DOI:
10.1039/D3QI01144E
(Review Article)
Inorg. Chem. Front., 2023,
10, 5244-5257
An overview of Mg-based IR nonlinear optical materials
Received
19th June 2023
, Accepted 24th July 2023
First published on 27th July 2023
Abstract
Infrared nonlinear optical (IR-NLO) materials play a vital role in generating IR laser output and have significant applications in the fields of communication, medicine, and security. At present, commercial IR-NLO crystals suffer from various performance drawbacks that constrain their range of applications. Therefore, the pursuit of designing and exploring new IR-NLO materials has emerged as an important avenue for the advancement of the IR laser industry. Benefiting from the various structural compositions, wide energy gaps, sufficient second-harmonic-generation intensities, strong laser-induced damage thresholds and favorable phase matching features, Mg-based IR-NLO materials have attracted wide attention in recent years. However, there has not been a specific review of this attractive family. In this overview, the recent advancements of Mg-based IR-NLO materials are summarized. These non-centrosymmetric compounds (including 36 chalcogenides and 4 pnictides) can be categorized into three types based on their chemical compositions: (i) ternary MgGa2Se4, MgSiP2, MgSiAs2, and Mg3Si6As8; (ii) quaternary Li4MgGe2S7, Li2Mg2MIII2S6 (MIII = Si, Ge), AEMg6Ga6S16 (AE = Ca, Sr, Ba), RE6MgSi2S14 (RE = Y, La–Nd, Sm, and Gd–Er), Li2MgMIVSe4 (MIV = Ge, Sn), Na4MgMIII2Se6 (MIII = Si, Ge), Cu2MgMIVQ4 (MIV = Si, Ge; Q = S, Se), MIMg3MIII3Q8 (MI = Na, Cu, Ag; MIII = Al, Ga; Q = S, Se), and Mg2In3Si2P7; and (iii) quinary Ba6Cu1.9Mg1.1Ge4S16 and Ba6Cu1.94Mg1.06Sn4S16. Their solid-state synthesis, crystal structures, optical properties and structure–activity relationships are systematically discussed. Finally, some useful conclusions and outlooks on this topic have been put forward.
1. Introduction
Nonlinear optical (NLO) crystals are crucial components of full-state lasers as they possess the capacity to enhance the frequency range, making them applicable in a variety of aspects such as laser spectroscopy, long distance laser communication, signal processing and so on.1–18 Due to the limitations of laser wavelength, significant research has been conducted on second order NLO materials, owing to their eminent ability to generate second harmonic generation (SHG). According to the various application bands, NLO materials can be divided into three groups, including ultraviolet (UV), visible-near infrared (Vis-NIR), mid- and far-infrared (MFIR) groups. Among them, NLO materials of UV and Vis-NIR wavelengths have already been deeply researched and commercialized in the past decades, such as KBe2BO3F2 (KBBF),19 β-BaB2O4 (β-BBO),20 LiB3O5 (LBO),21 KH2PO4 (KDP),22 and KTiOPO4 (KTP).23 Although traditional commercialized IR-NLO materials, such as AgGaS2,24 AgGaSe2,25 and ZnGeP2,26 have strong SHG coefficients (deff), they still possess some inevitable drawbacks such as an imperfect laser-induced damage threshold (LIDT) of AgGaS2, non-phase-matching property of AgGaSe2 and negative two-photon absorption of ZnGeP2.27,28 Therefore, the development of new outstanding IR-NLO materials is not only urgent but also challenging.
In order to meet the requirements of modern laser technology, high-performance IR-NLO materials need to fulfil the following conditions: (1) suitable birefringence (Δn at the range of 0.03–0.10) for phase-matching; (2) sufficient deff (>0.5 × AgGaS2, 13 pm V−1 for AgGaS2); (3) high LIDT (>1 × AgGaS2); (4) wide band gap Eg (>3.0 eV) and transparent range (covering the 3–5 μm and 8–12 μm atmospheric windows); (5) easiness to achieve growth of large size single crystals; and (6) satisfactory chemical and physical stability (mainly low hygroscopicity, resistance to the atmosphere, and possibility of cutting and grinding).29–40 In addition to the performance requirements mentioned above, it is also imperative that the material be crystallized in a non-centrosymmetric space group. This is because it is a prerequisite for it to function as an IR-NLO candidate.41–43 In recent decades, several design strategies have been developed. Among these, the most commonly used method for obtaining non-centrosymmetric structures is the introduction of various asymmetric building units (ABUs) into one structure. The common ABUs for IR-NLO materials are as follows: (i) distorted [MQ4] tetrahedra (M = main-group elements; Q = chalcogen);44–52 (ii) [MQn] polyhedra (M = transition-metal elements; n = 2, 3, and 4);53–57 (iii) distorted [REQn] polyhedra (RE = rare-earth-metal elements);58–61 (iv) lone-pair-cation-based [MQn] polyhedra (M = As(III), Sb(III), Bi(III), Pb(II), and Sn(II));62–71 and (v) mixed-anion [MOxQy] polyhedra.72–81
Recently, the introduction of Mg-based ABUs into the non-centrosymmetric structures has attracted considerable interest due to the following reasons: (i) Mg possesses large electropositivity (χ = 1.31), has a high Z/R ratio of 2.8 (Z, cation charge; R, cation radius), has light atomic mass and is non-poisonous. (ii) Mg is more inclined to form stable covalent bonds with chalcogen and pnictide atoms than are Ca, Sr, and Ba, which have strong ionic properties within the same family, for example, tetrahedral [MgQ4] and [MgPn4] (Pn = P, As) ABUs and octahedral [MgQ6] ABUs. (iii) Due to the absence of d–d and f–f electron transitions, Mg is beneficial for obtaining wide Eg values and expanding the IR transparent region.82–96 Moreover, highly polarizable Mg-based ABUs are helpful in maintaining strong deff and suitable Δn. However, a specific summary of non-centrosymmetric Mg-based materials has not been provided, even though they have been discovered to display excellent IR-NLO performances.
