DOI:
10.1039/C5QI00059A
(Review Article)
Inorg. Chem. Front., 2015,
2, 687-712
Nanoscopic molecular magnets
Received
10th April 2015
, Accepted 15th June 2015
First published on 16th June 2015
Abstract
Nanoscopic molecular magnets have attracted tremendous interest in recent years both from an experimental and a theoretical point of view because of their potential application in magnetic data storage devices, quantum computing and molecular spintronics. These molecules have crucial advantages over magnetic nanoparticles in terms of their perfectly mono-dispersed phase, chemical flexibility and high purity. This review discusses a few basic concepts that are needed to understand the magnetic properties observed in nanoscopic molecular magnets, a chemical approach for their synthesis and magneto-structural correlations. It includes a few selected examples and discusses the new trends in the field.
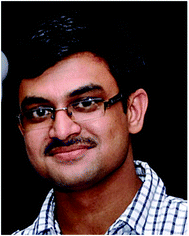 Soumyabrata Goswami | Soumyabrata Goswami obtained his B.Sc. from St. Paul's C.M. College, University of Calcutta in 2008 and M.Sc. from the University of Calcutta in 2010. Currently, he is pursuing his PhD at IISER Bhopal under the supervision of Dr Sanjit Konar. His research interests are the synthesis and characterization of transition and lanthanide metal based nanoscopic molecular magnets and multifunctional molecular materials. |
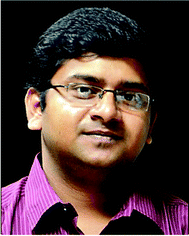 Amit Kumar Mondal | Amit Kr. Mondal obtained his B.Sc. from RKM Vidyamandira, University of Calcutta in 2010 and M.Sc. from IIT Guwahati in 2012. Currently, he is pursuing his Ph.D. at IISER Bhopal under the supervision of Dr Sanjit Konar. His research interests include the synthesis and study of slow magnetic relaxation in lanthanide-based molecular magnetic materials and spin-crossover behaviour in transition metal complexes. |
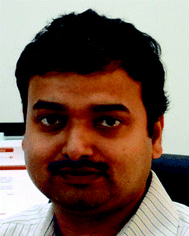 Sanjit Konar | Sanjit Konar received his Ph.D. degree from the Indian Association for the Cultivation of Science, Kolkata. He worked as a postdoctoral fellow at the University of Notre Dame and Texas A&M University, College Station, TX, USA. He is a recipient of an Alexander von Humboldt Fellowship and has worked at Universität Bielefeld, Germany. Currently he is working as an Associate Professor at the Department of Chemistry, IISER Bhopal. His research interests are in the area of molecular magnetic materials and multifunctional metal organic frameworks. He has published 70 peer-reviewed journal articles. |
1. Introduction
Study of nanoscopic molecular magnets is a very attractive area of research dealing with the magnetic properties of objects which have at least one dimension in the nanoscopic range (1–100 nm). It includes the detailed investigation and interpretation of the magnetic properties of nanoparticles as well as molecular nanomagnets. Explosive growth in research on molecular magnetic materials in the last two decades1 has been initiated by the discovery of some nanoscopic magnetic cage complexes like dodecametallic mixed valent manganeseIII,IV-acetate, [Mn12O12(OAc)16(H2O)4] (Mn12Ac),2 which has been termed as a Single Molecular Magnet (SMM).3 The discovery of these molecules ignited great hope that a piece of information could be stored in a single molecule and launched an active research field in chemistry as well as in physics. Ideally an SMM can be considered as a nanoscopic molecular magnet synthesized following a bottom up approach and should contain a finite number of bridging paramagnetic metal centres. These large molecules are at the interface of the classical and quantum worlds and combine the classical macroscale properties of a magnet with the quantum properties of a nanoscale entity.4 Physically these molecules are mostly zero dimensional and consist of a paramagnetic hydrophilic core (mostly metal-oxo) (Scheme 1) encapsulated in a diamagnetic shell made of organic moieties of the ligands. Additionally, these molecular materials have many important advantages over conventional nanoscale magnetic particles composed of metals, metal alloys or metal oxides. These include well-defined and reproducible crystal structures (in contrast with nanoparticles), light weight, uniform size, chemical flexibility, and the possibility to dissolve in common solvents and readily exchangeable peripheral ligands. The properties associated with these materials make them useful in future electronic and magnetic devices such as magnetic data storage devices, quantum computing and molecular spintronics.5,6 Accordingly, sincere efforts have been made by scientists for a long time to synthesize molecular magnetic cages with new emerging properties by incorporating different paramagnetic metal ions. Further, for a chemist, it provides excitement and intellectual challenges to synthesize new classes of nanoscopic compounds that have anticipated properties but that do not yet exist. The fundamental goal of this review is to shed light on the basic characteristics and synthetic strategies of SMMs and to briefly discuss some selected examples.
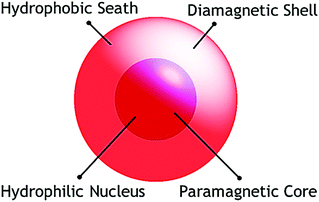 |
| Scheme 1 Schematic view of a zero dimensional ‘molecular nanomagnet’. | |
2. Characteristics of an SMM
SMMs are characterised by two important parameters:
(A) A typical double-well ground state potential, and
(B) Blocking of magnetization.
A. Typical double-well ground state potential
The unusual magnetic behaviour of SMMs is governed by a combination of large spin (S) and large, easy-axis-type anisotropic7a zero-field splitting (ZFS) parameters D and E, according to the Hamiltonian given in eqn (1), | Ĥ = |D|Sz2 + E(Sx2 − Sy2) | (1) |
where the axial (D) and rhombic (E) zero-field splitting parameters reflect the type of symmetry around the magnetic centre. For cubic symmetry both D and E are zero, while only E is zero in the case of axial symmetry. The sign of D is critical, since it determines the type of magnetic anisotropy associated with the S multiplet. The magnetization and relaxation processes in SMMs are traditionally described by a ‘double-well’ diagram (Fig. 1), where the ±MS states are plotted on different potential wells. At zero-field all MS ≠ 0 levels form degenerate pairs (Fig. 1A). However, when an external field is applied parallel to the magnetization axis the −MS levels are stabilized and the +MS levels are destabilized (Fig. 1B). If the magnetization of the system reaches its saturation value, then only the MS = −S level remains populated. Upon removal of the field the system returns to thermal equilibrium through a series of steps depicted in Fig. 1C and 1D.
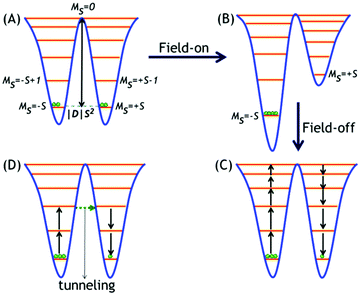 |
| Fig. 1 Schematic diagram representing the magnetization and magnetic relaxation processes in SMMs: (A) effect of zero-field splitting (ZFS) on a spin state S; the two wells are equally populated; (B) application of an external magnetic field (Zeeman effect) on the magnetization of a sample. The left well is selectively populated in a magnetic field; (C) representation of slow relaxation of the magnetization over the anisotropy barrier after removing the external magnetic field; (D) magnetic relaxation by ‘tunnelling’ through the anisotropy barrier between thermally activated states. | |
B. Blocking of magnetization
SMMs are characterized by a certain temperature called blocking temperature (Tb) below which the relaxation of the magnetization of the molecules is extremely slowed down and magnetic hysteresis of purely molecular origin is displayed. This is also manifested as peak maxima in magnetic susceptibility (χM) vs. temperature (T) plots, in a zero-field cooled (ZFC) curve.7 Further, the slow relaxation of magnetization is governed by an intrinsic energy barrier (Ueff) to spin inversion that significantly depends on the applied magnetic field (H) in the ZFC magnetization measurements as is evident from the following eqn (2):where HK = 2K/MS = anisotropy field, and MS and V are the spontaneous magnetization and the volume of the particle respectively. Therefore it is evident that as H increases, Ueff will decrease monotonically and, consequently, the Tb in the ZFC curve will also decrease.7b
Another important parameter is the relaxation time (τ) which can be defined as the time taken for the reversal of the magnetization. The τ increases exponentially on decreasing the temperature, according to the Arrhenius law given in eqn (3):
| τ = 1/2πν = τ0 exp(Ueff/kBT) | (3) |
where
τ0 = pre-exponential factor,
kB = Boltzmann constant and
T = temperature in Kelvin (K). If the moment of the sample is determined by a technique with a measuring time
tm, then the particle will be in the blocked state when
τ >
tm. Hence the blocking temperature is defined as
Tb =
Ueff/[
kB![[thin space (1/6-em)]](https://www.rsc.org/images/entities/char_2009.gif)
ln(
tm/
τ0)]. In a simple approximation, the larger the
Ueff, the longer will be the
τ value and smaller will be
τ0. The energy barrier to be overcome is given by
Ueff = |
D|
S2 for integer spins and by
Ueff = |
D|(
S2 − 1/4) for half-integer spins.
8
Several hundreds of SMM cages were isolated and characterized so far but the Tb for most of them is still very low. As the energy barrier is directly related to the blocking temperature, it is therefore the most critical determinant for the observation of SMM behaviour.9,10aUeff and Tb can be increased either by increasing D or by increasing S, although the large size and the presence of a large number of unpaired electrons in a molecule do not always result in higher Ueff or Tb. This is evident from the examples given in Fig. 2 which shows the number of Mn atoms vs. Ueff (in K) plot for a few selected Mn based SMMs.
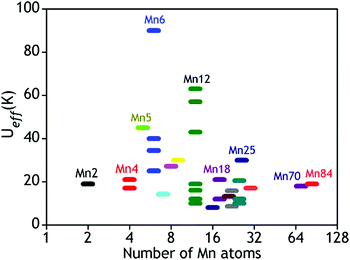 |
| Fig. 2 Number of Mn atoms in molecules vs. effective energy barrier (K) plot. | |
3. Source of magnetic anisotropy in an SMM
The most fundamental parameter of an SMM is ‘magnetic anisotropy’ which can be explained by the spin Hamiltonian given in eqn (4): |  | (4) |
The first term in eqn (4) is referred to as the isotropic exchange interaction, the second as the single-ion magnetic anisotropy, and the third as well as the fourth refer to the antisymmetric spin–spin contribution to the magnetic interaction or two-centre magnetic anisotropy. The contributions of each of the component are briefly discussed below.
A. Isotropic exchange interaction
This term tends to keep the spins either parallel or antiparallel to each other and forms the spin energy spectrum of an SMM, but does not contribute to the magnetic anisotropy. Isotropic exchange interactions (Fig. 3) are dominant for spin-only magnetic centres in comparison to anisotropic terms. Usually this exchange interaction is a short-range interaction and practically vanishes beyond the fifth nearest neighbours.
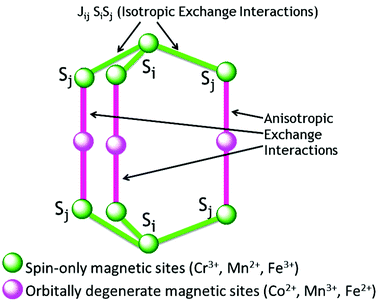 |
| Fig. 3 Schematic representation of the isotropic and anisotropic exchange interactions in molecular magnets. | |
B. Single-ion magnetic anisotropy
This term represents the orientation of the spins along a given direction in space. Single-ion anisotropy is also referred to simply as ‘magneto-crystalline anisotropy’, which is determined by the interaction between the orbital state of a magnetic ion and the surrounding strong crystal field. This interaction is transferred to the spin moments via the spin–orbit coupling, giving a weaker d-electron coupling of the spins to the crystal lattice. The single-ion anisotropy is a product of quenching of the orbital moment by the crystal field, which has the symmetry of the crystal lattice. When an external field is applied, the orbital moments may remain coupled to the lattice while the spins are freer to turn. The magnetic energy depends upon the orientation of the magnetization relative to the crystal axes. The single-ion magnetic anisotropy is also related to zero-field splitting (ZFS) on separate magnetic centres and contributions come from individual ions. For 3d ions the ZFS energy is generally limited by SiDiSi < 15 cm−1. For example, in CrIII: SiDiSi ∼ 0.1–1 cm−1, MnII, FeIII: SiDiSi ∼ 0.01–0.5 cm−1, MnIII: SiDiSi ∼ 10–15 cm−1, NiII: SiDiSi ∼ 1–10 cm−1. Therefore to obtain a good SMM having D|S|2 ∼ 1000 cm−1, the 3d transition metal cages should have at least 100 metal centres. For most of the ordinary medium-sized 3d ion based cages, the spin reversal barrier is limited to ∼100 cm−1 and is incapable of providing high energy barrier (Ueff) and blocking temperature Tb. Therefore magnetic centres having unquenched orbital momentum can be incorporated as a potential candidate for a good SMM.
In the last few years, considerable attention has therefore been given to the choice of atoms whose single-ion anisotropies are very high like orbitally degenerate transition metal ions (3d, 4d and 5d), lanthanides (Ln) and actinides (An). The transition metal ions include TiIII, VIII, CrIII, MnIII, FeIII, CoII, NiII, MoIII, ReIV and OsIII. Among the lanthanide ions, TbIII (4f8, 7F6, g = 3/2), DyIII (4f9, 6F15/2, g = 4/3), HoIII (4f10, 5I8, g = 5/4), and ErIII (4f11, 4I15/2, g = 6/5) are the most commonly used ones. Because of the strong angular dependence of the 4f orbitals, the electronic structures of TbIII and DyIII ions possess substantial anisotropy and can show SMM property even as single ions, for which they are also known as single ion magnets (SIM). A few actinide ions (UIII, UIV and UV) are also used in SMMs. A detail discussion about choice of the metal centres for SMM and their chemistry is given in the Design strategy for SMMs section.
C. Two-centre magnetic anisotropy
This term represents anisotropic spin coupling between magnetic centres and contributions from exchange-coupled pairs. The contribution from this component is comparable in magnitude with isotropic exchange interactions for the ions having unquenched orbital momentum. Under the influence of strongly anisotropic exchange interactions, magnetically isotropic transition metal ions give a large contribution to the global magnetic anisotropy of the SMM cluster. This contribution vanishes for the isotropic exchange, for which only the zero-field splitting on separate metal ions contributes to the global anisotropy.
4. Design strategy for SMMs
The construction of SMMs relies on many different parameters such as the type of ligands (primary ligands and co-ligands), the nature of metal ions, solvents, pH, reaction conditions etc.10b Among these, the roles of ligands and metal ions are the most important factors which modulate the magnetic properties of the SMMs. In the following section the type of ligands used, their bridging modes and the choice of metal ions for the fabrication of SMMs have been highlighted.
A. Choice of ligands
The geometry of a molecule, the ligand field effect and the type of magnetic exchange interactions between the metal centres govern the overall magnetic behaviour of the SMMs. The common ligands employed for the synthesis can be classified into three different categories: (a) short bridging ligands (OH−, O2−, S2−, F−, Cl−, CN−, pseudohalogens etc.) which can fine tune the exchange coupling between the metal ions; (b) ligands with bulky substituents which can obstruct 3D magnetic ordering between the molecules and make them isolated and (c) compartmental ligands with both O and N donor sites such as pyridonates, oximes, Schiff bases, hydrazones/hydrazides etc. (Table 1). The nature of magnetic interactions is very much dependent on the bridging ligands whereas some physical properties like solubility, hydrophobicity or hydrophilicity depend on terminal ligands. Herein, the most common ligands used for the synthesis of SMMs, their important structural features and the magnetic behaviors arising therefrom have been discussed.
Table 1 Compilation of the SMMs based on different ligands discussed in this review
Type of ligands |
Formulae of complexes |
U
eff (K) |
τ
0 (s) |
Ref. |
(I) Short bridging ligands
|
(a) Monoatomic bridging ligands |
[Dy5(μ4-OH)(μ3-OH)4(μ–η2-Ph2acac)4(η2-Ph2acac)6] (1) |
33 |
4.5 × 10−9 |
12
|
[Cp2Dy(μ-Cl)]2 (2) |
37.9 |
1.4 × 10−6 |
13
|
[{Cp′2Dy(μ-SSiPh3)}2] (3) |
192 |
2.38 × 10−7 |
14
|
(b) Diatomic bridging ligands |
{[{Fe(L-N3O2)(H2O)}2Cr(CN)6][ClO4]}·3H2O (4) |
44.3 |
1.4 × 10−9 |
16
|
{[(Me3Si)2N]2(THF)Tb}2(μ–η2:η2-N2)− (5) |
324.6 |
8.2 × 10−9 |
17
|
(c) Triatomic bridging ligands |
[Cu2(valpn)2Tb2(N3)6]·2CH3OH] (6) |
30.1 |
1.1 × 10−6 |
20
|
[Co7(bzp)6(N3)9(CH3O)3]·2ClO4·2H2O (7) |
— |
— |
21
|
[NEt4]3[Mn5(salox)3O(OCN)6Cl2] (8) |
33.9 |
— |
22
|
[Mn14O4(OH)2(OCN)6(O2CMe)2(dpkd)8(DMF)2(H2O)4](OH)2] (9) |
35 |
7.4 × 10−12 |
23
|
[Dy3(μ3-N3)(μ3-OH)(H2L)3(SCN)3](SCN)·3CH3OH·H2O (10) |
3 |
1 × 10−6 |
24
|
[Mn12O12(CN-o-C6H4CO2)12(CH3CO2)4(H2O)4]·8CH2Cl2 (11) |
58.7 (HT) |
1.3 × 10−8 |
26
|
34.8 (LT) |
2 × 10−9 |
[Dy4(μ3-OH)4(Acc)6(H2O)7(ClO4)]·(ClO4)7·11H2O (12) |
— |
— |
27
|
(II) Ligands with bulky substituents
|
(a) Polyalcohol and aminoalcohols |
[Dy24M2(OH)8(CH3COO)12(C6H10O6)6(C6H9O6)6(H2O)51][Dy(H2O)9](ClO4)29(H2O)80(C2H5OH)4 (M = Ni#, Mn§) (13) |
1.24# |
5.6 × 10−5# |
29
|
0.98§ |
6.6 × 10−5§ |
[NEt4][Fe11O4(O2CPh)10(thme)4(dmhp)2Cl4] (14) |
— |
— |
30
|
[Mn4Tb2O2(O2CtBu)6(edteH2)2(NO3)2] (15) |
20.3 |
1.4 × 10−11 |
31
|
[Mn2Dy2(LH)4(μ-OAc)2](NO3)2·2CH3OH·3H2O (16) |
24.2 |
— |
32
|
[Mn2Tb2(LH)4(μ-OAc)2](NO3)2·2H2O·2CH3OH·Et2O (17) |
48.6 |
— |
32
|
[CoII4CoIII3(Htea)3(tea)3](NO3)2·2CH3OH·1.5H2O (18) |
— |
— |
33
|
[CrIII2DyIII2(OMe)2-(mdea)2(hfacac)6] (19) |
28.6 |
1.6 × 10−7 |
34
|
(b) Phosphonates |
[Et3NH][Co8(chp)10(O3PPh)2(NO3)3(Hchp)2] (20) |
84 |
1.8 × 10−12 |
36
|
[Mn9Na(μ3-O)4(μ4-O)2(O3PPh)2(O2CCMe3)12(H2O)2(H2O)0.67(py)0.33] (21) |
17.7 |
— |
37
|
[H3O][Cu24Dy8(Ph3C–PO3)6(Ph3C–PO3H)6(MeCO2)12(MeCO2H)6(OH)42(NO3)(OH2)6] (22) |
4.6 |
2.1 × 10−8 |
38
|
[MnIIMnIII12(μ4-O)6(μ-OH)6(O3P-t-Bu)10(OH2)2(DMF)4]·2MeOH·4DMF (23) |
— |
— |
39
|
(III) N, O donor ligands
|
(a) Pyridonates |
[Ni12(chp)12(O2CMe)12(thf)6(H2O)6] (24) |
9–10 |
— |
40
|
[Co16(OH)6(chp)22(O3PC6H9)2(H2O)4] (25) |
— |
— |
41
|
(b) Oximes |
[Ni8Dy8O(OH)4(pao)28](ClO4)5(NO3) (26) |
— |
— |
43
|
[Dy3vanox2(Hvanox)4(EtOH)2](ClO4)·1.5EtOH·H2O (27) |
28.7 |
6.3 × 10−5 |
44
|
69.3 |
5.9 × 10−8 |
[MnIII6O2(Et-sao)6(O2CPh(Me)2)2(EtOH)6] (28) |
86.4 |
2 × 10−10 |
46
|
[MnII/III15O0.5(OH)11.5(pao)18(EtOH)(H2O)](ClO4)6.5·5MeCN·6H2O (29) |
10.3 |
— |
47
|
(c) Schiff bases |
[Mn(saltmen)(N3)]2 (30) |
17 |
— |
49
|
29 |
— |
[Mn(salen)(H2O)]2Na[XMo6(OH)6O18]·20H2O (X = Al and Cr) (31) |
13.2 |
7.2 × 10−7 |
50
|
9.1 |
16 × 10−7 |
[Dy2(Pc)2(L)H2O] (32) |
28.5 |
9.5 × 10−7 |
51
|
[Er(HL)2(NO3)3] (33) |
51.4 |
— |
52
|
(d) Hydrazones |
[Dy4(L)4(MeOH)6]·2MeOH (34) |
19.6 (FR) |
7.8 × 10−6 |
54
|
171.6 (SR) |
1.2 × 10−7 |
[Dy4(L1-2H)2(L1-H)2(N3)4(O)]·14H2O (35) |
51 (LT) |
3.0 × 10−9 |
55
|
91 (HT) |
4.5 × 10−7 |
[Dy3L12(H2O)9](Cl)5·6H2O (36) |
11.2 |
3.1 × 10−6 |
56
|
[Dy6L22(HCO2)4(μ3-OH)4(DMF)6(H2O)2](Cl)2·4H2O (37) |
9.7 |
6.4 × 10−6 |
56
|
A(I) Short bridging ligands.
