DOI:
10.1039/D4NR02361G
(Paper)
Nanoscale, 2025,
17, 1308-1316
Improvement of growth and lipid accumulation in microalgae with aggregation-induced emission-based nanomaterials towards sustainable lipid production†
Received
7th June 2024
, Accepted 25th November 2024
First published on 26th November 2024
Abstract
Microalgae are a hot research area owing to their promising applications for sustainable food, biofunctional compounds, and biofuel feedstock. However, low lipid content in algal biomass is still a challenge that needs to be resolved for commercial use. The current approaches are not satisfactory for achieving high growth and lipid accumulation in algal cells. This research aims to understand and evaluate the effects of light spectral shift on growth and lipid biosynthesis in a green microalga, Chlamydomonas reinhardtii. As a novel approach, an aggregation-induced emission luminogen (AIEgen), TPA-A (C21H19NO), was introduced into the culture media for tailoring the wavelength to a specific range to enhance photosynthesis and lipid production. Algal growth almost doubled at 10 μM TPA-A exposure compared to the control. A significant increase (*p < 0.05) in lipid accumulation was observed in the algal cells exposed to TPA-A. The elevated level of chlorophyll was attributed to fast algal growth. Furthermore, this luminogen was highly biocompatible (∼97% cell viability) on the HaCaT cell line at a concentration of 10 μM in under light conditions. No residues of TPA-A were detected after 7 days in culture media, indicating that this AIEgen was easily degradable. This AIE-based nanomaterial overcomes the conventional fluorophores’ aggregation-caused quenching effect by providing increased fluorescence with AIEgen. This approach for lipid induction with increased algal growth provides potential for the algal biofactory to produce sustainable bioproducts and eco-friendly biofuels.
1. Introduction
Photosynthetic microalgae have been a recent attraction of research for their essential biomolecules, specifically for their third- and fourth-generation feedstock and carbon-neutral biofuels.1–3 Biofuels from single-cell microalgae are considered an excellent alternative to stock-limited fossil fuels and a pragmatic solution for the recent price hike and future energy demand, without causing any interference with land-based crop production.4–6 Microalgae can minimise carbon emissions with 10–50 times higher efficiency than terrestrial plants.4 Furthermore, microalgae-derived polyunsaturated fatty acids (PUFAs) have various possible health-promoting effects with higher acceptability than PUFAs from other marine fish and shellfish.7,8 Apart from lipids, microalgae are an excellent source of different metabolites for biomedical and pharmaceutical applications.9,10 However, industry-scale microalgae culture for lipid and biomass production is still challenging due to its high operating costs.11 The lipid content in most microalgae can be maximised by applying physico-chemical stressors or nutrient starvation, which eventually inhibits cellular growth.12–14 Despite promising aspects, genetically engineered microalgal strains are associated with market acceptability and potential environmental hazards in an open culture system.15
Efficient light energy conversion during photosynthesis can promote the biomass yield and high-valued reaction products. The primary photosynthetic pigments in green microalgal photosystems are chlorophyll a and b, which are excited in the blue (chlorophyll a: 440 nm and chlorophyll b: 435 nm) and red regions (chlorophyll a: 660 nm and chlorophyll b: 643 nm) of photosynthetically active radiation (PAR, 400–700 nm).16,17 Spectral conversion to the acceptable range of light-harvesting molecules is an effective mechanism for the augmentation of photosynthesis. Some conventional luminogens have been investigated for growth and lipid production as spectral shift materials. However, partial light conversion, auto-absorption, strong background noise, low photostability, cytotoxicity in direct contact and weak fluorescence in solution due to aggregation-caused quenching (ACQ) eventually limited their application.18,19 Therefore, an effective fluorescent molecule with minimum drawbacks is needed to scale up industry-scale microalgal production.
In the past decade, with the discovery of the aggregation-induced emission (AIE) phenomenon, several AIE luminogens (AIEgens) have been synthesised with unique fluorescence properties. The recent advancements in AIE-based technologies offer greater prospects in microalgal research. AIE nanoparticles exhibit excellent light conversion efficiency in the aggregated state20 that can decrease the photon loss during light propagation in the microalgae culture medium. Moreover, the absence of ACQ effects, high photostability, superior brightness, low cytotoxicity, and long-term in situ retention ability have expanded their applications from augmented photosynthesis to image-guided photodynamic therapy.21
This study aims to improve the algal growth and lipid yield using a lipid-specific AIEgen, 4-(di-p-tolylamino) benzaldehyde (TPA-A), which can be synthesised from cheap materials with simple synthetic steps. This study used a model species of green microalgae, Chlamydomonas reinhardtii, to monitor lipid production performance after light wavelength conversion in the culture medium. This study reveals the suitability of TPA-A as an effective light spectral shift nanomaterial and a lipid bio-imaging tool to improve photosynthesis and lipid visualisation in the microalgal cells toward sustainability.