In this review, the recent advancements of Mg-based IR-NLO materials are summarized. These non-centrosymmetric compounds (including 36 chalcogenides and 4 pnictides) can be categorized into three types based on their chemical composition: (i) ternary MgGa2Se4, MgSiP2, MgSiAs2, and Mg3Si6As8; (ii) quaternary Li4MgGe2S7, Li2Mg2MIII2S6 (MIII = Si, Ge), AEMg6Ga6S16 (AE = Ca, Sr, Ba), Li2MgMIVSe4 (MIV = Ge, Sn), RE6MgSi2S14 (RE = Y, La–Nd, Sm, and Gd–Er), Na4MgMIII2Se6 (MIII = Si, Ge), Cu2MgMIVQ4 (MIV = Si, Ge; Q = S, Se), MIMg3MIII3Q8 (MI = Na, Cu, Ag; MIII = Al, Ga; Q = S, Se), and Mg2In3Si2P7; and (iii) quinary Ba6Cu1.9Mg1.1Ge4S16 and Ba6Cu1.94Mg1.06Sn4S16. Their solid-state synthesis, crystal structures, optical properties and structure–activity relationships are systematically discussed. Finally, some useful conclusions and outlooks on this topic have been put forward.
2. The survey on Mg-based IR-NLO materials
2.1 Ternary Mg-based chalcogenides and pnictides
2.1.1 MgGa2Se4.
The first defect-chalcopyrite-like Mg-based chalcogenide MgGa2Se4, with a non-centrosymmetric I
space group, was successfully designed and constructed via a structure prediction and experiment combined method by Li and co-workers in 2022. They used high-temperature solid-state reactions to create it, by mixing MgSe, Ga, and Se in a stoichiometric ratio of 1
:
2
:
3 at 1223 K.82
As shown in Fig. 1, Mg and Ga atoms both coordinate with Se atoms, forming a four-coordinated model represented by tetrahedral [MgSe4] and [GaSe4] ABUs. In the [Ga2Se7] channel, [MgSe4] ABUs are embedded via corner-sharing with adjacent [Ga1Se4] and [Ga2Se4], as depicted in Fig. 1b and c, resulting in a three-dimensional (3D) defect-chalcopyrite-like structure. Furthermore, this structure can also be achieved by utilizing chalcopyrite-like AgGaSe2, as depicted in Fig. 1d. When one Mg atom and one vacancy replace two Ag atoms in AgGaSe2, defect-chalcopyrite-like MgGa2Se4 is produced.
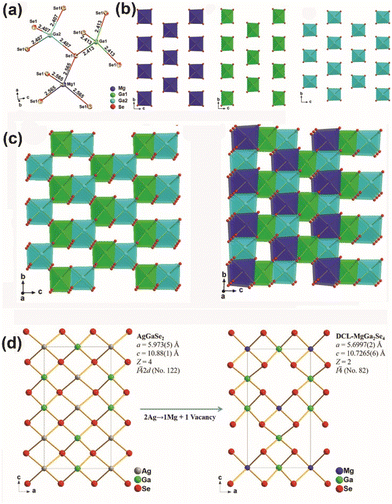 |
| Fig. 1 (a) Coordination environment of asymmetric building units in MgGa2Se4; (b) the arrangement of isolated [MgSe4], [Ga1Se4], and [Ga2Se4] tetrahedra in the bc plane; (c) [Ga2Se7]8− anionic framework (left) and 3D structure of MgGa2Se4 (right); (d) structural evolution from AgGaSe2 (left) to MgGa2Se4 (right). Copyright 2022 Wiley. | |
The developed MgGa2Se4 possesses a wide optical Eg (ca. 2.96 eV), a high LIDT (ca. 3.0 × AgGaS2), a broad transparency window (covering 3–12 μm), and a suitable phase-matching deff (ca. 0.9 × AgGaS2@150–200 μm). Therefore, MgGa2Se4 shows great potential as an IR-NLO candidate. Additionally, theoretical calculations reveal that the strong deff of MgGa2Se4 mainly originates from the [GaSe4] ABUs and nonbonding Se 4p states, and indicate that the tetrahedral [MgSe4] ABUs can effectively broaden the Eg of chalcopyrite-like compounds.
