DOI:
10.1039/D1QI01255J
(Research Article)
Inorg. Chem. Front., 2022,
9, 165-178
Phosphomolybdic acid encapsulated in ZIF-8-based porous ionic liquids for reactive extraction desulfurization of fuels†
Received
3rd October 2021
, Accepted 12th November 2021
First published on 15th November 2021
Abstract
Porous ionic liquids (PILs), with permanent porosity and fluidity, have been drawing great attention in theoretical research and application. Herein, a novel PIL (HPMo@ZIF-8-PIL) was fabricated by dispersing a phosphomolybdic acid-modified zeolitic imidazolate framework-8 (ZIF-8) into an ionic liquid for deep reactive extraction desulfurization. Size calculation results show that 1-ethyl-3-methylimidazolium bis(trifluoromethanesulfonylimide) ([Emim][NTf2]) with larger ion sizes cannot occupy pores of ZIF-8. In detail, the structure along with permanent porosity was well characterized by gas absorption and molecule displacement experiments, and it was found that HPMo@ZIF-8-PIL can not only promote the dispersion of the catalytically active sites but also retain the good liquidity and excellent extraction ability of ionic liquids. At room temperature, 100% removal of dibenzothiophene sulfur in a model oil could be achieved within 2 h using HPMo@ZIF-8-PIL and hydrogen peroxide as the catalyst and oxidant, respectively. Besides, the successful preservation of porosity increases the probability of contact between the oxidant and active sites, which is also in favor of the promotion of desulfurization performance. This work offers a new method for the modification of porous frameworks to construct PIL catalysts, which will provide a new insight into the application of PILs.
1. Introduction
The combustion of sulfides in fuel oils leads to severe ecological problems, such as acid rain, haze, and so on. Thus, reducing sulfide contents to upgrade fuel oil quality is very important for clean fuel production.1,2 Currently, many methods have been developed for the desulfurization of fuel oils, such as hydrodesulfurization (HDS),3–5 adsorptive desulfurization (ADS),6–8 extractive desulfurization (EDS),9,10 and oxidative desulfurization (ODS).11–14 Among all these desulfurization approaches, HDS, the most widely used method in the industry, has turned out to be an effective strategy for the removal of aliphatic sulfides in fuel oils. Yet, for the deep removal of aromatic sulfides, such as dibenzothiophene (DBT) and its derivatives, HDS requires harsher reaction conditions as well as higher hydrogen and energy consumption.15 Under this circumstance, a series of non-HDS methods have been developed for the removal of aromatic sulfides. For EDS, it is based on the difference in solubility of sulfides in the oil and extractant to achieve the removal of sulfur compounds. As a common extractant, ionic liquids (ILs) such as imidazolium and pyridinium ILs are widely used in the process.16 However, the extractive performance is relatively poor in a single run.10 Although highly efficient ODS can achieve deep desulfurization, the refractory separation of the catalyst along with potential co-oxidation of fuel oil turns out to be a barrier for the further application of ODS.13,17
Based on the above analysis, by combining the advantages of EDS and ODS, the concept of reactive extraction desulfurization (REDS) can be employed as a post-treatment for HDS to effectively remove aromatic sulfides. This proposed REDS system can be used in a reactive extractor for extraction coupled catalytic oxidation desulfurization, which comprises extraction and oxidation reaction processes (Fig. 1). In the extraction process, aromatic sulfides are extracted and concentrated in the extractant phase under mild conditions and then the sulfides are oxidized to high-polar sulfones. Recycled sulfones are the high value-added product,18 which improves the economy of the desulfurization process and accords with the trend of the refining process to chemical products. To achieve excellent REDS performance, a proper catalyst is essential which should efficiently extract aromatic sulfides and activate oxidants such as hydrogen peroxide (H2O2),19,20 ozone (O3),21 and molecular oxygen (O2).22,23 The catalyst can be recycled through a separator drum. It has been reported that dispersing catalytically active species in conventional ILs can extract sulfides and activate oxidants.24 However, because of the natural characteristic of ILs, the dispersion of catalytically active sites is relatively poor, resulting in low use efficiency of the catalytically active sites.
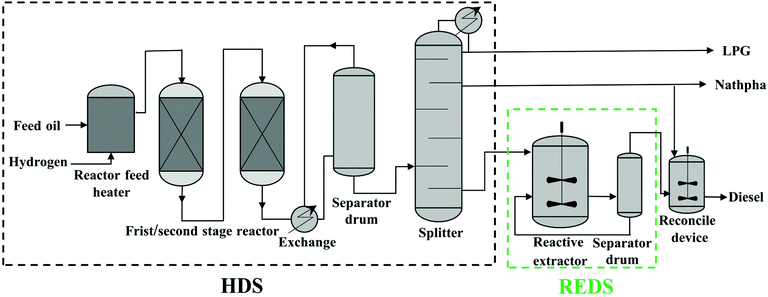 |
| Fig. 1 Flow diagram of HDS with REDS. | |
In recent years, porous ionic liquids (PILs), as a promising new type of liquid material with permanent porosity, have been receiving growing attention in the chemical engineering field.25–28 They are obtained by mixing traditional ILs with porous frameworks such as zeolite, metal–organic frameworks (MOFs), covalent organic frameworks (COFs), and so on.29–31 On the one hand, the pore sizes of the porous frameworks are smaller than the molecular diameters of ILs preventing the ILs from accessing the cavities within the frameworks. On the other hand, porous frameworks should be stable and uniformly dispersed in ILs to form a liquid phase. It is worthwhile to note that PILs have been employed as an effective material for the improvement of CO2 absorption and separation.28,32 Toward this end, PILs not only have similar properties to ILs for sulfide extraction but also can accommodate catalytically active sites in the porous frameworks for catalytic sulfide oxidation. Moreover, because of the porous structure of the frameworks, the catalytically active sites can have remarkable dispersion for enhanced catalytic performance. Thus, a PIL catalyst can be designed for reactive extraction desulfurization. For the preparation of the PIL catalyst, a suitable porous framework along with a proper ionic liquid with matching sizes is required. Zeolitic imidazolate framework-8 (ZIF-8), a chemically stable MOF material prepared by the coordination of Zn(II) with 2-methylimidazolate, has been used as an effective porous framework to prepare PILs because it possesses a pore opening size of 3.4 Å, which is small enough to prevent most solvents from accessing the cavities.33–35 For example, Liu et al. reported MOF-based PILs by dispersing ZIF-8 nanocrystallites in a [Bpy][NTf2] IL.27 Costa Gomes et al. prepared a new family of PILs based on ZIF-8 and phosphonium cations and carboxylate anions.28 Although PILs have many advantages, the PIL prepared by mixing ZIF-8 and conventional ILs still lacks active sites to catalyze the oxidation of sulfide substrates. Heteropoly acids (HPAs) are a kind of economically and environmentally benign acid catalyst and show remarkable catalytic ODS performance.36–39 Therefore, it is expected that the functionalization of ZIF-8 using HPAs will be practicable to act as the porous frameworks of PILs when applied to the extraction and oxidation reaction.
In this work, we report an example of phosphomolybdic acid (HPMo) encapsulated within ZIF-8 based porous ionic liquids (HPMo@ZIF-8-PIL) for REDS. HPMo as an active species was encapsulated in ZIF-8 by a one-pot synthesis and then the prepared porous frameworks were stable and uniformly dispersed in a voluminous 1-ethyl-3-methylimidazolium bis(trifluoromethanesulfonylimide) ([Emim][NTf2]) IL to maintain their porosity. In the REDS system, HPMo@ZIF-8-PIL showed enhanced performance for the removal of DBT, achieving complete removal of DBT within 120 min at room temperature. Detailed experiments show that the boosted desulfurization performance originates from the highly dispersed active sites induced by ZIF-8 along with the excellent extraction ability of ionic liquids, which can make the sulfides contact better with the catalytically active sites.
