DOI:
10.1039/D0NH00267D
(Minireview)
Nanoscale Horiz., 2020,
5, 1293-1302
Biomimetic hybrid membrane-based nanoplatforms: synthesis, properties and biomedical applications
Received
6th May 2020
, Accepted 22nd June 2020
First published on 22nd June 2020
Abstract
The rapid clearance and capture by the immune system pose a big challenge in targeted drug delivery using nanocarriers. Cell membrane coating endows nanoplatforms with prolonged blood circulation, enhanced immune escape, and improved targeting capability. However, monotypic cell membrane fails to meet the omnifarious needs of biomedical applications. The combination of different types of cell membranes provides a promising solution to provide multifunctional biomimetic nanoplatforms. In this review, we first discuss the feasibility of constructing biomimetic hybrid membranes and summarize current methods of preparing biomimetic hybrid membrane-based nanoplatforms (BHMNs) and their biomedical applications including drug delivery, cancer detection, detoxification, and cancer vaccines. Finally, the prospects and challenges of utilizing BHMNs for personalized medicine are also discussed.
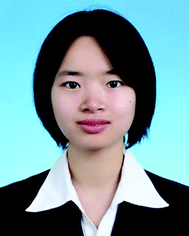
Yunyan Liao
| Yunyan Liao obtained her bachelor's degree from Harbin Medical University in 2018. Then she joined the Laboratory of Evolutionary Theranostics (LET) at Shenzhen University to pursue her Master's degree in Biomedical Engineering under the supervision of Prof. Peng Huang. Currently, her research focuses on the development of cell membrane-based nanomedicine. |
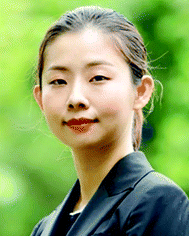
Yifan Zhang
| Yifan Zhang received her BS degree in Biotechnology from Nanjing University in 2011. In 2016, she obtained her PhD degree in Pharmacy from the Institute of Drug R & D at Nanjing University. After graduation, she joined the Laboratory of Evolutionary Theranostics (LET) at Shenzhen University as a postdoctoral fellow under the supervision of Prof. Peng Huang. She is now an Associate Professor in the LET at Shenzhen University. Currently, her research interest focuses on the design, development and application of stimuli-responsive nanomedicine for cancer theranostics and synergistic cancer therapy. |
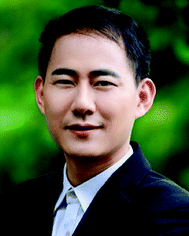
Peng Huang
| Peng Huang is a Distinguished Professor, Chief of the Laboratory of Evolutionary Theranostics (LET), and Director of the Department of Molecular Imaging, at the School of Biomedical Engineering, Shenzhen University Health Science Center, China. He received his PhD degree in Biomedical Engineering from the Shanghai Jiao Tong University in 2012. He then joined the Laboratory of Molecular Imaging and Nanomedicine (LOMIN) at the National Institutes of Health (NIH) as a postdoctoral fellow. In 2015, he moved to Shenzhen University as a Distinguished Professor. His research focuses on the design, synthesis, and biomedical applications of molecular imaging contrast agents, stimuli-responsive programmed targeting drug delivery systems, and activatable theranostics. |
1. Introduction
Both cancer and bacterial infections are serious global health threats, which caused an estimated 9.6 million deaths in 2018 and 8 million deaths in 2017.1,2 Nanoparticles have been widely investigated in drug delivery and disease detection owing to some distinct appealing features, such as improved circulation, reduced toxicity, and targeting capability.3–5 However, artificial nanomedicines have nonnegligible immunogenicity and are easily captured by the human reticuloendothelial system.6,7 It was estimated that only 0.7% (median) of drug intravenously administered was delivered to the tumor site, and the vast majority was either stuck in normal tissues or eliminated.8 The standard strategy to solve this problem is to modify the nanomedicines with an amphiphilic polymer, such as polyethylene glycol (PEG). However, it was reported that PEG also has immunogenicity and leads to formation of anti-PEG antibodies.9,10
In recent years, cell membrane coated-nanoparticles as biomimetic drug carriers have attracted attention in personalized theranostics including cancer imaging, cancer therapy, and antibacterial therapy.11–15 Cell membrane-based nanoplatforms can effectively avoid the surveillance of the immune system, prolong the blood circulation of the drug, and increase the drug accumulation in the tumor or inflammatory sites.16,17 For instance, CD47 is highly expressed on the membrane surface of red blood cells (RBCs), an important component of blood cells, and acts as a marker of “don't eat me” by binding with signal-regulatory protein α expressed on macrophages.18 Therefore, coating with the RBC membrane is like coating the nanoparticles with a “covert coating”, especially for immune cells.12 However, there is no targeting ligands on the surface of RBCs for the navigation of nanoparticles to the disease sites, which is the footstone of personalized medicine. In many other cases, tumor cell membranes are widely applied in cancer theranostics owing to their homotypic targeting capability.19 The abundant immunosuppressive molecules (e.g. PD-L1) expressed on the tumor cell membranes may interfere with the ability of the host immune system to identify tumor cells and enact anti-tumor immunity.20 However, current membrane extraction techniques can hardly guarantee the integrity of the tumor cell membrane, and tumor cells cultured in the laboratory are quite different from in vivo tumor cells, so the immune escape ability of tumor cell membrane-coated nanoparticles is limited. To enhance the immune escape and the therapeutic outcome, the integration of multiple cell membranes into hybrid membranes represents a promising approach.
Currently, a number of biomimetic hybrid membrane-based nanoplatforms (BHMNs) have been designed and developed for drug delivery, detoxification, cancer detection, and cancer vaccines.21–24 The membranes used in BHMNs are mainly from red blood cells (RBCs), platelets, tumor cells, immune cells (e.g. macrophage), and bacteria.25–27 Compared to a monotypic cell membrane, a hybrid membrane could combine the functions of the original membranes, such as half-life extension, immune escape, active tumor targeting, and adherence to tumor cells.23 In addition, the hybrid membrane also achieves novel functions that are not available in the original membranes, such as the presentation of tumor antigens to antigen-presenting cells (APCs) through lymph node homing.25 Furthermore, hybrid membranes can improve the tissue distribution of nanoparticles by reducing the adhesion of unrelated natural cells (e.g. white blood cells)24 or selectively improve the accumulation of nanoparticles in organs such as the liver and spleen.22 Owing to these distinct advantages, the integration of multiple cell membranes into hybrid membranes has shown great application potential in cancer therapy and bacterial detoxification. The hybrid membranes can be prepared either by extracting each cell membrane before membrane fusion or by fusing the two cells before membrane extraction. The existing membrane compositions and biological applications are shown in Scheme 1.