2.1.2 MgSiP2 and MgSiAs2.
In 2018, He's group conducted a systematic study of the electronic structures, linear and NLO properties, and thermodynamic stability of the MgMIVPn2 (MIV = Si, Ge, Sn; Pn = P, As) system through first-principles research. The aim of the study was to evaluate their potential in IR-NLO applications.83 In the same year, Kovnir and his colleagues successfully synthesized MgSiAs2 by reacting raw materials in the molar ratio of Mg
:
Si
:
As = 1.2
:
1
:
2 at the temperature of 1123 K.84 To obtain a single crystal, they performed three cycles of annealing and grinding coupled with acid treatment (HCl
:
H2O = 1
:
1). Recently, Ye and co-workers successfully obtained millimeter-level crystals of MgSiP2 (1.3 × 2.3 × 0.5 mm3) by mixing Mg, Si, P, and BaCl2 flux in a molar ratio of 1.33
:
1
:
2
:
2.67 and heating it to 1373 K.85
MgSiAs2 and MgSiP2 possess a classical chalcopyrite structure (space group: I
2d), with a 3D covalent framework comprising tetrahedral [SiPn4] (Pn = P, As) ABUs. The tunnels are filled with Mg2+ cations, which are coordinated with four closest Pn atoms (as depicted in Fig. 2). Computational and optical investigations have revealed that MgSiAs2 and MgSiP2 display direct Eg semiconductor properties with an optical Eg of 1.83 eV and 2.33 eV, respectively. MgSiAs2 exhibits a moderate deff (ca. 0.6 × AgGaS2@55–88 μm) and comparable LIDT (ca. 1.1 × AgGaS2), while MgSiP2 possesses a strong deff (3.5 × AgGaS2@150–212 μm), and a broad IR transparency window (0.53–10.3 μm). These studies indicate that the desired balance between Eg and deff can be achieved by rationally adjusting the ionicity–covalency–metallicity properties.
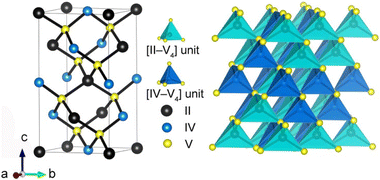 |
| Fig. 2 Crystal structure of II–IV–V2. Copyright 2018 IOP Publishing. | |
2.1.3 Mg3Si6As8.
In 2018, Kovnir and co-workers reported the discovery of Mg3Si6As8, which is the second ternary pnictide in the Mg–Si–As family. Single crystals of Mg3Si6As8 (3 × 1.5 × 1 mm3) were prepared through stoichiometric ratios of Mg, Si, and As and a 50 molar excess of Bi flux at 1150 K.84 It represents a new structure type and belongs to the non-centrosymmetric P4332 space group. The crystal structure of Mg3Si6As8 is composed of a complex 3D Si–As network with interspersed Mg cations. Two distinct environments can be observed for the Mg cations: i.e., tetrahedral [MgAs4] and octahedral [MgAs6] ABUs. Moreover, the Si–As network found in Mg3Si6As8 is similar to that of the binary SiAs, where six As atoms surround Si–Si dumbbells in both cases. The structure of Mg3Si6As8 creates a 3D rhombus grid fashion of Si2@As6 octahedra, as depicted in Fig. 3a. In contrast, these octahedra in the SiAs crystal structure form two-dimensional (2D) layers, as shown in Fig. 3b.
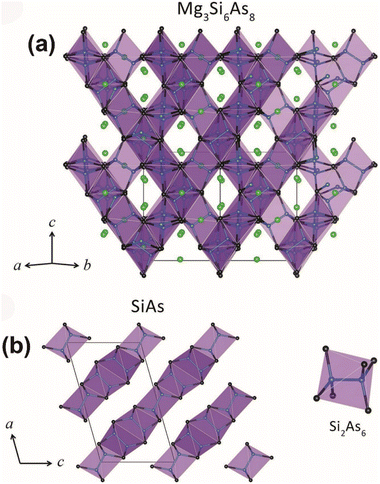 |
| Fig. 3 (a) Crystal structure of the 3D Si–As network in Mg3Si6As8 and (b) SiAs with Si2@As6 marked as purple octahedra. Green atom: Mg; blue atom: Si; black atom: As. Copyright 2018 Wiley. | |
Mg3Si6As8 displays more significant thermal stability than MgSiAs2 with a difference of 100 K (1240 K compared to 1140 K). Additionally, its experimental Eg is recorded as 2.02 eV. Unfortunately, it does not have SHG response due to its 432 Laue class.
2.2 Quaternary Mg-based chalcogenides and pnictides
2.2.1 Li4MgGe2S7.
The first alkali and alkaline-earth metal defect-chalcopyrite-like Mg-based chalcogenide Li4MgGe2S7, with a monoclinic Cc space group, was successfully designed based on the chemical substitute-oriented strategy in the MI4–MII–MIV2–Q7 system by Li and co-workers in 2021.86 Colorless block-shaped crystals of Li4MgGe2S7 were prepared by a high-temperature solution reaction using the starting materials Li2S, Mg, Ge, and S in the molar ratio of 2.5
:
1
:
2
:
5 at 1223 K.