(a) Monoatomic bridging ligands.
The discussion starts with the smallest possible monoatomic bridging ligands like OH−, O2−, S2−, F−, Cl−, etc.11 In general, magnetic interactions are very strong through these ligands; moreover, they can efficiently bridge more than two metal centres because of the absence of steric hindrance. So, using such ligands, large numbers of magnetic cages have been reported and some of them are very interesting in terms of structure and magnetic properties. In 2008, a pentanuclear dysprosium hydroxy complex [Dy5(μ4-OH)(μ3-OH)4(μ–η2-Ph2acac)4(η2-Ph2acac)6] (1, Fig. 4) (Ph2acac = dibenzoylmethanide) was reported by Powell, Roesky and co-authors,12 in which DyIII ions adopted square-based pyramidal arrangement. Each triangular face of the square pyramid was capped by one μ3-O moiety and in the square-based face, four DyIII ions atoms were linked by one μ4-O atom (from OH). No clear saturation was observed in the M vs. H data which confirmed the presence of low-lying excited states and/or anisotropy. AC susceptibility measurements at 0 DC field revealed SMM behaviour of the complex with the Ueff value of 33 K and τ0 of about 4.5 × 10−9 s. In order to suppress the possible fast zero-field quantum tunnelling of magnetization (QTM), AC data were also recorded under a small DC field which showed a slight shifting of the relaxation mode to a higher frequency, suggesting the absence of any quantum relaxation above 1.8 K. Another interesting chloro-bridged centrosymmetric dimeric complex is [Cp2Dy(μ-Cl)]2 (2, Fig. 5), reported by Winpenny et al.13 The dimer complex contained eight-coordinate DyIII ions coordinated by the strong crystal field ligand cyclopentadiene (Cp = η5-C5H5). From the χ′′Mvs. T data two maxima centred at 8.5 and 26 K (at 1200 Hz) were observed, both of which shifted to lower temperatures on reducing the frequency of the AC field.
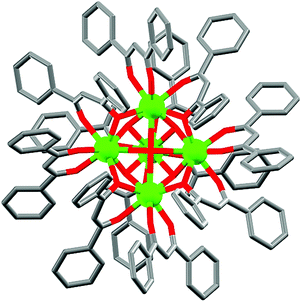 |
| Fig. 4 Molecular structure of 1. Color code: green – Dy, grey – C, red – O. | |
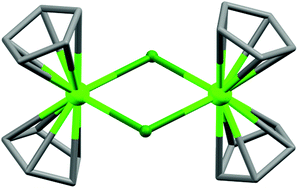 |
| Fig. 5 Molecular structure of 2. Color code: green – Dy; grey – C, dark green – Cl. | |
The shift was found to be more prominent for the high-temperature peak. Fitting the data according to the Arrhenius equation provided the Ueff value of 37.9 K and τ0 = 1.4 × 10−6 s confirming the SMM behaviour of the compound. A very similar thiolate (S2−) bridged dinuclear DyIII complex showing prominent SMM behaviour was also reported by Collison, Layfield and co-workers.14 The complex formulated as [{Cp′2Dy(μ-SSiPh3)}2] (Cp′ = η5-C5H4Me) (3) was the first sulphur bridged SMM and showed the highest magnetization reversal barrier for any dinuclear DyIII based SMM. Each DyIII ions were coordinated to two Cp′ ligands and to two sulfur atoms from two μ-SSiPh3 ligands exhibiting overall eight coordination geometry and the DyIII ions resided in pseudo-tetrahedral geometries with respect to Cp ligand centroids and the sulphur atoms. From DC magnetic data, unsaturated behaviour of the M vs. H plot and non-superimposition of the M vs. H/T plots were observed which indicate significant magnetic anisotropy and/or low-lying excited states in the complex. AC magnetic measurements showed temperature and frequency dependencies of χ′M and χ′′M components at 0 DC field with a single peak below 40 K which revealed the slow relaxation of magnetization features in the complex. Fitting the data above 20 K according to the Arrhenius equation gave Ueff = 192 K (τ0 = 2.38 × 10−7 s), which was much higher compared to that of a similar chloro-bridged complex 2, mentioned before. The experimentally observed Ueff value for 3 also matched well with that obtained from ab initio calculations. That was due to the fact that QTM was not approached even at 2 K and, accordingly, only the Orbach process involving the first excited state was measured in the AC susceptibility studies.
(b) Two-atom bridging ligands.
Two-atom bridges like cyano (CN), unsubstituted hydrazine (N2H4) and N23− radicals are also good candidates to mediate strong intermetallic magnetic interaction and form nanoscopic magnetic molecules. Owing to the rich magnetic behaviours of CN-bridged molecules, extensive studies on these systems have been done for a long time and are still being pursued. Reviews on CN-bridged complexes by Ohba et al., Gao et al. and Mallah et al.15 gave a vivid idea of the topologies and magnetic properties of such systems. An interesting example to mention here is the FeII based CN-bridged complex {[{Fe(L-N3O2)(H2O)}2Cr(CN)6][ClO4]}·3H2O (4, Fig. 6) (L-N3O2 = azaoxa-macrocycle ligand) reported by Gao, Sato and co-authors.16 In this complex each [Cr(CN)6]3− unit connected to two {Fe(L-N3O2)(H2O)}2+ moieties by two trans-cyano groups and the FeII ion displayed near-C5v distorted pentagonal-bipyramidal geometry. Variable temperature DC magnetic measurements showed significant ferromagnetic coupling between CrIII and FeII ions owing to a predominant orthogonality between the two sets of orbitals on the CrIII and FeII centres. Both the χ′M and χ′′M components of the AC susceptibility data above 1.8 K showed frequency dependence revealing SMM behaviour of the complex. Fitting to the Arrhenius law gave Ueff = 44.3 K and τ0 = 1.4 × 10−9 s, which was close to the record value in the family of cyanide bridged SMMs. Another notable example is the N23− radical-bridged dinuclear TbIII complex formulated as{[(Me3Si)2N]2(THF)Tb}2(μ–η2:η2–N2)− (5).17 The N23− radical ligands possess diffuse spin orbitals which can penetrate the core electron density of the TbIII ions and thereby result in strong exchange coupling. This shifts the degenerate MJ sublevels to different energies, which significantly reduces the probability of QTM and consequently increases the relaxation time. Thus, the combination of the strong anisotropy of TbIII ions with the strong exchange-coupling ability of the N23− radical results in an SMM with a record Tb of 14 K. AC susceptibility data were fitted according to the Arrhenius temperature law, wherefrom Ueff = 324.6 K and τ0 = 8.2 × 10−9 s were obtained.
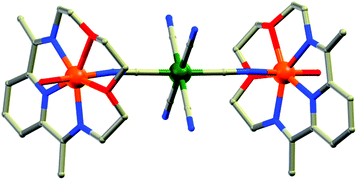 |
| Fig. 6 Molecular structure of 4. Color code: orange – Fe; dark green – Cr, grey – C, red – O, blue – N. | |
(c) Three-atom bridging ligands.
Three-atom bridges like azides (N3−), cyanates (OCN−), thiocyanates (SCN−), selenocyanates (SeCN−), carboxylates (RCOO−) etc. are also well known for their adaptable bridging modes (Scheme 2) and their ability to mediate very strong magnetic interactions between the metal centres. Among them, azide is one of the most versatile ligand and a large number of recent reports and reviews are dedicated to azido bridged molecular magnets.18 Considering the M–N3 distances to be similar, the M–(N3)n–M angles determine the type (ferro- or antiferro-) and magnitude of the exchange coupling. Moreover, some one, two and three dimensional complexes exhibit cooperative effects and show long-range magnetic ordering. Generally antiferromagnetic exchange interaction is transmitted between the paramagnetic metal centres for end-to-end (EE) azido bridging mode but ferromagnetic exchange is expected when the M–N3–M torsion angle is ∼90°.19 On the other hand, the behaviour of the end-on (EO) azido mode, although generally ferromagnetic, sometimes become unpredictable.
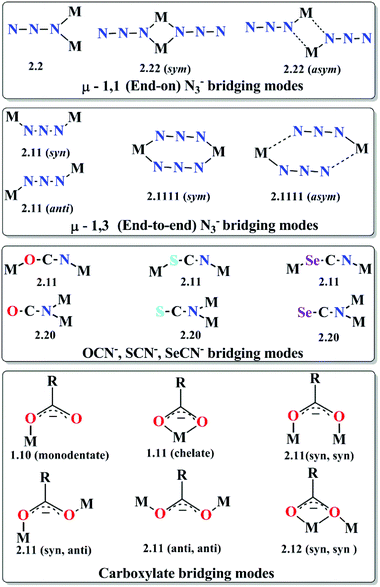 |
| Scheme 2 Common bridging modes of azide (N3−), cyanates (OCN−), thiocyanates (SCN−) and selenocyanates (SeCN−) and carboxylate ligands. | |
As mentioned, several azide bridged magnetic molecules are accounted in the literature, but only a few behave as SMMs. Recently a 3d–4f complex, [Cu2(valpn)2Tb2(N3)6]·2CH3OH (6, Fig. 7)20 (H2valpn = 1,3-propanediylbis(2-iminomethylene-6-methoxyphenol)), reported by Wang et al., shows SMM behavior. In the complex, two [CuTb] units are bridged by the double EO N3− between two Tb atoms to form a [CuTb]2 cluster. The χMT vs. T curve showed depopulation of the TbIII Stark levels and ferromagnetic TbIII–TbIII and CuII–TbIII interactions. The complex shows hysteresis loops below 2.4 K where the coercive fields increase with decreasing temperature and increasing field sweep rate, as expected for an SMM. From the AC magnetization data Ueff ≈ 30.1 K and τ0 ≈ 1.1 × 10−6 s were obtained. Gao et al. have reported a novel disc-like heptanuclear CoII-azide based cluster [Co7(bzp)6(N3)9(CH3O)3]·2ClO4·2H2O (7) (bzp = 2-benzoyl pyridine),21 where each of the four independent CoII ions assumes a distorted octahedral geometry. AC magnetic susceptibility measurements showed frequency-dependent χ′′M signals below 6 K in the 0 DC field. Both χ′M and χ′′M signals shifted to a higher temperature range on increasing the external DC field. Hysteresis loops obtained from single-crystal measurements carried out on a micro-SQUID setup showed the presence of two easy axis directions and a clear sign of a negative D value, consistent with the single molecule magnet behavior. In comparison to azide, OCN−, SCN− and SeCN− are less documented as bridging ligands and are not very efficient as magnetic couplers because of their two different donor sites (O/S/Se and N). Among the reported complexes with these bridging ligands, a very important OCN− bridged structurally characterized complex22 is [NEt4]3[Mn5(salox)3O(OCN)6Cl2] (saloxH2 = salicylaldoxime) (8) reported by Hendrickson et al. The MnIII–N–O–MnIII torsion angle (θ) was found to be ∼31°, which resulted in larger ferromagnetic exchange interactions between MnIII ions. AC magnetic susceptibility measurements showed the slow magnetization relaxation behaviour with Ueff = 33.9 K. Another interesting OCN− bridged complex, [Mn14O4(OH)2(OCN)6(O2CMe)2(dpkd)8(DMF)2(H2O)4](OH)2] (9), was synthesized using the di-2-pyridylketone (dpkd) ligand.23 This complex contained a MnII4MnIII4 rod like subunit attached on either side to two symmetry-related [MnIIMnIII2(μ-OR)3]5+ trinuclear subunits where Mn atoms are connected by a combination of two μ4-O2−, two μ3-O2−, two μ3-OH−, two η1:η1 OCN− and eight dpkd2− ligands. AC magnetic susceptibility data showed frequency dependency of the χ′′M signals and fit of these data according to the Arrhenius equation gave Ueff = 35 K and τ0 = 7.4 × 10−12 s. SCN− and SeCN− are also used as bridging ligands in many 3d as well as 4f based coordination complexes. Tang et al. have reported a novel trinuclear triangular DyIII circular helicate complex, [Dy3(μ3-N3)(μ3-OH)(H2L)3(SCN)3](SCN)·3CH3OH·H2O (10) (H3L = 2,6-diformyl-4-methylphenol di(benzoylhydrazone)),24 using SCN− anions, in which the coordination environment around DyIII ions was found to be distorted tricapped trigonal-prismatic and the triangle of Dy centers was capped by one μ3-OH and one μ3-N3− moieties. Fit of the experimental relaxation data to the Debye equation provides Ueff = 3 K and τ0 = 1 × 10−6 s. Similar to pseudohalogens, carboxylates are another class which can act as bridging as well as chelating ligands (Scheme 2). Interestingly, in the case of discrete molecules, the sizes of the R group attached with carboxylates or better to say steric hindrances generated by them play a significant role to restrict the growth of the cages. Depending on the nature of the R groups some physical properties of the cages like solubility, hydrophobicity/hydrophilicity and the magnetic properties can also be tuned. Numerous carboxylate bridged molecules are reported by various groups which are magnetically potent.25 An important example is the Mn12 oxocarboxylate complex [Mn12O12(CN-o-C6H4CO2)12(CH3CO2)4(H2O)4]·8CH2Cl2 (11) reported by Kushch et al.26 An interesting feature of the electronic structure of this complex is the higher overlap of the Mn 3d bands with the 2p bands of the O atoms as compared to the parent Mn12-acetate cage and that resulted in significant difference in the exchange interactions in comparison to the Mn12-acetate cage. ZFC and FC curves showed bifurcation below 4.5 K which gave evidence of slow relaxation of magnetization. Fitting of the relaxation rate (1/τ) vs. T curve by the Arrhenius equation gave Ueff = 58.7 K and τ0 = 1.3 × 10−8 s for the high temperature solvent free phase and Ueff = 34.8 K and τ0 = 2 × 10−9 s for the low temperature range. Another carboxylate-bridged polynuclear 4f complex, represented as [Dy4(μ3-OH)4(Acc)6(H2O)7(ClO4)]·(ClO4)7·11H2O (12) [Acc = 1-amino cyclohexanel-carboxylic acid], was reported by Long and Kong et al.27 The cationic cluster consists of a distorted cubane shaped core of [Dy4(μ3-OH)4]8+ units in which the four Dy centres form a nearly perfect tetrahedron. All the DyIII ions are eight coordinated with a bicapped trigonal prism geometry. Variable temperature DC magnetic measurements show a lack of saturation of the M vs. H curves and non-superimposition of M vs. H/T curves, which indicated significant anisotropy and/or low lying excited states. It is suggested that the Dy–O–Dy angles in the cubane cores influence the magnetic exchange coupling because the local tensor of anisotropy on each DyIII centre and their relative orientations may affect the molecular anisotropy and hence lead to slow relaxation of magnetization. Therefore, AC susceptibility measurements were performed which displayed frequency dependent χ′′M signal, confirming SMM behaviour of the complex.
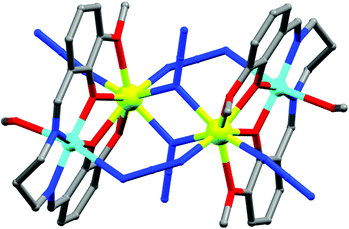 |
| Fig. 7 Molecular structure of 6. Color code: lime green – Tb, light blue – Cu, grey – C, red – O, blue – N. | |
A(II) Ligands with bulky substituents.
(a) Polyalcohols and aminoalcohol derivatives.
These are also an important class of ligands which drew substantial attention because of their dual behaviour i.e. bridging as well as chelating (Scheme 3). They have been successfully employed by many groups for the synthesis of magnetically potent transition metal as well as lanthanide based magnetic cages.28 Recently, Long et al. reported two isomorphous heterometallic Dy24M2 (M = Ni, Mn) cage-like cluster compounds, formulated as [Dy24M2(OH)8(CH3COO)12(C6H10O6)6(C6H9O6)6(H2O)51][Dy(H2O)9](ClO4)29·(H2O)80·(C2H5OH)4 (13),29 which were obtained through the self-assembly of metal ions and myo-inositol ligand. Structural investigation revealed that the compounds are made up of two [Dy9M(OH)4(CH3COO)6(C6H10O6)3(C6H9O6)3(H2O)15]4+ units, three [Dy2(H2O)7]6+ units and three non-coordinating ClO4− anions acting as templates. Each of the MII ions is hexa-coordinated whereas the DyIII ions are in hepta-coordinate, octa-coordinate and nona-coordinated environments. The maximum values of M(NμB) are smaller than the expected values for 25 uncoupled DyIII ions and 2 NiII/MnII ions, suggesting the presence of significant anisotropy and/or low-lying excited states. AC magnetic susceptibility data show the prominent frequency dependency of the χ′′Mvs. T curves revealing SMM behaviour of the compounds. From the generalised Debye model fitting of the relaxation data, Ueff = 1.24 K (τ0 = 5.6 × 10−5 s) for Dy24Ni2 and Ueff = 0.98 K (τ0 = 6.6 × 10−5 s) for Dy24Mn2 were obtained. A very interesting FeIII based cluster complex, [NEt4][Fe11O4(O2CPh)10(thme)4(dmhp)2Cl4] (14) (dmhp = 4,6-dimethyl-2-hydroxypyrimidine) formed by polyalcoholic ligand 1,1,1-tris(hydroxymethyl)ethane (H3thme), was reported by Mcinnes, Brechin and co-workers.30 The fascinating structure contains two identical [Fe4O6]6+ the rhombus one at the two ends of the molecule and a central linear [Fe3O4]1+ unit (Fig. 8). All the FeIII centres are hexa-coordinated with distorted octahedral geometries. Fitting of the magnetization data obtained from solid state DC magnetization measurements gave S = 11/2, g = 2.03 and D = −0.46 cm−1. Single crystal magnetic measurements performed using a micro-SQUID in the low-temperature range (1.2–0.04 K) showed hysteresis loops in M vs. H plots whose coercivities increase with decreasing temperature. Further detailed study of the field sweep rate dependence of the hysteresis loops showed that the hysteresis at non-zero fields is due to slow relaxation of magnetization because of the anisotropy barrier, confirming the SMM behaviour of the complex.