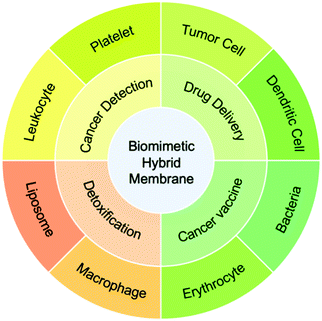 |
| Scheme 1 The membrane classifications and biomedical applications of BHMNs. | |
In this review, the state-of-art progress of BHMNs for anticancer and antibacterial therapies will be summarized. First, we introduce some main preparation procedures and classifications of hybrid membranes. Next, the applications of BHMNs in drug delivery, cancer vaccines, cancer detection, and detoxification are elaborated in detail. Last but not least, the prospects and challenges of BHMNs for further biomedical applications are discussed.
2. Classification of BHMNs
At present, multifarious BHMNs have been developed for biomedical applications. The key of the design is to choose appropriate types of cell membrane for specific applications. Generally, BHMNs are composed of one membrane that targets the sites of action and another membrane that enhances specific biological functions (Table 1). The former membrane includes tumor cell membranes that target tumor sites through homotypic adhesion, and platelet membranes that target tumor cells via P-selectin. The functions of the latter membranes mainly include enhancing targeting capability (e.g. white blood cell membranes in the detection of CTCs), circulation-extension (e.g. RBC membrane), immune evasion (e.g. immune cell membrane), and immune regulation (e.g. bacterial membrane, immune cell membrane).
Table 1 Compositions and biomedical applications of representative BHMNs
Membrane composition |
Merits |
Membrane proportion |
Membrane fusion method |
Nanoparticle |
Therapy model |
Mode of administration |
Dose (mg kg−1) |
Ref. |
AuNW: acoustic gold nano-wire; DOX/DTX: doxorubicin; SREBP1: sterol regulatory element-binding protein 1; LC: cross-linked peptide-lipoic acid micelles; PCN-224MOF: porphyrin-based Zr-metal–organic frameworks; ICG: indocyanine green; PLGA: poly(lactic-co-glycolic acid); CTC: circulating tumor cells; i.v.: intravenous; s.c.: subcutaneous.
|
Erythrocyte-platelet |
Targeting and absorbing toxins; binding to pathogen |
1 : 1 (w/w) |
Mix and stir |
An acoustic AuNWa robot |
— |
— |
— |
31
|
Long circulation; tumor targeting |
2 : 1 (w/w) |
Sonication |
Polypyrrol |
HCT116 tumor |
i.v.
|
5 (polypyrrol) |
32
|
Erythrocyte-tumor cells |
Long circulation; homotypic targeting; antigen library |
1 : 1 (w/w) |
Sonication |
CuS-DOX |
B16F10 tumor |
i.v.
|
5 (CuS) |
23
|
20 : 1 (w/w) |
Coextrusion |
Ag |
B16F10-Luc tumor; 4T1 tumor |
i.v.
|
0.5 (Ag) |
22
|
1 : 1 (w/w) |
Coextrusion |
Melanin |
MCF-7 tumor |
i.v.
|
4.76 (Melanin) |
33
|
— |
— |
Dextran-g-poly/BLZ-945 |
4T1 tumor |
i.v.
|
5 (BLZ-945, 2 days for 5 times) |
34
|
Platelet-leukocytes |
Targeting CTCs; reducing the binding of white blood cells |
— |
Coextrusion |
Magnetic beads |
CTCs in clinic blood samples |
— |
— |
24
|
Platelet-cancer stem cell |
Long circulation; targeting tumor stem cells |
— |
Mix and stir |
Fe3O4 |
CAL 27 tumor |
i.v.
|
25 (Fe) |
35
|
Tumor cells-leukocytes |
Homotypic targeting; avoiding the capture by mononuclear phagocyte system |
1 : 1 (w/w) |
Coextrusion |
PTX-Liposomal |
HN12 tumor |
i.v.
|
10 (PTX, six times for 4 days) |
36
|
— |
— |
Fe3O4@SiO2 |
CTCs in clinic blood samples |
— |
— |
37
|
Tumor cell-dendritic cells |
Antigen library; homotypic targeting; lymph node homing |
1 : 2 (w/w) |
Fusion cells |
PCN-224MOF |
4T1 tumor |
i.v./s.c. |
29.09 (MOF) |
21 and 25
|
Tumor cell-bacterial |
Homotypic targeting; antigen library; immune adjuvant |
— |
Coextrusion |
PLGA-ICG |
B16F10,4T1 tumor |
i.v./s.c. |
— |
27
|
Tumor cell-bone marrow mesenchymal stem cells |
Homotypic targeting |
1 : 1 (w/w) |
— |
LC/DTX/siSREBP1 |
BmCRPC tumor |
i.v.
|
1 (DTX) |
38
|
Liposome-erythrocyte |
Regulation of cell membrane fluidity; recruitment of toxins |
16 : 1 (v/v) |
Coextrusion |
— |
HIα |
i.v.
|
— |
39
|
Liposome-macrophage |
Stablization of the hybrid membrane for long circulation; recruitment of toxins |
— |
Coextrusion |
— |
Sepsis |
i.v.
|
— |
40
|
3. Synthesis of BHMNs
Since Dehaini et al. successfully prepared a hybrid cell membrane of RBC membrane and platelet membrane in 2017,26 a myriad of BHMNs have been developed for cancer detection, anticancer therapy, and antibacterial therapy. The fusion of two kinds of cell membranes is simple and convenient and does not require chemical modification, which thus allows mass production. The preparation process of natural cell membrane usually includes cell lysis, cell content elimination, membrane acquisition, and cell membrane purification.28 Among these steps, membrane acquisition is no doubt the key step. The process to acquire cell membranes mainly involves cell lysis by low-permeability buffer, sonication, homogenization, and repeated freeze–thaw.29 The only extra step to acquire a biomimetic hybrid membrane, of course, is to fuse two different cell membranes. Currently, there are two methods to extract the hybrid membrane from cells. One way is to first fuse two cells for membrane extraction (fusion to membrane extraction), and the other way is to extract each cell membrane and fuse them (membrane extraction to fusion) (Scheme 2).