Like other diamond-like chalcogenides, Li4MgGe2S7 can be seen as a derivative of wurtzite β-ZnS.97 Each metal is coordinated by four S atoms, forming tetrahedral [LiS4], [MgS4], and [GeS4] ABUs. The six [LiS4] ABUs are interconnected by sharing common sulfur atoms, creating a fascinating 6-membered ring (6-MR), with the [Ge1S4] tetrahedron located in the middle of this unit. Furthermore, the one-dimensional (1D) [GeMgS5] zigzag chains connect to the 6-MRs to construct a 2D honeycomb-like layer (Fig. 4a). The assembly of this honeycomb layer along the [101] direction is achieved via shared S atoms, resulting in the final 3D diamond-like framework (Fig. 4b). When viewed along the [203] direction, a clear depiction of the honeycomb-like 3D framework with an internal diameter of about 4.3 Å is observed (Fig. 4c). Additionally, unique [Ge2S7]6− dimers are discovered in Li4MgGe2S7 (Fig. 4d).
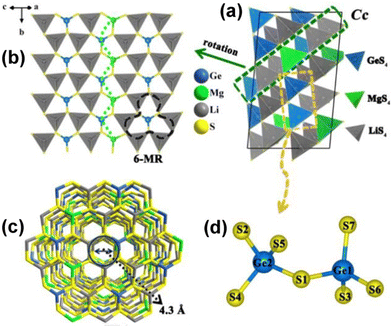 |
| Fig. 4 Crystal structure of Li4MgGe2S7: (a) 3D diamond-like structure; (b) single 2D layered fragment viewed along the [102] direction; (c) honeycomb-like 3D framework with an internal diameter of about 4.3 Å; (d) coordination environment of [Ge2S7]6− dimers. Copyright 2021 Wiley. | |
The obtained Li4MgGe2S7 exhibits the widest experimental Eg of 4.12 eV among reported quaternary metal chalcogenides. Additionally, it shows a suitable phase-matching deff (ca. 0.7 × AgGaS2 at 2090 nm, granularity range 15–200 μm) and a high LIDT (7 × AgGaS2 at 1064 nm), making it an excellent IR-NLO candidate for high-power laser applications. Theoretical calculations indicate that alkali metal Li and alkaline-earth metal Mg effectively enhance Eg, while covalent [Ge2S7]6− dimers contribute to a strong deff. This study enriches the diversity of diamond-like chalcogenides and offers a new route for the design and exploration of new IR-NLO materials with broad Eg and significant deff.
2.2.2 Li2Mg2MIII2S6 (MIII = Si, Ge).
The first Li-containing members of the A12−nxMxn+(T2Q6)2 family, Li2Mg2Si2S6 and Li2Mg2Ge2S6, were reported by Aitken and co-workers in 2022.87 Transparent and colorless single crystals of Li2Mg2Si2S6 and Li2Mg2Ge2S6 were obtained by the traditional high-temperature solid-phase technology using stoichiometric amounts of the reagents at 1173 K.
These two new compounds belong to the non-centrosymmetric polar space group P31m (No. 157) and represent a new structure type. As indicated in Fig. 5, each octahedral [LiS6] ABU shares its edge with three other [LiS6] ABUs to build a 2D layer in the ab plane, resulting in holes in the c direction. This layer is designated as “layer 1”. Similarly, along the c axis, there exist nearly identical layers formed by the octahedral [MgS6] ABUs, which are subsequently referred to as “layer 2”. These two layers, layer 1 and layer 2, are then interconnected along the c axis via face sharing of their respective octahedra to generate a 3D framework. These staggered ethane-like [Si2S6]6− groups are nestled within the holes present in layer 2 of the Mg–S layer, whereas the holes in layer 1 of the Li–S layer remain unoccupied. Each [MgS6] corner shares six of its corners with three ethane-like [Si2S6]6− units, and the orientation of Si–Si bonds is perpendicular to the [MgS6] layers.
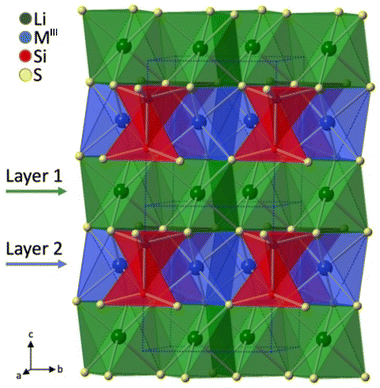 |
| Fig. 5 Polyhedral stacking structure of Li2Mg2MIII2S6 viewed along the bc plane. Copyright 2022 Wiley. | |
The optical Eg values of Li2Mg2Si2S6 and Li2Mg2Ge2S6 have been estimated through optical diffuse reflectance spectra, which are 3.24 eV and 3.18 eV, respectively. These compounds exhibit moderate deff of approximately 0.24 × KDP and 2.9 × α-SiO2 for Li2Mg2Si2S6, and 0.17 × KDP and 2.1 × α-SiO2 for Li2Mg2Ge2S6 when exposed to a Nd:YAG laser at 1064 nm.
The theoretical calculation results indicate that the atomic orbitals of Li and Mg atoms do not significantly contribute to the state near the valence band maximum. This finding is significant due to their positive electrical properties and the potential interaction with the primary sulfur ions.