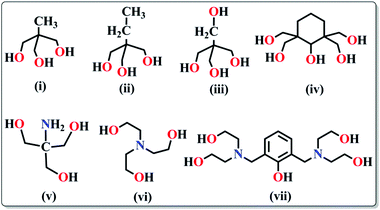 |
| Scheme 3 Commonly used polyalcohols (i–iv) and aminoalcohol (v–vii) ligands. | |
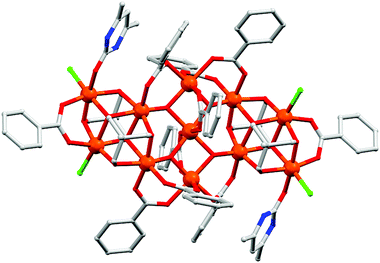 |
| Fig. 8 Molecular structure of 14. Color code: orange – Fe, red – O, grey – C, blue – N. | |
Another important family of Mn4Ln2 (Ln = Gd, Tb, Dy, Ho) complexes constructed from polyalcohol ligands has been reported by Christou et al.31 which exhibited face-fused, {M6O6} double-cubane topology, a very rare structural type, and was the first report of such a discrete unit in 3d–4f chemistry. The Tb analogue formulated as [Mn4Tb2O2(O2CtBu)6(edteH2)2(NO3)2] (edteH4 = N,N,N′,N′-tetrakis(2-hydroxyethyl)ethylenediamine) (15) showed SMM behaviour as confirmed from the observation of hysteresis below 0.9 K in M vs. H scans on a single crystal of the complex. It also exhibited a frequency dependent decrease in χ′MT below ∼3 K and a simultaneous frequency dependent χ′′M. The fit to the thermally activated region according to the Arrhenius relation gave Ueff = 20.3 K and τ0 = 1.4 × 10−11 s. Below ∼0.3 K, the relaxation becomes independent of temperature, which indicates the occurrence of QTM through the anisotropy barrier for the relaxation. Recently Chandrasekhar's group reported a series of tetranuclear {MnIII2LnIII2} (Ln = Dy, Gd, Tb, Ho) clusters using the polyalcoholic type ligand 2,2′-(2-hydroxy-3-methoxy-5-methyl benzylazanediyl)diethanol (LH3).32 All the compounds are dicationic in nature with an arch-type topology and the MnIII ions being present in the periphery and the LnIII ions in the centre. Magnetochemical analysis revealed the SMM behaviour of the Dy and Tb complexes {[Mn2Dy2(LH)4(μ-OAc)2](NO3)2·2CH3OH·3H2O (16) and {[Mn2Tb2(LH)4(μ-OAc)2](NO3)2·2H2O·2CH3OH·Et2O (17)}, with Ueff = 24.2 K and 48.6 K respectively. Besides the polyalcoholic ligands, amino-alcohol ligand based complexes are also exhaustively studied and are well known for their interesting magnetic properties. A Co7 disc like cage [CoII4CoIII3(Htea)3(tea)3](NO3)2·2CH3OH·1.5H2O (18) (H3tea = triethanolamine) was reported by Tangoulis et al.33 The heptanuclear complex contained six outer Co atoms which were arranged at the corners of a hexagon with Co⋯Co distances of ∼3 Å and one central Co atom was located approximately in the middle of the hexagon with Co⋯Co distances also of ∼3 Å. Ferromagnetic interactions between the central CoII ion and the peripheral ones are observed in agreement with the Co–O–Co bond angles in the range of 91.3–95.7°. AC magnetic susceptibility measurements revealed a decrease in the χ′MT curves which became more pronounced at higher frequencies and started to diverge below 4 K. A prominent frequency dependent χ′′M signal was also observed which confirmed the SMM behaviour of the complex. Recently, Murray et al. reported a series of heterometallic 3d–4f clusters, using aminoalcohol ligands like N-n-butyldiethanolamine (bdeaH2) and N-methyldiethanolamine (mdeaH2).34 A representative {CrIII2DyIII2} complex, formulated as [CrIII2DyIII2(OMe)2-(mdea)2(hfacac)6] (19) (hfacacH = hexafluoroacetylacetone), possessed a ‘butterfly’ or ‘diamond’ shaped metallic core, with two DyIII ions occupying the “body” positions and two CrIII ions residing in the outer “wing-tip” sites. One chelating [hfacac]− ligand completes the coordination environment of the CrIII ions resulting in six-coordinate CrIII ions with octahedral geometries while two [hfacac]− ligands complete the coordination environment of the DyIII ions ensuring an eight-coordinate environment with distorted square antiprismatic geometries. Variable temperature and variable frequency AC measurements showed that both χ′M and χ′′M susceptibilities display frequency and temperature dependency, which indicates blocking of magnetization due to an anisotropy barrier. Data also revealed that the relaxation follows a thermally activated mechanism above 1.8 K with the plots of ln(τ) versus 1/T being linear. Fitting of these relaxation data according to the Arrhenius equation yield Ueff = 28.6 K and τ0 = 1.6 × 10−7 s.
(b) Phosphonates.
Among the ligands with bulky substituents phosphonates are widely used for the synthesis of high nuclearity 3d and 3d–4f nanoscopic cages with diverse magnetic properties.35 Despite synthetic difficulties, the presence of three O donors and their various bridging modes enable them to coordinate up to nine metal centres (Scheme 4). In order to overcome the solubility problem of the metal phosphonate complexes, three different approaches have been followed by Clearfield, Winpenny, Chandrasekhar and others. The first approach is to use some pre-synthesized small cages as a starting material, the second approach is to incorporate some large substituents on the phosphonates to increase the organic part and the third approach is to use some co-ligands like carboxylates, pyrazolates etc. Winpenny et al. reported an excellent octanuclear CoII based phosphonate cage complex, [Et3NH][Co8(chp)10(O3PPh)2(NO3)3(Hchp)2]36 (20, Fig. 9) [Hchp = 6-chloro-2-hydroxypyridine], which exhibited an irregular structure with two P atoms and four Co atoms lying on the vertices of a central trigonal prism and the remaining four Co atoms lying above the triangular faces of the prism. Two of the Co sites within the trigonal prism are five-coordinated while the other six Co centres are six-coordinated. AC susceptibility measurement reveals that a frequency dependent maximum is observed in both χ′M and χ′′M at 4 K and 6 K; however the latter is about one-tenth the height of the equivalent maximum in χ′M, which suggests that only a fraction of the magnetization relaxes slowly. The relaxation time was determined from the χ′′Mvs. T plot using the Arrhenius equation which gave Ueff = 84 K and τ0 = 1.8 × 10−12 s. Micro-SQUID analysis shows a hysteresis loop at 4 K and lower temperatures, but below 0.5 K the hysteresis narrows and changes towards a “butterfly” shape. This feature indicates the presence of two relaxation mechanisms in the complex. Another interesting homovalent MnIII based phosphonate cage complex, [Mn9Na(μ3-O)4(μ4-O)2(O3PPh)2(O2CCMe3)12(H2O)2(H2O)0.67(py)0.33] (21) [PhPO3H2 = phenylphosphonic acid], was reported by Winpenny and co-authors in 2006.37 The complex is described as fragment of an icosahedron in which eight of the Mn atoms, two P atoms and a Na atom lie at the vertices of a distorted icosahedron and all the MnIII ions show Jahn–Teller elongation. From AC susceptibility measurements a frequency-dependent decrease in the χ′Mvs. T signal and a frequency-dependent out-of-phase signal at T < 2.5 K is noticed which confirm the SMM behaviour of the complex. Fitting of the data using the Arrhenius equation provides Ueff = 17.7 K and therefrom a D value of 0.27 K was obtained. The first heterometallic 3d–4f based phosphonate cluster, [H3O][Cu24Dy8(Ph3C–PO3)6(Ph3C–PO3H)6(MeCO2)12(MeCO2H)6(OH)42(NO3)(OH2)6] (22), was reported in 2010.38 The molecular structure consists of (i) a metal core, composed of eight DyIII centres at the vertices of a cube, twelve inner CuII sites arranged in the form of a cuboctahedron and twelve CuII centres outside the cube and (ii) an organic sheath which includes twelve phosphonates and eighteen acetate groups. Measurements show a frequency dependency of both the χ′M and χ′′M signals. The SMM behaviour was also confirmed by micro-SQUID measurements that show increasing coercivity with decreasing the temperature of magnetization hysteresis loops. From the relaxation time data Ueff = 4.6 K and τ0 = 2.1 × 10−8 s were obtained. Very recently, Chandrasekhar and co-workers reported a mixed-valent Mn based phosphonate cage, [MnIIMnIII12(μ4-O)6(μ-OH)6(O3P-t-Bu)10(OH2)2(DMF)4]·2MeOH·4DMF (23)39 that is composed of a [MnIIMnIII12(μ4-O)6] disc-like core. The core is made of a central MnII ion which is surrounded by 12 other MnIII ions. The coordination geometry of all the Mn centres is distorted octahedral. AC magnetic studies revealed frequency-dependent responses of χ′M and χ′′M data below 3.5 K which is the signature of SMM behaviour. The χ′′Mvs. χ′M (Cole–Cole) plots are not perfectly semi-circular manifesting a highly complicated relaxation process which can be attributed to spin–spin cross relaxation, dipolar interactions or low-lying excited states.
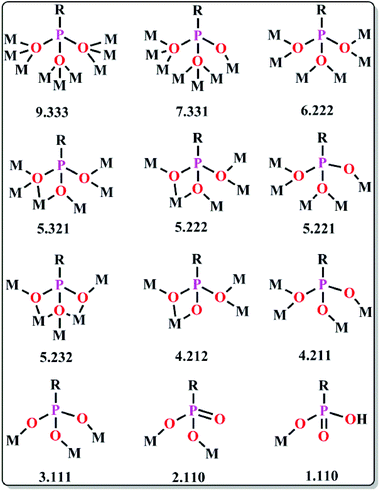 |
| Scheme 4 Common bridging modes of phosphonate ligands. | |
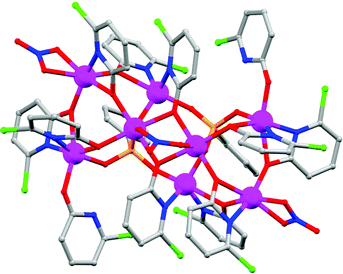 |
| Fig. 9 Core structure of 20. Color code: pink – Co, grey – C, red – O, blue – N, saffron – P, green – Cl. | |
A(III) N, O donor ligands.
Among N, O donor ligands, pyridonates, oximes, Schiff bases, and hydrazones/hydrazides are well explored for the synthesis of SMMs.
(a) Pyridonates.
In last few years, Winpenny and some other groups extensively used pyridonates for the synthesis of nanomagnetic cages. Apparently it is a chelating ligand but can bridge more than one metal via the same N or O atoms and result in 2.10, 2.11, 2.21, 3.21 etc. bridging modes (Scheme 5(i–iv)). An important example is the cyclic complex [Ni12(chp)12(O2CMe)12(thf)6(H2O)6] (24, Fig. 10) reported in 2002.40 The complex contained two independent NiII centres with distorted octahedral geometries. The adjacent NiII centres were bridged by either oxygen atoms derived from chp ligands and by carboxylate oxygen atoms or by oxygen atoms of chp ligand, water and acetate ions. An isothermal magnetisation study at 150 mK showed an S = 12 ground state and an AC susceptibility study showed a frequency dependent maximum in the χ′′M component, indicative of SMM behaviour. Analysis of the data by the Arrhenius equation gave Ueff values in between 9 and 10 K but the exact value could not be determined, as at very low temperature tunnelling became prominent. Furthermore, magnetic measurements on single crystals of the complex oriented at a field parallel to the crystallographic c-axis exhibited hysteresis loops in plots of M vs. H and at lower temperatures below 250 mK, the hysteresis loops were resolved into steps which confirmed QTM in the complex.
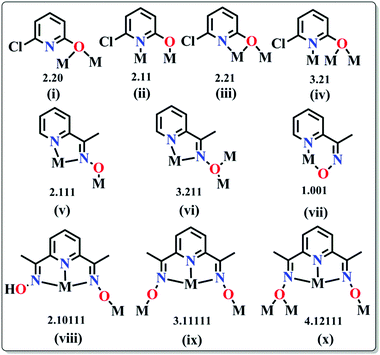 |
| Scheme 5 Common bridging modes of pyridonate (i–iv) and oxime (v–x) ligands. | |
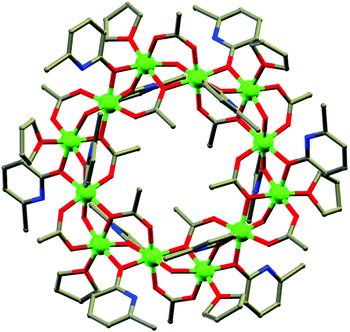 |
| Fig. 10 Molecular structure of 24. Color code: light green – Ni, grey – C, red – O, blue – N. | |
Pyridonate ligands have also been employed as co-ligands along with phosphonate ligands and a wheel-like hexadecanuclear cobalt phosphonate compound, [Co16(OH)6(chp)22(O3PC6H9)2(H2O)4] (25),41 was synthesized. The compound was comprised of a centrosymmetric core which was composed of two equivalent μ2-O bridged CoII8 segments. Ten CoII centres formed a distorted wheel while the remaining six atoms lie above and below the mean plane. CoII–O–CoII angles varied in the range of 94.8–129.6(3)° and are responsible for the overall antiferromagnetic behaviour in the compound. Magnetic susceptibility measurements show a steady decline of χMT value at higher temperatures and that was due to spin–orbit coupling of CoII. Temperature dependent AC magnetic susceptibilities at 0 DC and 5 G AC fields in the frequency domain of 1–1500 Hz show frequency dependent χ′′M signals at low temperature. This behavior was attributed to superparamagnetic relaxation of the magnetization.
(b) Oximes.
Various oxime based ligands (Scheme 5(v–x)) have also been widely employed to date in the synthesis of structurally and magnetically interesting 3d, mixed 3d and 3d–4f based SMMs.42 These ligands are particularly useful for 3d–4f magnetic cages because the deprotonated O atoms usually favour binding to oxophilic LnIII ions whereas the softer N atoms favour the 3d MII ions. An important oxime based heterometallic high-nuclearity cage, [Ni8Dy8O(OH)4(pao)28]·(ClO4)5·(NO3) (26) (pao = 2-pyridinealdoxime), was reported by Christou et al.43 The asymmetric unit consisted of two independent [Ni8Dy8O(OH)4(pao)28]6+ cations in each of which there was an inner Dy8 core and an outer Ni8 shell linked through 16 diatomic oximate bridges. The reported Dy⋯Dy distances were in the range of 3.547–8.805 Å and Dy–O–Dy angles in between 103.8–114.8°. The outer Ni8 shell had a nonplanar square-based topology where the Ni⋯Ni distances were ∼11.6 Å and Ni⋯Ni⋯Ni angles were in the range of 89.0–89.5°. DC magnetic data showed a rapid descent of the χMT value at lower temperatures which revealed depopulation of the Stark sublevels of the DyIII 6F15/2 state and also NiII⋯DyIII antiferromagnetic interactions. AC magnetic susceptibility data (at <3 K) showed frequency dependent χ′′M signals. Another oxime based homometallic complex [Dy3vanox2(Hvanox)4(EtOH)2](ClO4)·1.5EtOH·H2O (27, Fig. 11) (vanox = Schiff-base o-vanillin oxime) was reported by Powell et al.44 The complex contained a nearly linear Dy3 core, with Dy⋯Dy distances of ∼3.7 Å and a Dy⋯Dy⋯Dy angle of 166.29°. Of the three atoms, the coordination geometry of two Dy centres was between dodecahedral and square antiprismatic while the environment of the remaining Dy is a nearly perfect dodecahedron. Static magnetic susceptibility data showed weak ferromagnetic intra-complex interactions in the lower temperature regime which was also confirmed from the ab initio calculations providing a coupling constant value of +3.9 K. In the out-of-phase signal at higher frequencies, more than two maxima were observed which was indicative of multiple relaxation processes and confirmed the SMM nature of the compound. Modelling the data according to the Arrhenius equation results in Ueff values of 28.7 and 69.3 K and τ0 of 6.3 × 10−5 and 5.9 × 10−8 s, below and above 6 K respectively.
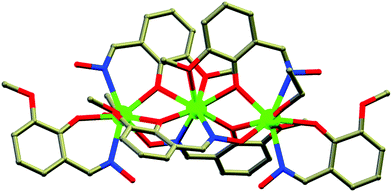 |
| Fig. 11 Molecular structure of 27. Color code: green – Dy, grey – C, red – O, blue – N. | |
Homometallic 3d based oximate complexes with high nuclearity and behaving as SMMs are also well-accounted in the literature.45 A few examples include [MnIII6O2(Et-sao)6(O2CPh(Me)2)2(EtOH)6] (Et-saoH2 = 2-hydroxyphenylpropanone oxime) (28)46 and the mixed valence compound [MnII/III15O0.5(OH)11.5(pao)18(EtOH)(H2O)](ClO4)6.5·5MeCN·6H2O (29).4728 shows an Mn–N–O–Mn torsion angle of 39.1° and simulation of the DC magnetic susceptibility data confirms the presence of ferromagnetic interactions in the complex with J = +1.6 cm−1. Low temperature and high field magnetization data suggested S = 12 ground state, with g = 1.99 and D = −0.43 cm−1. Frequency-dependent χ′′M signals were observed below ∼10 K and fit of these data according to the Arrhenius equation yielded Ueff = 86.4 K and τ0 = 2 × 10−10 s. On the other hand, complex 29 is one of the largest mixed valent Mn clusters so far known and presented a rare closed-like structural topology. Solid-state DC magnetic susceptibility data gave a clear indication of predominant antiferromagnetic exchange interactions and fitting of the magnetization data by matrix diagonalization provides parameters S = 6, g = 1.99 and D = −0.20 cm−1. The ground state value of 6 and the negative value of D indicated SMM behaviour of the complex with Ueff = 10.3 K.
(c) Schiff bases.
