Obata, S. H. Libardi, R. O. Trevisan, V. M. Deflon, U. Abram, F. B. Ferreira, L. A. S. Costa, A. O. T. Patrocínio, M. V. da Silva, J. C. Borges and P. I. S. Maia, Pharmaceutics, 2024, 16, 452 CrossRef CAS PubMed.
- K. P. Silva, T. V. Seraphim and J. C. Borges, Biochim. Biophys. Acta, 2013, 1834, 351 CrossRef CAS PubMed.
- S. Bi, L. Ding, Y. Tian, D. Song, X. Zhou, X. Liu and H. Zhang, J. Mol. Struct., 2004, 703, 37 CrossRef CAS.
-
J. R. Lakowicz, Principles of fluorescence Spectroscopy, Kluwer/Plenum, New York, 2nd edn, 1999 Search PubMed.
- D. E. Epps, T. J. Raub, V. Caiolfa, A. Chiari and M. Zamai, J. Pharm. Pharmacol., 1999, 51, 41 CrossRef CAS PubMed.
- H. M. Berman, J. Westbrook, Z. Feng, G. Gilliland, T. N. Bhat, H. Weissig, I. N. Shindyalov and P. E. Bourne, Nucleic Acids Res., 2000, 28, 235 CrossRef CAS PubMed.
- M. L. Verdonk, J. C. Cole, M. J. Hartshorn, C. W. Murray and R. D. Taylor, Proteins, 2003, 52, 609 CrossRef CAS PubMed.
- K. Stierand and M. Rarey, ACS Med. Chem. Lett., 2010, 1, 540 CrossRef CAS PubMed.
- R. A. Laskowski and M. B. Swindells, J. Chem. Inf. Model., 2011, 51, 2778 CrossRef CAS PubMed.
- G. Berkelhammer and G. Asato, Science, 1968, 162, 1146 CrossRef CAS PubMed.
- G. Chauvière, B. Bouteille, B. Enanga, C. de Albuquerque, S. L. Croft, M. L. Dumas and J. Périé, J. Med. Chem., 2003, 46, 427 CrossRef PubMed.
- H. B. Leites, F. S. Damasceno, A. M. Silber, R. Z. Mendonça and C. N. Alburquerque, Pharm. Biol. Eval., 2018, 5, 40 Search PubMed.
- M. H. Moshafi, M. Sorkhi, S. Emami, M. Nakhjiri, A. Yahya-Meymandi, A. S. Negahbani, F. Siavoshi, M. Omrani, E. Alipour, M. Vosooghi, A. Shafiee and A. Foroumadi, Arch. Pharm. Chem. Life Sci., 2011, 344, 178 CrossRef CAS PubMed.
- Á. Díaz-Ortiz, P. Prieto and A. De la Hoz, Chem. Rec., 2019, 19, 85 CrossRef PubMed.
- M. Gaba and N. Dhingra, Indian J. Pharm. Educ. Res., 2010, 45, 175 Search PubMed.
-
J. B. Lambert and E. P. Mazzola, Nuclear Magnetic Resonance Spectroscopy: an Introduction to Principles, Applications, and Experimental Methods, Prentice Hall, New Jersey, 1st edn, 2002 Search PubMed.
-
D. L. Pavia, G. M. Lampman and G. S. Kriz, in Introduction to Spectroscopy, Thomson Learning Inc., Bellingham, Washington, 3rd edn, 2001 Search PubMed.
- A. Gómez, G. Jara, E. Flores, T. Maldonado, T. Godoy, M. Muoz-Osses, A. Vega, R. Mera, C. Silva and J. Pavez, New J. Chem., 2020, 44, 14171 RSC.
- D. V. Griffiths, Y. Cheong, P. Duncanson and M. Motevalli, Dalton Trans., 2011, 40, 10215 RSC.
- J. Palion-Gazda, K. Choroba, M. Penkala, P. Rawicka and B. Machura, Inorg. Chem., 2024, 63, 1356 CrossRef CAS PubMed.
- R. Kushwaha, V. Singh, S. Peters, A. K. Yadav, T. Sadhukhan, B. Koch and S. Banerjee, J. Med. Chem., 2024, 67, 6537 CrossRef CAS PubMed.
- B. Bouteille, A. Marie-Daragon, G. Chauvière, C. de Albuquerque, B. Enanga, M. L. Dardé, J. M. Vallat, J. Périé and M. Dumas, Acta Trop., 1995, 60(2), 73 CrossRef CAS PubMed.
- L. Dobiás, M. Cerná, P. Rössner and R. Srám, Mutat. Res., 1994, 317(3), 177 Search PubMed.
- F. Nesslany, S. Brugier, M.-A. Mouriès, F. Le Curieux and D. Marzin, Mutat. Res., 2004, 560(2), 147 CAS.
- E. J. Chatelain, J. Biomol. Screening, 2015, 20, 22 CrossRef PubMed.
- E. Chatelain and J.-R. Ioset, Drug Des., Dev. Ther., 2011, 5, 175 Search PubMed.
- A. Almeida, B. L. Oliveira, J. D. G. Correia, G. Soveral and A. Casini, Coord. Chem. Rev., 2013, 257, 2689 CrossRef.
- R. E. Williams and N. C. Bruce, Microbiol., 2002, 148, 1607 CrossRef CAS PubMed.
- B. K. Kubata, Z. Kabututu, T. Nozaki, C. J. Munday, S. Fukuzumi, K. Ohkubo, M. Lazarus, T. Maruyama, S. K. Martin, M. Duszenko and Y. Urade, J. Exp. Med., 2002, 196, 1241 CrossRef CAS PubMed.
- F. Díaz-Viraqué, M. L. Chiribao, A. Trochine, F. González-Herrera, C. Castillo, A. Liempi, U. Kemmerling, J. D. Maya and C. Robello, Front. Immunol., 2018, 9, 456 CrossRef PubMed.
- Y. Meah and V. Massey, Proc. Natl. Acad. Sci. U. S. A., 2000, 97, 10733 CrossRef CAS PubMed.
- S. M. F. Murta, M. A. Krieger, L. R. Montenegro, F. F. M. Campos, C. M. Probst, A. R. Avila, N. H. Muto, R. C. de Oliveira, L. R. Nunes, P. Nirde, O. Bruna-Romero, S. Goldenberg and A. J. Romanha, Mol. Biochem. Parasitol., 2006, 146, 151 CrossRef CAS PubMed.
- N. Okamoto, K. Yamaguchi, E. Mizohata, K. Tokuoka, N. Uchiyama, S. Sugiyama, H. Matsumura, K. Inaka, Y. Urade and T. Inoue, J. Biochem., 2011, 150(5), 563–568 CrossRef CAS PubMed.
- Z. A. Carneiro, J. C. Lima, C. D. Lopes, A. P. S. Gaspari, S. de Albuquerque, L. R. Dinelli, L. L. W. Veloso-Silva, M. O. Paganelli, S. H. Libardi, C. G. Oliveira, V. M. Deflon, R. J. Oliveira, J. C. Borges and P. I. S. Maia, Eur. J. Med. Chem., 2019, 180, 213 CrossRef CAS PubMed.
- Z. D. Nagel and J. P. Klinman, Nat. Chem. Biol., 2009, 5, 543–550, DOI:10.1038/nchembio.204.
|
This journal is © The Royal Society of Chemistry 2024 |
Click here to see how this site uses Cookies. View our privacy policy here.