2. Experimental
2.1. Microalga culture
A pure strain of C. reinhardtii was obtained from the Biology Discovery Centre, Flinders University, Australia. The culture was established in a modified Wood Hole (MBL) medium22 after autoclaving at 121 °C for 15 min. The 1 L culture medium contained CaCl2·2H2O (0.04 g), MgSO4·7H2O (0.04 g), NaHCO3 (0.01 g), K2HPO4 (0.008 g), NaNO3 (0.08 g), EDTA-Na (0.004 g), FeCl3·6H2O (0.004 g), CuSO4·5H2O (0.01 mg), ZnSO4·7H2O (0.02 g), CoCl2·6H2O (0.01 mg), MnCl2·4H2O (0.02 mg), Na2MoO4·2H2O (0.006 mg), C63H88CoN14O14P (vitamin B12, 0.0005 mg), C12H17ClN4OS·HCl (vitamin B1, 0.1 mg), C10H16N2O3S (biotin, 0.0005 mg) and C4H11NO3 (tris(hydroxymethyl)aminomethane, 0.25 g). The pH of the culture medium was adjusted to 7.2. The culture was maintained under continuous white light illumination (LED) with a light intensity of 50 μmol photons per m2 per s with continuous rotation at 100 rpm. The temperature was set to 25 °C. All required reagents for the MBL culture medium were obtained from Thermo Fisher Scientific, Australia.
2.2. Determination of fluorescence spectra of TPA-A
The absorption and photoluminescence (PL) spectra of TPA-A in DMSO/water mixtures were recorded using a fluorescence spectrophotometer (Cary Eclipse, MY17180002, Agilent Technologies, CA, USA) with quartz cuvettes of 1 cm path length. Fluorescence spectra of 10 μM TPA-A in DMSO (Sigma-Aldrich, Australia) and 60–99% water fractions (fw) were measured.
Spectrophotometric analysis of the fluorescence intensity profile of 10 μM TPA-A at 99% fw was performed on different days to determine the functional stability of TPA-A. Confocal analysis was conducted using a Zeiss LSM 880 Airyscan confocal microscope on day 4 algal culture in 10 μM TPA-A to confirm the TPA-A stability. The excitation and emission for the TPA-A channel were set at 405 nm and 410–597 nm, respectively.
2.3. Growth and biomass analysis of C. reinhardtii
To determine the effect of TPA-A concentrations on C. reinhardtii growth, 1.0 ± 0.001 (×104) cells were introduced into the culture medium under the pre-defined conditions. Different TPA-A concentrations (5, 10 and 20 μM) dissolved in DMSO were introduced directly to the C. reinhardtii culture as treatments. The culture with no TPA-A was used as the control. All cultures were conducted with three replications (n = 3). The growth rate of C. reinhardtii was determined by counting the cells with a haemocytometer (Improved Marienfeld Neubauer, Germany) under a light microscope at regular intervals for up to five days.
For biomass analysis, the 7 day culture of 1.0 L C. reinhardtii cells was centrifuged at 4000g for 5 min. The cell suspension was stored at 180 °C for 24 h before freeze drying for 48 h. The freeze-dried samples were weighed and immediately stored at −20 °C for further analysis.
2.4. Fluorescence staining and confocal analysis of lipid accumulation
The autofluorescence of chlorophyll in C. reinhardtii was determined to avoid interference with lipid droplets (LDs) during fluorescence staining (Fig. S1†). Confocal imaging with ZEN 2.6 software (Carl Zeiss, Australia) was used to determine the effect of TPA-A concentrations on lipid accumulation in C. reinhardtii cells. After a 7 day culture, algal cells were centrifuged to adjust at 106 cells per mL and stained with 10 μM lipid-specific TPA-A for 20 min in the dark. The final concentration of DMSO was adjusted to 0.1%. The lipid specificity of TPA-A was previously determined (Fig. S2†). The samples were subsequently washed with deionised water followed by centrifugation (2000g, 60 s) and further resuspended in distilled water. For confocal analysis, based on the pre-determined autofluorescence properties of C. reinhardtii, excitation and emission of TPA-A were set at 405 nm and 410–597 nm, respectively. The relative fluorescence per cell at 0, 5, 10 and 20 μM TPA-A was calculated to determine the lipid accumulation and chlorophyll content in algal cells.
2.5. Lipid extraction and fatty acid analysis
Microalgal lipids were extracted using an adapted method.23,24 Lyophilised biomass from different treatments was weighed to 10 mg and dissolved in 50 μL of 1.5% NaCl buffer. An organic solvent (1 mL) of 2
:
1 chloroform and methanol (v/v) was then added and homogenised thoroughly for 10 min, followed by incubation at room temperature for 10 min. An additional 200 μL of 1.5% NaCl buffer was added and homogenised thoroughly for 5 min. Phase separation was achieved by centrifugation at 6000g for 5 min. Of the organic phase, 500 μL was extracted and concentrated by nitrogen gas evaporation. Dried lipid extracts were determined gravimetrically and stored at −20 °C for further gas chromatography and mass spectrometry (GC-MS) analysis of fatty acids.
For GC-MS, dried lipid extracts were resuspended in 1
:
1 chloroform and trimethylsulphonium hydroxide (v/v) to generate fatty acid methyl esters (FAMEs). FAMEs were then subjected to analysis using an Agilent 7890A GC system with a 30 m Agilent DB-FastFAME column (Agilent Technologies) coupled with an Agilent 5975C MSD system (Agilent Technologies) to complete mass spectrometry. FAME species were identified relative to a FAME mix C4–C24 standard (Sigma-Aldrich).