Significant numbers of Schiff bases (Scheme 6(i–iv)) and other macrocyclic ligands (porphyrins, cryptants etc.) are also exploited by several groups as a chelating ligand for MNM synthesis.48 Some of them have even been employed for heterometallic cage preparation. In this case the choice of donor atoms in the ligand plays an important role to place the metals at preferred sites. Few of the Schiff base complexes that behave as good candidates for SMMs are accounted here. An interesting dimeric MnIII-Schiff base complex, [Mn(saltmen)(N3)]2 (30) (H2saltmen = condensation product of salicylaldehyde and 1,1,2,2-tetramethylethyldiamine), was reported by Gao et al.49 and can be referred to as a good example in this class. In this complex two MnIII centres within one dimer were connected through phenolate oxygen atoms with a Mn–O–Mn angle of 98.4°. The intradimer Mn⋯Mn distance was found to be ∼3.9 Å while the nearest Mn⋯Mn separation between the dimers was ∼8 Å. DC magnetic susceptibility data show intradimer ferromagnetic interaction as was evident from the Mn–O–Mn angle, and simulation of the data yielded J = 0.6 cm−1. AC measurement manifested frequency dependency of both the χ′M and χ′′M signals even under 0 DC field which indicates the SMM behaviour of the complex. Following the Arrhenius law a lower Ueff value of 17 K was obtained at 0 DC field which was attributed to QTM. Applying a higher DC field of 1 kOe, QTM was minimized and a higher Ueff value of 29 K was achieved. In 2009, Wang and co-authors showed an innovative way for the synthesis of a new class of SMMs, by incorporating non-SMM {MnIII2}-Schiff base complexes into a series of polyoxometallate (POM) systems.50 This was done taking advantage of the fact that POM can well separate high-spin anisotropic units, thereby preventing any possible intermolecular exchange and revealing the intrinsic SMM behaviour of the MnIII-Schiff base complexes. These new complexes formulated as [Mn(salen)(H2O)]2Na[XMo6(OH)6O18]·20H2O (X = Al and Cr, salen = N,N′-bis(salicylideneaminato)-ethylene) (31) consisted of [Mn(salen)(H2O)]22+ dimers, [XMo6(OH)6O18]3− polyoxoanions, Na+ cations and H2O molecules and exhibited an alternate arrangement of inorganic and metal-organic layers. The separation between two {Mn2} units in a particular layer was ∼8 Å, while the shortest distance between {Mn2} units in two alternate layers was ∼15 Å. These considerable distances helped in reducing the inter-complex antiferromagnetic interactions dramatically as compared to the precursor MnIII-salen complex. DC magnetic susceptibility studies showed ferromagnetic interaction with J = +0.89 and 0.63 K while AC measurements at zero as well as higher fields revealed frequency dependent χ′′M signals, suggesting SMM behaviour. Using Arrhenius’ equation Ueff values of 13.2 and 9.1 K and τ0 = 7.2 × 10−7 s and 16 × 10−7 s were obtained. Another example is the mixed (phthalocyaninato)(Schiff-base)–Dy sandwich complex [Dy2(Pc)2(L)H2O] (32, Fig. 12) (H2Pc = phthalocyanine, H2L = N,N′-bis(3-methyloxysalicylidene)benzene-1,2-diamine) reported by Jiang et al.51 The complex had a triple-decker structure with two phthalocyanine ligands, one common Schiff-base ligand and two crystallographically independent Dy ions. One of the Dy centres had a square anti-prismatic geometry while the other adopted a seven-coordinated geometry. An intramolecular Dy⋯Dy separation of ∼3.8 Å gave a probable indication of the presence of intramolecular interionic magnetic interactions which was confirmed by the static DC magnetic investigation. Analysis disclosed a strong ferromagnetic coupling between the two Dy ions. AC susceptibility measurements showed slow relaxation of magnetization; however no full peaks were observed at 0 field because of QTM, which was suppressed by an optimum field of 2000 Oe. From the data at 2000 Oe, a Ueff value of 28.5 K and τ0 = 9.5 × 10−7 s were determined. Very recently, SMM behaviour of a new asymmetric ErIII-Schiff base complex, [Er(HL)2(NO3)3] (HL = 2-methoxy-6-[(E)-phenyliminomethyl]phenol) (33), was studied both experimentally and theoretically by Rajaraman and Shanmugam et al.52 The unit cell of this complex contained two molecules with the same molecular formula but with different orientations of ligands around the metal centre as is evident from the N–Er–N angles and the ErIII ion existed in ten coordinate distorted bicapped square anti-prism geometry. AC measurements showed frequency dependent χ′′M signals even in 0 applied field which was a rare feature for such a type of asymmetric complex. The molecular origin of the magnetic relaxation was also proved by a dilution method in a 50% diluted sample of the complex. The Ueff value obtained by fitting the AC data of the diluted sample was 51.4 K, which was significantly lower than for the organometallic ErIII mononuclear SMMs. Ab initio calculations were performed to analyse the g-tensors, relative energies of Kramers doublets (KDs), crystal field parameters etc. and it was observed that the principal magnetization axes (gzz) were oriented towards one of the –O atoms of the attached –NO3 ligand. Calculations also showed that the principal magnetization axes of the first excited states for the two molecules in the unit cell were tilted by 86.8 and 90° from their ground states and this determined the barrier heights to be 70.4 K and 150.9 K. This disparity between the experimental and theoretical observations was attributed to QTM existing between the ground state KDs, hyperfine interactions and strong intermolecular interactions.
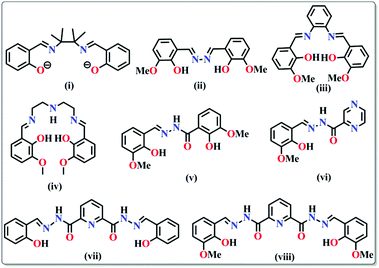 |
| Scheme 6 Commonly used Schiff bases (i–iv) and hydrazone (v–viii) ligands. | |
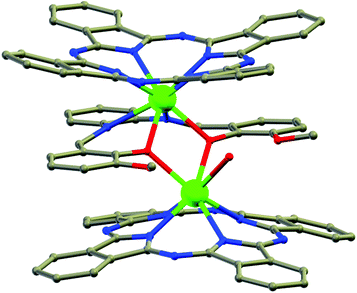 |
| Fig. 12 Molecular structure of 32. Color code: green – Dy, grey – C, red – O, blue – N. | |
(d) Hydrazones.
Hydrazones are another class of ligands which provide several O, N-based multichelating sites (Scheme 6(v–viii)) favourable for the formation of 3d as well as 3d–4f polynuclear nanoscopic complexes. Several hydrazone based complexes are reported by groups like Thompson, Tang and a few others including us.53 The linear tetranuclear DyIII complex [Dy4(L)4(MeOH)6]·2MeOH (34, Fig. 13) (H3L = (2-hydroxy-3-methoxyphenyl)methylene hydrazide) reported by Tang and co-workers is an important example of this category.54 The complex consisted of a Dy4 chain with Dy–Dy–Dy angles of 149.99(1)° and the DyIII ions are bridged by anionic phenolate or amido oxygen atoms. The two internal DyIII ions are eight-coordinated with a distorted bicapped trigonal prismatic geometry and the two terminal DyIII ions are nine-coordinated and display a monocapped square antiprismatic geometry. Frequency and temperature dependences of the χ′′M AC susceptibility data show the presence of two relaxation phases corresponding to the high-frequency peaks (fast relaxation phase, FR) and the low-frequency peaks (slow relaxation phase, SR). From the Arrhenius plots, Ueff = 19.59 K (τ0 = 7.8 × 10−6 s) and 171.6 K (τ0 = 1.2 × 10−7 s) were obtained for the FR and SR phases respectively. Thompson and co-workers reported a very interesting DyIII based [2 × 2] square grid SMM using a ditopic carbohydrazone ligand (L1).55 The complex [Dy4(L1-2H)2(L1-H)2(N3)4(O)]·14H2O (35) contained four DyIII ions which occupied two tridentate N2O pockets of the four ligands and each of the DyIII ions was nine coordinated with an N6O3 coordination environment. The four DyIII ions were bridged by a central μ4-O (oxide) ion and the Dy–O(central)–Dy angle was in the range of 88.9–91.4° which indicated the square planar arrangement of the Dy4(μ4-O) subunit. AC magnetic susceptibility data show frequency dependency of the χ′′M signals even in 0 applied field at temperatures below 35 K, with a large shoulder at around 20 K which suggests the presence of two relaxation processes. From the de-convoluted peak positions and using the Arrhenius equation, Ueff values of 51 K (τ0 = 3.0 × 10−9 s) and 91 K (τ0 = 4.5 × 10−7 s) were obtained for the low and high temperature domains respectively. Our group recently reported two new DyIII based trinuclear and hexanuclear SMMs,5 using two similar flexible and multidentate carbohydrazide ligands bis[(2-pyridyl)methylene]pyridine-2,6-dicarbohydrazide (H2L1) and bis[2-hydroxy benzylidene]pyridine-2,6-dicarbohydrazide (H4L2)6 and the complexes are formulated as [Dy3L12(H2O)9](Cl)5·6H2O (36) and [Dy6L22(HCO2)4(μ3-OH)4(DMF)6(H2O)2](Cl)2·4H2O (37) respectively. In the trinuclear complex 36 two L1 bound three DyIII ions in a twisted fashion at an angle of 71.98° on one side and at an angle of 70.79° on the other side and the packing diagram showed a double helical arrangement of the complex through intramolecular and intermolecular H-bonding as well as π–π interactions. All the DyIII centres in the complex exhibited a nine coordinated tri-capped trigonal prismatic geometry. The hexanuclear complex, 37, on the other hand, presented a different structural feature composed of four distorted hemi-cubane like units each of which shared both edges and vertices with its neighbouring units. The DyIII ions in this case are octa-coordinated with a square antiprismatic geometry. From DC magnetization data, non-superimposition of M vs. H/T curves was observed which confirms the presence of magnetic anisotropy in both the molecules. For the trinuclear complex, at 0 DC field the χ′M AC signal was frequency independent but χ′′M shows frequency dependency. The hexanuclear complex shows frequency dependency in both χ′M and χ′′M signals. At higher DC fields QTM could be much more suppressed and prominent frequency dependency was observed in χ′M and χ′′M signals for both the tri- and hexanuclear complexes. Using the Debye equations, Ueff values of 11.2 K (τ0 = 3.1 × 10−6 s−1) and 9.7 K (τ0 = 6.4 × 10−6 s−1) were obtained respectively. This difference in the energy barrier values was attributed to the disparity in coordination geometry around DyIII centres which affected the orientation of the easy-axes and magnetic anisotropy in the complexes.
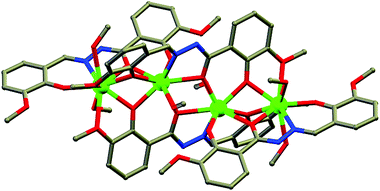 |
| Fig. 13 Molecular structure of 34. Color code: green – Dy, grey – C, red – O, blue – N. | |
B. Choice of metal ions
With the birth of Mn12Ac as an SMM, a lot of effort has been devoted towards the discovery of new and remarkable molecular magnets. These resulted in the addition of a large number of attractive SMMs in the literature; but most of these were based on MnIII or mixed valent MnII/III complexes. Gradually, other transition metals like V, Cr, Fe, Co and Ni were also explored. Most of the manganese and vanadium based SMMs are mixed valent (+2, +3 for Mn and +4, +5 for V); cobalt and iron based magnets are based on either +2 or +3 oxidation states whereas in Ni-based SMMs, nickel ions are found only in the +2 oxidation state.
In the recent past, considerable attention has been given to the design of lanthanide based SMMs. The large amount of spin in conjunction with spin–orbit coupling in certain Ln ions like TbIII, DyIII, HoIII and ErIII makes them attractive candidates for SMM. Because of different ground state bi-stability, the magnetic properties of the Ln based SMMs are different from transition metal SMMs. For 3d transition metals, the ligand field effects are much more pronounced than the spin–orbit coupling and the total spin S and the ensuing [2S + 1] Ms substates lead to ground-state bi-stability. In contrast, for Ln ions, the orbital contribution to the magnetic moment is large and unquenched (except for the ions with ground electronic terms 1S0 and 8S7/2), and ligand field effects although small can be regarded as significant perturbation. Therefore, the [2J + 1] mJ microstates within the spin–orbit-coupled ground term, (2S+1)LJ, give rise to the ground-state bi-stability. A simple consideration of the number of unpaired electrons in Ln ions gives insignificant idea of the magnetic properties of lanthanide-based complexes. Furthermore, it has been observed that, although the exchange interactions for orbitally degenerate 3d ions and their complexes are strong (J ∼ 10–100 cm−1), their exchange anisotropy is rather weak (r ∼ 0.1–0.5). On the other hand, the 4f ions and their complexes have high exchange anisotropy (r ∼ 1), but weak exchange interactions (J < 10 cm−1). In this regard, the 4d and 5d ions can be the best candidates over 3d and 4f metal ions to design high temperature SMMs. That is because the 4d and 5d magnetic orbitals are more diffuse than 3d orbitals and they overlap much better with the valence orbitals of bridging groups, thus providing much higher exchange parameters for a similar molecular geometry. Moreover, in 3d orbitally-degenerate complexes distortions quench the orbital momentum, while in 5d complexes the orbital momentum remains unquenched. In 4d complexes the situation is intermediate. Very recently, 5f (especially uranium) and mixed 3d–5f compounds have been identified as attractive candidates to manifest SMM behaviour because of the high magnetic anisotropy of the uranium ion over a range of oxidation states, combined with its ability to engage in strong magnetic interactions with other metal ions. All these factors contribute greatly towards the fabrication of mixed 3d–4d, 3d–5d, 4d–5d as well as 4d–4f and 3d–5f based SMMs besides the well explored 3d, 4f and 3d–4f based SMMs.
B(I) Single metal/mononuclear SMMs.
Recently, effort has been made to search for the smallest possible SMMs where a single ion can act as an SMM. In this method single metal ions are being used as magnetic centres and the molecules could behave as nanomagnets.57 In most of the cases lanthanides have been introduced as metal ions because they possess stronger single-ion anisotropy and slower relaxation as compared to 3d, 4d and 5d ions. The common synthetic strategy is to choose a ligand system which could provide an axial crystal field acting on the 4f-ion and stabilizing a Stark sublevel with a large absolute value of the total angular momentum projection |MJ|, thus an easy axis of magnetization is achievable. Ishikawa and co-workers have explored a class of SIMs with phthalocyanine and related macrocyclic ligands having double or triple decker structures.58 Phthalocyanines have four N-donation sites which are less in number than that required for a lanthanide to satisfy its coordination number and the cavity of the ligand is not big enough to house such large cations. Therefore, they form a mononegative ion having the general formula [LnPc2]− and double decker structure where the metal centres are sandwiched between two deprotonated ligands (Fig. 14a).59 The antiprismatic D4d crystal field (CF) induced by the octacoordinated atoms around the LnIII ion splits its ground magnetic state into ±MJ sublevels. This creates a barrier that explains the SMM behaviour observed in the Tb derivative with the maximum MJ value = ±6. Another important class of SIMs is based on polyoxometallates (POM).60 The first POM based SIM was reported by AlDamen et al. in 2008.61 It is a sodium salt of [ErW10O36]9− polyanion (38, Fig. 14b and Table 2) which consisted of two anionic [W5O18]6− moieties sandwiching the ErIII ion. Each anionic [W5O18]6− moiety is twisted with respect to the other with a skew angle of 44.2°. This angle is very near that expected for an ideal D4d symmetry (Φ = 45°) and hence the coordination geometry was described as slightly distorted square-antiprismatic. DC magnetic susceptibility data followed the Curie–Weiss law in the high-temperature region affording a Curie constant C = 11.4 cm3 K mol−1 and a Weiss constant Θ = −10.5 K and fitting of the low temperature AC magnetic susceptibility data provided Ueff = 55.2 K and τ0 = 1.6 × 10−8 s. 1,4,7,10-Tetraazacyclododecane-N,N′,N′′,N′′′-tetraacetic acid (H4DOTA) was also employed as a promising alternative to the phthalocyanine derivative for the synthesis of mononuclear SMMs. For example, in this complex, Na[Dy(DOTA)(H2O)]·4H2O (39),62 the Dy ion, resides in an ideal square antiprismatic environment with a twist angle of 39°. AC magnetic susceptibility measurement showed slow relaxation of magnetization behaviour and a linear nature in the Arrhenius plot in the presence of an external DC field of 900 Oe down to ∼2.8 K, with Ueff = 61 K and τ0 = 7 × 10−11 s. Another interesting mononuclear SMM complex, [Dy(acac)3(H2O)2] (40),63 was synthesized using the acetylacetonate (acac) ligand where the DyIII centre was octa-coordinated by eight oxygen atoms and forms a square-antiprismatic coordination polyhedron with a local symmetry close to D4d. Both χ′M and χ′′M AC susceptibilities show frequency dependence and fitting to the Arrhenius equation gave Ueff = 66.1 K and τ0 = 8 × 10−7 s. Gao et al. had reported an organometallic SIM, (Cp*)Er(COT) (41), where an ErIII ion is sandwiched between the two aromatic rings, pentamethylcyclopentadienide (C5Me5− = Cp*) and cyclooctatetraenide (C8H82− = COT).64 This suggested that by using delocalized ligands it is possible to design SIMs with prominent uniaxial anisotropy. The ErIII ion was located in a low-symmetry coordination environment with Cs point group. The molecule shows a butterfly-shaped hysteresis loop in the temperature range of 1.8–5 K. AC magnetic susceptibility measurements disclosed the presence of two thermally activated magnetic relaxation processes with Ueff values of 197 and 323 K respectively. Recently our group has also reported two mononuclear complexes, [Dy(H4daps)(H2O)3(NO3)]·(NO3)2·H2O (42) and [Dy(H3daps)(H2O)2(NO3)]·(NO3)·MeOH (43). The molecules were obtained from the reaction of Dy(NO3)3 and a pentadentate organic ligand, H4daps (H4daps = 2,6-bis(1-salicyloylhydrazonoethyl)pyridine).65 In comparison to complex 42, complex 43 shows a slight difference in local symmetry around the DyIII centre and that was attributed to the deprotonated ligand and also to different binding modes of the peripheral NO3− anion. AC magnetic susceptibility measurements show that both complexes exhibit SMM behaviour, with Ueff = 32.7 K (τ0 = 1.82 × 10−6 s) and 23.8 K (τ0 = 9.14 × 10−5 s) for 42 and 43 respectively. Our investigation revealed that small differences in the coordination environment around the DyIII centre can play a significant role in the difference in relaxation dynamics of the complexes. The tuning of magnetic properties by a small modification in the ligand system around the DyIII centre was also investigated by Mallah et al.66a Two isostructural complexes DyIII-N,N′-bis(imine-2-yl)methylene-1,8-diamino-3,6-dioxaoctane (44) and DyIII-N,N′-bis(amine-2-yl)methylene-1,8-diamino-3,6-dioxaoctane (45) were synthesized where the two imine groups were replaced by amine groups in the latter.66b The coordination geometry around the DyIII centre was best described as a distorted bicapped square antiprism with D4d symmetry. The main difference arises from the ligand field because imine nitrogen atoms provide a stronger ligand field than amine nitrogen atoms. The stronger ligand field possesses a larger electron density and results in a larger effective energy barrier for 44. From the fitting of the Arrhenius plots different Ueff values of 50 K and 34 K and τ0 values of 6.8 × 10−7 s and 2.5 × 10−6 s were obtained for 44 and 45 respectively. In parallel with the extensive study on 4f based SIMs, significant effort has also been dedicated to the research on 3d metal based SIMs. In 2010, Chang and Long et al. have reported the trigonal pyramidal [(tpaMes)Fe]− complex (46) with C3v symmetry which was the first example of a mononuclear transition metal based SMM.67 To probe the magnetization dynamics of 46, AC susceptibility data were collected in the presence of an applied DC field and frequency-dependent peaks were observed in χ′′Mvs. T plots. From the Arrhenius plot, effective spin-reversal barrier Ueff ∼ 60 K and τ0 = 2 × 10−9 s were calculated. Several other transition metal based SIMs with a variety of coordination geometries68 have been accounted so far. Recently, Mallah et al. have reported a trigonal bipyramidal NiII complex, [Ni(Me6tren)Cl]·(ClO4) (47),69 where the ligands are located at the apices of a trigonal bipyramid with C3 symmetry. The complex shows a huge uniaxial magnetic anisotropy with an experimental D value estimated to be between −120 and −180 cm−1. In 2014, Ruiz et al. have reported a trigonal-pyramidal NiII complex, K{Ni(N[CH2C(O)NC(CH3)3]3)} (48) with the largest magnetic anisotropy ever reported.70 The experimental D value was found to be −200 cm−1, which is in good agreement with the theoretical value of −244 cm−1. Along with the successful advances in 3d and 4f metal based SIMs, uranium based SIMs are currently attracting great attention because of their large single-ion anisotropy.71 The first monometallic UV complex, [U(O)-(TrenTIPS)] (49) [TrenTIPS = {N(CH2CH2NSiiPr3)3}3−],72a was reported by Liddle and McInnes. The complex was synthesized by two-electron oxidation of the trivalent uranium complex [U(TrenTIPS)]72b with trimethyl-N-oxide in toluene. Structural characterization reveals distorted a trigonal bipyramidal geometry of the UV centre with tertiary amine and oxo groups occupying the axial positions. AC magnetic measurements, under a small DC field of 1 kG, showed a frequency dependency of both χ′M and χ′′M suggesting SMM behaviour of the complex. The ln(τ) vs. T−1 plot was fitted according to the Arrhenius law which provided Ueff = 21.5 K and τ0 = 2.6 × 10−7 s.