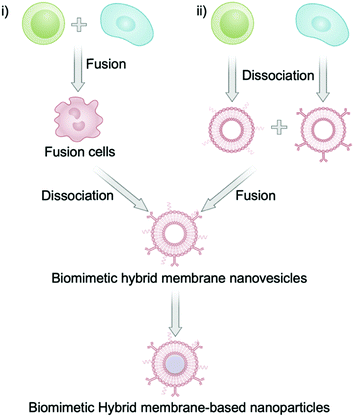 |
| Scheme 2 Two main fusion strategies for the synthesis of biomimetic hybrid membrane-based nanoplatforms. (i) Membrane fusion before membrane extraction and (ii) membrane extraction before membrane fusion. | |
Specifically, the first approach is to prepare a fusion cell before extracting the membrane by conventional methods.25 This special fusion cell is usually obtained by stimulation with polyethylene glycol or electrofusion.30 Interestingly, the protein expression of fusion cell probably differs from the original cells because this method generates new proteins. For instance, Liu et al. prepared the fusion cells of tumor cells and dendritic cells before extraction of the hybrid membranes. It was found that the obtained hybrid membrane had new membrane proteins compared to the membrane protein of the original cells (Fig. 1).21 Notably, the proportion of each membrane significantly influences the functions of the as-prepared biomimetic nanoplatforms, such as circulation time and tissue distribution.22 Another approach of cell membrane fusion is to extract each kind of cell membrane and then to stir the mixture of two membranes at 37 °C or in an ice bath for 10 min to promote the membrane fusion.26 Hybrid membranes can also be obtained by sonication of the membrane mixture.23 In some cases, the sonicated membranes were co-extruded instead of squeezing when wrapping the nanoparticles in order to facilitate the membrane fusion.22 These procedures are not only suitable for the fusion of a eukaryotic cell membrane and a eukaryotic cell membrane, but also for the fusion of a prokaryotic cell membrane and a eukaryotic cell membrane. For example, Chen et al. prepared a biomimetic hybrid membrane nanovesicle composed of tumor cell membrane and bacterial membrane by the co-extrusion of the membranes for cancer vaccines.27
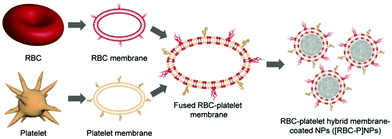 |
| Fig. 1 Schematic of the synthesis of RBC-platelet hybrid membrane-coated NPs ([RBC-P]NPs). Membrane materials are derived from the fusion of RBC and platelet membranes. The resulting hybrid membrane is then used to coat poly(lactic-co-glycolic acid) (PLGA) polymeric cores to synthesize [RBC-P]NPs. Copyright © 2018 Wiley-VCH Verlag GmbH & Co. KGaA, Weinheim. | |
4. Biomedical applications of BHMNs
Recently, the BHMNs have been widely investigated as personalized nanomedicine owing to the multifunctionality and targeting capability of the hybrid membranes. In this review, the biomedical applications of BHMNs in drug delivery, cancer detection, detoxification, and cancer vaccines are discussed.
4.1 Drug delivery
The poor tumor accumulation of nanomedicine remains a huge hurdle for effective drug delivery. To solve this problem, PEG is a standard polymer that is broadly applied to modify the nanoparticle and prolong its blood circulation. However, it was found that the PEGylated nanoparticles can induce the generation of anti-PEG immunoglobulin M and G antibodies and then trigger a quick clearance of the nanoparticles, which is called the accelerated blood clearance or “ABC” phenomenon.9 Compared to artificial polymers, endogenous cell membrane has several unique advantages including low immunogenicity, targeting capability, and abundant functional protein library. For instance, RBCs are the most abundant blood cells in the human body, and mature RBCs have no organelles such as nuclei. Thus, the extraction of RBC membrane has several advantages such as high purity and high yield. CD47 on the surface of RBC membrane can help macrophages escape and realize the long blood circulation of nanoparticles.41 Compared with PEGylated nanoparticles, the half-life of RBC membrane-based nanoparticles can be doubled in vivo.12 But the pure RBC membrane lacks active targeting. Platelets are also abundant blood cells that promote the blood coagulation. Once there is a damaged tissue in the body, platelets can immediately adsorb to and repair it. Platelet membrane not only highly expressed CD47, but also expressed CD55 and CD59, which can inhibit the immune complement system and further improve the long circulation.28 The hybrid RBC/platelet membrane combines the long circulation of RBC membrane and the targeting capability of platelet membrane for effective anticancer therapy. For example, Liu et al. used these hybrid membrane-coated polypyrrol nanoparticles to enhance the photothermal therapy of colorectal HCT116 tumors and give prolonged blood circulation and tumor-specific targeting compared to a single cell membrane.32
Tumor cells can adhere to each other through intercellular adhesion molecules (e.g. cadherin and Ig superfamily), which are highly expressed on the surface of tumor cells, as well as galactose adhesin.42 Even in vitro, tumor cells easily assemble into 3D cell spheres.43 This homotypic adhesion between tumor cells also occurs between tumor cell membranes and tumor cells. In addition, many studies have shown that the CD47 expressed on the surface of tumor cells can avoid immune surveillance.44,45 However, a pure tumor cell membrane may lose its integrity of constituent membrane proteins during the preparation of the membrane vesicles, and fail to avoid immune surveillance completely. The combination of a tumor cell membrane with a leukocyte membrane can make up for this defect (Fig. 2a). He et al. showed that paclitaxel (PTX)-loaded hybrid tumor cell/leukocyte membrane-based nanovesicles can effectively accumulate at the tumor site (79.1 ± 6.6% ID per gram of tumor) and effectively reduce the phagocytosis of leukocytes (Fig. 2b and c).36 In addition, the hybrid tumor stem cell/platelet membrane is deemed to enhance the active targeting capability of tumor stem cells and also reduce the “ABC” phenomenon in blood. For example, Bu et al. camouflaged Fe3O4 nanoparticles with a hybrid platelet/tumor stem cell membrane, which effectively achieved the immune escape of the ABC phenomenon, and it had excellent performance in the Tgfbr1/Pten double knock-out head and neck tumor mouse model, which has a similar tumor microenvironment and immune function to human head and neck cancer.35 Hybrid tumor cell/RBC membrane-based nanoplatforms have also been investigated in cancer therapy (Fig. 3a). The RBC membrane can increase the half-life of biomimetic nanoplatforms, while the tumor cell membrane can target the nanoplatforms to the tumor sites via homotypic targeting. For example, Wang et al. prepared a hybrid RBC/tumor cell membrane-camouflaged adriamycin-loaded hollow copper sulfide nanoparticle (DCuS@[RBC-B16]NPs), which achieved significantly longer half-life than the nanoparticles coated with the pure RBC membrane (Fig. 3b) and higher tumor accumulation than the nanoparticles coated with the pure tumor cell membrane (Fig. 3c).23