2.6. Cytotoxicity analysis of TPA-A
To determine the cytotoxicity of TPA-A in living cells, the 3-(4,5-dimethyl 2-thiazolyl)-2,5-diphenyltetrazolium bromide (MTT) assay was performed according to Zerboni et al.,25 with slight modification. HaCaT cell lines were cultured in 10 μM TPA-A as a treatment and 0.2% DMSO as a control for 24 h. The optical density (OD) was measured by enzyme-linked immunosorbent assay (ELISA) at 490 nm. The cell inhibitory rate was determined by using the formula: Cell inhibitory rate (%) = (1 − OD treatment/OD control) × 100.
2.7. Data analysis
The confocal microscopy data were analysed with ImageJ 1.52a software.26 In brief, the raw images were exported to ImageJ in tiff format. After subtracting the background from each image using a sliding paraboloid, the fluorescence channel was calculated to determine the area, area fraction mean grey value, and integrated density. The relative fluorescence intensity per cell was determined from the total cells by subtracting the background IntDen value from the IntDen value. Differences between the control and other treatments were calculated with SPSS statistics software (version 23) at the p < 0.05 level through the paired sample t-test.
3. Results and discussion
3.1. Photophysical properties of TPA-A
The absorption spectrum of 10 μM TPA-A in a DMSO–water mixture at 0–99% fw ranged from 330 to 420 nm with a gradual red-shift pattern at 90% and 99% fw (Fig. 1a). The normalized PL intensity was recorded from 420 to 650 nm with a slight blue-shift emission peak at 490 nm at 99% fw (Fig. 1b). These results revealed the distinguishable absorption and emission spectra with a significant stokes shift. The emissions of TPA-A were quenched up to 70% fw. The emission intensity rapidly increased with the increase of fw (Fig. 1c), suggesting the typical AIE properties of TPA-A.
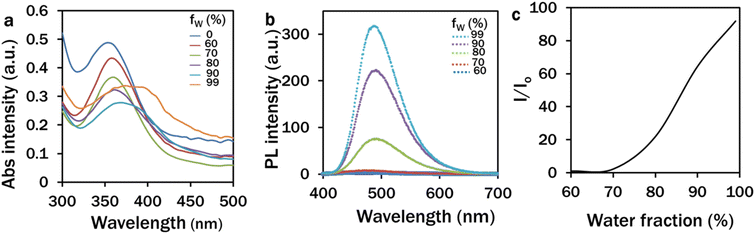 |
| Fig. 1 Photophysical properties of 10 μM TPA-A in DMSO–water mixtures at different water fractions (a–c). (a) Absorbance spectra of TPA-A; (b) and (c) PL intensities of TPA-A. | |
The spectrophotometric analysis of the PL intensity of 10 μM TPA-A in DMSO–water mixtures (99% fw) on different consecutive days suggested that the TPA-A remained functional for three days (Fig. 2a). The confocal analysis of the 4 day C. reinhardtii culture showed that the TPA-A channel was completely dark with no fluorescence (Fig. 2d).
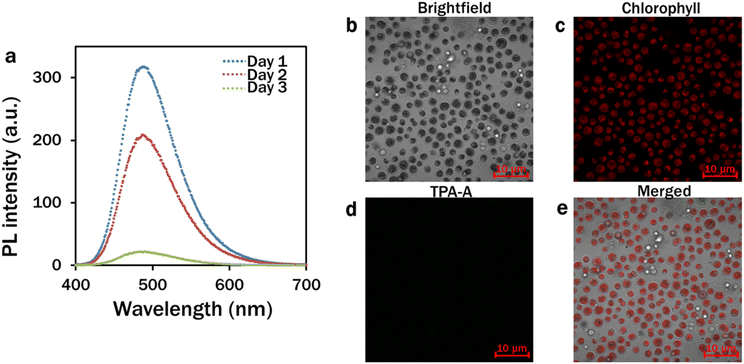 |
| Fig. 2 Stability of TPA-A at different days. (a) Spectrophotometric analysis of the fluorescence intensity of TPA-A at 99% fw (λex: 380 nm); (b–e) confocal analysis of TPA-A fluorescence after 4-day culture of C. reinhardtii with 10 μM TPA-A. (b) Brightfield; (c) chlorophyll: λex: 488 nm and λem: 685–758 nm; (d) TPA-A: λex: 405 nm and λem: 410–597 nm; and (e) merged images. | |
Upon excitation at 380 nm, TPA-A converted UV light to blue light with a peak at 490 nm for further absorption by chlorophyll a and b in the reaction centres during photosynthesis. Red light with narrower spectral outputs is more efficient for photosynthesis.27–29 However, the photons of blue light exhibit higher energy (2.8 eV per photon) than those of red light (1.8 eV per photon) to mediate effective charge separation from pigment molecules in the electron transport chain of the photosynthesis pathway.30 In addition, a higher plant-like phototropin homolog has been identified in C. reinhardtii, and the basic action mechanism is highly conserved in strong blue light for chloroplast avoidance movement to protect the photosynthetic apparatus for augmenting photosynthesis.31,32 Moreover, outside the PAR, the extended absorption of TPA-A to the UV range has provided adequate energy for photosynthesis, avoiding the UV-associated DNA and protein damage of microalgal cells. Different conventional luminogens have been reported as light spectral shift materials with low biomass yields at indirect contact with algae and plant cells.33,34 Most of these luminogens have overlapped excitation and emission wavelengths with minimum stokes shift, leading to the self-absorption of fluorescence and do not play a substantial role in the effective spectral shift. Being an AIE nanomaterial, upon excitation at an appropriate wavelength, TPA-A exhibited distinguishable absorption and emission spectra (Fig. 1a and b). In comparison with the commercially used BODIPY 505/515 dye, which typically has a stokes shift of 5–15 nm, TPA-A has a larger stokes shift of 122 nm35,36 that eventually reveals its potentiality as an effective spectral shift material for light harvesting and energy conversion.