2. Experimental
Materials
Methanol (CH3OH, AR), phosphomolybdic acid (H3PMo12O40·xH2O, AR), chloroform (CHCl3, AR), tetrachloromethane (CCl4, AR), zinc nitrate hexahydrate (Zn (NO3)2·6H2O, AR) and hydrogen peroxide (H2O2, 30 wt%) were received from Sinopharm Chemical Reagent Co., Ltd. 2-Methylimidazole (2-MeIM, 98%) and hexadecane (C16H34, AR) were purchased from Aladdin Chemistry. 1-Ethyl-3-methylimidazolium bis(trifluoromethanesulfonylimide) ([Emim][NTf2], 99%) was purchased from Lanzhou Aolic Chemical Co., Ltd. Dodecane (C12H26, 98%) was purchased from Shanghai Macklin Biochemical Co., Ltd. Dibenzothiophene (DBT, 98%), 4-methyldibenzothiophene (4-MDBT, 96%), and 4,6-dimethyldibenzothiophene (4,6-DMDBT, 97%) were provided by Sigma-Aldrich.
Preparation of samples
Preparation of the HPMo@ZIF-8 porous framework.
Zn (NO3)2·6H2O (1.476 g) and 2-MeIM (3.286 g) were dissolved in CH3OH (50 mL), respectively. The above two solutions were mixed and stirred for 1 h at 50 °C. During the synthesis procedure, 0.05 g of HPMo was added. The precipitate was obtained by filtration and then washed with CH3OH. After that, the sample was dried at 80 °C overnight. The sample was denoted as HPMo@ZIF-8. The synthesis of ZIF-8 was the same as that of HPMo@ZIF-8 without the addition of HPMo.
Preparation of HPMo@ZIF-8-PIL.
In a typical preparation, the synthesized porous frameworks (50 mg) were added into [Emim][NTf2] (1 mL), and then the mixture was stirred and ultrasonicated alternately for 2 h at room temperature to obtain uniformly liquid samples. The final product was denoted as HPMo@ZIF-8-PIL.
Characterization
The crystal structure of the samples was obtained using a Shimadzu XRD-6100 with Cu Kα radiation (λ = 1.5406 Å). Fourier transform infrared (FT-IR) spectra were recorded with a Nicolet IS50 FT-IR spectrometer. N2 adsorption–desorption isotherms were measured on a Micromeritics ASAP 2460 with the solid samples pretreated in a vacuum at 100 °C. Scanning electron microscopy (SEM) was collected on a JSM-7800F to observe the surface morphology. Transmission electron microscopy (TEM) was operated with a JEM-2100 (HR) microscope. Differential scanning calorimetry (DSC) was measured through the DSC Q200 TA instrument under a N2 atmosphere. Thermo-gravimetric analysis (TGA) measurements were obtained through a Netzsch STA 449 C instrument under a N2 atmosphere. The reaction products were detected by gas chromatography-mass spectrometry (GC-MS 7890A/5975C, Agilent).
The procedure of reactive extraction desulfurization
Different model oils with 200 ppm of S were obtained by dissolving different substrates (DBT, 4-MDBT, and 4,6-DMDBT) in dodecane. Hexadecane was added to the model oils as an internal standard. In a typical reactive extractant desulfurization process, a mixture containing 1 mL of HPMo@ZIF-8-PIL, 3 mL of model oil, and a certain amount of 30 wt% H2O2 in a double-necked flask was stirred at a definite temperature. In the process, the upper oil phase was taken out at regular intervals and analyzed by a gas chromatograph (Agilent 7890A). The extraction desulfurization process was the same as the above process, except that H2O2 was not added. Sulfur removal was used to test the catalytic performance and calculated through the following formula: | Sulfur removal (%) = (C0 − Ct)/C0 × 100% | (1) |
where C0 referred to the initial S-concentration and Ct referred to the S-concentration at time t (min).
3. Results and discussion
Characterization of the HPMo@ZIF-8 porous framework
The HPMo@ZIF-8 porous framework was available through in situ self-assembly of zinc nitrate, 2-MeIM, and HPMo. It should be noted that the diameter of HPMo (10 Å) matches well with the cavity size (11.2 Å), and is smaller than the cage diameter of ZIF-8 (3.4 Å), which enables the stability of encapsulation of HPMo.40 XRD was first employed to characterize the crystal structure of ZIF-8 and HPMo@ZIF-8 (Fig. 2a). ZIF-8 exhibits the crystalline planes of diffraction of (011), (112), (222), and so on, which is consistent with previous reports,41,42 indicating the successful preparation of ZIF-8. Meanwhile, the XRD pattern of HPMo@ZIF-8 shows that with the addition of HPMo, the characteristic structure of ZIF-8 can be retained and no significant peak for HPMo is observed, which is attributed to the encapsulation and high dispersion of HPMo in the ZIF-8 cages.43 The successful encapsulation of HPMo was further verified by FT-IR in Fig. 2b. It can be seen from the FT-IR spectrum of ZIF-8 that the adsorption peaks at 421 cm−1 and 990 cm−1 can correspond to the characteristic vibration of the Zn–N and C–N bonds in ZIF-8, respectively.44 For the FT-IR spectrum of the pure HPMo, a series of characteristic peaks at 1065 cm−1 (P–O), 960 cm−1 (Mo
O), and 871 cm−1 (Mo–Ob–Mo) is observed, which are assigned to Keggin units of HPMo.40,45 The spectrum of HPMo@ZIF-8 shows that the absorption peaks at 421 cm−1 and 990 cm−1 still can be detected, indicating the structural stability of ZIF-8 after encapsulation of HPMo. Moreover, the characteristic stretching vibration peaks of Keggin units are also observed. These results illustrate the successful encapsulation of HPMo.
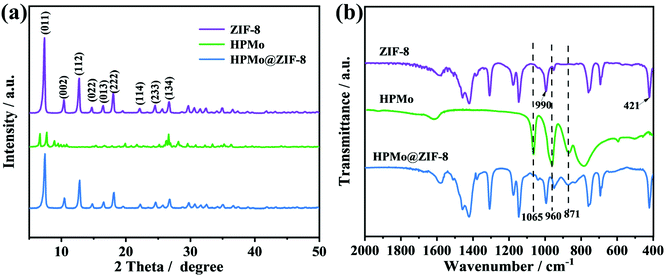 |
| Fig. 2 (a) XRD patterns and (b) FT-IR spectra of ZIF-8, HPMo, and HPMo@ZIF-8. | |
Then, the N2 adsorption–desorption isotherms were collected to examine the change of porosity after the encapsulation of HPMo. As shown in Fig. 3a, the isotherm of HPMo@ZIF-8 exhibits a typical microporous structure, which is the same as that of ZIF-8. Besides, both loop curves of ZIF-8 and HPMo@ZIF-8 show pronounced H4 hysteresis loops in the partial pressure range of 0.4–1.0, demonstrating the presence of a few mesopores and macropores. However, it is noteworthy that with the incorporation of HPMo, the specific surface area and pore volume decrease (Table S1†), which is another evidence to prove that the active component HPMo is encapsulated into the ZIF-8.46 In addition, the pore size distribution curves in Fig. 3b illustrate that there are abundant micropores in ZIF-8 and HPMo@ZIF-8. With the encapsulation of HPMo, the micropores show a decreasing trend, which also demonstrates the encapsulation of HPMo in the pores of ZIF-8.
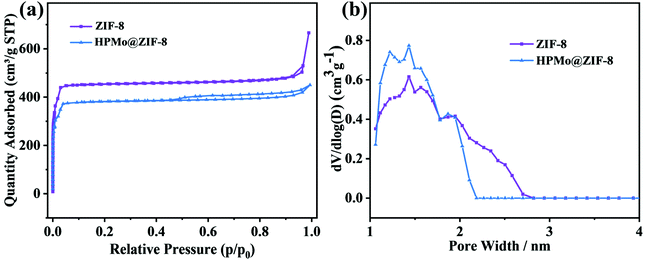 |
| Fig. 3 (a) N2 adsorption–desorption isotherms and (b) pore size distribution curves of ZIF-8 and HPMo@ZIF-8. | |
SEM image in Fig. 4a shows that ZIF-8 presents a hexagonal block shape with uniform particle sizes of 100–150 nm. Meanwhile, the SEM image in Fig. 4b demonstrates that HPMo@ZIF-8 maintains the hexagonal morphology of the skeleton. The small and uniform size of the HPMo@ZIF-8 framework implies abundant surfaces for the interaction between the porous hosts (ZIF-8) and the ionic liquid. The small and uniform particle sizes of HPMo@ZIF-8 indicate that particles can possess a highly stable dispersion ability in a liquid environment.47 Most importantly, it can be seen from the SEM image of HPMo@ZIF-8 that it also possesses a smooth surface, which is like that of pristine ZIF-8, further illustrating that HPMo is encapsulated in the pores of ZIF-8 instead of the surfaces. Moreover, to intuitively understand the elemental distribution on the sample, the elements Zn, P, and Mo were analyzed by the combined mapping technology. According to the mapping images, the three elements were present and uniformly distributed (Fig. 4c) and no significant agglomeration could be detected, demonstrating that HPMo was highly dispersed in the ZIF-8, which is in accordance with XRD characterization.