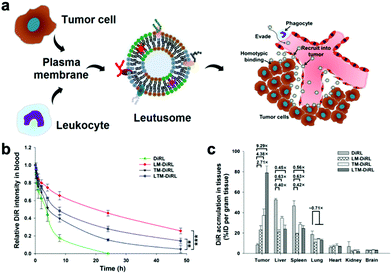 |
| Fig. 2 The utilization of a hybrid leukocyte/tumor cell membrane to hydrate the thin film to load paclitaxel or fluorescent dyes for drug delivery. (a) Schematic of the utilization of the hybrid membrane of leukocyte/HN12 tumor cells to encapsulate paclitaxel to prepare PTX-loaded liposomal nanoparticles. (b) Biodistribution in the major organs at 48 h post-intravenous (i.v.) injection. (c) Quantification of fluorescence intensity of DiR in the major organs. Copyright © 2018, American Chemical Society. | |
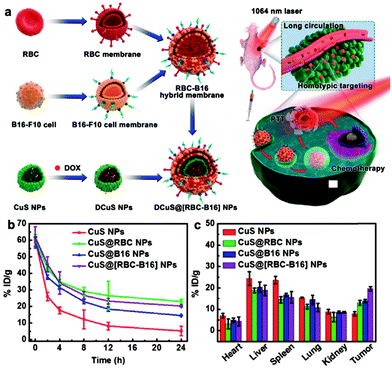 |
| Fig. 3 The hybrid RBC/tumor cell membrane-coated hollow copper sulfide nanoparticles for drug delivery. (a) Schematic of the hybrid membrane of RBCs/B16-F10 cells used to camouflage DOX-loaded hollow copper sulfide nanoparticles (DCuS NPs) to produce DCuS@[RBC-B16]NPs and the synergistic photothermal/chemotherapy of melanoma. (b) The blood retention and (c) tissue distribution 24 h after i.v. injection of CuS NPs, CuS@RBC NPs, CuS@B16 NPs, and CuS@[RBC-B16]NPs. Copyright © 2018, American Chemical Society. | |
4.2 Cancer vaccines
The tumor cell membrane has a complete tumor antigen library, which is a prerequisite for an effective cancer vaccine. However, the insufficient delivery of tumor antigens to APCs will reduce the effectiveness of cancer vaccines.46 Dendritic cells (DCs) recognize the tumor antigens expressed on the membrane of tumor cells, and then process and present the antigens in the form of an antigen peptides-major histocompatibility complex (pMHC) on the membranes.47 Therefore, the integration of a tumor cell membrane and a DC membrane is deemed to not only achieve a strong expression of both the whole tumor antigen complexes and immunological co-stimulatory molecules, but also present the tumor antigens to DCs to activate them and thus boost the immune response. The intratumoral infiltration of CD8+ cytotoxic T lymphocytes (CTLs) can then be analyzed to evaluate the immune response. Liu et al. proved that this hybrid membrane-coated PCN-224MOF led to a significant increment of CD8+ CTL proportion to 14.45%, which was higher than that of MOF coated with the tumor membrane (9.69%) and the DC membrane (11.02%) groups.21 In addition, a bacterial membrane has inherent immune adjuvant and immunity enhancement effects.48,49 The fusion of the bacterial and tumor cell membranes generates a powerful cancer vaccine, which strongly stimulates the immune system and causes a tumor-specific immune response.27 In another case, Chen et al. demonstrated that this combination significantly increased the tumor infiltration percentage of CD8+ CTLs to 16.4%, but that of tumor cell membrane or bacterial membrane only increased 3.11–3.31%.27
The spleen is an important secondary lymphoid organ, which contains abundant B cells, T cells, natural killer (NK) cells, and APCs (e.g. DC cells).50–52 The tumor-associated antigens are presented to the splenic APCs to trigger a T cell immune response. Since aging or damaged RBCs are removed from the spleen, incomplete RBCs can be used to deliver tumor-associated antigens (TAA) to APCs in critical secondary lymphoid organs. Han et al. developed a hybrid RBC/tumor cell membrane-coated nano-Ag with a diameter of about 200 nm (Fig. 4a and b). However, it was found that when the protein mass ratio of erythrocyte membrane to tumor cell membrane was 20
:
1, the tumor antigen accumulation in the spleen was higher than that in the liver with a better activated T cell immune response (Fig. 4c–e).22
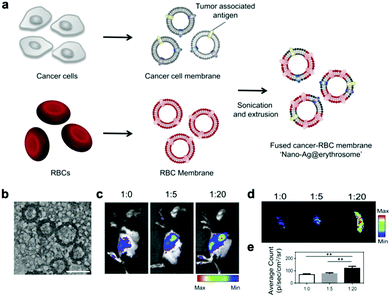 |
| Fig. 4 The hybrid RBC/tumor cell membrane-coated nano-Ag (nano-Ag@erythrosomes) as a cancer vaccine. (a) Schematic of the preparation of nano-Ag@erythrosomes by fusing tumor antigen-associated cell membrane into nanoerythrosomes. (b) Representative TEM image of nano-Ag@erythrosomes. Scale bar, 250 nm. (c) Fluorescence images of C57BL/6 mice injected with nano-Ag@erythrosomes in different ratios (n = 3); (d) ex vivo image of spleens and (e) the corresponding quantitative results (n = 3) 1 h after i.v. injection of nano-Ag@erythrosomes in various proportions. Copyright © 2019 American Association for the Advancement of Science. | |
4.3 Cancer detection
Tumor metastasis causes more than 90% of cancer-related deaths, which occurs due to the circulating tumor cells (CTCs) in primary solid tumors entering blood circulation.53,54 Therefore, the detection of CTCs plays an important role in the early detection, monitoring, and prognosis of malignant tumors. Many studies have shown that platelet activation and blood coagulation promote cancer metastasis.55 Moreover, CTCs are often accompanied by an aggregated thrombus to avoid the attack of immune cells such as natural killer cells, neutrophils, macrophages, and cytotoxic T cells. Thus, platelet membrane could be used to target the CTCs and tumor metastases.56 In addition to platelet membranes, leukocyte membranes could also effectively reduce the interference of homologous leukocytes and improve the CTCs’ isolation efficiency, and have been used for the separation of CTCs (Fig. 5a).57 For instance, compared with pure a platelet membrane and a leukocyte membrane, the tumor cell separation efficiency of immunomagnetic beads (IMBs) wrapped with the hybrid membrane of the two increased from 90.69% and 73.56% to 91.77%, while the separation purity increased from 81.99% and 92.47% to 96.98% (Fig. 5b and c).24 The utilization of tumor cell membranes to replace platelet membranes achieved similar effects owing to the homotypic targeting ability. Ding et al. found that, using magnetic beads wrapped with the hybrid membranes of tumor cells and leukocytes, the CTC capture efficiency and purity reached as high as 97.63% and 96.96%, respectively.37 The above studies demonstrate that hybrid membrane-coated magnetic beads could realize efficient CTC detection probably with a higher efficiency than the monotypic membrane-coating.