3.2. Effects of TPA-A on growth and biomass of C. reinhardtii
After a 5 day continuous culture, maximum growth of 50 × 104 cells per ml occurred in the 10 μM TPA-A treated culture, followed by 5, 20 and 0 (control) μM TPA-A (Fig. 3a). The growth curve followed a similar pattern for the following consecutive days except for a slight decrease after day 2 in the 10 μM TPA-A culture, which was possibly due to the decrease of functional stability of TPA-A. Dry biomass from different concentrations of TPA-A treatments was recorded with a maximum yield of 0.2 g L−1 for the 10 μM TPA-A exposed cells (Fig. 3b). Compared to the control (0.1 g L−1), biomass production doubled in 10 μM TPA-A.
 |
| Fig. 3 Growth and biomass production in C. reinhardtii at different concentrations of TPA-A. (a) Growth of C. reinhardtii cells (×104) for 5 days and (b) dry biomass (g L−1) at different concentrations of TPA-A. Data are presented as the mean ± SE (*p < 0.05, **p < 0.01). | |
Microalgal growth enhancement with other different AIEgens has also been reported for their commercial appeal.37,38 As a triphenylamine-based material, TPA-A is a strong electron donor and can facilitate intramolecular charge transfer (ICT) for a more extended emission range, providing a potential approach for photosynthetic pigments to absorb more energy for algal growth and lipid biosynthesis. This entrapped higher energy enters the electron transport chain in the thylakoid lumen to produce a subsequent amount of adenosine triphosphate (ATP) in the presence of light. We maintained C. reinhardtii culture under continuous light conditions that might enact ferredoxin:NADP+ reductase (FNR) for electron cycling from ferredoxin to NADP, since the activity of FNR is inhibited in the dark.39
3.3. TPA-A induced lipid accumulation and fatty acid proliferation
In our study, TPA-A significantly (*p < 0.05 and **p < 0.01) induced lipid accumulation in C. reinhardtii cells. In the control, lipid accumulation was insignificant in C. reinhardtii cells as the TPA-A channel had the least fluorescence upon excitation at an appropriate wavelength (Fig. 4c). The C. reinhardtii cells cultured at 5 μM TPA-A concentration had comparatively higher lipid accumulation than that of the control with brighter fluorescence in the TPA-A channel (Fig. 4g). The brightest fluorescence at 10 μM concentration revealed that TPA-A at 10 μM concentration provided the best lipid accumulation in C. reinhardtii cells (Fig. 4k). Relative fluorescence per cell at 0, 5, 10 and 20 μM TPA-A was calculated to determine the cells’ relative lipid and chlorophyll contents. The result showed that the chlorophyll content was higher in both treatments with 5 and 10 μM TPA-A, and the maximum lipid accumulation was observed in the treatment with 10 μM TPA-A (Fig. 4q).
 |
| Fig. 4 Confocal analysis of lipid drops and chlorophyll in C. reinhardtii. The cells were cultured in MBL medium with 0 μM (a–d), 5 μM (e–h), 10 μM (i–l) and 20 μM (m–p) TPA-A for 7 days. Lipid drops were labelled with 10 μM TPA-A, 20 min incubation in the dark. Brightfield (a, e, i and m); chlorophyll (b, f, j and n): λex: 488 nm and λem: 685–758 nm; TPA-A (c, g, k and o): λex: 405 nm and λem: 410–597 nm; and merged images (d, h, l and p); (q) relative fluorescence intensity per cell. Values are relative to the control. Data are presented as the mean ± SE; (*p < 0.05, **p < 0.01). | |
In comparison with the control, an almost 2-fold upturn in the total crude lipid production was observed in 10 μM TPA-A-exposed 7 day culture cells and measured as 14.36 ± 1.92% dry weight (DW) and 30.65 ± 2.09% DW, respectively (Fig. 5).
 |
| Fig. 5 Total lipid production (% DW) at different TPA-A concentrations. Data are presented as the mean ± SE (*p < 0.05). | |
Among the fatty acid (FA) groups, saturated fatty acids (SFAs) upsurged by ∼15.75% in comparison with that of the control and dominated by palmitic acid (C16:0) and margaric acid (C17:1), the precursors of biodiesel production (Table 1). Increased accumulation of health beneficiary oleic acid (C18:1) and α-linoleic acid (C18:3) was outweighed in the mono and polyunsaturated groups (MUFAs and PUFAs) (Table 1).