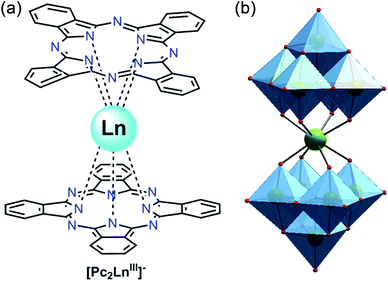 |
| Fig. 14 (a) View of the first SIM (Ln = Tb, Dy); (b) structure of 38. Lemon green – Er. | |
Table 2 Compilation of the SMMs based on different metals discussed in this review
No. of metal centre based SMMs |
Formulae of complexes |
U
eff (K) |
τ
0 (s) |
Ref. |
(I) Mononuclear SMMs
|
|
[ErW10O36]9− (38) |
55.2 |
1.6 × 10−8 |
61
|
|
Na[Dy(DOTA)(H2O)]·4H2O (39) |
61 |
7 × 10−11 |
62
|
|
[Dy(acac)3(H2O)2] (40) |
66.1 |
8 × 10−7 |
63
|
|
(Cp*)Er(COT) (41) |
197 |
— |
64
|
323 |
— |
|
[Dy(H4daps)(H2O)3(NO3)](NO3)2(H2O) (42) |
32.7 |
1.82 × 10−6 |
65
|
|
[Dy(H3daps)(H2O)2(NO3)](NO3)(MeOH) (43) |
23.8 |
9.14 × 10−5 |
65
|
|
DyIII-N,N′-bis(imine-2-yl)methylene-1,8-diamino-3,6-dioxaoctane (44) |
50 |
6.8 × 10−7 |
66b
|
|
DyIII-N,N′-bis(amine-2-yl)methylene-1,8-diamino-3,6-dioxaoctane (45) |
34 |
2.5 × 10−6 |
66b
|
|
[(tpaMes)Fe]− (46) |
60 |
2 × 10−9 |
67
|
|
[Ni(Me6tren)Cl]·(ClO4) (47) |
— |
— |
69
|
|
K{Ni(N[CH2C(O)NC(CH3)3]3)} (48) |
— |
— |
70
|
|
[U(O)-(TrenTIPS)] (49) |
21.5 |
2.6 × 10−7 |
72a
|
(II) Polynuclear SMMs
|
(a) 3d based |
K6[V15As6O42(H2O)]·8H2O (V15) (50) |
— |
— |
75
|
(NH4)6[V14As8O42(SO3)] (V14) (51) |
— |
— |
75
|
[V4O2(O2CEt)7(bpy)2](ClO4) (52) |
19.4 |
— |
76
|
[V4O2(O2CEt)7(pic)2](NEt4) (53) |
— |
— |
76
|
[Mn22O6(OMe)14(O2CMe)16(tmp)8(HIm)2] (54) |
19 |
3 × 10−11 |
81e
|
[Fe8O2(OH)12(tacn)6]8+ (55) |
29 |
— |
83
|
[Fe19(metheidi)10(OH)14O6(H2O)12](NO3)·24H2O (56) |
15.7 |
— |
84
|
[Co4(hmp)4(MeOH)4Cl4] (57) |
— |
— |
86
|
[Co12(bm)12(NO3)(O2CMe)6(EtOH)6](NO3)5 (58) |
15 |
1.94 × 10−7 |
87
|
[Ni12(chp)12(O2CMe)12(thf)6(H2O)6] (59) |
9.6 |
— |
89
|
[Ni10(tmp)2(N3)8(acac)6(MeOH)6]·H2O (60) |
14 |
— |
90
|
(b) 4f based |
[Dy2(hmi)2(NO3)2(MeOH)2] (61) |
56 |
3 × 10−7 |
93
|
|
71 |
7 × 10−8 |
[Dy2(ovph)2Cl2(MeOH)3]·MeCN (62) |
— |
— |
94
|
[Dy3(Hsal)5(sal)2(phen)3] (63) |
45 |
1.5 × 10−5 |
96
|
[Dy4(μ3-OH)2(bmh)2(msh)4Cl2] (64) |
6.7 (LT) |
3.2 × 10−5 |
97
|
118 (HT) |
4 × 10−7 |
[Dy6(μ3-OH)3(μ3-CO3)(μ-OMe)(HL)6(MeOH)4(H2O)2]·3MeOH·2H2O (65) |
26 |
3.8 × 10−6 |
98
|
3.9 |
4.2 × 10−5 |
[Dy7(OH)6(thmeH2)5(thmeH)(tpa)6(MeCN)2][NO3]2 (66) |
140 |
7.2 × 10−9 |
99
|
[Dy30I(μ3-OH)24(μ3-O)6(NO3)9(IN)41(OH)3(H2O)38] (67) |
— |
— |
100
|
[Dy(hfac)3L]n·H2O (68) |
16.35 |
1.81 × 10−6 |
101
|
(c) 3d–4f based |
[Ni(μ-L)(μ-BzO)Dy(NO3)2] (69) |
9.2 |
4.4 × 10−6 |
103
|
[Ni(μ-L)(μ-9-An)Dy(9-An)(NO3)2] (70) |
10.1 |
3.4 × 10−6 |
103
|
[CoII2Dy(LH3)4](NO3)3 (71) |
— |
— |
104
|
[Ni2Dy2(μ3-OH)2(OH)(OAc)4(HL)2(MeOH)3](ClO4) (72) |
7.6 |
7.5 × 10−6 |
105
|
[CoII2(L)2(PhCOO)2DyIII2(hfac)4] (73) |
8.8 |
2.0 × 10−7 |
106
|
7.8 |
3.9 × 10−7 |
[Zn3Dy(LPr)(NO3)3(MeOH)3]·4H2O (74) |
25.8 |
— |
108
|
[Cr4Dy4(μ3-OH)4(μ-N3)4(mdea)4(piv)4]·3CH2Cl2 (75) |
15 |
1.9 × 10−7 |
109
|
[MnIII9MnII2Gd2(O)8(OH)2(piv)10.6(fca)6.4(NO3)2(H2O)]·13CH3CN·H2O (76) |
18.4 |
— |
110
|
[Mn21DyO20(OH)2(ButCO2)20(HCO2)4(NO3)3-(H2O)7] (77) |
74 |
— |
111
|
[Fe12Sm4(μ4-O)6(μ3-O)4(μ3-OH)4(PhCO2)24] (78) |
|
|
112
|
(H3O)[Cu24Dy8(Ph3CPO3)6(PhCPO3H)6(OAc)12(HOAc)6(OH)42(NO3)(H2O)6] (79) |
4.6 |
2.1 × 10−8 |
113
|
(d) 3d–4d/3d–5d & 4d–4f based |
[(PY5Me2)4Mn4Re(CN)7](PF6)5·6H2O (80) |
47.5 |
2.4 × 10−8 |
115
|
(NEt4)[Mn2(5-Brsalen)2(MeOH)2Os(CN)6] (81) |
20 |
— |
116
|
K[(Me3tacn)6MnMo6(CN)18]–(ClO4)3 (82) |
14.3 |
7 × 10−7 |
117
|
[RuIII2DyIII2(OMe)2(O2CPh)4(mdea)2(NO3)2] (83) |
16 |
4.1 × 10−6 |
118
|
(e) 3d–5f based |
[{UO2(salen)}2Mn(Py)3]6 (84) |
142 |
3 × 10−12 |
119b
|
[{[Mn(TPA)I][UO2(Mesaldien)][Mn(TPA)I]}I] (85) |
81 |
5.02 × 10−10 |
119c
|
B(II) Polynuclear SMMs.
B(II) (a) 3d-based SMMs.
Compared to other 3d transition metals,73 aggregates of VIII (d2, S = 1) which behave as molecular magnets74 are in fact less reported and that is mainly because of the air-sensitivity of VIII oxidation state. Gatteschi, Müller and co-authors first reported vanadium based magnetic complexes which were formulated as K6[V15As6O42(H2O)]·8H2O (V15) (50) and (NH4)6[V14As8O42(SO3)] (V14) (51).75 V15 is important because of its behaviour as a magnetic multilayer, whereas in V14, many different spin orientations in different zones of the molecule result in several different exchange pathways. A couple of interesting vanadium complexes with the V4O2 ‘butterfly-type’ core was reported by Christou et al.76 The complexes were formulated as [V4O2(O2CEt)7(bpy)2](ClO4) (52) (bpy = 2,2′-bipyridine) and [V4O2(O2CEt)7(pic)2](NEt4) (53) (pic = 2-picolinate) and prepared in acetone from the reaction of VCl3(THF)3, the corresponding chelate and NaO2CEt under anaerobic conditions. In both the complexes the oxide ions were positioned slightly out of their respective V3 planes and DC magnetic susceptibility measurements show that the complex 52 exhibits spin frustrated behaviour. The presence of S = 3 ground state and D ≈ −1.5 cm−1 results in an appreciable Ueff ≈ 19.4 K.
Literature study reveals that manganese based SMMs77–81 form the largest group in the 3d-based single-molecule magnets. The first and still the best studied SMMs are the mixed-valent Mn12 complexes [Mn12O12(O2-CR)16(H2O)4] (R = Me, Et etc.) with S = 10 ground state, Ueff ≈ 74 K and Tb = 3.5 K.77 Among the higher nuclearity Mn complexes, the complex that needs special mention is a Mn22 system having the formula [Mn22O6(OMe)14(O2CMe)16(tmp)8(HIm)2] (54, Fig. 15) [tmp = 1,1,1-tris-(hydroxymethyl)propane and HIm = imidazole] reported by Brechin et al.81e The molecule consists of a metallic core of two MnIV, eighteen MnIII and two MnII ions and the metal–oxygen core is made up of [Mn3O4] partial cubes and [Mn3O] triangles. Preliminary AC magnetic susceptibility measurements showed frequency and temperature dependency confirming the SMM behaviour of the complex with Tb ∼ 1.3 K. Magnetization vs. applied DC field measurements at a field sweep rate of 0.07 T s−1 result in a wide hysteresis loop without any step-wise feature which indicates the occurrence of QTM in the molecule. Fitting of the AC data according to the Arrhenius law yielded Ueff = 19 K and τ0 = 3 × 10−11 s.
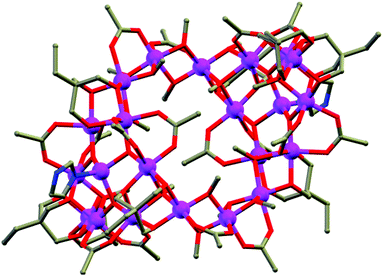 |
| Fig. 15 Molecular structure of 54. Color code: purple – Mn, grey – C, red – O, blue – N. | |
Among the Fe based SMMs,82 the Fe8 cage reported by Sessoli and co-authors83 is worthy of special mention. The cationic complex [Fe8O2(OH)12(tacn)6]8+ (55) (tacn = 1,4,7-triazacyclononane) with a ‘butterfly’ like arrangement of the eight Fe atoms was the second reported Fe based molecular species to show SMM behaviour with an appreciable Ueff value of 29 K. Another important high-nuclearity complex, [Fe19(metheidi)10(OH)14O6(H2O)12](NO3)·24H2O (H3metheidi = N-(1-hydroxymethylethyl)iminodiacetic acid)84 (56), having a large ground state spin S = 33/2 was reported by Goodwin et al. in 2000. The field dependence of magnetisation showed saturation with a value of 32.5μB at the highest field of 70 kOe, which is in good agreement with the expected value for S = 33/2 and g = 2. The obtained effective energy barrier for the reversal of magnetization was 15.7 K.
Cobalt complexes can also be considered as suitable candidates for SMM85 behaviour as the CoII ion possesses three unpaired electrons (S = 3/2) and displays large single-ion anisotropy. The first CoII based SMM [Co4(hmp)4(MeOH)4Cl4] (57)86 (hmp− = anion of hydroxymethylpyridine) was reported by Christou and co-workers in 2002. Variable-field and variable-temperature magnetization data showed that the molecule has an S = 6 ground state with axial zero-field splitting parameter of −4 K. Another fascinating higher nuclearity CoII SMM is [Co12(bm)12(NO3)(O2CMe)6(EtOH)6](NO3)5 (58, Fig. 16) (Hbm = (1H-benzimidazol-2-yl)methanol) reported by Zeng et al.87 The complex was a rare example of a 3d-metal cage with both O and N ligation. AC magnetic susceptibility measurement showed the slow relaxation effects, which was evident from the frequency dependency of the χ′M and χ′′M plots. Fitting of the Arrhenius equation for the magnetization data gave Ueff = 15 K and τ0 = 1.94 × 10−7 s.
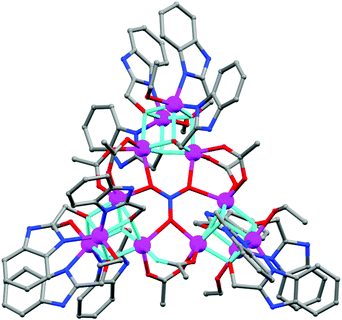 |
| Fig. 16 Molecular structure of 58. Color code: pink – Co, grey – C, red – O, blue – N. | |
Ni-based SMMs88 are relatively new in the family of 3d based SMMs. The first NiII based SMM, [Ni12(chp)12(O2CMe)12(thf)6(H2O)6] (59), was reported by Winpenny et al. in 2001.89 The complex exhibits a cyclic structure with twelve NiII centres bridged by pyridonate and acetate ligands. It contains an S6 symmetry and all rings within the structure pack parallel to each other, and perpendicular to the crystallographic c-axis. Isothermal magnetisation curve showed a saturation value of 25.5μB, which confirms S = 12 ground state and g = 2.13 and fitting of the data provided a D/k value of −0.07 K (k = Boltzmann constant). The effective energy barrier for the reversal of magnetization was calculated from the equation Ueff = |D|S2 which yields Ueff = 9.6 K. Another important NiII based decametallic SMM [Ni10(tmp)2(N3)8(acac)6(MeOH)6]·H2O (60) (H3tmp = 1,1,1-tris(hydroxymethyl)propane)90 was reported by Brechin et al. The basic structure contained a planar disc-like [Ni10O10N8]2+ unit held together by a combination of tmp3− and N3− ligands and contained ten edge-sharing [Ni3] triangles. The complex displays one of the largest spin ground states S = 10 among the reported Ni based clusters and is a rare example of ferromagnetic Ni based SMM. It also exhibits the largest Ueff value of ∼14 K. Besides the above mentioned 3d based SMMs, various groups also reported the SMM behaviours of mixed transition metal (3d–3d, 3d–4d, 3d–5d) cages.91 We are unable to cover all of them in this review, but a few of them are discussed in section B(II)(d).
B(II) (b) 4f-based SMMs.
Along with the 3d based SMMs, the lanthanide based polynuclear SMMs92 also accounted for some of the most striking progress in molecular magnetism. DyIII based SMMs (on increasing nuclearity basis) have been discussed first, since they form the largest class in the LnIII based SMMs. An interesting example of a dinuclear Dy based complex, [Dy2(hmi)2(NO3)2(MeOH)2] (61), was reported by Murugesu et al. in 2008.93 The complex was synthesized using a rigid chelating ligand {(2-hydroxy-3-methoxyphenyl)methylene(isonicotino)hydrazine} (H2hmi). The complex exists as an isolated {Dy2} molecule as well as extended into 2D sheets of [Dy2(hmi)2(NO3)2(MeOH)2]n·MeCN on a slight change of the reaction conditions. The DyIII ions were bridged by two μ-phenoxide moieties from two ligands producing magnetic superexchange pathways. Large Ueff values of 56 K and 71 K and τ0 values of 3 × 10−7 and 7 × 10−8 s were observed for the dinuclear cluster and the 2D sheet respectively. Unsymmetrical dinuclear DyIII complexes offer chances to study how the interactions between DyIII ions with different coordination geometries affect the dynamic magnetic properties. This is well manifested in the phenolate-bridged dinuclear DyIII complex [Dy2(ovph)2Cl2(MeOH)3]·MeCN (H2ovph = ortho-vanillin picolinylhydrazone)94 (62) reported by Tang and Zhang et al. The complex contains an eight-coordinated and a seven-coordinated DyIII centre with intramolecular Dy⋯Dy distances of ∼3.8 Å and two Dy–O–Dy angles of 112.3° and 111.5°. DC magnetic susceptibility measurements disclose intramolecular ferromagnetic interaction between the DyIII ions which arise from a ferromagnetic dipolar interaction (Jdip = 5.36 cm−1) due to an almost parallel alignment of the local anisotropy axes and the line connecting the DyIII ions. AC magnetic measurements exhibit prominent temperature and frequency dependencies at 0 applied field revealing the SMM behaviour of the complex and also QTM at very low temperatures. Although quantum tunnelling is an usual feature of Ln-containing single-ion magnets, 62 is quiet unique in the sense that it showed ferromagnetic coupling as well as quantum tunneling simultaneously. While O-bridging is very well known in dinuclear LnIII complexes, bridging by other atoms like N, S etc. is a bit less explored. In this context, Layfield and co-authors have reported a few dinuclear LnIII complexes of different bridging atoms such as N, S and studied their effects on the strength of magnetic interaction in {Dy2} organometallic cyclopentadienyl (Cp) complexes.95 Fang et al. have reported a linear trimetallic DyIII based carboxylate-bridged complex, [Dy3(Hsal)5(sal)2(phen)3] (H2sal = salicylic acid) (63), which consisted of three chemically different eight-coordinated DyIII ions.96 The AC magnetic susceptibility measurements show their temperature dependency in two distinct temperature ranges. From the Arrhenius plot, Ueff = 45 cm−1 (τ0 = 1.5 × 10−5 s) was obtained when T = 15–35 K, and below 15 K, an abrupt transition to a temperature-independent regime was observed, indicating relaxation by QTM. Another interesting hydrazone based tetranuclear complex, [Dy4(μ3-OH)2(bmh)2(msh)4Cl2] (64)97 {H2bmh = bis(2-hydroxy-3-methoxybenzylidene)hydrazone and Hmsh = 3-methoxysalicylaldehyde hydrazone}, was reported by Murugesu et al. The complex consists of four coplanar DyIII ions, and each DyIII ion occupied eight-coordinate distorted square antiprismatic environments. The most important feature of this complex is the presence of two modes of magnetic relaxation. From Arrhenius plots, Ueff = 6.7 cm−1 (τ0 = 3.2 × 10−5 s) and Ueff = 118 cm−1 (τ0 = 4 × 10−7 s) were determined for the low and high temperature relaxation processes, respectively. Such behaviour in a weakly coupled molecular system is apparently due to the large intrinsic magnetic anisotropy of the DyIII ions. An example of a hexanuclear DyIII complex, [Dy6(μ3-OH)3(μ3-CO3)(μ-OMe)(HL)6(MeOH)4(H2O)2]·3MeOH·2H2O (65, Fig. 17),98 was reported by Tang et al. The complex was synthesized using the multidentate Schiff-base ligand (E)-N′-(2-hydroxybenzylidene)pyrazine-2-carbohydrazide (H2L). The structure is based on an irregular array of eight-coordinated DyIII ions with the μ-carbonate ligands. Study of the AC magnetic susceptibility data showed the presence of two relaxation processes with Ueff = 26 cm−1 (τ0 = 3.8 × 10−6 s) and 3.9 cm−1 (τ0 = 4.2 × 10−5 s). Collison et al. reported a heptametallic complex, [Dy7(OH)6(thmeH2)5(thmeH)(tpa)6(MeCN)2][NO3]2 (66) (thmeH3 = tris(hydroxymethyl)ethane, tpaH = triphenylacetic acid),99 in which six μ3-OH ligands bridge between the peripheral and central DyIII ions, alternating above and below the Dy7 plane. Applying the Arrhenius analysis to the AC susceptibility data above 10.5 K, a Ueff value of 140 K and τ0 = 7.2 × 10−9 s were obtained. Below 10 K the relaxation slowly changed from a thermally activated mechanism to a QTM regime. Another example of a high nuclearity Dy30 complex was reported by Gu et al. The complex formulated as [Dy30I(μ3-OH)24(μ3-O)6(NO3)9(IN)41(OH)3(H2O)38] (67) (HIN = isonicotinic acid)100 consists of six [Dy4(μ3-OH)4] tetrahedra connected to six DyIII ions. From the M vs. H data at 1.8 K, lack of saturation was observed which suggested that the presence of significant anisotropy or low lying excited states in the compound is in agreement with weak intra-complex interactions. The AC magnetic susceptibility data also showed temperature and frequency dependency of the χ′′M component which confirmed the SMM behaviour of the complex, but the characteristic relaxation time of the system could not be obtained in the available temperature range. The first example of a one dimensional chain DyIII complex which showed SMM behaviour with two distinct magnetic relaxation processes is [Dy(hfac)3L]n·H2O (68) (hfac− = 1,1,1,5,5,5-hexafluoroacetyl acetonate and L = (−)-4,5-pinenepyridyl-2-pyrazine).101 The geometry of the nine-coordinate DyIII ion was best described as a tricapped trigonal prism geometry. The frequency dependency of the AC magnetic susceptibility data showed SMM behaviour and Ueff = 16.35 K (τ0 = 1.81 × 10−6 s) was obtained.