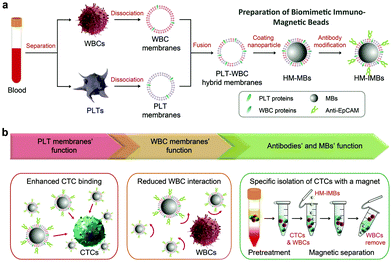 |
| Fig. 5 The hybrid tumor cell/white blood cell membrane-coated immunomagnetic beads (HM-IMBs) for cancer detection. (a) HM-IMBs inherited the enhanced affinity with CTCs from PLT membranes, and reduced interaction with homologous WBCs from WBC membranes, and thereby were used for the high-efficiency and high-purity isolation of CTCs. (b) Flow cytometry analysis of the captured cells from the mixed blood samples. (c) Capture efficiency of various IMBs based on different IMB volumes. The MCF-7 cells were incorporated into the whole blood of healthy donors. Copyright © 2018 Wiley-VCH Verlag GmbH & Co. KGaA, Weinheim. | |
4.4 Detoxification
Multi-drug resistance of bacteria is one of the major hurdles of antibacterial therapies such as antibiotic therapy.58 Unlike direct antimicrobial therapy, the detoxification of bacterial toxins can selectively remove the camouflage of pathogens, which has appealing advantages of retaining symbiotic flora, low antibiotic selective pressure, and slow development of drug resistance.59 Mimicking cell membranes such as liposome membranes are utilized in anti-virulence strategies because they are convenient for artificial designing and can provide adhesive lipid bilayers for bacterial toxins.60 For example, Henry et al. designed liposomes composed of sphingomyelin and high-concentration artificial cholesterol (cholesterol = 66 mol/%) to effectively compete with cells in vivo for bacterial toxins.61 Compared to pure lipid structure, the surface of cell membranes has other functional molecules, such as sugars and proteins, and can also act as a carrier for bacterial toxins. Therefore, to further enhance the detoxification efficiency of membrane vesicle, researchers have also developed cell membrane-coated nanoparticles for the application of antibacterial therapy.60
Systemic inflammatory response syndrome caused by bacterial infections (sepsis) is a major cause of the increase in mortality in intensive care units (ICUs) worldwide.62 The sepsis caused by Gram-negative bacteria is mainly through the secretion of endotoxins.63 Nanoparticles encapsulated in a macrophage membrane that absorb endotoxin and pro-inflammatory cytokines are proved to effectively treat this type of sepsis.64 In addition, many nanoparticles camouflaged by an erythrocyte membrane were capable of adsorbing toxins such as pore forming toxins on the surface of Gram-positive bacteria.11,65 Notably, He et al. proved that compared to an erythrocyte membrane and a liposome membrane (Fig. 6a), the integration of a liposome membrane and an erythrocyte membrane exhibited the most effective detoxification both in vitro (Fig. 6b and c) and in vivo (Fig. 6d).39 Similarly, the fusion of the liposome membrane and the macrophage membrane has been proved to prolong the in vivo blood circulation compared to that of the macrophage membrane alone.40 In addition, the RBC membrane can target the bacteria and absorb the pore-forming toxins on the surface, while the platelet membrane can adhere to the toxin produced by the bacteria. Esteban-Fernández de Ávila et al. proved that the hybrid RBC/platelet membrane coating can effectively enhance the detoxification efficiency of an acoustic AuNW robot.31 The long blood circulation promoted the contact between nanoparticles and bacterial toxins, and the strategy of targeting bacterial toxins with this hybrid membrane was more effective than that with a single membrane. Therefore, this strategy has the potential to be an adjuvant therapy for severe bacterial infections in the clinic.
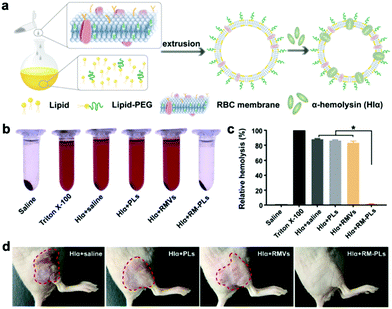 |
| Fig. 6 The hybrid RBC/liposome membrane for detoxification. (a) Schematic of the preparation of RM-PLs. Purified RBC membranes were fused to liposomes through extrusion. (b) Photographs and (c) the corresponding quantifications of hemolysis of RBCs incubated with Hlα mixed with saline, PLs, RMVs, and RM-PLs, respectively. Saline and Triton X-100 without Hlα were used as negative and positive controls, respectively; n = 3, *P < 0.0001. (d) After subcutaneous (s.c.) challenge with Hlα, mice were s.c. injected with mixed Hlα containing saline, PLs, RMVs, and RM-PLs, and skin lesions were photographed 3 days after injection. Copyright © 2019, American Chemical Society. | |
5. Prospects and challenges
Hybrid membrane camouflage presents an effective strategy to enhance the targeting ability, prolong the circulation time, and mitigate the side effect of drugs. Compared to conventional surface modification methods, this approach is more convenient and biocompatible. Compared to a monotypic cell membrane, biomimetic hybrid membranes provide more functions and adjustable tissue distribution profiles. Until now, hybrid membrane-based nanomedicine has been widely investigated in the fields of drug delivery, cancer detection, cancer vaccines, and detoxification. But its potential applications are yet to be fully developed. For example, BHMNs also have potential applications as a drug carrier to treat acute inflammation-related diseases, such as acute myocardial infarction, atherosclerosis, stroke, and arthritis. The nanometer size of BHMNs allows convenient passage through the vascular endothelium to reach the lesion site.66,67 When inflammation occurs, immune cells first aggregate in the inflamed tissue, and platelets and stem cells play an important role in tissue repair. In addition, some types of membranes could facilitate the immune system escape and non-specific adhesion of blood cells (e.g. erythrocytes and white blood cells). The integration of these membranes has a potential use in targeted drug delivery to blood cells. Therefore, compared to a monotypic membrane, a hybrid membrane possesses more functions and broader biomedical application prospects.
Furthermore, more types of membranes can be involved in hybrid membranes. For example, in addition to DC membranes, other immune cell membranes including NK cell membranes, neutrophil membranes, and virus capsids also have application prospects in biomedicine owing to several common merits like targeting enhancement, immune regulation ability, etc.68–70 Bacterial membranes not only have immune adjuvant properties, but also have been found to endow nanoparticles with a targeting capability to the hypoxic and necrotic areas of tumor tissues by receptors that are located at the bacterial poles on the outer membrane for the sensing of nutrients.71–73
Last but not least, hybrid membrane coatings have extensive application prospects in the development of cancer vaccines. The pure tumor cell membrane can hardly maintain the same integrity as the whole tumor cell during the process of membrane extraction. An incomplete tumor cell membrane may lose some proteins that assist avoiding immune surveillance, and then be captured by the immune system, which brings safety and efficacy risks. The combination of other cell membranes with a tumor cell membrane to avoid the recognition and clearance of nanoparticles by the immune system has proved to be an effective strategy. In addition, some hybrid membrane coated-nanomedicines can regulate the immune system, suppress tumor recurrence, and enhance the immune memory.