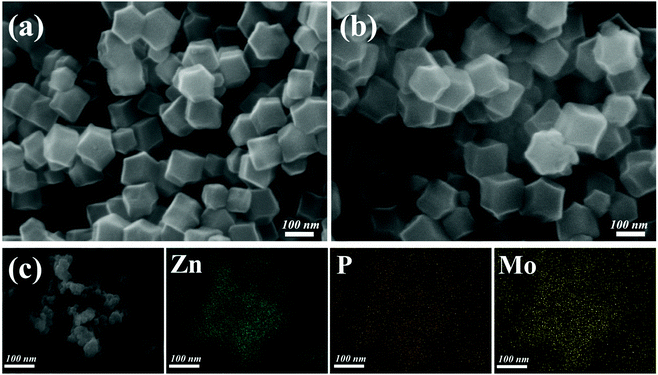 |
| Fig. 4 SEM images of (a) ZIF-8, (b) HPMo@ZIF-8 and (c) mapping images of HPMo@ZIF-8. | |
Characterization of HPMo@ZIF-8-PIL
The above analysis shows that HPMo is uniformly encapsulated by ZIF-8 in HPMo@ZIF-8. Furthermore, selecting a suitable ionic liquid solvent to dissolve the porous framework is another important factor. Herein, by theoretical calculation, the [Emim][NTf2] IL was selected as the solvent for HPMo@ZIF-8 dispersion. The calculations were performed at the B3LYP/6-31g(d) level in the Gaussian 16 program and the molecular size was measured in Multiwfn. Because the dimensions of the [Emim] cation (5.27 × 9.83 × 6.46 Å3) and [NTf2] anion (6.26 × 9.28 × 6.64 Å3) are larger than the pore opening size of ZIF-8 (3.4 Å), the ionic liquid can be prevented from entering the cavities of ZIF-8 (Fig. 5). ZIF-8 was dispersed in [Emim][NTf2] by vigorous stirring and ultrasound instead of adding an additional solvent (such as methanol) for the preparation of PILs (HPMo@ZIF-8-PIL). Generally, a strong interaction between the solvent ions and porous framework can occur, which may originate from the interactions between the abundant reactive Zn2+ ions on the surface of ZIF-8 and nitrogen atoms of the cation of the ionic liquid. Because of the existence of strong interactions between the solvent ions and porous framework, the obtained suspension remained stable and no sedimentation was observed at room temperature (Fig. 5). As shown in the SEM image in Fig. 5, the PIL was successfully assembled, and the IL efficiently surrounded the solid framework, which is in sharp contrast to HPMo@ZIF-8 (Fig. 4b). The SEM characterization indicates the formation of the PIL by dispersing HPMo@ZIF-8 in the [Emim][NTf2] IL. The TEM image in Fig. S1† further shows that HPMo@ZIF-8 maintains the original morphology and particle size with a block structure and is well dispersed in the ionic liquid phase. Thus, it can be concluded that the prepared HPMo@ZIF-8-PIL is a homogeneous and stable fluid at room temperature.
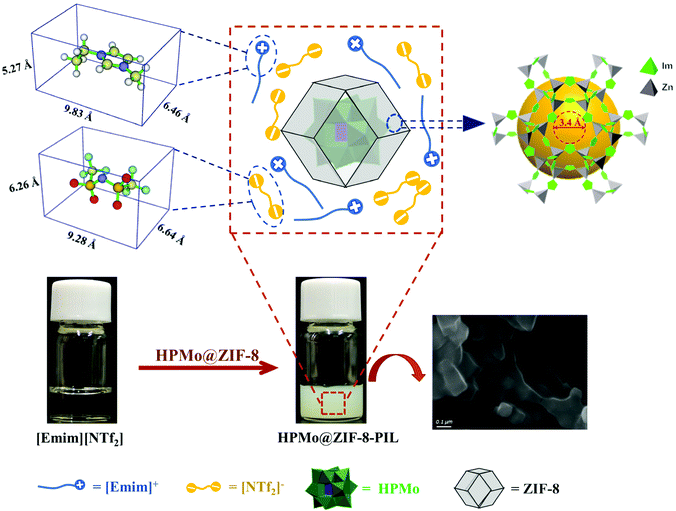 |
| Fig. 5 Schematic illustration of the formation of HPMo@ZIF-8-PIL. | |
To further verify the structure of the PILs, a series of characterization methods was carried out. Fig. 6a shows the XRD patterns of the [Emim][NTf2] and HPMo@ZIF-8-PIL. The XRD pattern of [Emim][NTf2] shows a couple of broadened peaks for [Emim][NTf2] at 12° and 20°. Meanwhile, in the XRD pattern of HPMo@ZIF-8-PIL, the characteristic peaks for [Emim][NTf2] and the typical peaks of HPMo@ZIF-8 still can be found without any significant shift, which confirms the stability of the crystal structure of ZIF-8 during the PIL catalyst construction process.48,49 In addition, FT-IR results show a combination of characteristic peaks of the HPMo@ZIF-8 framework and the [Emim][NTf2] IL. As shown in Fig. 6b, the characteristic peaks at 1172 cm−1 and 1050 cm−1 correspond to the νas(S
O) and νas(S–N–S) in the [Emim][NTf2] IL.50,51 Compared with the [Emim][NTf2] IL, HPMo@ZIF-8-PIL exhibits additional absorption peaks at 421 cm−1 and 990 cm−1, which are assigned to the Zn–N and the C–N stretching vibrations of HPMo@ZIF-8, respectively. The FT-IR results further demonstrate that all the structures of ZIF-8, HPMo, and [Emim][NTf2] remain stable during the PIL catalyst engineering process. The thermal stability of [Emim][NTf2] along with the PIL was determined by TGA analysis in Fig. 6c. As shown in the results that both [Emim][NTf2] and the PIL show no evident mass loss below 320 °C, indicating the remarkable thermal stability of the [Emim][NTf2] IL and the resultant PIL. Also, the addition of HPMo@ZIF-8 does not significantly decrease the thermal stability of the ionic liquid, further confirming the structural stability during the PIL catalyst construction process. Besides, the DSC analysis in Fig. 6d shows that the melting temperature (Tm) of the PIL is close to that of the pristine IL (Tm = −17 °C), which also demonstrates the structural stability of [Emim][NTf2]. It is noteworthy that the Tm of the PIL is far below room temperature, indicating that the PIL remains in a liquid state and possesses fluidity at room temperature.