2.2.3 AEMg6Ga6S16 (AE = Ca, Sr, Ba).
The first examples of a double alkaline-earth metal chalcogenide, AEMg6Ga6S16 (AE = Ca, Sr, Ba), were successfully discovered by Yu's group in 2022.88 Millimeter-level pale-yellow crystals of AEMg6Ga6S16 were prepared by the solid-state method in sealed silica tubes using the starting materials MgS, Ga2S3, and AES in the molar ratio 1.22
:
0.6
:
0.21 at 1323 K.
As a representative, SrMg6Ga6S16 (space group: P
) is adopted to describe the structure. In this structure, octahedral [MgS6] ABUs share both corners and faces to form [Mg3S14] trimers, while tetrahedral [GaS4] ABUs interconnect through vertex-sharing to create two distinct kinds of 1D Ga–S chains along the c direction, namely, [Ga(1)S3]∞ single chains (Fig. 6a) and [Ga(2,3)2S4]∞ double chains (Fig. 6b). Then, these [Mg3S14] trimers connect through vertex-sharing (in the a–b plane) and edge-sharing (along the c-axis) with two types of 1D Ga–S chains to form a 3D open [Mg6Ga6S16]2− framework (Fig. 6c). Finally, charge-balancing Sr atoms are introduced to fill the channels of this framework (Fig. 6d).
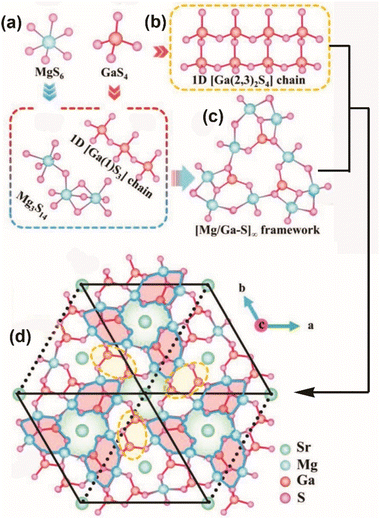 |
| Fig. 6 (a) Coordination environment of [Mg3S14] trimers and 1D [Ga(1)S3] chain; (b) 1D [Ga(2,3)2S4] chain; (c) 3D [(Mg/Ga)S]∞ framework and (d) crystal structure of SrMg6Ga6S16 viewed along the ab plane. Copyright 2022 Wiley. | |
The obtained chalcogenides melt congruently and are stable in both air and water, which facilitates the growth of single crystals. Performance characterization demonstrates that they can achieve a well-balanced combination of large phase-matching deff (ca. 0.7–0.8 × AgGaS2 at 2090 nm, granularity range 180–250 μm), wide Eg (3.50–3.54 eV), high LIDTs (ca. 11 × AgGaS2 at 1064 nm), and broad IR transparent windows (0.35–20 μm), which suggests that AEMg6Ga6S16 are potential IR-NLO candidates. Upon further studying the structure–property relationships, it has been shown that the excellent NLO properties of the material predominantly arise from the combined contribution of the polarizable [GaS4] and [MgS6] ABUs in the 3D open [Mg6Ga6S16]2− framework. These findings offer a brand-new family for developing IR-NLO candidates with stable framework structures and excellent properties.
2.2.4 RE6MgSi2S14 (RE = Y, La–Nd, Sm, and Gd–Er).
RE6MgSi2S7 (RE = Y, La–Nd, Sm, and Gd–Er) with a NCS P63 space group were systematically researched by zur Loye's group in 2023.89 With the exception of La6MgSi2S7,98 these compounds were all discovered for the first time. These compounds were synthesized by the flux method and crystallized by combining the boron chalcogen mixture method99 and the molten flux method.
The crystal structure of RE3Mg0.5SiS7 contains three types of olyhedral: [MgS6], [SiS4], and [RES8]. The 3D structure (Fig. 7a) is constructed from bi-capped trigonal prisms of [RES8], which are shared at the edges and corners to produce a ring-shaped arrangement (Fig. 7b). Isolated [SiS4] tetrahedra (Fig. 7c) are distributed throughout the structure, positioned between the ring-shaped [RES8] assemblies (Fig. 7d). Furthermore, face-sharing [MgS6] octahedra are situated centrally within each ring arrangement and interact with their neighbouring assemblies.
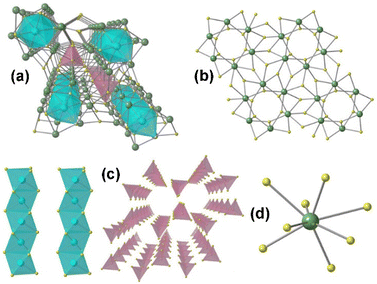 |
| Fig. 7 (a) Crystal structure of RE6MgSi2S14 from the c-axis; coordination environment of (b) 3D RE-S network, (c) 1D chains of face-sharing [MgS6] octahedra (left) and isolated [GeS4] tetrahedra (right), and (d) [RES8] polyhedron. Copyright 2023 American Chemistry Society. | |
The experimental values for RE3Mg0.5SiS7 are an Eg of 2.77 eV and an approximate deff of 0.16 × KDP at a wavelength of 1064 nm. However, the SHG activity was not tested in any other samples due to difficulties in measuring it arising from crystal colouration in other compositions.