Notably, the extraction method should be carefully selected in terms of the applications, because the complicated procedures will influence the integrity of the membrane proteins. The state-of-the-art characterization tools required to confirm the membrane protein retention of BHMNs are listed in Table 2. Additionally, once the BHMNs enter the blood vessel, a protein corona will be absorbed on the surface of nanoparticles.74 The compositions of the protein corona and its role in the biological events of BHMNs such as toxicity, immune response, biodistribution, metabolism, and clearance need to be carefully identified in order to optimize the theranostic effects. Besides, the type and proportion of membranes should also be carefully considered to enhance the functions or remedy the defects of each membrane, or to improve the tissue distribution and stability of the obtained biomimetic nanomedicine. For example, tumor cell membranes can enhance the active tumor targeting of nanoparticles through homotypic targeting. The integration of an RBC membrane can further enhance the tumor accumulation of nanoparticles by prolonging the long circulation time of nanoparticles, which cannot be achieved by using other types of tumor cell membranes. In terms of tumor cell membrane, since the complexity of an in vivo solid tumor is much higher than that of commercial tumor cell lines, it is advisable to fabricate this biomimetic tumor nanovaccine using tumor cells that are isolated and expanded ex vivo. Moreover, the existing few studies are far from enough to guarantee the biosafety of the hybrid membrane cell-based method. Perhaps some genetic or chemical methods can be used to inactivate the immunogenic components at the membrane surface, such as knocking out related genes or blocking a receptor protein using specific ligands. The repeatability and biosafety of the hybrid membrane-based nanomedicine need to be thoroughly verified in the preclinical and early clinical stages.
Table 2 State-of-the-art characterization tools of proteins on a hybrid membrane
Method |
Purpose |
Mechanism |
Ref. |
Western blotting |
The identification of membrane proteins |
The specific antigen–antibody binding |
21, 35, 36, 39 and 40
|
SDS-polyacrylamide gel electrophoresis |
The retention of membrane proteins |
Proteins with different mass have different migrate speeds |
21, 25, 39 and 40
|
Immunoelectron microscopy |
The identification of membrane proteins |
The specific antigen–antibody binding |
23 and 33
|
Immunofluorescence |
The identification of membrane proteins |
The specific antigen–antibody binding |
25
|
Proteomics analysis |
The identification of membrane protein compositions |
Two-dimensional electrophoresis and mass spectrum |
39
|
6. Conclusion
In summary, BHMNs have been widely applied in cancer detection, cancer therapy, and antibacterial therapy because of their versatility and good biocompatibility. This strategy can remedy the deficiencies of monotypic cell membrane camouflage such as the active targeting ability of red cell membrane and the immune escape ability of tumor cell membrane, and perform multiple theranostic functions. In this review, we summarize the main preparation methods and types of hybrid membranes, and the biomedical applications of BHMNs in drug delivery, cancer detection, cancer vaccines, and detoxification. Overall, the development of BHMNs is still in its infancy. More types of membranes and application fields are yet to be explored. Continuous efforts need to be devoted toward the in-depth research on its efficacy, biosafety, and storage stability for clinical applications.
Conflicts of interest
There are no conflicts to declare.
Acknowledgements
This work was financially supported by the Basic Research Program of Shenzhen (JCYJ20180507182413022, JCYJ20180305163452667, JCYJ20170412111100742), the Fok Ying-Tong Education Foundation for Young Teachers in the Higher Education Institutions of China (161032), the National Natural Science Foundation of China (81903564, 21874119), the National Key Research and Development Program (2018YFA0704003), and the Guangdong Province Natural Science Foundation of Major Basic Research and Cultivation Project (2018B030308003).
Notes and references
- F. Bray, J. Ferlay, I. Soerjomataram, R. L. Siegel, L. A. Torre and A. Jemal, Ca-Cancer J. Clin., 2018, 68, 394–424 CrossRef PubMed
.
- A. A. Ordonez, M. A. Sellmyer, G. Gowrishankar, C. A. Ruiz-Bedoya, E. W. Tucker, C. J. Palestro, D. A. Hammoud and S. K. Jain, Sci. Transl. Med., 2019, 11, eaax8251 CrossRef PubMed
.
- Z. Zhang, S. H. Lee and S. Feng, Biomaterials, 2007, 28, 1889–1899 CrossRef CAS PubMed
.
- X. He, C. S. Alves, N. Oliveira, J. Rodrigues, J. Zhu, I. Banyai, H. Tomas and X. Shi, Colloids Surf., B, 2015, 125, 82–89 CrossRef CAS
.
- J. Shi, P. W. Kantoff, R. Wooster and O. C. Farokhzad, Nat. Rev. Cancer, 2017, 17, 20–37 CrossRef CAS PubMed
.
- S. Yang, W. Guo, Y. Lin, X. Deng, H. Wang, H. Sun, Y. Liu, X. Wang, W. Wang, M. Chen, Y. Huang and Y. Sun, J. Phys. Chem. C, 2007, 111, 17761–17764 CrossRef CAS
.
- C. Buzea, I. I. Pacheco and K. Robbie, Biointerphases, 2007, 2, MR17–MR71 CrossRef PubMed
.
- S. Wilhelm, A. J. Tavares, Q. Dai, S. Ohta, J. Audet, H. F. Dvorak and W. C. W. Chan, Nat. Rev. Mater., 2016, 1, 16014 CrossRef CAS
.
- E. T. M. Dams, P. Laverman, W. J. G. Oyen, G. Storm, G. L. Scherphof, J. W. M. Van der Meer, F. H. M. Corstens and O. C. Boerman, J. Pharmacol. Exp. Ther., 2000, 292, 1071–1079 CAS
.
- H. Schellekens, W. E. Hennink and V. Brinks, Pharm. Res., 2013, 30, 1729–1734 CrossRef CAS PubMed
.
- C. J. Hu, R. H. Fang, J. Copp, B. T. Luk and L. Zhang, Nat. Nanotech., 2013, 8, 336–340 CrossRef CAS PubMed
.
- C. J. Hu, L. Zhang, S. Aryal, C. Cheung, R. H. Fang and L. Zhang, Proc. Natl. Acad. Sci. U. S. A., 2011, 108, 10980–10985 CrossRef CAS PubMed
.
- S. Tan, T. Wu, D. Zhang and Z. Zhang, Theranostics, 2015, 5, 863–881 CrossRef CAS PubMed
.
- K. Xiong, W. Wei, Y. Jin, S. Wang, D. Zhao, S. Wang, X. Gao, C. Qiao, H. Yue, G. Ma and H. Xie, Adv. Mater., 2016, 28, 7929–7935 CrossRef CAS PubMed
.
- Y. Chen, H. Chen, D. Zeng, Y. Tian, F. Chen, J. Feng and J. Shi, ACS Nano, 2010, 4, 6001–6013 CrossRef CAS PubMed
.
- M. Xuan, J. Shao, L. Dai, J. Li and Q. He, ACS Appl. Mater. Interfaces, 2016, 8, 9610–9618 CrossRef CAS PubMed
.
- R. H. Fang, A. V. Kroll, W. Gao and L. Zhang, Adv. Mater., 2018, 30, 1706759 CrossRef PubMed
.
- P.-A. Oldenborg, A. Zheleznyak, Y. Fang, C. F. Lagenaur, H. D. Gresham and F. P. Lindberg, Science, 2000, 288, 2051–2054 CrossRef CAS PubMed
.
- J. Zhu, D. Zheng, M. Zhang, W. Yu, W. Qiu, J. Hu, J. Feng and X. Zhang, Nano Lett., 2016, 16, 5895–5901 CrossRef CAS PubMed
.