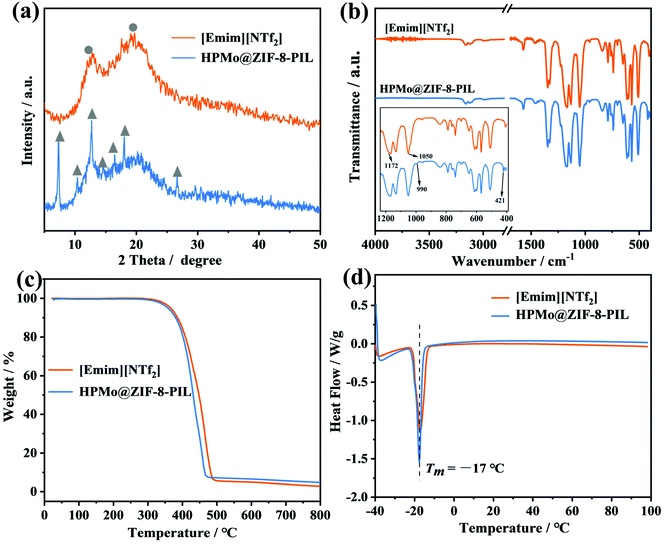 |
| Fig. 6 (a) XRD patterns (triangle: HPMo@ZIF-8, circle: [Emim][NTf2]); (b) FT-IR spectra; (c) TGA and (d) low temperature DSC curves of [Emim][NTf2] and HPMo@ZIF-8-PIL. | |
The N2 adsorption–desorption results above show that the HPMo@ZIF-8 framework has a porous structure. However, it is difficult to confirm the porous structure of the PIL by N2 adsorption–desorption curves because of the liquid characteristic of PILs. On this occasion, SO2 was employed as a probing molecule to verify the permanent porosity of HPMo@ZIF-8-PIL. Typically, the SO2 sorption capacity of HPMo@ZIF-8-PIL was measured, and [Emim][NTf2] was also employed as a reference sample. The SO2 sorption experiments were performed with the vapor–liquid equilibrium apparatus according to our previous work.52 As the results in Fig. 7a show, with the increase of pressure, the SO2 sorption capacity of the [Emim][NTf2] IL showed a significant increase. The adsorption of SO2 molecules originates from the chemical interactions between SO2 molecules and the [Emim][NTf2] IL. Meanwhile, HPMo@ZIF-8-PIL shows a similar trend. In particular, HPMo@ZIF-8-PIL possesses higher SO2 sorption capacity than the [Emim][NTf2] IL under all pressures. The enhanced SO2 sorption capacity results from the existence of permanent porosity in HPMo@ZIF-8-PIL, which can provide additional free volume to accommodate SO2 molecules. Such increment in SO2 sorption capacity of HPMo@ZIF-8-PIL demonstrates the existence of permanent porosity.
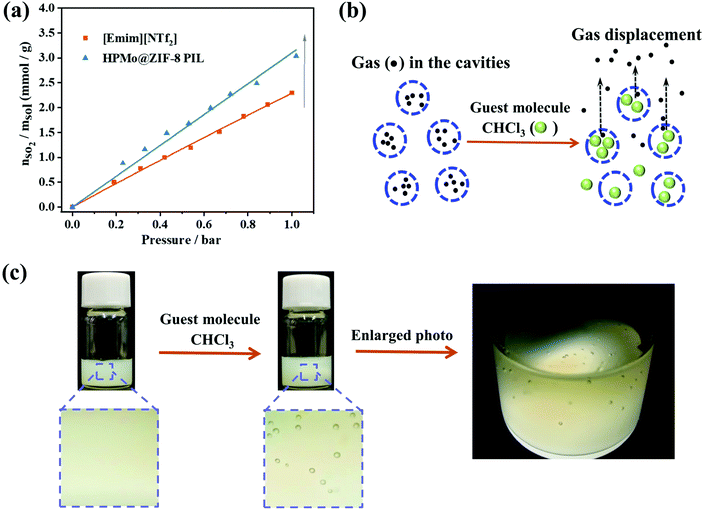 |
| Fig. 7 (a) Gas sorption capacity of HPMo@ZIF-8-PIL; (b) demonstration of molecular displacement mechanism; (c) the addition of a small guest molecule (CHCl3) to HPMo@ZIF-8-PIL leads to the displacement of gas from the cavities and formation of bubbles. | |
To further provide evidence of the porosity in the resultant HPMo@ZIF-8-PIL, a molecular displacement experiment was further employed with a small guest molecule (CHCl3) selected as a gas displacer. Generally, CHCl3 possesses a smaller molecule size, which is much smaller than the pore sizes of ZIF-8, making the CHCl3 molecule able to easily enter the cages of ZIF-8 and thereby discharging gas from the cavity and leading to the formation of bubbles (Fig. 7b). As shown in Fig. 7c, bubbles were observed after adding CHCl3 and stirring for a few seconds at room temperature. This phenomenon of gas switching behavior can be explained by a molecule displacement mechanism instead of a change of the bulk solvent properties.53,54 When as a control experiment CHCl3 was added to the [Emim][NTf2] IL, no gas was released from it because of the lack of the pore structure (Fig. S2†). Such a result demonstrates that there are numerous permanent pores in the HPMo@ZIF-8-PIL sample. Thus, both the results confirm that the porosity can be retained after the formation of the PIL, and the ionic liquid does not enter the cages of ZIF-8.
HPMo@ZIF-8-PIL for reactive extraction desulfurization
Since an HPMo@ZIF-8-PIL catalyst, which combines the advantages of ZIF-8, ionic liquids, and high dispersion of HPMo, has been developed, to highlight its catalytic performance, the HPMo@ZIF-8-PIL catalyst was employed in a REDS system for deep desulfurization of fuel oils. Several catalytic systems were developed to remove aromatic sulfur compounds (such as DBT) with hydrogen peroxide (H2O2) as the oxidant.
First, the extraction performance of different catalysts was tested as listed in Table S2.† It can be observed that [Emim][NTf2], HPMo/[Emim][NTf2], ZIF-8/[Emim][NTf2], and HPMo@ZIF-8-PIL exhibit similar sulfur removal of ∼20%. The result demonstrates that the [Emim][NTf2] IL shows limited desulfurization performance in a single run. Meanwhile, it was also found that the introduction of HPMo or ZIF-8 and the formation of the PIL do not significantly increase the extractive desulfurization performance, and the extractive desulfurization performance mainly originates from the interaction between the [Emim][NTf2] IL and sulfides.
Furthermore, H2O2 was added to the reaction system to engineer REDS systems and the results were recorded in Fig. 8a. It can be seen from the results that with the addition of H2O2, the desulfurization activity of [Emim][NTf2] and ZIF-8/[Emim][NTf2] shows no obvious increase, which is attributed to the lack of catalytically active sites. Meanwhile, when HPMo has been dispersed in the [Emim][NTf2] IL, the REDS activity was only 34.4%, only slightly higher than its extractive desulfurization performance. The very low REDS activity of HPMo/[Emim][NTf2] results from the poor dispersion of HPMo in [Emim][NTf2], which is determined by the nature of ILs, limiting the utilization of catalytically active sites for catalytic oxidation. Meanwhile, the HPMo was dispersed by ZIF-8 to obtain an HPMo@ZIF-8 sample for REDS, the desulfurization activity was still as low as 40.0%. This is attributed to the lack of a proper reaction medium.
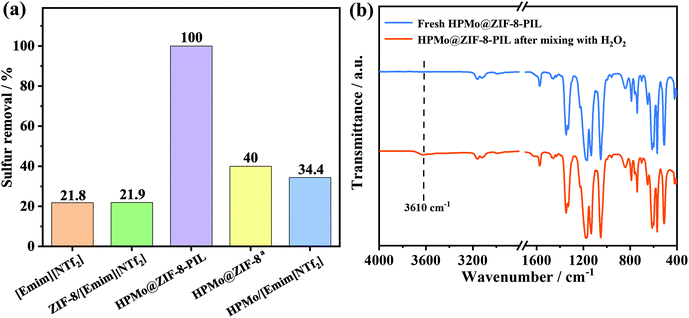 |
| Fig. 8 (a) The sulfur removal of different catalysts. Experimental conditions: V (model oil) = 3 mL, V (cat.) = 1 mL, T = 30 °C, t = 120 min, V (H2O2) = 25 μL. a V (model oil) = 3 mL, m (cat.) = 0.05 g, T = 30 °C, t = 120 min, V (H2O2) = 25 μL; (b) FT-IR spectra of fresh HPMo@ZIF-8-PIL and HPMo@ZIF-8-PIL after mixing with H2O2. | |
When the HPMo was encapsulated in ZIF-8 and uniformly dispersed in the [Emim][NTf2] ionic liquid, HPMo@ZIF-8-PIL could induce excellent sulfur removal of 100%. The significantly enhanced desulfurization performance may be attributed to the excellent dispersion of active sites which is induced by ZIF-8 with a high specific surface area and rich pore structure. Meanwhile, the facilitated contact between substrates and catalytically active sites by the [Emim][NTf2] IL in the desulfurization reaction process contributes to the enhanced activity as well. On the other hand, owing to the molecular diameter of H2O2 being 2.72 × 4.14 × 4.32 Å3, which is smaller than the opening pore size of ZIF-8, H2O2 can pass through the opening pore to contact with active sites with continuous stirring reaction. Compared to the fresh HPMo@ZIF-8-PIL, the FT-IR spectrum of HPMo@ZIF-8-PIL after mixing with H2O2 shows a new peak (3610 cm−1), which is ascribed to the O–H bond,55 demonstrating that H2O2 has entered the pore structure (Fig. 8b). Therefore, it is believed that the permanent porosity of porous ionic liquids can leave space for the entry of oxidants, facilitating the activation of H2O2 and further promoting catalytic performance. Compared with the ionic liquids reported previously, the temperature of deep desulfurization has been greatly lowered, which is only 30 °C (Table 1). Thus, the results prove that permanent porosity, high dispersion of catalytically active sites, and remarkable sulfide extraction performance of HPMo@ZIF-8-PIL contribute to the excellent desulfurization performance.