Table 1 Percentage of FAMEs in Chlamydomonas reinhardtii cells at different TPA-A concentrations
FAMEs (%) |
TPA-A treatments |
0 μM |
5 μM |
10 μM |
20 μM |
Non-detectable.
|
C8:0 |
0.53 ± 0.17 |
1.98 ± 0.13 |
1.12 ± 0.2 |
1.56 ± 0.14 |
C10:0 |
0.12 ± 0.11 |
0.13 ± 0.08 |
0.18 ± 0.15 |
0.15 ± 0.42 |
C11:0 |
0.13 ± 0.04 |
0.16 ± 0.11 |
0.16 ± 0.03 |
|
C12:0 |
|
0.12 ± 0.07 |
0.15 ± 0.17 |
0.11 ± 0.03 |
C13:0 |
0.11 ± 0.02 |
0.12 ± 0.03 |
0.13 ± 0.02 |
0.15 ± 0.02 |
C14:0 |
0.18 ± 0.02 |
0.33 ± 0.05 |
0.41 ± 0.04 |
0.2 ± 0.21 |
C14:1 |
0.29 ± 0.07 |
0.41 ± 0.1 |
0.81 ± 0.31 |
0.57 ± 0.13 |
C15:0 |
|
0.19 ± 0.21 |
0.12 ± 0.01 |
|
C15:1 |
0.18 ± 0.14 |
1.19 ± 0.13 |
2.32 ± 0.22 |
1.5 ± 0.13 |
C16:0 |
14.32 ± 1.05 |
17.09 ± 1.08 |
23.09 ± 2.23
|
16.84 ± 0.74 |
C16:1 |
7.01 ± 0.52 |
4.80 ± 0.74 |
2.63 ± 1.19 |
5.25 ± 0.31 |
C17:0 |
6.29 ± 1.32 |
5.81 ± 1.27 |
5.16 ± 1.32 |
6.04 ± 1.14 |
C17:1 |
12.53 ± 0.87 |
14.15 ± 1.73 |
16.17 ± 2.02
|
11.89 ± 1.51 |
C18:0 |
4.02 ± 0.62 |
2.13 ± 0.68 |
1.4 ± 0.32 |
2.87 ± 0.73 |
C18:1 |
11.15 ± 0.92 |
13.94 ± 2.12 |
14.3 ± 2.77 |
12.73 ± 1.45 |
C18:2 |
16.65 ± 1.93 |
14.34 ± 1.72 |
9.56 ± 2.08 |
15.48 ± 1.42 |
C18:3 |
17.97 ± 1.68 |
19.12 ± 2.14 |
23.65 ± 2.71
|
18.06 ± 1.71 |
C20:0 |
1.31 ± 0.02 |
0.20 ± 0.01 |
0.17 ± 0.01 |
0.19 ± 0.21 |
C20:1 |
2.72 ± 0.05 |
0.58 ± 0.07 |
|
0.26 ± 0.08 |
C20:3 |
1.38 ± 0.04 |
0.32 ± 0.03 |
0.32 ± 0.01 |
0.35 ± 0.09 |
C23:0 |
|
0.37 ± 0.74 |
0.48 ± 0.01 |
|
C24:0 |
|
0.29 ± 0.01 |
1.86 ± 0.06 |
0.19 ± 0.07 |
C24:1 |
|
1.58 ± 1.73 |
2.72 ± 0.74 |
|
SAFAs |
38.66 ± 6.29 |
40.05 ± 5.05 |
44.75 ± 4.95
|
39.03 ± 5.01 |
MUFAs |
22.69 ± 6.07 |
23.8 ± 5.05 |
24.47 ± 4.35 |
22.9 ± 5.26 |
PUFAs |
32.67 ± 5.01 |
31.46 ± 3.05 |
30.85 ± 4.03 |
32.05 ± 6.43 |
Although this study exhibited a promising upsurge in lipid and FA production, the exact mechanisms could not be fully elucidated due to the complex regulatory pathways of lipid biogenesis in microalgal cells.40 Herein, the maximum lipid production at 10 μM TPA-A exposed cells was perhaps due to the increased supply of high reducing power in the form of NADPH in the mevalonic acid (MVA) pathway with possible modulation of the competing biosynthesis pathways of other biomolecules.41 As an efficient light-shifting material with an extended emission range, TPA-A provided additional light density for photosynthetic pigments to absorb more energy to reserve ATP and NADPH for lipid biosynthesis. In contrast, the declined lipid accumulation at the 20 μM TPA-A exposed cells was possibly due to the excess light density at this concentration. In excess light intensity, different photoreceptors, such as phototropin, neochrome, phytochromes, rhodopsins, and cryptochromes, participate in chloroplast avoidance movement to avoid cell damage, where the chlorophyll moves towards the cell wall from the surface to reduce the photosynthesis, and hence decrease the lipid production. In the present study, we observed a significant amount of chlorophyll at both 5 and 10 μM TPA-A-exposed C. reinhardtii cells (Fig. 4q). In contrast, downregulation of the chlorophyll content with elevated lipid proliferation was previously reported.42 The declined level of this photosynthetic apparatus is due to its utilisation as reserved nitrogen for stress alleviation during lipid biogenesis.43,44 Moreover, adequate ATP reserve due to the AIE nanomaterial-mediated efficient light utilisation facilitates further rapid chlorophyll synthesis from the glutamate precursor in the case of any stress-mediated decline. Therefore, the introduction of AIE nanomaterials in microalgal culture is a novel approach to induce growth and lipid accumulation without nutrient deprivation and associated stress factors.