- A. Ribas and J. D. Wolchok, Science, 2018, 359, 1350–1355 CrossRef CAS PubMed
.
- W. Liu, M. Zou, T. Liu, J. Zeng, X. Li, W. Yu, C. Li, J. Ye, W. Song, J. Feng and X. Zhang, Nat. Commun., 2019, 10, 3199 CrossRef PubMed
.
- X. Han, S. Shen, Q. Fan, G. Chen, E. Archibong, G. Dotti, Z. Liu, Z. Gu and C. Wang, Sci. Adv., 2019, 5, eaaw6870 CrossRef PubMed
.
- D. Wang, H. Dong, M. Li, Y. Cao, F. Yang, K. Zhang, W. Dai, C. Wang and X. Zhang, ACS Nano, 2018, 12, 5241–5252 CrossRef CAS PubMed
.
- L. Rao, Q. Meng, Q. Huang, Z. Wang, G. Yu, A. Li, W. Ma, N. Zhang, S. Guo, X. Zhao, K. Liu, Y. Yuan and W. Liu, Adv. Funct. Mater., 2018, 28, 1803531 CrossRef
.
- W. Liu, M. Zou, T. Liu, J. Zeng, X. Li, W. Yu, C. Li, J. J. Ye, W. Song, J. Feng and X. Zhang, Adv. Mater., 2019, 31, 1900499 CrossRef PubMed
.
- D. Dehaini, X. Wei, R. H. Fang, S. Masson, P. Angsantikul, B. T. Luk, Y. Zhang, M. Ying, Y. Jiang, A. V. Kroll, W. Gao and L. Zhang, Adv. Mater., 2017, 29, 1606209 CrossRef PubMed
.
- Q. Chen, G. Huang, W. Wu, J. Wang, J. Hu, J. Mao, P. K. Chu, H. Bai and G. Tang, Adv. Mater., 2020, e1908185, DOI:10.1002/adma.201908185
.
- C. J. Hu, R. H. Fang, K. Wang, B. T. Luk, S. Thamphiwatana, D. Dehaini, N. Phu, P. Angsantikul, C. H. Wen, A. V. Kroll, C. Carpenter, M. Ramesh, V. Qu, S. H. Patel, J. Zhu, W. Shi, F. M. Hofman, T. C. Chen, W. Gao, K. Zhang, S. Chien and L. Zhang, Nature, 2015, 526, 118–121 CrossRef CAS PubMed
.
- Z. He, Y. Zhang and N. Feng, Mater. Sci. Eng., C, 2020, 106, 110298 CrossRef CAS PubMed
.
- T. H. Scott-Taylor, R. Pettengell, I. Clarke, G. Stuhler, M. C. La Barthe, P. Walden and A. G. Dalgleish, BBA, Mol. Basis Dis., 2000, 1500, 265–279 CrossRef CAS
.
- B. Esteban-Fernández de Ávila, P. Angsantikul, D. E. Ramírez-Herrera, F. Soto, H. Teymourian, D. Dehaini, Y. Chen, L. Zhang and J. Wang, Sci. Robot., 2018, 3, eaat0485 CrossRef
.
- Y. Liu, X. Wang, B. Ouyang, X. Liu, Y. Du, X. Cai, H. Guo, Z. Pang, W. Yang and S. Shen, J. Mater. Chem. B, 2018, 6, 7033–7041 RSC
.
- Q. Jiang, Y. Liu, R. Guo, X. Yao, S. Sung, Z. Pang and W. Yang, Biomaterials, 2019, 192, 292–308 CrossRef CAS PubMed
.
- Y. Wang, Z. Luan, C. Zhao, C. Bai and K. Yang, Eur. J. Pharm. Sci., 2020, 142, 105136 CrossRef CAS
.
- L. Bu, L. Rao, G. Yu, L. Chen, W. Deng, J. Liu, H. Wu, Q. Meng, S. Guo, X. Zhao, W. Zhang, G. Chen, Z. Gu, W. Liu and Z. Sun, Adv. Funct. Mater., 2019, 29, 1807733 CrossRef
.
- H. He, C. Guo, J. Wang, W. J. Korzun, X. Wang, S. Ghosh and H. Yang, Nano Lett., 2018, 18, 6164–6174 CrossRef CAS
.
- C. Ding, C. Zhang, S. Cheng and Y. Xian, Adv. Funct. Mater., 2020, 1909781, DOI:10.1002/adfm.201909781
.
- J. Chen, Z. Wu, W. Ding, C. Xiao, Y. Zhang, S. Gao, Y. Gao and W. Cai, Theranostics, 2020, 10, 1619–1632 CrossRef PubMed
.
- Y. He, R. Li, H. Li, S. Zhang, W. Dai, Q. Wu, L. Jiang, Z. Zheng, S. Shen, X. Chen, Y. Zhu, J. Wang and Z. Pang, ACS Nano, 2019, 13, 4148–4159 CrossRef CAS PubMed
.
- L. Jiang, R. Li, J. Xu, P. Luan, Q. Cui, Z. Pang, J. Wang, G. Lin and J. Zhang, Chem. Eng. J., 2019, 371, 15–25 CrossRef CAS
.
- X. Han, C. Wang and Z. Liu, Bioconjugate Chem., 2018, 29, 852–860 CrossRef CAS PubMed
.
- J. Xia, Y. Cheng, H. Zhang, R. Li, Y. Hu and B. Liu, Expert Rev. Anticancer Ther., 2017, 17, 517–526 CrossRef CAS PubMed
.
- A. Puliafito, A. De Simone, G. Seano, P. A. Gagliardi, L. Di Blasio, F. Chianale, A. Gamba, L. Primo and A. Celani, Sci. Rep., 2015, 5, 15205 CrossRef CAS
.
- G. Steinert, S. Schoelch, T. Niemietz, N. Iwata, S. A. Garcia, B. Behrens, A. Voigt, M. Kloor, A. Benner, U. Bork, N. N. Rahbari, M. W. Buechler, N. H. Stoecklein, J. Weitz and M. Koch, Cancer Res., 2014, 74, 1694–1704 CrossRef CAS PubMed
.
- R. Majeti, M. P. Chao, A. A. Alizadeh, W. W. Pang, S. Jaiswal, K. D. Gibbs, Jr., N. van Rooijen and I. L. Weissman, Cell, 2009, 138, 286–299 CrossRef CAS PubMed
.
- H. Wang and D. J. Mooney, Nat. Mater., 2018, 17, 761–772 CrossRef CAS PubMed
.
- C. M. Le Gall, J. Weiden, L. J. Eggermont and C. G. Figdor, Nat. Mater., 2018, 17, 474–475 CrossRef CAS PubMed
.
- Q. Chen, H. Bai, W. Wu, G. Huang, Y. Li, M. Wu, G. Tang and Y. Ping, Nano Lett., 2020, 20, 11–21 CrossRef CAS PubMed
.