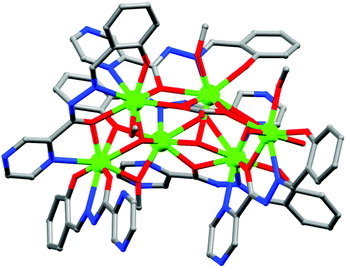 |
| Fig. 17 Molecular structure of 65. Colour code: green – Dy, grey – C, red – O, blue – N. | |
B(II) (c) 3d–4f based SMMs.
Recently a lot of interest has been focused on the area of mixed 3d–4f metal based complexes because of the fact that the significant magnetic exchange between 3d and 4f ions can efficiently overcome the zero-field QTM and increase the effective energy barrier for spin reversal.102 A series of triply bridged dinuclear complexes, [Ni(μ-L)(μ-BzO)Dy(NO3)2] (69) and [Ni(μ-L)(μ-9-An)Dy(9-An)(NO3)2] (70) (9-An = 9-anthracenecarboxylate), have been prepared by using a flexible non-Schiff base compartmental ligand, N,N′,N′′-trimethyl-N,N′′-bis(2-hydroxy-3-methoxy-5-methylbenzyl)di-ethylenetriamine) (H2L).103 Dynamic AC magnetic susceptibility measurements were carried out under a DC field of 1000 Oe and both the complexes exhibited typical SMM behaviour below 5 K with maxima in the range of 2.25–2.75 K and 2–3 K respectively. The thermally activated energy barriers were estimated to be 9.2 and 10.1 K and the flipping rates were 4.4 × 10−6 s and 3.4 × 10−6 s respectively. Another important linear trinuclear complex, [CoII2Dy(LH3)4](NO3)3 (71), was synthesized using the Schiff-base ligand 2-[2-hydroxy-3-(hydroxymethyl)-5-methylbenzylideneamino]2-methylpropane-1,3-diol) (LH4).104 In the complex, each of the two terminal CoII centres are bound by two deprotonated LH3− ligands and bonded to two phenolate O-atoms, two imine N-atoms and two –CH2OH units and display a distorted octahedral coordination geometry around the CoII centres. The central DyIII ion shows a square-antiprismatic DyO8 geometry. DC magnetic measurements show ferromagnetic interactions at low temperatures and AC measurements show frequency dependency even at 0 applied field but no full peaks were noticed. The tetranuclear heterometallic 3d–4f compound [Ni2Dy2(μ3-OH)2(OH)(OAc)4(HL)2(MeOH)3] (ClO4) (72) has been prepared by Liu et al. using the ditopic Schiff-base type ligand 2-(benzothiazol-2-ylhydrazonomethyl)-6-methoxyphenol (H2L).105 The cubane-like core [Dy2Ni2O4] consists of two distorted octahedral NiII centres and two eight coordinated DyIII centres which were held together by four μ3-O atoms from OH− groups or Schiff-base ligand. AC magnetic susceptibility measurements disclose a frequency-dependent out-of-phase signal and the relaxation follows a thermally activated mechanism above 2.3 K with a Ueff of 7.6 K and τ0 of 7.5 × 10−6 s. Another tetranuclear heterometallic complex [CoII2(L)2(PhCOO)2DyIII2(hfac)4] (73) (H2L = N,N′-dimethyl-N,N′-bis(2-hydroxy-3,5-dimethylbenzyl)ethylenediamine) was recently reported by Chaudhury et al. where CoII and DyIII centres are arranged alternately at the corners of a rectangular plane of the {Co2Dy2O4(CO2)2} core.106 The coordination environment of the CoII centres is described as distorted octahedral and DyIII centres as in a bicapped pseudotrigonal prismatic geometry. The AC susceptibility measurements show a slow magnetic relaxation below 6 K, with Ueff = 8.8 K (τ0 = 2.0 × 10−7 s) under zero field and Ueff = 7.8 K (τ0 = 3.9 × 10−7 s) at 0.1 T. Organic macrocycles with discrete metal ion binding pockets are also explored in developing 4f and 3d–4f based SMMs.107 Brooker and co-workers108 reported a new hexaimine [3 + 3] macrocycle, [(LPr)6−, Pr = propyl)] which was complexed in situ to generate the tetrametallic complex [Zn3Dy(LPr)(NO3)3(MeOH)3]·4H2O (74). The fit of the out-of-phase AC susceptibility data at an enhanced DC field of 1500 Oe gave a Ueff of 25.8 K between 4 K and 5.5 K temperature regions. Another beautiful example is the octanuclear complex [Cr4Dy4(μ3-OH)4(μ-N3)4(mdea)4(piv)4]·3CH2Cl2 (75, Fig. 18) (H2mdea = methyldiethanolamine and Hpiv = pivalic acid),109 where each pair of adjacent Dy centres was connected by a μ3-OH− to a CrIII, and the four CrIII centres are placed alternately above and below the Dy4 square. The complex exhibits slow relaxation of magnetization below 5 K with an energy gap of 15 K and τ0 = 1.9 × 10−7 s. Another interesting 3d–4f based high-nuclearity heterometallic MnIII/II–LnIII complex, [MnIII9MnII2Gd2(O)8(OH)2(piv)10.6(fca)6.4(NO3)2(H2O)]·13CH3CN·H2O (76) (Hfca = 2-furan-carboxylic acid), was reported by Powell and co-authors.110 The shell of the bell-shaped complex is formed by the MnIII and MnII centres, whereas the LnIII centres served as the bell's clapper. One MnIII ion is placed at the apex of the bell, other four Mn centres at the shoulder and the remaining six MnIII centres make the rim of the bell. The complex represents the first mixed-valence MnII,III–LnIII 3d–4f complex which shows SMM behaviour with a Ueff of 18.4 K above 0.5 K. Recently a similar study of MnIII,IV–LnIII based complex [Mn21DyO20(OH)2(ButCO2)20(HCO2)4(NO3)3-(H2O)7] (77) was reported by Christou et al.111 The complex shows a record Ueff of 74 K. The largest Fe–Ln cluster, [Fe12Sm4(μ4-O)6(μ3-O)4(μ3-OH)4(PhCO2)24] (78), was reported by Gao et al. in 2010.112 In the complex four FeIII centres are connected by two μ3-OH− and two μ4-O2− and form a [Fe4O2(OH)2] cubane unit. Frequency dependent AC magnetic susceptibility measurements disclose the SMM behaviour. Winpenny and co-authors reported the first phosphonate based 3d–4f cluster (H3O)[Cu24Dy8(Ph3CPO3)6(PhCPO3H)6(OAc)12(HOAc)6(OH)42(NO3)(H2O)6] (79),113 where eight DyIII centres are placed at the vertices of a cube with twelve CuII centres inside and twelve CuII centres outside the cube. The AC susceptibility measurements show slow relaxation of magnetization and the effective energy barrier of 4.6 K (τ0 = 2.1 × 10−8 s) was obtained.
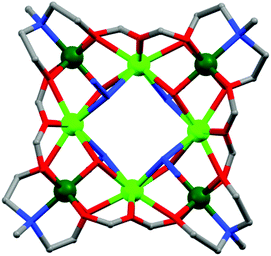 |
| Fig. 18 Molecular structure of 75. Colour code: green – Dy, dark green – Cr, grey – C, red – O, blue – N. | |
B(II) (d) 3d–4d/3d–5d and 4d–4f based SMMs.
A comparatively less explored but efficient approach to increase the energy barrier for magnetization reversal is to incorporate metal ions with first-order orbital angular momentum i.e. the 4d and 5d ions.114 In 4d and 5d ions, the spin–orbit interaction is larger in magnitude than for 3d ions, and that results in the ground doublet being more isolated from the excited levels. Compared to the 3d–4f and 4f–4f counterparts, 4d and 5d ions favour stronger magnetic exchange due to their highly anisotropic nature (Ising or quasi-Ising) with more diffuse d-orbitals. These parameters are responsible for the significantly increased magnetisation energy reversal barrier which is desired for an SMM. An important 3d–5d based complex, [(PY5Me2)4Mn4Re(CN)7](PF6)5·6H2O (80, Fig. 19) (PY5Me2 = 2,6-bis(1,1-bis(2-pyridyl)ethyl)pyridine), was reported by Long et al.115 It was the first report of a heptacyanometalate that involved a star-shaped cluster and was comprised of a central [Re(CN)7]4− anionic unit connected to four surrounding [(PY5Me2)Mn]2+ cationic units through C
N bridges. Coordination geometry of the ReIII centre is nearly ideal pentagonal bipyramid and the four MnII cationic units are arranged in a slightly distorted square with two of the MnII ions binding axial cyanide ligands and the other two binding equatorial cyanide ligands. DC magnetic susceptibility studies showed ferromagnetic interaction between the central S = 1/2 [Re(CN)7]3− complex and the surrounding four S = 5/2 MnII centres. AC susceptibility data show distinct maxima and frequency dependency in the χ′′Mvs T plot and confirm SMM behaviour of the complex. Fitting of the data according to the Arrhenius expression gave a Ueff value of 47.5 K and τ0 = 2.4 × 10−8 s. Another recent example of a 3d–5d heterometallic complex, (NEt4)[Mn2(5-Brsalen)2(MeOH)2Os(CN)6] (81, Fig. 20), was reported by Pederson et al. It represents the first SMM to incorporate the [Os(CN)6]3− moiety.116 The complex has a trimeric, cyanide bridged MnIII–OsIII–MnIII skeleton in which six coordinated MnIII centres are axially bonded to the OsIII centre via cyanide ligands. SMM behaviour of this complex was investigated using terahertz electron paramagnetic resonance (THz-EPR), inelastic neutron scattering (INS) and SQUID measurements which revealed a three-axis (Jxx, Jyy, and Jzz) anisotropic magnetic exchange coupling between MnIII and OsIII ions. Jxx [−18(2) cm−1] and Jzz [−33(2) cm−1] are found to be antiferromagnetic while Jyy [−35(2) cm−1] is ferromagnetic. The relatively high value of the exchange interactions was attributed to the more diffuse nature of the 5d orbitals of OsIII and that result in a larger overlap with the cyanide ligand bridges. The unsaturated nature of the M(H) curves for 81 reveals the presence of strong anisotropy and/or low-lying excited states. Arrhenius fitting of the AC data results in a Ueff value of 20 K. The first 3d–4d based complex K[(Me3tacn)6MnMo6(CN)18](ClO4)3 (Me3tacn = N,N′,N′′-trimethyl-1,4,7-triazacyclononane) (82) that exhibits SMM behaviour was reported by Long et al. in 2002.117 The complex contains six fac-[(Me3tacn)Mo(CN)3] units surrounding a central MnII ion and each of the units coordinated only through one cyanide ligand to the central MnII ion leading to an approximately trigonal prismatic geometry. DC magnetic susceptibility data suggest antiferromagnetic interaction (J = −6.7 cm−1) between the MnII and MoIII ions, giving rise to an S = 13/2 ground state and fitting of the magnetization data revealed an Ising-type magnetic anisotropy with zero-field splitting parameter (D) = −0.33 cm−1. AC susceptibility measurements show frequency dependent χ′′M signals at the lower temperature region and also distinct maxima at higher frequency values, proving SMM behaviour of the complex. Fitting of the data according to the Arrhenius relation yielded Ueff = 14.3 K and τ0 = 7 × 10−7 s. Chibotaru, Murray and co-workers have recently reported the first 4d–4f based complex [RuIII2DyIII2(OMe)2(O2CPh)4-(mdea)2(NO3)2] (mdeaH2 = N-methyldiethanolamine) (83),118 which displays a tetrametallic butterfly type core where two DyIII ions occupied the body sites and two RuIII ions occupied the outer wing positions. The RuIII and DyIII ions are bridged via two μ3-methoxide ligands as well as by four μ-syn–syn benzoate ligands. The RuIII ions are six coordinated with distorted octahedral geometries and the DyIII ions are eight-coordinated with distorted square-antiprismatic geometries. DC magnetic susceptibility studies show a decrease in χMT value at the higher temperature range, due to the depopulation of the excited mJ states of the DyIII ions, and an increase in χMT value at lower temperatures which indicate appreciable exchange interactions between the RuIII and the DyIII ions. DFT studies show good agreement between the calculated and experimental data. AC susceptibility measurements showed prominent frequency and temperature dependency of both χ′M and χ′′M components signifying SMM behaviour of the complex. Fitting of the data according to the Arrhenius equation yielded a Ueff value of 16 K (τ0 = 4.1 × 10−6 s) which is in excellent parity with the value determined from the ab initio calculations (19.8 K). However, the obtained anisotropic energy barrier value was found to be much lower compared to the analogous isostructural 3d–4f {CrIIIDyIII} complex. This is due to weaker Dy⋯Ru exchange interaction compared to Dy⋯Cr interaction and also due to a lower spin value (S = 1/2) of Ru than Cr (S = 3/2) which led to lesser levels in the blocking barrier.
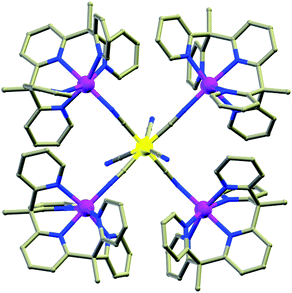 |
| Fig. 19 Molecular structure of 80. Color code: purple – Mn, yellow – Re, grey – C, red – O, blue – N. | |
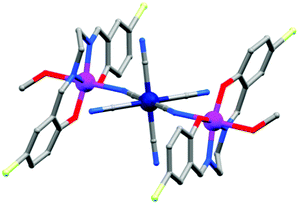 |
| Fig. 20 Molecular structure of 81. Color code: purple – Mn, navy blue – Os, grey – C, red – O, blue – N, faint green – Br. | |
B(II) (e) 3d–5f based SMMs.
Heterobimetallic uranium complexes, particularly 3d–5f system, are promising candidates as SMMs because of higher covalency of uranium as compared to lanthanide ions and this enables stronger magnetic communication between the metal centres.119a A very interesting cation-promoted self-assembled mixed-metal uranium cluster, [{UO2(salen)}2Mn(Py)3]6 (84, Fig. 21),119b was reported by Mazzanti et al. in 2012. The wheel-shaped centrosymmetric hexameric complex represents the first example of a UV cluster that is stable with respect to a disproportionation reaction and also the first to contain UO2+–MnII cation–cation interactions. The shortest intermetallic distance of 8–10 Å ruled out the possibility of any intermolecular magnetic interaction. From AC susceptibility measurements the frequency dependency of χ′′M data is observed which clearly indicates the occurrence of slow magnetic relaxation or SMM behaviour. The relaxation data fitting corresponds to a thermally activated regime, and Ueff ≈ 142 K and τ0 ≈ 3 × 10−12 s are obtained. The appreciably small value of the relaxation time was attributed to active orbital degrees of freedom that can affect the magnetoelastic interaction. Recently Mazzanti and co-authors reported two isostructural trimeric heterodimetallic 3d–5f complexes, [{[M(TPA)I][UO2(Mesaldien)][M(TPA)I]}I] (M = Mn and Cd) (85)119c [Mesaldien = N,N′-(2-aminomethyl)diethylenebis(salicylidene imine) and TPA = tris(2-pyridylmethyl)amine)], which are unique examples to contain only one UO2+ moiety. Structure analysis revealed that the complex is composed of two [M(TPA)I]+ cations bound to two oxo groups of the [UO2(Mesaldien)]− anion in a linear fashion by cation–cation interaction. The UV ion is heptacoordinated and the MnII centres are hexacoordinated. Both χ′M and χ′′M components of the AC susceptibility data show strong frequency dependence indicating SMM behaviour. For the Mn–U complex, fitting of the magnetic relaxation data affords Ueff ≈ 81 K and τ0 = 5.02 × 10−10 s. This Ueff value is appreciably larger compared to other reported uranium-based SMMs.
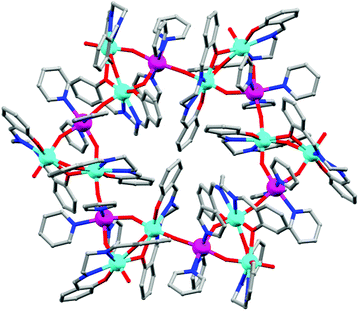 |
| Fig. 21 Molecular structure of 84. Color code: purple – Mn, cyan – U, grey – C, red – O, blue – N. | |
5. Conclusions
This review discusses fundamentals of molecular magnets and can be used as a small handbook. The field of nanomagnets is growing rapidly and many more compounds with fascinating magnetic behaviours are expected to come up in future years. Substantial attempts are needed to increase the effective energy barrier and the blocking temperature in the molecules. For that, a synthetic strategy is very crucial since it can provide a suitable chemical environment to trap anisotropic ions and also alter the coordinating groups within the ligands to favour certain electronic states for the ions that can influence the magnetic relaxation. On the other hand, reducing the dimensionality of molecular magnets is also very important for miniaturization and therefore more efforts should be dedicated to explore ligand systems which can provide an axial crystal field to the metal ions and stabilize the Stark sublevels. High level ab initio or DFT calculations on SMMs, as performed by a few groups,120 are becoming a valuable tool for predicting the local anisotropy and elucidating the relationship between the electronic structure and relaxation processes.
Acknowledgements
S.G. acknowledges IISER Bhopal for an SRF fellowship. A.K.M. thanks UGC for an SRF fellowship. S.K. thanks the Science and Engineering Research Board (SERB), DST, Government of India (project no. EMR/2014/00053) and IISER Bhopal for generous financial and infrastructural support. The authors thank Mr Govind Prasad Agrawal for generous scientific help.
References
-
(a) R. Sessoli and A. K. Powell, Coord. Chem. Rev., 2009, 253, 2328 CrossRef CAS PubMed;
(b) L. Sorace, C. Benelli and D. Gatteschi, Chem. Soc. Rev., 2011, 40, 3092 RSC.
- T. Lis, Acta Crystallogr., Sect. B: Struct. Crystallogr. Cryst. Chem., 1980, 36, 2042 CrossRef.
- R. Sessoli, H. L. Tsai, A. R. Schake, S. Y. Wang, J. B. Vincent, K. Folting, D. Gatteschi, G. Christou and D. N. Hendrickson, J. Am. Chem. Soc., 1993, 115, 1804 CrossRef CAS.
-
D. Gatteschi, R. Sessoli and J. Villain, Molecular Nanomagnets, Oxford University Press, Oxford, 2006 Search PubMed.
- M. N. Leuenberger and D. Loss, Nature, 2001, 410, 789 CrossRef CAS PubMed.
- J. Tejada, Polyhedron, 2001, 20, 1751 CrossRef CAS.
-
(a) G. Christou, D. Gatteschi, D. N. Hendrickson and R. Sessoli, MRS Bull., 2000, 25, 66 CrossRef CAS;
(b) R. K. Zheng, H. Gu, B. Xu and X. X. Zhang, J. Phys.: Condens. Matter, 2006, 18, 5905 CrossRef CAS PubMed;
(c) S. K. Langley, D. P. Wielechowski, V. Vieru, N. F. Chilton, B. Moubaraki, B. F. Abrahams, L. F. Chibotaru and K. S. Murray, Angew. Chem., Int. Ed., 2013, 52, 12014 CrossRef CAS PubMed.