In addition, to tailor growth and lipid accumulation in C. reinhardtii, TPA-A is an effective bio-imaging tool for detecting lipid droplets in microalgal cells. Due to the AIE properties, TPA-A has the advantage of lipid visualisation in microalgal cells by fluorescence imaging. The red-shifted absorption spectrum with the increase of water fraction (Fig. 1a) was resulted from the increased polarity and the twisted intramolecular charge transfer (TICT). The addition of two methyl groups can integrate strong D–A interaction and restrict intramolecular π–π interaction to activate the radiative decay channels and yield the unique fluorescence properties of AIEgens.20,45,46 The rapid increase of the PL intensity with the increase of fw from 60–99% (Fig. 1b and c) was due to the nano-aggregation of TPA-A in the DMSO–water mixture without aggregation-caused quenching (ACQ). The lipid specificity of TPA-A was previously determined with the commercial lipid-specific probe Nile Red with a colocalisation efficiency of 0.88.36 Furthermore, we investigated its lipid specificity with 2% sunflower oil under a confocal microscope (Fig. S2†). Compared to conventional lipid probes like BODIPY or Nile Red, TPA-A exhibited its suitability for microalgal lipid staining due to the absence of background interference with the chlorophyll channel and auto-absorption. Moreover, TPA-A remains stable after 80 laser scans, whereas Nile Red and BODIPY lose 95% and 90% of their signals after 60 scans, respectively.21,36,47
3.4. Cytotoxicity analysis of TPA-A
In our experiment, the TPA-A nanomaterial was biocompatible with C. reinhardtii at 10 μM as the growth rate was the maximum at this concentration. However, growth and lipid production declined when the cell was cultured with 20 μM TPA-A. Therefore, the 10 μM TPA-A concentration was chosen to further determine the cytotoxicity of 10 μM TPA-A on HaCaT cell lines with the MTT assay. Compared to the 100% cell viability in the control, 97.37% was found in the 10 μM TPA-A-treated cells, indicating high biocompatibility of this AIEgen on living cells (Fig. 6).
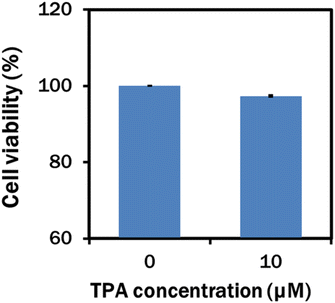 |
| Fig. 6 Cell viability (%) of HaCaT cells incubated with 10 μM TPA-A for 24 h before the MTT assay. | |
In our study, TAP-A was directly added to the culture media for the effective light spectral shift. The luminogens of coumarin derivatives, laser dye and photoluminescent phosphor (Ca0.59Sr0.40Eu0.01S) are not suitable for direct application due to their minimum biocompatibility in living cells or growth impairment.33,48 GCMS analysis of 10 μM TPA-A at day 0 and day 7 showed that the functional molecules of TPA-A were completely diminished after 7 days (Fig. S3–S5†), therefore suggesting that TPA-A can be used as a degradable, non-hazardous and environment friendly nano-aggregate for commercial biomass and lipid production in microalgae.
4. Conclusions
This study identified the synergic effect of AIE-based TPA-A nanomaterials for growth and lipid proliferation in C. reinhardtii under laboratory conditions. The efficient light conversion from UV to blue with a wide emission range enabled the light-harvesting pigments to utilise light energy beyond the PAR by avoiding UV-associated damage. The prolonged photostability, strong biocompatibility, and high lipid targeting ability of TPA-A ensure its further applications in biomedical research for in vivo lipid visualisation. After extraction of different biomolecules, the remnant can be used as a safe protein-enriched food and feed and a mineral-enriched biofertiliser. Additionally, TPA-A can be synthesised from cheap materials involving simple synthetic steps. Thus, it is a cost-effective AIE nanomaterial for enhancing sustainable food and eco-friendly biofuel production.
Author contributions
Sharmin Ferdewsi Rakhi: investigation, data curation, methodology, and writing – original draft. AHM Mohsinul Reza: investigation, writing – review and editing, and data curation. Brynley Davies: investigation and writing – review and editing, Jianzhong Wang: investigation and data curation, Jianguang Qin: conceptualisation, supervision, and writing – review and editing, Youhong Tang: conceptualisation, supervision, and writing – review and editing. All authors contributed to the final manuscript.
Data availability
The data supporting this article have been included as part of the ESI.†
Conflicts of interest
There is no conflict to declare.