2.2.5 Li2MgMIVSe4 (MIV = Ge, Sn).
Two new diamond-like chalcogenides with the formula of Li2MgMIVSe4 (MIV = Ge, Sn) have been reported by Pan and co-workers in 2021.90 Both of them adopt the NCS space group of Pmn21 and were synthesized by direct combination of the stoichiometric elements at 1153 K. As depicted in Fig. 8, all of the ions within the structures occupy general sites and exhibit tetrahedral coordination models. The 3D diamond-like framework of Li2MgMIVSe4 is formed by tetrahedral [(Li/Mg)Se4] [LiSe4] and [MIVSe4] ABUs through corner-sharing Se atoms (Fig. 8a and c). For both compounds, there are similar channel-like structures with a channel diameter of approximately 6 Å on the ab direction, as given in Fig. 8b and d.
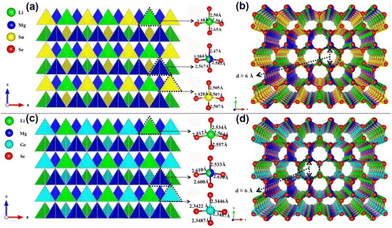 |
| Fig. 8 Crystal structure of Li2MgMIVSe4 (MIV = Ge, Sn): (a and c) 3D diamond-like structures viewed along the c direction; (b and d) 3D channel-like structures viewed along the ab plane. | |
The calculated results suggest that the d33 of Li2MgSnSe4 and Li2MgGeSe4 are 12.19 and 14.77 pm V−1, respectively, which primarily stem from the tetrahedral [MIVSe4] ABUs and are comparable to that of the widely accepted reference material AgGaS2 (d14 = 13.7 pm V−1). The calculated Eg for Li2MgSnSe4 and Li2MgGeSe4 was 2.42 and 2.44 eV, correspondingly. Notably, these two selenides represent the first series in the MI2–MII–MIV–Q4 system to feature alkali and alkaline-earth metals, thereby expanding the variety of structures found among diamond-like chalcogenides.
2.2.6 Na4MgMIII2Se6 (MIII = Si, Ge).
Na4MgMIII2Se6 (MIII = Si, Ge), with a C2 non-centrosymmetric space group, were first reported by Pan's group in 2015.91 They were discovered using traditional solid-state reactions with reaction materials Na, Mg, MIII, Ge in a molar ratio of 4
:
1
:
2
:
6 at 973 K (for Na4MgSi2Se6) and 873 K (for Na4MgGe2Se6).
Due to their similar structures, Na4MgSi2Se6 has been chosen as the representative. The Mg atoms are coordinated with six Se atoms to generate octahedral [MgSe6] ABUs, which are then connected with [Si2Se6] ABUs to build a 2D layered structure. Finally, charge-balancing Na+ cations fill the channels and interlayer spaces to further form a 3D framework (Fig. 9a). When compared to the formerly reported Na8Pb2(Si2Se6)2 (Fig. 9b),100 which also owns the same ethane-like [Si2Se6] dimers, a significant difference occurs in their different space groups (C2/m vs. C2).
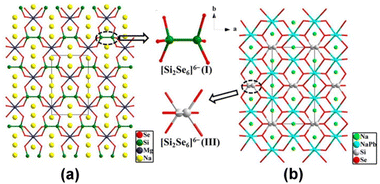 |
| Fig. 9 Crystal structures of (a) Na4MgSi2Se6 and (b) Na8Pb2(Si2Se6)2 viewed along the ab plane. Copyright 2015 American Chemistry Society. | |
Significantly, they display high power LIDTs of 9 and 7 times that of AgGaS2, moderate phase-matching deff of 0.5 and 1.3 times that of AgGaS2 within the particle size range of 150–200 μm, and wide IR transmission windows of 0.45–20 μm. These properties could potentially remove the key drawbacks, such as small LIDTs and harmful TPA, observed in commercially available IR-NLO crystals. Based on the calculation results, that calculated Δn values are close to 0.10 for Na4MgMIII2Se6 at the wavelength of 1064 nm and the charge transitions from Se 4p, MIII 3p/4p and Mg 2p play the important role for deff.
2.2.7 Cu2MgMIVQ4 (MIV = Si, Ge; Q = S, Se).
Three new diamond-like chalcogenides with the formula Cu2MgMIVQ4 (MIV = Si, Ge; Q = S, Se) have been obtained by the traditional high-temperature solid-state method by Guo and co-workers in 2013.92 All of them belong to the non-centrosymmetric space group of Pmn21 in the wurtzite-type superstructure and synthesized by direct combination of the stoichiometric elements at 1223 K. As depicted in Fig. 10, all of the ions within the structures occupy general sites and exhibit tetrahedral coordination models. Each Q2− anion is tetrahedrally coordinated with one Mg2+ cation, one M4+ cation, and two Cu+ cations, resulting in a 3D honeycomb structure. The remarkable structural characteristic of Cu2MgMIVQ4 is the introduction of a tetrahedrally coordinated Mg2+ cation to the MII sites of diamond-like chalcogenides with the formula MI2–MII–MIV–Q4 (where MI = group 11 metals and Li; MII = group 12 metals; MIV = group 14 metals; Q = S, Se).