-
(a) D. Gatteschi and R. Sessoli, Angew. Chem., Int. Ed., 2003, 42, 268 CrossRef CAS PubMed;
(b) L. Thomas, L. Lionti, R. Ballou, D. Gatteschi, R. Sessoli and B. Barbara, Nature, 1996, 383, 145 CrossRef CAS PubMed;
(c) S. Maheswaran, G. Chastanet, S. J. Teat, T. Mallah, R. Sessoli, W. Wernsdorfer and R. E. P. Winpenny, Angew. Chem., 2005, 117, 5172 (
Angew. Chem., Int. Ed.
, 2005
, 44
, 5044
) CrossRef PubMed.
- J. D. Rinehart and J. R. Long, Chem. Sci., 2011, 2, 2078 RSC.
-
(a) R. Sessoli, D. Gatteschi, A. Caneschi and M. A. Novak, Nature, 1993, 365, 141 CrossRef CAS PubMed;
(b) K. S. Pedersen, J. Bendix and R. Clérac, Chem. Commun., 2014, 50, 4396 RSC.
- Q. Zhou, F. Yang, D. Liu, Y. Peng, G. Li, Z. Shi and S. Feng, Inorg. Chem., 2012, 51, 7529 CrossRef CAS PubMed.
- M. T. Gamer, Y. Lan, P. W. Roesky, A. K. Powell and R. Clérac, Inorg. Chem., 2008, 47, 6581 CrossRef CAS PubMed.
- S. A. Sulway, R. A. Layfield, F. Tuna, W. Wernsdorfer and R. E. P. Winpenny, Chem. Commun., 2012, 48, 1508 RSC.
- F. Tuna, C. A. Smith, M. Bodensteiner, L. Ungur, L. F. Chibotaru, E. J. L. McInnes, R. E. P. Winpenny, D. Collison and R. A. Layfield, Angew. Chem., Int. Ed., 2012, 51, 6976 CrossRef CAS PubMed.
-
(a) D.-F. Weng, Z.-M. Wang and S. Gao, Chem. Soc. Rev., 2011, 40, 3157 RSC;
(b) M. Ohba and H. O. Kawa, Coord. Chem. Rev., 2000, 198, 313 CrossRef CAS;
(c) J.-N. Rebilly and T. Mallah, Struct. Bonding, 2006, 122, 103 CrossRef CAS.
- Y.-Z. Zhang, B.-W. Wang, O. Sato and S. Gao, Chem. Commun., 2010, 46, 6959 RSC.
- J. D. Rinehart;, M. Fang;, W. J. Evans; and J. R. Long, J. Am. Chem. Soc., 2011, 133, 14236 CrossRef PubMed.
-
(a) J. D. Rinehart, M. Fang, W. J. Evans and J. R. Long, J. Am. Chem. Soc., 2011, 133, 14236 CrossRef CAS PubMed;
(b) J. D. Rinehart, M. Fang, W. J. Evans and J. R. Long, Nat. Chem., 2011, 3, 538 CrossRef CAS PubMed;
(c) V. Tangoulis, D. Panagoulis, C. P. Raptopoulou and C. D. Samara, Dalton Trans., 2008, 1752 RSC;
(d) K. C. Mondal, O. Sengupta, M. Nethaji and P. S. Mukherjee, Dalton Trans., 2008, 767 RSC;
(e) P. L. Feng, C. J. Stephenson, A. Amjad, G. Ogawa, E. Barco and D. N. Hendrickson, Inorg. Chem., 2010, 49, 1304 CrossRef CAS PubMed;
(f) T.-F. Liu, D. Fu, S. Gao, Y.-Z. Zhang, H.-L. Sun, G. Su and Y.-J. Liu, J. Am. Chem. Soc., 2003, 125, 13976 CrossRef CAS PubMed;
(g) S. Mukherjee and P. S. Mukherjee, Acc. Chem. Res., 2013, 46, 2556 CrossRef CAS PubMed.
- J. Ribas, A. Escuer, M. Monfort, R. Vicente, R. Cortés, L. Lezama and T. Rojo, Coord. Chem. Rev., 1999, 193–195, 1027 CrossRef CAS.
- X.-C. Huang, C. Zhou, H.-Y. Wei and X.-Y. Wang, Inorg. Chem., 2013, 52, 7314 CrossRef CAS PubMed.
- Y.-Z. Zhang, W. Wernsdorfer, F. Pan, Z.-M. Wanga and S. Gao, Chem. Commun., 2006, 3302 RSC.
- P. L. Feng, C. J. Stephenson, A. Amjad, G. Ogawa, E. del Barco and D. N. Hendrickson, Inorg. Chem., 2010, 49, 1304 CrossRef CAS PubMed.
- D. I. Alexandropoulos, C. Papatriantafyllopoulou, C. Li, L. Cunha-Silva, M. J. Manos, A. J. Tasiopoulos, W. Wernsdorfer, G. Christou and T. C. Stamatatos, Eur. J. Inorg. Chem., 2013, 2286 CrossRef CAS PubMed.
- S.-Y. Lin, L. Zhao, Y.-N. Guo, P. Zhang, Y. Guo and J. Tang, Inorg. Chem., 2012, 51, 10522 CrossRef CAS PubMed.
-
(a) Y.-Z. Zheng, Z. Zheng and X.-M. Chen, Coord. Chem. Rev., 2014, 258, 1 CrossRef PubMed;
(b) S.-J. Liu, C.-C. Xie, J.-M. Jia, J.-P. Zhao, S.-D. Han, Y. Cui, Y. Li and X.-H. Bu, Chem. – Asian J., 2014, 9, 1116 CrossRef CAS PubMed;
(c) M.-H. Zeng, Y.-L. Zhou, M.-C. Wu, H.-L. Sun and M. Du, Inorg. Chem., 2010, 49, 6436 CrossRef CAS PubMed;
(d) H. Bußkamp, G. B. Deacon, M. Hilder, P. C. Junk, U. H. Kynast, W. W. Lee and D. R. Turner, CrystEngComm, 2007, 9, 394 RSC;
(e) H. Arora and R. Mukherjee, New J. Chem., 2010, 34, 2357 RSC.
- L. A. Kushch, V. D. Sasnovskaya, A. I. Dmitriev, E. B. Yagubskii, O. V. Koplak, L. V. Zorina and D. W. Boukhvalov, Dalton Trans., 2012, 41, 13747 RSC.
- J.-B. Peng, Y.-P. Ren, X.-J. Kong, L.-S. Long, R.-B. Huang and L.-S. Zheng, CrystEngComm, 2011, 13, 2084 RSC.
-
(a) O. Roubeau and R. Clérac, Eur. J. Inorg. Chem., 2008, 4325 CrossRef CAS PubMed;
(b) J. W. Sharples and D. Collison, Coord. Chem. Rev., 2014, 260, 1 CrossRef CAS PubMed.
- D.-P. Liu, J.-B. Peng, X.-P. Lin, Q. Huang, X.-J. Kong, L.-S. Long, R.-B. Huang and L.-S. Zheng, CrystEngComm, 2014, 16, 5527 RSC.
- L. F. Jones, D. M. Low, M. Helliwell, J. Raftery, D. Collison, G. Aromí, J. Cano, T. Mallah, W. Wernsdorfer, E. K. Brechin and E. J. L. Mcinnes, Polyhedron, 2006, 25, 325 CrossRef CAS PubMed.
- A. Saha, M. Thompson, K. A. Abboud, W. Wernsdorfer and G. Christou, Inorg. Chem., 2011, 50, 10476 CrossRef CAS PubMed.
- V. Chandrasekhar, P. Bag, M. Speldrich, J. van Leusen and P. Kögerler, Inorg. Chem., 2013, 52, 5035 CrossRef CAS PubMed.
- V. Tangoulis, M. Skarlis, C. P. Raptopoulou, V. Psycharis and C. Dendrinou-Samara, Eur. J. Inorg. Chem., 2014, 2678 CrossRef CAS PubMed.
- S. K. Langley, C. Le, L. Ungur, B. Moubaraki, B. F. Abrahams, L. F. Chibotaru and K. S. Murray, Inorg. Chem., 2015, 54, 3631 CrossRef CAS PubMed.
-
(a) V. Chandrasekhar, T. Senapati, A. Dey and S. Hossain, Dalton Trans., 2011, 40, 5394 RSC;
(b) T. Zheng, S.-S. Bao, M. Ren and L.-M. Zheng, Dalton Trans., 2013, 42, 16396 RSC;
(c) L.-X. Xie and D.-G. Ding, Inorg. Chem. Commun., 2009, 12, 552 CrossRef CAS PubMed.
- S. J. Langley, M. Helliwell, R. Sessoli, P. Rosa, W. Wernsdorfer and R. E. P. Winpenny, Chem. Commun., 2005, 5029 RSC.
- M. Shanmugam, G. Chastanet, T. Mallah, R. Sessoli, S. J. Teat, G. A. Timco and R. E. P. Winpenny, Chem. – Eur. J., 2006, 12, 8777 CrossRef CAS PubMed.
- V. Baskar, K. Gopal, M. Helliwell, F. Tuna, W. Wernsdorfer and R. E. P. Winpenny, Dalton Trans., 2010, 39, 4747 RSC.
- V. Chandrasekhar, J. Goura, K. Gopal, J. Liu and P. Goddard, Polyhedron, 2014, 72, 35 CrossRef CAS PubMed.
- H. Andres, R. Basler, A. J. Blake, C. Cadiou, G. Chaboussant, C. M. Grant, H.-U. Güdel, M. Murrie, S. Parsons, C. Paulsen, F. Semadini, V. Villar, W. Wernsdorfer and R. E. P. Winpenny, Chem. – Eur. J., 2002, 8, 4867 CrossRef CAS.
- Y.-S. Ma, Y. Song, X.-Y. Tang and R.-X. Yuan, Dalton Trans., 2010, 39, 6262 RSC.
-
(a) P. Chaudhuri, Coord. Chem. Rev., 2003, 243, 143 CrossRef CAS;
(b) C. J. Milios, T. C. Stamatatos and S. P. Perlepes, Polyhedron, 2006, 25, 134 CrossRef CAS PubMed.
- C. Papatriantafyllopoulou, T. C. Stamatatos, C. G. Efthymiou, L. Cunha-Silva, F. A. A. Paz, S. P. Perlepes and G. Christou, Inorg. Chem., 2010, 49, 9743 CrossRef CAS PubMed.
- I. J. Hewitt, Y. Lan, C. E. Anson, J. Luzon, R. Sessoli and A. K. Powell, Chem. Commun., 2009, 6765 RSC.
-
(a) T. C. Stamatatos, B. S. Luisi, B. Moulton and G. Christou, Inorg. Chem., 2008, 47, 1134 CrossRef CAS PubMed;
(b) I.-R. Jeon and R. Clérac, Dalton Trans., 2012, 41, 9569 RSC;
(c) T. C. Stamatatos, K. A. Abboud, S. P. Perlepes and G. Christou, Dalton Trans., 2007, 3861 RSC;
(d) J. M. Lillo, N. Dolan and E. K. Brechin, Dalton Trans., 2014, 43, 4408 RSC;
(e) M. Hołyńska, N. Frank, C. Pichon, I.-R. Jeon, R. Clérac and S. Dehnen, Inorg. Chem., 2013, 52, 7317 CrossRef PubMed;
(f) C.-I. Yang, Z.-Z. Zhang and S.-B. Lin, Coord. Chem. Rev., 2015, 289–290, 289 CrossRef CAS PubMed;
(g) R. Inglis, J. Bendix, T. B. Nannestad, H. Weihe, E. K. Brechin and S. Piligkos, Chem. Sci., 2010, 1, 631 RSC;
(h) C. J. Milios, A. Vinslava, P. A. Wood, S. Parsons, W. Wernsdorfer, G. Christou, S. P. Perlepes and E. K. Brechin, J. Am. Chem. Soc., 2007, 129, 8 CrossRef CAS PubMed.
- C. J. Milios, A. Vinslava, W. Wernsdorfer, S. Moggach, S. Parsons, S. P. Perlepes, G. Christou and E. K. Brechin, J. Am. Chem. Soc., 2007, 129, 2754 CrossRef CAS PubMed.
- D. I. Alexandropoulos, C. Papatriantafyllopoulou, G. Aromí, O. Roubeau, S. J. Teat, S. P. Perlepes, G. Christou and T. C. Stamatatos, Inorg. Chem., 2010, 49, 3962 CrossRef CAS PubMed.
-
(a) T. Kajiwara, M. Nakano, S. Takaishi and M. Yamashita, Inorg. Chem., 2008, 47, 8604 CrossRef CAS PubMed;
(b) T. Kajiwara, K. Takahashi, T. Hiraizumi, S. Takaisi and M. Yamashita, CrystEngComm, 2009, 11, 2110 RSC;
(c) J. P. Costes, J. Garcia-Tojal, J. P. Tuchagues and L. Vendier, Eur. J. Inorg. Chem., 2009, 3801 CrossRef CAS PubMed;
(d) J. P. Costes and L. Vendier, Eur. J. Inorg. Chem., 2010, 2768 CrossRef CAS PubMed;
(e) E. Colacio, J. Ruiz-Sanchez, F. J. White and E. K. Brechin, Inorg. Chem., 2011, 50, 7268 CrossRef CAS PubMed;
(f) M. Andruh, J. P. Costes, C. Diaz and S. Gao, Inorg. Chem., 2009, 48, 3342 CrossRef CAS PubMed.
- Z. Lü, M. Yuan, F. Pan, S. Gao, D. Zhang and D. Zhu, Inorg. Chem., 2006, 45, 3538 CrossRef PubMed.
- Q. Wu, Y.-G. Li, Y.-H. Wang, R. Clérac, Y. Lua and E.-B. Wang, Chem. Commun., 2009, 5743 RSC.
- H. Wang, C. Liu, T. Liu, S. Zeng, W. Cao, Q. Ma, C. Duan, J. Dou and J. Jiang, Dalton Trans., 2013, 42, 15355 RSC.
- C. Das, A. Upadhyay, S. Vaidya, S. K. Singh, G. Rajaraman and M. Shanmugam, Chem. Commun., 2015, 51, 6137 RSC.
-
(a) L. N. Dawe, K. V. Shuvaev and L. K. Thompson, Inorg. Chem., 2009, 48, 3323 CrossRef CAS PubMed;
(b) A. Adhikary, H. S. Jena, S. Khatua and S. Konar, Chem. – Asian J., 2014, 9, 1083 CrossRef CAS PubMed;
(c) A. Adhikary, S. Goswami, J. A. Sheikh and S. Konar, Eur. J. Inorg. Chem., 2014, 963 CrossRef CAS PubMed;
(d) H. Tian, L. Zhao, Y.-N. Guo, Y. Guo, J. Tang and Z. Liu, Chem. Commun., 2012, 48, 708 RSC.
- Y.-N. Guo, G.-F. Xu, P. Gamez, L. Zhao, S.-Y. Lin, R. Deng, J. Tang and H.-J. Zhang, J. Am. Chem. Soc., 2010, 132, 8538 CrossRef CAS PubMed.
- M. U. Anwar, L. K. Thompson, L. N. Dawe, F. Habibb and M. Murugesu, Chem. Commun., 2012, 48, 4576 RSC.
- A. Adhikary, J. A. Sheikh, S. Biswas and S. Konar, Dalton Trans., 2014, 43, 9334 RSC.
-
(a) P. Zhang, Y.-N. Guo and J. Tang, Coord. Chem. Rev., 2013, 257, 1728 CrossRef CAS PubMed;
(b) N. F. Chilton, Inorg. Chem., 2015, 54, 2097 CrossRef CAS PubMed;
(c) R. J. Blagg, L. Ungur, F. Tuna, J. Speak, P. Comar, D. Collison, W. Wernsdorfer, E. J. L. McInnes, L. F. Chibotaru and R. E. P. Winpenny, Nat. Chem., 2013, 5, 673 CrossRef CAS PubMed;
(d) J.-L. Liu, Y.-C. Chen, Y.-Z. Zheng, W.-Q. Lin, L. Ungur, W. Wernsdorfer, L. F. Chibotaru and M.-L. Tong, Chem. Sci., 2013, 4, 3310 RSC.
-
(a) S. Sakaue, A. Fuyuhiro, T. Fukuda and N. Ishikawa, Chem. Commun., 2012, 48, 5337 RSC;
(b) D. Tanaka, T. Inose, H. Tanaka, S. Lee, N. Ishikawa and T. Ogawa, Chem. Commun., 2012, 48, 7796 RSC;
(c) T. Fukuda, K. Matsumura and N. Ishikawa, J. Phys. Chem. A, 2013, 117, 10447 CrossRef CAS PubMed.
- N. Ishikawa, M. Sugita, T. Ishikawa, S.-y. Koshihara and Y. Kaizu, J. Am. Chem. Soc., 2003, 125, 8694 CrossRef CAS PubMed.
-
(a) J. J. Baldoví, S. C. Serra, J. M. C. Juan, E. Coronado, A. G. Ariño and A. Palii, Inorg. Chem., 2012, 51, 12565 CrossRef PubMed;
(b) A. Müller and F. Peters, Chem. Rev., 1998, 98, 239 CrossRef PubMed.
- M. A. AlDamen, J. J. Clemente, E. Coronado, C. M. Gastaldo and A. G. Ariño, J. Am. Chem. Soc., 2008, 130, 8874 CrossRef CAS PubMed.
- P.-E. Car, M. Perfetti, M. Mannini, A. Favre, A. Caneschi and R. Sessoli, Chem. Commun., 2011, 47, 3751 RSC.
- S.-D. Jiang, B.-W. Wang, G. Su, Z.-M. Wang and S. Gao, Angew. Chem., Int. Ed., 2010, 49, 7448 CrossRef CAS PubMed.
- S.-D. Jiang, B.-W. Wang, H.-L. Sun, Z.-M. Wang and S. Gao, J. Am. Chem. Soc., 2011, 133, 4730 CrossRef CAS PubMed.
- A. K. Mondal, S. Goswami and S. Konar, Dalton Trans., 2015, 44, 5086 RSC.
-
(a) L. J. Batchelor, I. Cimatti, R. Guillot, F. Tuna, W. Wernsdorfer, L. Ungur, L. F. Chibotaru, V. E. Campbell and T. Mallah, Dalton Trans., 2014, 43, 12146 RSC;
(b) V. E. Campbell, H. Bolvin, E. Rivière, R. Guillot, W. Wernsdorfer and T. Mallah, Inorg. Chem., 2014, 53, 2598 CrossRef CAS PubMed.
- D. E. Freedman, W. H. Harman, T. D. Harris, G. J. Long, C. J. Chang and J. R. Long, J. Am. Chem. Soc., 2010, 132, 1224 CrossRef CAS PubMed.