Table 1 Comparison of oxidation desulfurization performance of different ionic liquid catalysts
Entry |
Catalysts |
T/°C |
Oxidant |
t/h |
Sulfur removal/% |
Ref. |
1 |
[(C18H37)2N(CH3)2]5[IMo6O24] |
80 |
O2 |
8 |
100 |
56
|
2 |
[C6mim]PMoV |
50 |
H2O2 |
3 |
100 |
57
|
3 |
[HMIm]BF4 |
90 |
H2O2 |
6 |
93.0 |
58
|
4 |
[Hnmp]BF4 |
60 |
H2O2 |
0.5 |
100 |
59
|
5 |
WO(O2)2Phen·H2O/[bmim]BF4 |
70 |
H2O2 |
3 |
98.6 |
60
|
6 |
Na2MoO4·2H2O/[bmim]BF4 |
70 |
H2O2 |
3 |
99.0 |
61
|
7 |
HPMo@ZIF-8-PIL |
30 |
H2O2 |
2 |
100 |
This work |
Optimization of desulfurization parameters
Furthermore, to optimize reaction conditions of the REDS system with HPMo@ZIF-8-PIL as the catalyst, a series of condition experiments was carried out. First, the removal of DBT was tested at various temperatures (20 °C, 30 °C, 40 °C, and 50 °C) to investigate the influence of the reaction temperature on the desulfurization performance of HPMo@ZIF-8-PIL (Fig. 9a). The desulfurization performance decreases in the following order of 30 °C (100%) > 40 °C (97.1%) > 20 °C (89%) > 50 °C (83.6%). The optimal reaction temperature is 30 °C, with a 100% sulfur removal after 120 min of reaction. The desulfurization performance at 20 °C is relatively poor because it is difficult to activate H2O2 efficiently at such a low temperature. At higher reaction temperatures (40 °C and 50 °C), sulfur removal was only 95% and 83.6% respectively because of the severe self-decomposition reaction of H2O2 with the increasing temperature,62 which results in poor utilization efficiency of H2O2 in the oxidative desulfurization step.
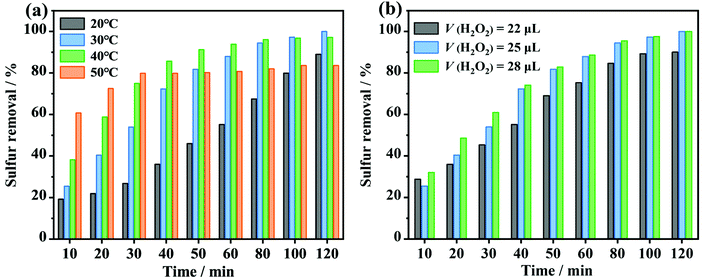 |
| Fig. 9 (a) Effect of reaction temperature on catalytic activity; (b) effect of the amount of oxidant on catalytic activity. | |
In the REDS system, another main factor affecting the desulfurization performance is the dosage of the oxidant (H2O2) and the result is shown in Fig. 9b. Compared to the relatively low removal rate of 90% when the dosage of H2O2 was 22 μL, DBT can be completely removed after 120 min with the dosage of H2O2 increased to 25 μL. With the increase of H2O2 dosage (28 μL), the desulfurization effect was not significantly improved. Therefore, 25 μL of H2O2 is preferred as the optimal oxidant amount.
Catalytic performance for different substrates and kinetics study
Based on the condition experiments described above, HPMo@ZIF-8-PIL is verified to be effective to remove DBT in the REDS system. The reaction system was further extended to remove other sulfur-containing substrates to expand the scope of the catalytic system for desulfurization reactions. DBT, 4-MDBT, and 4,6-DMDBT were chosen as model substrates in the study (Fig. 10a). After 120 min of reaction, promising desulfurization activities can be gained, indicating the potential application of the removal of different sulfides by the REDS system. Besides, the order of catalytic efficiency for the three sulfides is DBT > 4-MDBT > 4,6-DMDBT. According to us, this difference in catalytic activity is mainly related to the Fukui functions (f+(r)) of the different sulfides.63 The f+(r) values of sulfur atoms in DBT, 4-MDBT and 4,6-DMDBT are 0.095, 0.076 and 0.070, respectively. The reactivity is positively correlated with the value of the f+(r) function. The lowest f+(r) value is 4,6-DMDBT, thus it has the lowest reactivity.
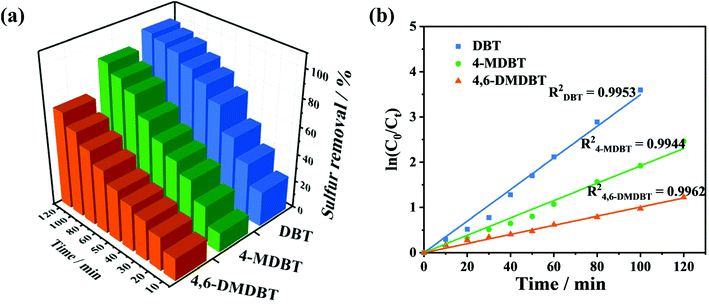 |
| Fig. 10 (a) Effect of the features of different substrates; (b) pseudo-first-order kinetics for the removal of different substrates. Experimental conditions: V (model oil) = 3 mL, V (cat.) = 1 mL, T = 30 °C, t = 120 min, V (H2O2) = 25 μL. | |
The kinetics of the oxidation of different substrates was further studied (Fig. 10b) and provided greatly important parameters in REDS. The relationship between ln(C0/Ct) and reaction time is linear, which indicates that the desulfurization of S-compounds by HPMo@ZIF-8-PIL follows the pseudo-first-order reaction. Besides, the constant rate k, half-lives t1/2, and correlation coefficient R2 are shown in Table S3.†
| ln C0/Ct = kt | (3) |
where
C0 and
Ct were the sulfur concentrations at the initial time and time
t (min), and
k was the first-order rate constant (min
−1).
Regeneration and recycling of HPMo@ZIF-8-PIL
The recycling performance of HPMo@ZIF-8-PIL was investigated in a REDS system containing DBT-containing model oil. The catalyst phase (underlayer) was centrifugated after the reaction and dried to evaporate the residual model oil at 70 °C. Afterward, fresh H2O2 and model oil were added for the next reaction. From experimental results, the desulfurization activity dropped to 87.3% after the third cycle. The decreased catalytic activity may be due to the generated product as the reaction progresses, covering the surface of the porous framework, thereby hindering the interaction between the active sites and the reaction substrates. To regenerate the catalyst and improve the cycling performance, the PIL phase was re-extracted with CCl4 twice at the end of each cycle. The results indicated that sulfur removal could remain 95% after the sixth recycling (Fig. 11). FT-IR characterization was performed to analyse the catalysts after reaction and regeneration (Fig. S3†). No changes of the structures were observed before and after regeneration, which provides evidence for the high stability of HPMo@ZIF-8-PIL.