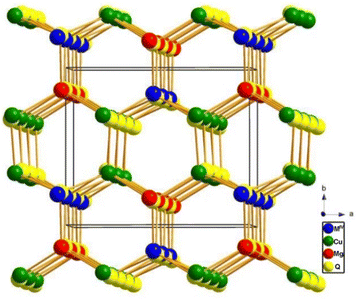 |
| Fig. 10 3D diamond-like structure of Cu2MgMIVQ4 viewed slightly along the c direction. Copyright 2013 Elsevier. | |
The experimental Eg were determined to be 3.20 and 2.36 eV for Cu2MgSiS4 and Cu2MgGeS4, respectively. Although both of them belong to the non-centrosymmetric space group, no noticeable SHG response was observed when testing a modified /Kurtz-NLO system with 1064 and 2100 nm laser radiation.
2.2.8 MIMg3MIII3Q8 (MI = Na, Cu, Ag; MIII = Al, Ga; Q = S, Se).
MIMg3MIII3Q8 (MI = Na, Cu, Ag; MIII = Al, Ga; Q = S, Se) represents a newly discovered quaternary chalcogenide family within the MI2Q–MIIQ–MIII2Q3 system. These 9 materials constitute the first series discovered in this family by Li and co-workers in 2022.93 The NaMg3Ga3Q8, NaMg3Al3Q8 and CuMg3Ga3S8 compounds were synthesized utilizing a flux method. The necessary initial reactant, Na/Cu, Mg, and Ga/Al, along with S/Se, were used in a molar ratio of 2
:
3
:
3
:
8. The synthesis process was carried out at a temperature of 1273 K. For the AgMg3Ga3Q8 and LiMg3Ga3Q8 compounds, the same method was applied; however, Ga2O3 replaced Ga as the raw material.
Given that MIMg3MIII3Q8 adopt the same non-centrosymmetric P
space group and exhibit similar structural features, AgMg3Ga3S8 has been chosen as the representative. The S atoms are coordinated with Mg, Ga and Ag atoms to form octahedral [MgS6], tetrahedral [GaS4] and triangle-planar [AgS3] ABUs (Fig. 11a). Interestingly, diverse connection modes such as vertex-, edge-, and face-sharing between these ABUs can be found in this structure. For example, [GaS4] ABUs interconnect to form 1D [GaS4]∞ and [Ga2S7]∞via corner-sharing. Meanwhile, [MgS6] ABUs connect together to construct 1D [MgS6]∞ and [Mg2S9]∞ chains via edge- or/and face-sharing (Fig. 11b). Moreover, the resulting 1D [GaS4]∞ and [MgS6]∞ chains arrange alternately by vertex-sharing, creating a distinctive windmill-like [Mg3Ga3S24] unit (Fig. 11c). Finally, the [Mg3Ga3S24] units are linked by the 1D [Ga2S7]∞ and [Mg2S9]∞ chains, and the Ag atoms are positioned in the interior of the [Mg3Ga3S24] channels, forming the 3D framework of AgMg3Ga3S8 (Fig. 11d). Compared to the [Ga6S18] unit in AgGaS2, the [Mg3Ga3S24] unit is formed by replacing three meta-position [GaS4] ABUs with three [MgS6] ABUs (Fig. 11d).
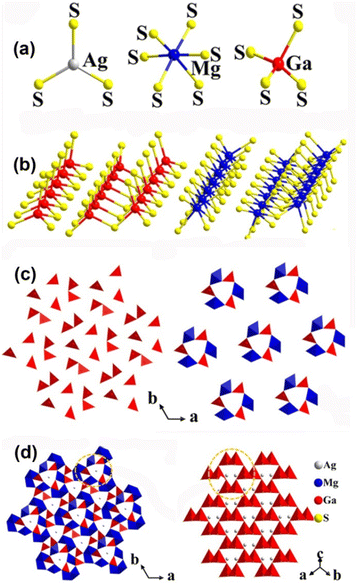 |
| Fig. 11 (a) Coordinated environment of [AgS3], [MgS6], and [GaS4] atoms; (b) 1D [GaS4], [Ga2S7], [MgS6], and [Mg2S9] chains; (c) 1D Ga–S chains and [Mg3Ga3S24] groups viewed along the ab plane; (d) 3D structure of AgMg3Ga3S8 (left) and AgGaS2 (right) with the [Ga6S18] units marked (yellow dashed circle). Copyright 2022 American Chemistry Society. | |
The experimental results suggest that NaMg3Ga3Se8 has potential as an IR-NLO candidate due to its sufficient deff (∼1 × AgGaS2@180–212 μm), wide selenide Eg (2.77 eV), suitable Δn (0.079@546 nm), and large LIDT (∼2.3 × AgGaS2). Moreover, the SHG-density maps indicate that the large deff in NaMg3Ga3Se8 is mainly provided by the NLO-active [GaSe4] ABUs. Besides, the optical and NLO parameters of other compounds are presented in Table 1.