-
(a) W. H. Harman, T. D. Harris, D. E. Freedman, H. Fong, A. Chang, J. D. Rinehart, A. Ozarowski, M. T. Sougrati, F. Grandjean, G. J. Long, J. R. Long and C. J. Chang, J. Am. Chem. Soc., 2010, 132, 18115 CrossRef CAS PubMed;
(b) D. Weismann, Y. Sun, Y. Lan, G. Wolmershäuser, A. K. Powell and H. Sitzmann, Chem. – Eur. J., 2011, 17, 4700 CrossRef CAS PubMed;
(c) P. H. Lin, N. C. Smythe, S. I. Gorelsky, S. Maguire, N. J. Henson, I. Korobkov, B. L. Scott, J. C. Gordon, R. T. Baker and M. Murugesu, J. Am. Chem. Soc., 2011, 133, 15806 CrossRef CAS PubMed;
(d) S. Mossin, B. L. Tran, D. Adhikari, M. Pink, F. M. Heinemann, J. Sutter, R. K. Szilagyi, K. Meyer and D. J. Mindiola, J. Am. Chem. Soc., 2012, 134, 13651 CrossRef CAS PubMed;
(e) J. M. Zadrozny, M. Atanasov, A. M. Bryan, C. Y. Lin, B. D. Rekken, P. P. Power, F. Neese and J. R. Long, Chem. Sci., 2013, 4, 125 RSC;
(f) T. Jurca, A. Farghal, P. H. Lin, I. Korobkov, M. Murugesu and D. S. Richardson, J. Am. Chem. Soc., 2011, 133, 15814 CrossRef CAS PubMed;
(g) J. M. Zadrozny and J. R. Long, J. Am. Chem. Soc., 2011, 133, 20732 CrossRef CAS PubMed;
(h) J. M. Zadrozny, J. Liu, N. A. Piro, C. J. Chang, S. Hill and J. R. Long, Chem. Commun., 2012, 48, 3927 RSC;
(i) J. Vallejo, I. Castro, R. R. García, J. Cano, M. Julve, F. Lloret, G. D. Munno, W. Wernsdorfer and E. Pardo, J. Am. Chem. Soc., 2012, 134, 15704 CrossRef CAS PubMed;
(j) Y. Y. Zhu, C. Cui, Y. Q. Zhang, J. H. Jia, X. Guo, C. Gao, K. Qian, S. D. Jiang, B. W. Wang, Z. M. Wang and S. Gao, Chem. Sci., 2013, 4, 1802 RSC;
(k) G. A. Craig and M. Murrie, Chem. Soc. Rev., 2015, 44, 2135 RSC.
- R. Ruamps, R. Maurice, L. Batchelor, M. Boggio-Pasqua, R. Guillot, A. L. Barra, J. J. Liu, E. Bendeif, S. Pillet, S. Hill, T. Mallah and N. Guihery, J. Am. Chem. Soc., 2013, 135, 3017 CrossRef CAS PubMed.
- S. Gómez-Coca, E. Cremades, N. Aliaga-Alcalde and E. Ruiz, Inorg. Chem., 2014, 53, 676 CrossRef PubMed.
-
(a) J. D. Rinehart and J. R. Long, Chem. Sci., 2011, 2, 2078 RSC;
(b) P. L. Arnold, G. M. Jones, S. O. Odoh, G. Schreckenbach, N. Magnani and J. B. Love, Nat. Chem., 2012, 4, 221 CrossRef CAS PubMed;
(c) J. J. Baldoví, S. Cardona-Serra, J. M. Clemente-Juan, E. Coronado and A. Gaita-Ariño, Chem. Sci., 2013, 4, 938 RSC.
-
(a) D. M. King, F. Tuna, J. McMaster, W. Lewis, A. J. Blake, E. J. L. McInnes and S. T. Liddle, Angew. Chem., Int. Ed., 2013, 52, 4921 CrossRef CAS PubMed;
(b) D. M. King, F. Tuna, E. J. L. McInnes, J. McMaster, W. Lewis, A. J. Blake and S. T. Liddle, Science, 2012, 337, 717 CrossRef CAS PubMed.
- G. Aromí and E. K. Brechin, Struct. Bonding, 2006, 122, 1 CrossRef.
-
(a) R. H. Laye, M. Murrie, S. Ochsenbein, A. R. Bell, S. J. Teat, J. Raftery, H.-U. Güdel and E. J. L. McInnes, Chem. – Eur. J., 2003, 9, 6215 CrossRef CAS PubMed;
(b) P. L. W. T. Piggott, H. Weihe, J. Bendix, A.-L. Barra and H. U. Gudel, Inorg. Chem., 1999, 38, 5928 CrossRef;
(c) A. Müller, R. Sessoli, E. Krickemeyer, H. Bögge, J. Meyer, D. Gatteschi, L. Pardi, J. Westphal, K. Hovemeier, R. Rohlfing, J. Döring, F. Hellweg, C. Beugholt and M. Schmidtmann, Inorg. Chem., 1997, 36, 5239 CrossRef;
(d) P. Knopp, K. Wieghardt, B. Nuber, J. Weiss and W. S. Sheldrick, Inorg. Chem., 1990, 29, 363 CrossRef CAS;
(e) H. Kumagai and S. Kitagawa, Chem. Lett., 1996, 471 CrossRef CAS;
(f) Q. Chen and J. Zubieta, Coord. Chem. Rev., 1992, 114, 107 CrossRef CAS.
-
(a) A.-L. Barra, D. Gatteschi, L. Pardi, A. Müller and J. Döring, J. Am. Chem. Soc., 1992, 114, 8509 CrossRef CAS;
(b) D. Gatteschi, L. Pardi, A.-L. Barra, A. Müller and J. Döring, Nature, 1991, 354, 463 CrossRef CAS PubMed.
-
(a) S. L. Castro, Z. Sun, C. M. Grant, J. C. Bollinger, D. N. Hendrickson and G. Christou, J. Am. Chem. Soc., 1998, 120, 2365 CrossRef CAS;
(b) Z. Sun, C. M. Grant, S. L. Castro, D. N. Hendrickson and G. Christou, Chem. Commun., 1998, 721 RSC.
- N. E. Chakov, S.-C. Lee, A. G. Harter, P. L. Kuhns, A. P. Reyes, S. O. Hill, N. S. Dalal, W. Wernsdorfer, K. Abboud and G. Christou, J. Am. Chem. Soc., 2006, 128, 6975 CrossRef CAS PubMed.
- T. C. Stamatatos, V. Nastopoulos, A. J. Tasiopoulos, E. E. Moushi, W. Wernsdorfer, G. Christou and S. P. Perlepes, Inorg. Chem., 2008, 47, 10081 CrossRef CAS PubMed.
- M. Soler, W. Wernsdorfer, K. Folting, M. Pink and G. Christou, J. Am. Chem. Soc., 2004, 126, 2156 CrossRef CAS PubMed.
- A. J. Tasiopoulos, A. Vinslava, W. Wernsdorfer, K. A. Abboud and G. Christou, Angew. Chem., Int. Ed., 2004, 43, 2117 CrossRef CAS PubMed.
-
(a) E. K. Brechin, M. Soler, G. Christou, M. Helliwell, S. J. Teat and W. Wernsdorfer, Chem. Commun., 2003, 1276 RSC;
(b) M. Cavaluzzo, Q. Chen and J. Zubieta, J. Chem. Soc., Chem. Commun., 1993, 131 RSC;
(c) E. K. Brechin, M. Soler, J. Davidson, D. N. Hendrickson, S. Parsons and G. Christou, Chem. Commun., 2002, 2252 RSC;
(d)
E. K. Brechin, M. Murugesu, C. Muryn and G. Christou, unpublished results;
(e) M. Murugesu, J. Raftery, W. Wernsdorfer, G. Christou and E. K. Brechin, Inorg. Chem., 2004, 43, 4203 CrossRef CAS PubMed;
(f) E. C. Sañudo, W. Wernsdorfer, K. A. Abboud and G. Christou, Inorg. Chem., 2004, 43, 4137 CrossRef PubMed.
-
(a) A. L. Barra, A. Caneschi, A. Cornia, F. F. Biani, D. Gatteschi, C. Sangregorio, R. Sessoli and L. Sorace, J. Am. Chem. Soc., 1999, 121, 5302 CrossRef CAS;
(b) C. Benelli, J. Cano, Y. Journaux, R. Sessoli, G. A. Solan and R. E. P. Winpenny, Inorg. Chem., 2001, 40, 188 CrossRef CAS;
(c) L. F. Jones, E. K. Brechin, D. Collison, M. Helliwell, T. Mallah, S. Piligkos, G. Rajaraman and W. Wernsdorfer, Inorg. Chem., 2003, 42, 6601 CrossRef CAS PubMed;
(d) G. W. Powell, H. N. Lancashire, E. K. Brechin, D. Collison, S. L. Heath, T. Mallah and W. Wernsdorfer, Angew. Chem., Int. Ed., 2004, 43, 5772 CrossRef CAS PubMed;
(e) A. K. Boudalis, B. Donnadieu, V. Nastopoulos, J. M. C. Juan, A. Mari, Y. Sanakis, J.-P. Tuchagues and S. P. Perlepes, Angew. Chem., Int. Ed., 2004, 43, 2266 CrossRef CAS PubMed;
(f) H. Oshio, N. Hoshino and T. Ito, J. Am. Chem. Soc., 2000, 122, 12602 CrossRef CAS;
(g) J. M. Lillo, N. Dolan and E. K. Brechin, Dalton Trans., 2014, 43, 4408 RSC.
- A. L. Barra, P. Debrunner, D. Gatteschi, C. E. Schulz and R. Sessoli, Europhys. Lett., 1996, 35, 133 CrossRef CAS.
- J. C. Goodwin, R. Sessoli, D. Gatteschi, W. Wernsdorfer, A. K. Powell and S. L. Heath, J. Chem. Soc., Dalton Trans., 2000, 1835 RSC.
-
(a) M. Murrie, Chem. Soc. Rev., 2010, 39, 1986 RSC;
(b) M. Murrie, S. J. Teat, H. S. Evans and H. U. Güdel, Angew. Chem., Int. Ed., 2003, 42, 4653 CrossRef CAS PubMed;
(c) A. Ferguson, A. Parkin, J. S. Benitez, K. Kamenev, W. Wernsdorfer and M. Murrie, Chem. Commun., 2007, 3473 RSC;
(d) D. Wu, D. Guo, Y. Song, W. Huang, C. Duan, Q. Meng and O. Sato, Inorg. Chem., 2009, 48, 854 CrossRef CAS PubMed.
- E. C. Yang, D. N. Hendrickson, W. Wernsdorfer, M. Nakano, L. N. Zakharov, R. D. Sommer, A. L. Rheingold, M. L. Gairaud and G. Christou, J. Appl. Phys., 2002, 91, 7382 CrossRef CAS PubMed.
- M.-H. Zeng, M.-X. Yao, H. Liang, W.-X. Zhang and X.-M. Chen, Angew. Chem., Int. Ed., 2007, 46, 1832 CrossRef CAS PubMed.
-
(a) S. T. Ochsenbein, M. Murrie, E. Rusanov, H. S. Evans, C. Sekine and H. U. Güdel, Inorg. Chem., 2002, 41, 5133 CrossRef CAS PubMed;
(b) A. Bell, G. Aromí, S. J. Teat, W. Wernsdorfer and R. E. P. Winpenny, Chem. Commun., 2005, 2808 RSC;
(c) A. K. Boudalis, M. Pissas, C. P. Raptopoulou, V. Psycharis, B. Abarca and R. Ballesteros, Inorg. Chem., 2008, 47, 10674 CrossRef CAS PubMed.
- C. Cadiou, M. Murrie, C. Paulsen, V. Villar, W. Wernsdorfer and R. E. P. Winpenny, Chem. Commun., 2001, 2666 RSC.
- G. Aromí, S. Parsons, W. Wernsdorfer, E. K. Brechin and E. J. L. McInnes, Chem. Commun., 2005, 5038 RSC.
-
(a) E. J. Schelter, A. V. Prosvirin and K. R. Dunbar, J. Am. Chem. Soc., 2004, 126, 15004 CrossRef CAS PubMed;
(b) C. P. Berlinguette, D. Vaughn, C. C. Vilalta, J. R. G. Mascarás and K. R. Dunbar, Angew. Chem., Int. Ed., 2003, 42, 1523 CrossRef CAS PubMed;
(c) Y. Song, P. Zhang, X.-M. Ren, X.-F. Shen, Y.-Z. Li and X.-Z. You, J. Am. Chem. Soc., 2005, 127, 3708 CrossRef CAS PubMed;
(d) C.-F. Wang, J.-L. Zuo, B. M. Bartlett, Y. Song, J. R. Long and X.-Z. You, J. Am. Chem. Soc., 2006, 128, 7162 CrossRef CAS PubMed;
(e) K. Qian, X.-C. Huang, C. Zhou, X.-Z. You, X.-Y. Wang and K. R. Dunbar, J. Am. Chem. Soc., 2013, 135, 13302 CrossRef CAS PubMed.
-
(a) D. N. Woodruff, R. E. P. Winpenny and R. A. Layfield, Chem. Rev., 2013, 113, 5110 CrossRef CAS PubMed;
(b) P. Zhang, L. Zhang and J. Tang, Dalton Trans., 2015, 44, 3923 RSC;
(c) L. Ungur, S.-Y. Lin, J. Tang and L. F. Chibotaru, Chem. Soc. Rev., 2014, 43, 6894 RSC;
(d) S. Goswami, A. Adhikary, H. S. Jena and S. Konar, Dalton Trans., 2013, 42, 9813 RSC;
(e) S. Biswas, H. S. Jena, A. Adhikary and S. Konar, Inorg. Chem., 2014, 53, 3926 CrossRef CAS PubMed.
- P.-H. Lin, T. J. Burchell, R. Clérac and M. Murugesu, Angew. Chem., Int. Ed., 2008, 47, 8848 CrossRef CAS PubMed.
- Y. Guo, G. Xu, W. Wernsdorfer, L. Ungur, Y. Guo, J. Tang, H. Zhang, L. F. Chibotaru and A. K. Powell, J. Am. Chem. Soc., 2011, 133, 11948 CrossRef CAS PubMed.
- R. A. Layfield, J. W. McDouall, S. A. Sulway, F. Tuna, D. Collison and R. E. P. Winpenny, Chem. – Eur. J., 2010, 16, 4442 CrossRef CAS PubMed.
- C. S. Liu, M. Du, C. E. Sañudo, J. Echeverria, M. Hu, Q. Zhang, L. M. Zhou and S. M. Fang, Dalton Trans., 2011, 40, 9366 RSC.
- P.-H. Lin, T. J. Burchell, L. Ungur, L. F. Chibotaru, W. Wernsdorfer and M. Murugesu, Angew. Chem., Int. Ed., 2009, 48, 9489 CrossRef CAS PubMed.
- H. Tian, Y.-N. Guo, L. Zhao, J. Tang and Z. Liu, Inorg. Chem., 2011, 50, 8688 CrossRef CAS PubMed.
- J. W. Sharples, Y. Zheng, F. Tuna, E. J. L. McInnes and D. Collison, Chem. Commun., 2011, 47, 7650 RSC.
- X. Gu, R. Clérac, A. Houri and D. Xue, Inorg. Chim. Acta, 2008, 361, 3873 CrossRef CAS PubMed.
- X.-L. Li, C.-L. Chen, H.-P. Xiao, A.-L. Wang, C.-M. Liu, X. Zheng, L.-J. Gao, X.-G. Yanga and S.-M. Fang, Dalton Trans., 2013, 42, 15317 RSC.
-
(a) K. Liu, W. Shi and P. Cheng, Coord. Chem. Rev., 2015, 289–290, 74 CrossRef CAS PubMed;
(b) J.-L. Liu, J.-Y. Wu, Y.-C. Chen, V. Mereacre, A. K. Powell, L. Ungur, L. F. Chibotaru, X.-M. Chen and M.-L. Tong, Angew. Chem., Int. Ed., 2014, 53, 12966 CrossRef CAS PubMed.
- E. Colacio, J. Ruiz, A. J. Mota, M. A. Palacios, E. Cremades, E. Ruiz, F. J. Whitea and E. K. Brechin, Inorg. Chem., 2012, 51, 5857 CrossRef CAS PubMed.
- V. Chandrasekhar, S. Das, A. Dey, S. Hossain, S. Kundu and E. Colacio, Eur. J. Inorg. Chem., 2014, 23, 397 CrossRef PubMed.
- Y. Gao, L. Zhao, X. Xu, G.-F. Xu, Y.-N. Guo, J. Tang and Z. Liu, Inorg. Chem., 2011, 50, 1304 CrossRef CAS PubMed.
- S. M. T. Abtab, M. C. Majee, M. Maity, J. Titiš, R. Boča and M. Chaudhury, Inorg. Chem., 2014, 53, 1295 CrossRef CAS PubMed.
- A. Yamashita, A. Watanabe, S. Akine, T. Nabeshima, M. Nakano, T. Yamamura and T. Kajiwara, Angew. Chem., Int. Ed., 2011, 50, 4016 CrossRef CAS PubMed.
- H. L. C. Feltham, Y. Lan, F. Klöwer, L. Ungur, L. F. Chibotaru, A. K. Powell and S. Brooker, Chem. – Eur. J., 2011, 17, 4362 CrossRef CAS PubMed.
- J. Rinck, G. Novitchi, W. V. Heuvel, L. Ungur, Y. Lan, W. Wernsdorfer, C. E. Anson, L. F. Chibotaru and A. K. Powell, Angew. Chem., Int. Ed., 2010, 49, 7583 CrossRef CAS PubMed.
- V. M. Mereacre, A. M. Ako, R. Clérac, W. Wernsdorfer, G. Filoti, J. Bartolomé, C. E. Anson and A. K. Powell, J. Am. Chem. Soc., 2007, 129, 9248 CrossRef CAS PubMed.
- C. Papatriantafyllopoulou, W. Wernsdorfer, K. A. Abboud and G. Christou, Inorg. Chem., 2011, 50, 421 CrossRef CAS PubMed.
- Y.-F. Zeng, G.-C. Xu, X. Hu, Z. Chen, X.-H. Bu, S. Gao and E. C. Sañudo, Inorg. Chem., 2010, 49, 9734 CrossRef CAS PubMed.
- V. Baskar, K. Gopal, M. Helliwell, F. Tuna, W. Wernsdorfer and R. E. P. Winpenny, Dalton Trans., 2010, 39, 4747 RSC.
-
(a) A. Palii, B. Tsukerblat, S. Klokishner, K. R. Dunbar, J. M. Clemente-Juan and E. Coronado, Chem. Soc. Rev., 2011, 40, 3130 RSC;
(b) X.-Y. Wang, A. V. Prosvirin and K. R. Dunbar, Angew. Chem., Int. Ed., 2010, 49, 5081 CrossRef CAS PubMed;
(c) M. G. Hilfiger, M. Shatruk, A. Prosvirin and K. R. Dunbar, Chem. Commun., 2008, 5752 RSC;
(d) V. Hoeke, A. Stammler, H. Bögge, J. Schnack and T. Glaser, Inorg. Chem., 2014, 53, 257 CrossRef CAS PubMed.
- D. E. Freedman, D. M. Jenkins, A. T. Iavarone and J. R. Long, J. Am. Chem. Soc., 2008, 130, 2884 CrossRef CAS PubMed.
- K. S. Pedersen, M. Schau-Magnussen, J. Bendix, H. Weihe, A. V. Palii, S. I. Klokishner, S. Ostrovsky, O. S. Reu, H. Mutka and P. L. W. Tregenna-Piggott, Chem. – Eur. J., 2010, 16, 13458 CrossRef CAS PubMed.
- J. J. Sokol, A. G. Hee and J. R. Long, J. Am. Chem. Soc., 2002, 124, 7656 CrossRef CAS PubMed.
- S. K. Langley, D. P. Wielechowski, V. Vieru, N. F. Chilton, B. Moubaraki, L. F. Chibotaru and K. S. Murray, Chem. Commun., 2015, 51, 2044 RSC.
-
(a) S. A. Kozimor, B. M. Bartlett, J. D. Rinehart and J. R. Long, J. Am. Chem. Soc., 2007, 129, 10672 CrossRef CAS PubMed;
(b) V. Mougel, L. Chatelain, J. Pécaut, R. Caciuffo, E. Colineau, J.-C. Griveau and M. Mazzanti, Nat. Chem., 2012, 4, 1011 CrossRef CAS PubMed;
(c) L. Chatelain, J. P. S. Walsh, J. Pécaut, F. Tuna and M. Mazzanti, Angew. Chem., Int. Ed., 2014, 53, 13434 CrossRef CAS PubMed.
-
(a) L. Ungur, W. Van den Heuvel and L. F. Chibotaru, New J. Chem., 2009, 33, 1224 RSC;
(b) I. J. Hewitt, J. Tang, N. T. Madhu, C. E. Anson, Y. Lan, J. Luzon, M. Etienne, R. Sessoli and A. K. Powell, Angew. Chem., Int. Ed., 2010, 49, 6352 CrossRef CAS PubMed.
|
This journal is © the Partner Organisations 2015 |
Click here to see how this site uses Cookies. View our privacy policy here.