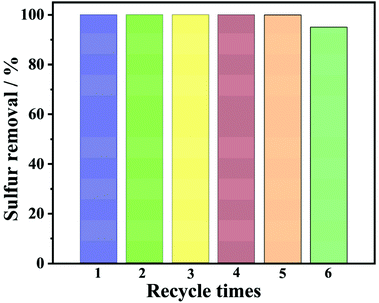 |
| Fig. 11 Recycling of HPMo@ZIF-8-PIL in the desulfurization system. | |
Analysis of products and the possible mechanism
To further study the process of the REDS system, the products of DBT were detected and analyzed by GC-MS. After the reaction, the model oil and PIL catalyst were separated, and 1 μL of the upper oil phase was directly injected into a GC-MS to detect oxidation products. HPMo@ZIF-8-PIL was re-extracted with CCl4 and analyzed. As shown in Fig. 12a, the DBT signal disappeared in the oil phase after the reaction, and only a peak of DBTO2 was detected in the catalyst phase, indicating that DBTO2 is the only desulfurization product. Also, the CCl4 was distilled at 80 °C until a white crystal solid was produced. The FT-IR spectrum of the white solid demonstrated two absorption bands at 1289 cm−1 and 1165 cm−1, being attributed to characteristic groups of sulfone64 (Fig. 12b). This result further proves that DBTO2 was the product of the reaction, and the recovery of the product could be achieved with CCl4 from the catalyst. To this end, a possible REDS mechanism is illustrated in Fig. 12c. Firstly, the sulfur-containing compounds, such as DBT, can be easily extracted from the oil phase by the IL section in HPMo@ZIF-8-PIL, then absorbed on the surface of the framework. Meanwhile, H2O2 as an oxidant gradually passed through the window pores and entered the cavities. HPMo reacted with H2O2 to form the active peroxo-species.38,65,66 At last, the sulfide DBT is oxidized into its corresponding sulfone and retained in the catalyst phase. The oxidizing product was separated by re-extraction. The HPMo@ZIF-8-PIL worked not only as a catalyst but also as an extractant in the process of desulfurization.
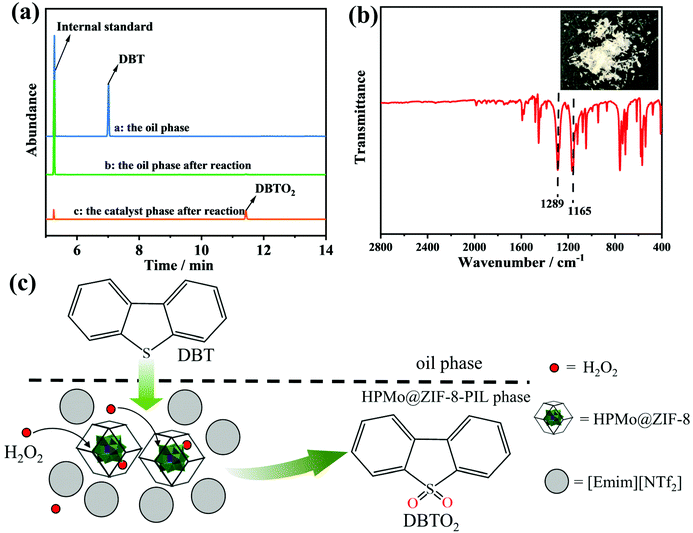 |
| Fig. 12 (a) GC-MS analysis of a: the oil phase, b: the oil phase after reaction and c: the catalyst phase after the reaction; (b) FT-IR spectrum and photograph (insets) of the oxidizing product; (c) possible reaction mechanism of REDS with HPMo@ZIF-8-PIL catalyst. | |
4. Conclusions
In conclusion, based on the ZIF-8 encapsulated with HPMo as the porous framework and the [Emim][NTf2] ionic liquid as the solvent, a novel porous ionic liquid (HPMo@ZIF-8-PIL) was successfully synthesized. ZIF-8 with a high specific surface area and rich pore structure enabled the active site to be highly dispersed. The ionic liquid functioned as a solvent and its good extraction capacity was conducive to the interaction between the substrate and the active site. HPMo@ZIF-8-PIL displayed extraordinary REDS activity for the removal of DBT, achieving complete removal of sulfur within 2 h at room temperature. Furthermore, HPMo@ZIF-8-PIL showed improved catalytic oxidation performance than the pristine IL because of its excellent solubility of oxidants. Our work provides a new strategy to broaden the application range of PILs, by introducing the active components into the frameworks to construct MOF-based PIL catalysts.
Conflicts of interest
There are no conflicts to declare.
Acknowledgements
This work was supported by the Innovative Research Team Project of Hainan Natural Science Foundation (220CXTD436), the National Natural Science Foundation of China (22178154, 22008094 and 21766007), the Natural Science Foundation of Jiangsu Province (BK20190852 and BK20190854), China Postdoctoral Science Foundation (No. 2019M651743), Natural Science Foundation for Jiangsu Colleges and Universities (19KJB530005).
References
- W. Zhu, P. Wu, L. Yang, Y. Chang, Y. Chao, H. Li, Y. Jiang, W. Jiang and S. Xun, Pyridinium-based temperature-responsive magnetic ionic liquid for oxidative desulfurization of fuels, Chem. Eng. J., 2013, 229, 250–256 CrossRef CAS.
- Z. Yao, H. N. Miras and Y. Song, Efficient concurrent removal of sulfur and nitrogen contents from complex oil mixtures by using polyoxometalate-based composite materials, Inorg. Chem. Front., 2016, 3, 1007–1013 RSC.
- Z. Liu, W. Han, D. Hu, S. Sun, A. Hu, Z. Wang, Y. Jia, X. Zhao and Q. Yang, Effects of Ni–Al2O3 interaction on NiMo/Al2O3 hydrodesulfurization catalysts, J. Catal., 2020, 387, 62–72 CrossRef CAS.
- Q. Meng, P. Du, A. Duan, Z. Zhao, J. Liu, D. Shang, B. Wang, Y. Jia, C. Liu and D. Hu, Trimetallic Catalyst Supported Zirconium-Modified Three-Dimensional Mesoporous Silica Material and Its Hydrodesulfurization Performance of Dibenzothiophene and 4,6-Dimethydibenzothiophene, Ind. Eng. Chem. Res., 2019, 59, 654–667 CrossRef.
- L. Zhang, X. Chen and C. Liang, Improving the hydrodesulfurization performance of the sulfur-resistant intermetallic Ni2Si based on a MOF-derived route, Inorg. Chem. Front., 2021, 8, 1122–1127 RSC.
- J. Xiao, S. Sitamraju, Y. Chen, S. Watanabe, M. Fujii, M. Janik and C. Song, Air-promoted adsorptive desulfurization of diesel fuel over Ti-Ce mixed metal oxides, AIChE J., 2015, 61, 631–639 CrossRef CAS.
- Q. Huo, J. Li, G. Liu, X. Qi, X. Zhang, Y. Ning, B. Zhang, Y. Fu and S. Liu, Adsorption desulfurization performances of Zn/Co porous carbons derived from bimetal-organic frameworks, Chem. Eng. J., 2019, 362, 287–297 CrossRef CAS.
- J. Luo, C. Wang, J. Liu, Y. Wei, Y. Chao, Y. Zou, L. Mu, Y. Huang, H. Li and W. Zhu, High–performance adsorptive desulfurization by ternary hybrid boron carbon nitride aerogel, AIChE J., 2021, 67, e17280 CrossRef CAS.
- H. Lu, P. Li, C. Deng, W. Ren, S. Wang, P. Liu and H. Zhang, Deep catalytic oxidative desulfurization (ODS) of dibenzothiophene (DBT) with oxalate-based deep eutectic solvents (DESs), Chem. Commun., 2015, 51, 10703–10706 RSC.
- J. Gao, S. Zhu, Y. Dai, C. Xiong, C. Li, W. Yang and X. Jiang, Performance and mechanism for extractive desulfurization of fuel oil using modified polyethylene glycol, Fuel, 2018, 233, 704–713 CrossRef CAS.
- B. N. Bhadra and S. H. Jhung, Oxidative desulfurization and denitrogenation of fuels using metal-organic framework-based/-derived catalysts, Appl. Catal., B, 2019, 259, 118021 CrossRef CAS.