Acknowledgements
The authors want to thank Dr Haixiang Liu, Shenzhen Research Institute, Hong Kong University of Science and Technology for synthesising and purifying the TPA-A. S. F. Rakhi is grateful for the financial support from the Flinders University Research Scholarship (FURS) for her study at Flinders University, Australia.
References
- J. V. Gerpen, Fuel Process. Technol., 2005, 86, 1097–1107 CrossRef.
- I. M. Atadashi, M. K. Aroua and A. A. Aziz, Renewable Sustainable Energy Rev., 2010, 14, 1999–2008 CrossRef CAS.
- A. E. Atabani, A. S. Silitonga, I. A. Badruddin, T. M. I. Mahlia, H. H. Masjuki and S. A. Mekhilef, Renewable Sustainable Energy Rev., 2012, 16, 2070–2093 CrossRef.
- A. Raheem, A. W. A. K. G. Wan, Y. Y. H. Taufiq, M. K. Danquah and R. Harun, Renewable Sustainable Energy Rev., 2015, 49, 990–999 CrossRef CAS.
- M. V. Rodionova, R. S. Poudyal, I. Tiwari, R. A. Voloshin, S. K. Zharmukhamedov, H. G. Nam, B. K. Zayadan, B. D. Bruce, H. J. M. Hou and S. I. Allakhverdiev, Int. J. Hydrogen Energy, 2017, 42, 8450–8461 CrossRef CAS.
- S. Eyley, D. Vandamme, S. Lama, G. Mooter, K. Muylaert and W. Thielemans, Nanoscale, 2015, 7, 14413 RSC.
- A. Kusmayadi, Y. K. Leong, H. W. Yen, C. Y. Huang and J. S. Chang, Chemosphere, 2021, 271, 129800 CrossRef CAS PubMed.
- E. E. M. Luiten, I. Akkerman, A. Koulman, P. Kamermans, H. Reith, M. J. Barbosa, D. Sipkema and R. H. Wijffels, Biomol. Eng., 2003, 20, 429–439 CrossRef CAS PubMed.
- F. Khavari, M. Saidijam, M. Taheri and F. Nouri, Mol. Biol. Rep., 2021, 48, 4757–4765 CrossRef CAS PubMed.
- J. Talukdar, B. Bhadra, T. Dattaroy, V. Nagle and S. Dasgupta, Biomed. Pharmacother., 2020, 132, 110886 CrossRef CAS PubMed.
- N. Lesniewska, J. F. L. Duval, C. Caillet, A. Razafitianamaharavo, J. P. Pinheiro, I. Bihannic, R. Gley, H. L. Cordier, V. Vyas, C. Pagnout, B. Sohm and A. Beaussart, Nanoscale, 2024, 16, 5149–5163 RSC.
- Y. Gao, M. Yang and C. Wang, Bioresour. Technol., 2013, 147, 484–491 CrossRef CAS PubMed.
- E. B. Sydney, T. E. Silva, A. Tokarski, A. C. Novak, J. C. Carvalho de, A. L. Woiciecohwski, C. Larroche and C. R. Soccol, Appl. Energy, 2011, 88, 3291–3294 CrossRef CAS.
- T. M. Mata, A. A. Martins and N. S. Caetano, Renewable Sustainable Energy Rev., 2010, 14, 217–222 CrossRef CAS.
- P. Singh, S. Kumari, A. Guldhe, R. Misra, I. Rawat and F. Bux, Renewable Sustainable Energy Rev., 2016, 55, 1–16 CrossRef CAS.
- A. A. Fernandez-Jaramillo, C. Duarte-Galvan, L. M. Contreras-Medina, I. Torres-Pacheco, R. D. J. Romero-Troncoso, R. G. Guevara-Gonzalez and J. R. Millan-Almaraz, Sensors, 2012, 12, 11853–11869 CrossRef CAS PubMed.
- G. Verhoeven, AARGnews, 2017, 55, 13–18 Search PubMed.
- P. Greenspan, E. P. Mayer and S. D. Fowler, J. Cell Biol., 1985, 100, 965–973 CrossRef CAS PubMed.
- S. Daemen, M. A. M. J. van Zandvoort, S. H. Parekh and M. K. C. Hesselink, Mol. Metab., 2015, 5, 153–163 CrossRef PubMed.
- Z. Zhao, B. He and B. Z. Tang, Chem. Sci., 2015, 6, 5347–5365 RSC.
- Z. Wang, Z. Engui, E. Zhao, J. Wang, X. Li, A. Qin, Z. Zhao, Z. Yu and B. Z. Tang, ACS Appl. Mater. Interfaces, 2016, 8, 10193–10200 CrossRef CAS PubMed.
-
H. W. Nichols, Handbook of Physiological Methods, ed. J. R. Stein Camb. Univ. Press, United Kingdom, 1973, pp. 16–17 Search PubMed.
- E. G. Bligh and W. J. Dyer, Can. J. Biochem. Physiol., 1959, 37, 911 CrossRef CAS PubMed.
- M. Zang, A. Ascari, F. G. Adams, S. Alquethamy and B. A. Eijkelkamp, Cell Surf., 2022, 9, 100092 CrossRef PubMed.