- O. Y. Kim, H. T. Park, N. T. H. Dinh, S. J. Choi, J. Lee, J. H. Kim, S.-W. Lee and Y. S. Gho, Nat. Commun., 2017, 8, 9 CrossRef PubMed
.
- L. M. Kranz, M. Diken, H. Haas, S. Kreiter, C. Loquai, K. C. Reuter, M. Meng, D. Fritz, F. Vascotto, H. Hefesha, C. Grunwitz, M. Vormehr, Y. Hüsemann, A. Selmi, A. N. Kuhn, J. Buck, E. Derhovanessian, R. Rae, S. Attig, J. Diekmann, R. A. Jabulowsky, S. Heesch, J. Hassel, P. Langguth, S. Grabbe, C. Huber, Ö. Türeci and U. Sahin, Nature, 2016, 534, 396–401 CrossRef PubMed
.
- R. Ing, M. Segura, N. Thawani, M. Tam and M. M. Stevenson, J. Immunol., 2006, 176, 441–450 CrossRef CAS PubMed
.
- A. Banz, M. Cremel, A. Rembert and Y. Godfrin, Vaccine, 2010, 28, 2965–2972 CrossRef CAS PubMed
.
- A. F. Chambers, A. C. Groom and I. C. MacDonald, Nat. Rev. Cancer, 2002, 2, 563–572 CrossRef CAS PubMed
.
- P. Mehlen and A. Puisieux, Nat. Rev. Cancer, 2006, 6, 449–458 CrossRef CAS PubMed
.
- L. J. Gay and B. Felding-Habermann, Nat. Rev. Cancer, 2011, 11, 123–134 CrossRef CAS PubMed
.
- J. Li, Y. Ai, L. Wang, P. Bu, C. C. Sharkey, Q. Wu, B. Wun, S. Roy, X. Shen and M. R. King, Biomaterials, 2016, 76, 52–65 CrossRef CAS PubMed
.
- X. Zhou, B. Luo, K. Kang, Y. Zhang, P. Jiang, F. Lan, Q. Yi and Y. Wu, Small, 2019, 15, 1900558 CrossRef PubMed
.
- K. Bush, P. Courvalin, G. Dantas, J. Davies, B. Eisenstein, P. Huovinen, G. A. Jacoby, R. Kishony, B. N. Kreiswirth, E. Kutter, S. A. Lerner, S. Levy, K. Lewis, O. Lomovskaya, J. H. Miller, S. Mobashery, L. J. V. Piddock, S. Projan, C. M. Thomas, A. Tomasz, P. M. Tulkens, T. R. Walsh, J. D. Watson, J. Witkowski, W. Witte, G. Wright, P. Yeh and H. I. Zgurskaya, Nat. Rev. Microbiol., 2011, 9, 894–896 CrossRef CAS
.
- M. Lakemeyer, W. Zhao, F. A. Mandl, P. Hammann and S. A. Sieber, Angew. Chem., Int. Ed., 2018, 57, 14440–14475 CrossRef CAS
.
- R. H. Fang, B. T. Luk, C. J. Hu and L. Zhang, Adv. Drug Delivery Rev., 2015, 90, 69–80 CrossRef CAS PubMed
.
- B. D. Henry, D. R. Neill, K. A. Becker, S. Gore, L. Bricio-Moreno, R. Ziobro, M. J. Edwards, K. Muehlemann, J. Steinmann, B. Kleuser, L. Japtok, M. Luginbuehl, H. Wolfmeier, A. Scherag, E. Gulbins, A. Kadioglu, A. Draeger and E. B. Babiychuk, Nat. Biotechnol., 2015, 33, 81–88 CrossRef CAS
.
- D. C. Angus and T. van der Poll, N. Engl. J. Med., 2013, 369, 840–851 CrossRef CAS
.
- L. C. Casey, R. A. Balk and R. C. Bone, Ann. Intern. Med., 1993, 119, 771–778 CrossRef CAS PubMed
.
- S. Thamphiwatana, P. Angsantikul, T. Escajadillo, Q. Zhang, J. Olson, B. T. Luk, S. Zhang, R. H. Fang, W. Gao, V. Nizet and L. Zhang, Proc. Natl. Acad. Sci. U. S. A., 2017, 114, 11488–11493 CrossRef CAS PubMed
.
- T. Escajadillo, J. Olson, B. T. Luk, L. Zhang and V. Nizet, Front. Pharmacol., 2017, 8, 477 CrossRef PubMed
.
- M. E. Lobatto, C. Calcagno, A. Millon, M. L. Senders, F. Fay, P. M. Robson, S. Ramachandran, T. Binderup, M. P. M. Paridaans, S. Sensarn, S. Rogalla, R. E. Gordon, L. Cardoso, G. Storm, J. M. Metselaar, C. H. Contag, E. S. G. Stroes, Z. A. Fayad and W. J. M. Mulder, ACS Nano, 2015, 9, 1837–1847 CrossRef CAS
.
- Y. Kim, M. E. Lobatto, T. Kawahara, B. Lee Chung, A. J. Mieszawska, B. L. Sanchez-Gaytan, F. Fay, M. L. Senders, C. Calcagno, J. Becraft, M. Tun Saung, R. E. Gordon, E. S. G. Stroes, M. Ma, O. C. Farokhzad, Z. A. Fayad, W. J. M. Mulder and R. Langer, Proc. Natl. Acad. Sci. U. S. A., 2014, 111, 1078–1083 CrossRef CAS PubMed
.
- G. Deng, Z. Sun, S. Li, X. Peng, W. Li, L. Zhou, Y. Ma, P. Gong and L. Cai, ACS Nano, 2018, 12, 12096–12108 CrossRef CAS PubMed
.
- B. L. Thomas and M. Perretti, Nat. Nanotechnol., 2018, 13, 1098–1099 CrossRef CAS PubMed
.
- J. Wu, H. Wu, S. Nakagawa and J. Gao, Biomater. Sci., 2020, 8, 1058–1072 RSC
.
- J. A. MacDiarmid, N. B. Mugridge, J. C. Weiss, L. Phillips, A. L. Burn, R. P. Paulin, J. E. Haasdyk, K.-A. Dickson, V. N. Brahmbhatt, S. T. Pattison, A. C. James, G. Al Bakri, R. C. Straw, B. Stillman, R. M. Graham and H. Brahmbhatt, Cancer Cell, 2007, 11, 431–445 CrossRef CAS
.
- N. Van Dessel, C. A. Swofford and N. S. Forbes, Ther. Delivery, 2015, 6, 385–399 CrossRef CAS
.
- D. M. Wall, C. V. Srikanth and B. A. McCormick, Oncotarget, 2010, 1, 721–728 CrossRef
.
- R. Cai and C. Chen, Adv. Mater., 2019, 31, 1805740 CrossRef CAS PubMed
.
Footnote |
† These authors contributed equally to this work. |
|
This journal is © The Royal Society of Chemistry 2020 |
Click here to see how this site uses Cookies. View our privacy policy here.