- L. Lu, J. He, P. Wu, Y. Wu, Y. Chao, H. Li, D. Tao, L. Fan, H. Li and W. Zhu, Taming electronic properties of boron nitride nanosheets as metal-free catalysts for aerobic oxidative desulfurization of fuels, Green Chem., 2018, 20, 4453–4460 RSC.
- Y. Dong, J. Zhang, Z. Ma, H. Xu, H. Yang, L. Yang, L. Bai, D. Wei, W. Wang and H. Chen, Preparation of Co-Mo-O ultrathin nanosheets with outstanding catalytic performance in aerobic oxidative desulfurization, Chem. Commun., 2019, 55, 13995–13998 RSC.
- K. Chen, N. Liu, M. Zhang and D. Wang, Oxidative desulfurization of dibenzothiophene over monoclinic VO2 phase-transition catalysts, Appl. Catal., B, 2017, 212, 32–40 CrossRef CAS.
- L. F. Ramírez-Verduzco, E. Torres-García, R. Gómez-Quintana, V. González-Peña and F. Murrieta-Guevara, Desulfurization of diesel by oxidation/extraction scheme: influence of the extraction solvent, Catal. Today, 2004, 98, 289–294 CrossRef.
- H. Fu, Y. Wu, J. Chen, X. Wang, J. Zheng and X. Li, Promoted hydrogen release from alkali metal borohydrides in ionic liquids, Inorg. Chem. Front., 2016, 3, 1137–1145 RSC.
- P. Sikarwar, V. Gosu and V. Subbaramaiah, An overview of conventional and alternative technologies for the production of ultra-low-sulfur fuels, Rev. Chem. Eng., 2019, 35, 669–705 CAS.
- P. Kowalski, K. Mitka, K. Ossowska and Z. Kolarska, Oxidation of sulfides to sulfoxides. Part 1: Oxidation using halogen derivatives, Tetrahedron, 2005, 61, 1933–1953 CrossRef CAS.
- H. Lü, P. Li, Y. Liu, L. Hao, W. Ren, W. Zhu, C. Deng and F. Yang, Synthesis of a hybrid Anderson-type polyoxometalate in deep eutectic solvents (DESs) for deep desulphurization of model diesel in ionic liquids (ILs), Chem. Eng. J., 2017, 313, 1004–1009 CrossRef.
- P. Wu, L. Lu, J. He, L. Chen, Y. Chao, M. He, F. Zhu, X. Chu, H. Li and W. Zhu, Hexagonal boron nitride: A metal-free catalyst for deep oxidative desulfurization of fuel oils, Green Energy Environ., 2020, 5, 166–172 CrossRef.
- C. Ma, D. Chen, F. Liu, X. Sun, F. Xiao and B. Dai, Oxidative desulfurization of a model fuel using ozone oxidation generated by dielectric barrier discharge plasma combined with Co3O4/γ-Al2O3 catalysis, RSC Adv., 2015, 5, 96945–96952 RSC.
- W. Zhang, H. Zhang, J. Xiao, Z. Zhao, M. Yu and Z. Li, Carbon nanotube catalysts for oxidative desulfurization of a model diesel fuel using molecular oxygen, Green Chem., 2014, 16, 211–220 RSC.
- M. Liu, J. He, P. Wu, L. Lu, C. Wang, L. Chen, M. Hua, W. Zhu and H. Li, Carbon nitride mediated strong metal–support interactions in a Au/TiO2 catalyst for aerobic oxidative desulfurization, Inorg. Chem. Front., 2020, 7, 1212–1219 RSC.
- Y. Jiang, W. Zhu, H. Li, S. Yin, H. Liu and Q. Xie, Oxidative desulfurization of fuels catalyzed by Fenton-like ionic liquids at room temperature, ChemSusChem, 2011, 4, 399–403 CrossRef CAS PubMed.
- N. O'Reilly, N. Giri and S. L. James, Porous liquids, Chem. – Eur. J., 2007, 13, 3020–3025 CrossRef PubMed.
- M. Costa Gomes, L. Pison, C. Cervinka and A. Padua, Porous Ionic Liquids or Liquid Metal-Organic Frameworks?, Angew. Chem., Int. Ed., 2018, 57, 11909–11912 CrossRef CAS.
- S. Liu, J. Liu, X. Hou, T. Xu, J. Tong, J. Zhang, B. Ye and B. Liu, Porous Liquid: A Stable ZIF-8 Colloid in Ionic Liquid with Permanent Porosity, Langmuir, 2018, 34, 3654–3660 CrossRef CAS PubMed.
- J. Avila, L. F. Lepre, C. C. Santini, M. Tiano, S. Denis-Quanquin, K. Chung Szeto, A. A. H. Padua and M. Costa Gomes, High-Performance Porous Ionic Liquids for Low-Pressure CO2 Capture*, Angew. Chem., Int. Ed., 2021, 60, 12876–12882 CrossRef CAS PubMed.
- S. He, L. Chen, J. Cui, B. Yuan, H. Wang, F. Wang, Y. Yu, Y. Lee and T. Li, General Way To Construct Micro- and Mesoporous Metal-Organic Framework-Based Porous Liquids, J. Am. Chem. Soc., 2019, 141, 19708–19714 CrossRef CAS.
- A. Bavykina, A. Cadiau and J. Gascon, Porous liquids based on porous cages, metal organic frameworks and metal organic polyhedra, Coord. Chem. Rev., 2019, 386, 85–95 CrossRef CAS.
- M. Y. Masoomi, A. Morsali, A. Dhakshinamoorthy and H. Garcia, Mixed-Metal MOFs: Unique Opportunities in Metal-Organic Framework (MOF) Functionality and Design, Angew. Chem., Int. Ed., 2019, 58, 15188–15205 CrossRef CAS PubMed.
- X. Zhao, Y. Yuan, P. Li, Z. Song, C. Ma, D. Pan, S. Wu, T. Ding, Z. Guo and N. Wang, A polyether amine modified metal organic framework enhanced the CO2 adsorption capacity of room temperature porous liquids, Chem. Commun., 2019, 55, 13179–13182 RSC.
- C. Keum, H. Lee, C. Kwon, B. Han and S.-Y. Lee, Metal-Induced Self-Assembly Template for Controlled Growth of ZIF-8 Nanorods, Chem. Mater., 2020, 32, 7941–7950 CrossRef CAS.
- B. N. Bhadra, I. Ahmed, S. Kim and S. H. Jhung, Adsorptive removal of ibuprofen and diclofenac from water using metal-organic framework-derived porous carbon, Chem. Eng. J., 2017, 314, 50–58 CrossRef CAS.
- N. A. Khan, B. N. Bhadra and S. H. Jhung, Heteropoly acid-loaded ionic liquid@metal-organic frameworks: Effective and reusable adsorbents for the desulfurization of a liquid model fuel, Chem. Eng. J., 2018, 334, 2215–2221 CrossRef CAS.
- Q. Yu, D. Tan, T. Huang, T. Zhao and L. Li, Preparation of asphalt-based microporous organic polymers catalyzed by heteropoly acids, Green Chem., 2018, 20, 4746–4751 RSC.
- R. Ghubayra, C. Nuttall, S. Hodgkiss, M. Craven, E. F. Kozhevnikova and I. V. Kozhevnikov, Oxidative desulfurization of model diesel fuel catalyzed by carbon-supported heteropoly acids, Appl. Catal., B, 2019, 253, 309–316 CrossRef CAS.
- Y. Peng, J. Liu, H. Zhang, D. Luo and D. Li, A size-matched POM@MOF composite catalyst for highly efficient and recyclable ultra-deep oxidative fuel desulfurization, Inorg. Chem. Front., 2018, 5, 1563–1569 RSC.
- X. Chen, M. Zhang, Y. Wei, H. Li, J. Liu, Q. Zhang, W. Zhu and H. Li, Ionic liquid-supported 3DOM silica for efficient heterogeneous oxidative desulfurization, Inorg. Chem. Front., 2018, 5, 2478–2485 RSC.