- A. Zerboni, R. Bengalli, G. Baeri, L. Fiandra, T. Catelani and P. Mantecca, Nanomaterials, 2019, 9, 1302 CrossRef CAS PubMed.
- A. H. M. M. Reza, Y. Zhou, J. Tavakoli, Y. Tang and J. Qin, Mater. Chem. Front., 2021, 5, 268–283 RSC.
- T. H. Kim, Y. Lee, S. H. Han and S. J. Hwang, Bioresour. Technol., 2013, 130, 75–80 CrossRef CAS PubMed.
- A. Khalili, G. D. Najafpour, G. Amini and F. Samkhaniyani, Biotechnol. Bioprocess Eng., 2015, 20, 284–290 CrossRef CAS.
- A. P. Carvalho, S. O. Silva, J. M. Baptista and F. X. Malcata, Appl. Microbiol. Biotechnol., 2011, 89, 1275–1288 CrossRef CAS PubMed.
- N. Sharma, G. Fleurent, F. Awwad, M. Cheng, F. Meddeb-Mouelhi, S. M. Budge, H. Germain and I. Desgagné-Penix, Appl. Sci., 2020, 10, 2531 CrossRef CAS.
- E. G. Govorunova, K. H. Jung, O. A. Sineshchekov and J. L. Spudich, Biophys. J., 2004, 86, 2342–2349 CrossRef CAS PubMed.
- P. Hermanowicz, A. K. Banaś, O. Sztatelman, H. Gabryś and J. Łabuz, Front. Plant Sci., 2019, 10, 1279–1291 CrossRef PubMed.
- A. Prokop, M. F. Quinn, M. Fekri, M. Murad and S. A. Ahmed, Biotechnol. Bioeng., 1984, 26, 1313–1322 CrossRef CAS PubMed.
- L. Shen, R. Lou, Y. Park, Y. Guo, E. J. Stallknecht, Y. Xiao, D. Rieder, R. Yang, E. S. Runkle and X. Yin, Nat. Food, 2021, 2, 434–441 CrossRef PubMed.
- A. Loudet and K. Burgess, Chem. Rev., 2007, 107, 4891–4932 CrossRef CAS PubMed.
- H. Liu, N. Yan, T. Y. Wong, H. Lam, J. W. Y. Lam, R. T. K. Kwok, J. Sun and B. Z. Tang, ACS Nano, 2022, 16, 14973–14981 CrossRef CAS PubMed.
- H. T. Bai, H. X. Liu, X. Chen, R. Hu, M. Li, W. He, J. Du, Z. Y. Liu, A. J. Qin, J. W. Y. Lam, R. T. K. Kwok and B. Z. Tang, Mater. Horiz., 2021, 5, 1433–1438 RSC.
- H. Liu, H. Bai, N. Yan, T. Y. Wong, D. Dang, J. S. Ni, J. W. Y. Lam, H. Lam, R. T. K. Kwok, W. X. Wang and B. Z. Tang, ACS Sustainable Chem. Eng., 2021, 9, 15258–15266 CrossRef CAS.
- E. Talts, V. Oja, H. Rämma, B. Rasulov, A. Anijalg and A. Laisk, Photosynth. Res., 2007, 94, 109–120 CrossRef CAS PubMed.
- B. Liu and C. Benning, Curr. Opin. Biotechnol., 2013, 24, 300 CrossRef CAS PubMed.
- X. Sun, L. Ren, Q. Zhao, X. Ji and H. Huang, Biochim. Biophys. Acta, Mol. Cell Biol. Lipids, 2019, 1864, 552–566 CrossRef CAS PubMed.
- A. H. M. M. Reza, S. F. Rakhi, X. Zhu, Y. Tang and J. Qin, Biosensors, 2022, 12, 208–232 CrossRef CAS PubMed.
- R. Miller, G. Wu, R. R. Deshpande, A. Vieler, K. Gärtner, X. Li, E. R. Moellering, S. Zäuner, A. J. Cornish and B. Liu, Plant Physiol., 2010, 154, 1737–1752 CrossRef CAS PubMed.
- C. Shene, J. A. Asenjo and Y. Chisti, Plant J., 2018, 96, 1076–1088 CrossRef CAS PubMed.
- Y. Hong, J. W. Lam and B. Z. Tang, Chem. Commun., 2009, 29, 4332–4353 RSC.
- D. Wang, M. M. S. Lee, G. Shan, R. T. K. Kwok and J. W. Y. Lam, Adv. Mater., 2018, 30, 1802105 CrossRef PubMed.
- L. Li, F. Zhou, Q. Gao, Y. Lu, X. Xu, R. Hu, Z. Wang, M. Peng, Z. Yang and B. Z. Tang, iScience, 2019, 21, 261–272 CrossRef CAS PubMed.
- L. Wondraczek, M. Batentschuk, M. A. Schmidt, R. Borchardt, S. Scheiner, B. Seemann, P. Schweizer and C. J. Brabec, Nat. Commun., 2013, 4, 2047 CrossRef PubMed.
|
This journal is © The Royal Society of Chemistry 2025 |
Click here to see how this site uses Cookies. View our privacy policy here.