- L. Zhang, T. Mi, M. A. Ziaee, L. Liang and R. Wang, Hollow POM@MOF hybrid-derived porous Co3O4/CoMoO4 nanocages for enhanced electrocatalytic water oxidation, J. Mater. Chem. A, 2018, 6, 1639–1647 RSC.
- X. Huang, Y. Lin, J. Zhang and X. Chen, Ligand-Directed Strategy for Zeolite-Type Metal–Organic Frameworks: Zinc(II) Imidazolates with Unusual Zeolitic Topologies, Angew. Chem., 2006, 118, 1587–1589 CrossRef.
- X. Yang, Z. Wen, Z. Wu and X. Luo, Synthesis of ZnO/ZIF-8 hybrid photocatalysts derived from ZIF-8 with enhanced photocatalytic activity, Inorg. Chem. Front., 2018, 5, 687–693 RSC.
- R. S. Malkar and G. D. Yadav, Synthesis of cinnamyl benzoate over novel heteropoly acid encapsulated ZIF-8, Appl. Catal., A, 2018, 560, 54–65 CrossRef CAS.
- S. Mukhopadhyay, J. Debgupta, C. Singh, A. Kar and S. K. Das, A Keggin Polyoxometalate Shows Water Oxidation Activity at Neutral pH: POM@ZIF-8, an Efficient and Robust Electrocatalyst, Angew. Chem., Int. Ed., 2018, 57, 1918–1923 CrossRef CAS.
- Q. Wang, E. Liu, C. Zhang, S. Huang, Y. Cong and Y. Zhang, Synthesis of Cs3PMo12O40/Bi2O3 composite with highly enhanced photocatalytic activity under
visible-light irradiation, J. Colloid Interface Sci., 2018, 516, 304–311 CrossRef CAS PubMed.
- M. Stuckart and K. Y. Monakhov, Polyoxometalate encapsulation into metal–organic frameworks: the way towards functional nanomaterials for water splitting, J. Mater. Chem. A, 2018, 6, 17849–17853 RSC.
- J. Cahir, M. Y. Tsang, B. Lai, D. Hughes, M. A. Alam, J. Jacquemin, D. Rooney and S. L. James, Type 3 porous liquids based on non-ionic liquid phases – a broad and tailorable platform of selective, fluid gas sorbents, Chem. Sci., 2020, 11, 2077–2084 RSC.
- J. Zhang, S. H. Chai, Z. A. Qiao, S. M. Mahurin, J. Chen, Y. Fang, S. Wan, K. Nelson, P. Zhang and S. Dai, Porous liquids: a promising class of media for gas separation, Angew. Chem., Int. Ed., 2015, 54, 932–936 CrossRef CAS PubMed.
- P. Li, H. Chen, J. A. Schott, B. Li, Y. Zheng, S. M. Mahurin, D. E. Jiang, G. Cui, X. Hu, Y. Wang, L. Li and S. Dai, Porous liquid zeolites: hydrogen bonding-stabilized H-ZSM-5 in branched ionic liquids, Nanoscale, 2019, 11, 1515–1519 RSC.
- H. Zhang, G. Cao, Y. Yang and Z. Gu, Capacitive performance of an ultralong aligned carbon nanotube electrode in an ionic liquid at 60 °C, Carbon, 2008, 46, 30–34 CrossRef CAS.
- J. Wu, L. Mu, X. Feng, X. Lu, R. Larsson and Y. Shi, Poly(alkylimidazolium bis(trifluoromethylsulfonyl)imide)-Based Polymerized Ionic Liquids: A Potential High-Performance Lubricating Grease, Adv. Mater. Interfaces, 2019, 6, 1801796 CrossRef.
- Q. Zhu, C. Wang, J. Yin, H. Li, W. Jiang, J. Liu, P. Li, Q. Zhang, Z. Chen and W. Zhu, Efficient and remarkable SO2 capture: A discovery of imidazole-based ternary deep eutectic solvents, J. Mol. Liq., 2021, 330, 115595 CrossRef CAS.
- N. Giri, M. G. Del Popolo, G. Melaugh, R. L. Greenaway, K. Ratzke, T. Koschine, L. Pison, M. F. Gomes, A. I. Cooper and S. L. James, Liquids with permanent porosity, Nature, 2015, 527, 216–220 CrossRef CAS.
- E. B. Hemming, A. F. Masters and T. Maschmeyer, The encapsulation of metal nanoparticles within porous liquids, Chem. Commun., 2019, 55, 11179–11182 RSC.
- T. Kang, B. Li, Q. Hao, W. Gao, F. Bin, K. N. Hui, D. Fu and B. Dou, Efficient Hydrogen Peroxide (H2O2) Synthesis by CaSnO3 via Two-Electron Water Oxidation Reaction, ACS Sustainable Chem. Eng., 2020, 8, 15005–15012 CrossRef CAS.
- H. Lü, Y. Zhang, Z. Jiang and C. Li, Aerobic oxidative desulfurization of benzothiophene, dibenzothiophene and 4,6-dimethyldibenzothiophene using an Anderson-type catalyst [(C18H37)2N(CH3)2]5[IMo6O24], Green Chem., 2010, 12, 1954–1958 RSC.
- J. Zhuang, B. Hu, J. Tan and X. Jin, Deep oxidative desulfurization of dibenzothiophene with molybdovanadophosphoric heteropolyacid-based catalysts, Transition Met. Chem., 2013, 39, 213–220 CrossRef.
- L. Lu, S. Cheng, J. Gao, G. Gao and M. He, Deep Oxidative Desulfurization of Fuels Catalyzed by Ionic Liquid in the Presence of H2O2, Energy Fuels, 2007, 21, 383–384 CrossRef CAS.
- D. Zhao, J. Wang and E. Zhou, Oxidative desulfurization of diesel fuel using a Brønsted acid room temperature ionic liquid in the presence of H2O2, Green Chem., 2007, 9, 1219–1222 RSC.
- W. Zhu, H. Li, X. Jiang and Y. Yan, Oxidative Desulfurization of Fuels Catalyzed by Peroxotungsten and Peroxomolybdenum Complexes in Ionic Liquids, Energy Fuels, 2007, 21, 2514–2516 CrossRef CAS.
- W. Zhu, H. Li, X. Jiang, Y. Yan, J. Lu, L. He and J. Xia, Commercially available molybdic compound-catalyzed ultra-deep desulfurization of fuels in ionic liquids, Green Chem., 2008, 10, 641–646 RSC.
- L. C. Caero, F. Jorge, A. Navarro and A. Gutiérrez-Alejandre, Oxidative desulfurization of synthetic diesel using supported catalysts : Part II. Effect of oxidant and nitrogen-compounds on extraction-oxidation process, Catal. Today, 2006, 116, 562–568 CrossRef.
- H. Li, W. Zhu, S. Zhu, J. Xia, Y. Chang, W. Jiang, M. Zhang, Y. Zhou and H. Li, The selectivity for sulfur removal from oils: An insight from conceptual density functional theory, AIChE J., 2016, 62, 2087–2100 CrossRef CAS.
- A. Nisar, Y. Lu, J. Zhuang and X. Wang, Polyoxometalate nanocone nanoreactors: magnetic manipulation and enhanced catalytic performance, Angew. Chem., Int. Ed., 2011, 50, 3187–3192 CrossRef CAS.
- X. Chang, X. F. Yang, Y. Qiao, S. Wang, M. H. Zhang, J. Xu, D. H. Wang and X. H. Bu, Confined Heteropoly Blues in Defected Zr-MOF (Bottle Around Ship) for High-Efficiency Oxidative Desulfurization, Small, 2020, 16, e1906432 CrossRef PubMed.
- X. Li, J. Zhang, F. Zhou, Y. Wang, X. Yuan and H. Wang, Oxidative desulfurization of dibenzothiophene and diesel by hydrogen peroxide: Catalysis of H3PMo12O40 immobilized on the ionic liquid modified SiO2, Mol. Catal., 2018, 452, 93–99 CrossRef CAS.
Footnotes |
† Electronic supplementary information (ESI) available. See DOI: 10.1039/d1qi01255j |
‡ These authors have contributed equally to this work. |
|
This journal is © the Partner Organisations 2022 |
Click here to see how this site uses Cookies. View our privacy policy here.