Table 1 A summary of reported Mg-based IR-NLO materials
Compound |
Mg polyhedron |
Unit cell |
Space group |
E
g a (eV) |
Window of transparence (μm) |
d
eff
|
LIDTb |
Δnc |
PM/NPMd |
Ref. |
Experimental value.
Powder sample.
Theoretical value.
PM = phase-matchability, NPM = nonphase-matchability.
N/A = not available.
|
MgGa2Se4 |
MgSe4 |
Tetragonal |
I![[4 with combining macron]](https://www.rsc.org/images/entities/char_0034_0304.gif) |
2.96 |
1.35–12.43 |
0.9 × AgGaS2 (@2.09 μm) |
3.0 × AgGaS2 |
0.048 (@0.55 μm) |
PM |
82
|
MgSiP2 |
MgP4 |
Tetragonal |
I 2d |
2.34 |
0.53–10.3 |
3.5 × AgGaS2 (@2.05 μm) |
N/Ae |
N/A |
PM |
84
|
MgSiAs2 |
MgAs4 |
Tetragonal |
I 2d |
1.83 |
N/A |
0.6 × AgGaS2 (@2.09 μm) |
1.12 × AgGaS2 |
N/A |
PM |
85
|
Li4MgGe2S7 |
MgS4 |
Monoclinic |
Cc
|
4.12 |
N/A |
0.7 × AgGaS2 (@2.09 μm) |
7.0 × AgGaS2 |
0.035 (@1.06 μm) (exp.) |
PM |
86
|
Li2Mg2Si2S6 |
MgS6 |
Trigonal |
P31m |
3.24 |
N/A |
0.3 × KDP (@1.06 μm) |
N/A |
N/A |
N/A |
87
|
Li2Mg2Ge2S6 |
MgS6 |
Trigonal |
P31m |
3.18 |
N/A |
0.2 × KDP (@1.06 μm) |
N/A |
N/A |
N/A |
87
|
CaMg6Ga6S16 |
MgS6 |
Hexagonal |
P![[6 with combining macron]](https://www.rsc.org/images/entities/char_0036_0304.gif) |
3.54 |
0.35–20 |
0.7 × AgGaS2 (@2.09 μm) |
11.7 × AgGaS2 |
0.046 (@1.06 μm) (exp.) |
PM |
88
|
SrMg6Ga6S16 |
MgS6 |
Hexagonal |
P![[6 with combining macron]](https://www.rsc.org/images/entities/char_0036_0304.gif) |
3.51 |
0.35–20 |
0.7 × AgGaS2 (@2.09 μm) |
11.5 × AgGaS2 |
0.042 (@1.06 μm) (exp.) |
PM |
88
|
BaMg6Ga6S16 |
MgS6 |
Hexagonal |
P![[6 with combining macron]](https://www.rsc.org/images/entities/char_0036_0304.gif) |
3.50 |
0.35–20 |
0.7 × AgGaS2 (@2.09 μm) |
11.5 × AgGaS2 |
0.041 (@1.06 μm) (exp.) |
PM |
88
|
Gd6MgGe2S14 |
MgS6 |
Hexagonal |
P63 |
2.77 |
N/A |
0.2 × KDP (@1.06 μm) |
N/A |
N/A |
N/A |
89
|
Li2MgGeSe4 |
(Li/Mg)Se4 |
Orthorhombic |
Pmn21 |
2.44 (cal.) |
N/A |
d
33 = 12.19 pm V−1 (cal.) |
N/A |
0.012 (@1.06 μm) |
N/A |
90
|
Li2MgSnSe4 |
(Li/Mg)Se4 |
Orthorhombic |
Pmn21 |
2.62 |
N/A |
d
33 = 14.77 pm V−1 (cal.) |
N/A |
0.011 (@1.06 μm) |
N/A |
90
|
Na4MgSi2Se6 |
MgSe6 |
Monoclinic |
C2 |
2.53 |
0.45–20 |
0.5 × AgGaS2 (@2.09 μm) |
9.0 × AgGaS2 |
0.100 (@1 μm) |
PM |
91
|
Na4MgGe2Se6 |
MgSe6 |
Monoclinic |
C2 |
2.85 |
0.45–20 |
1.3 × AgGaS2 (@2.09 μm) |
7.0 × AgGaS2 |
0.092 (@1 μm) |
PM |
91
|
AgMg3Ga3S8 |
MgS6 |
Hexagonal |
P![[6 with combining macron]](https://www.rsc.org/images/entities/char_0036_0304.gif) |
3.59 |
N/A |
d
11 = 3.74 pm V−1 (cal.) |
N/A |
0.091 (@1.06 μm) |
N/A |
93
|
AgMg3Ga3Se8 |
MgS6 |
Hexagonal |
P![[6 with combining macron]](https://www.rsc.org/images/entities/char_0036_0304.gif) |
2.43 (cal.) |
N/A |
d
11 = 10.2 pm V−1 (cal.) |
N/A |
0.17 (@1.06 μm) |
N/A |
93
|
NaMg3Ga3S8 |
MgS6 |
Hexagonal |
P![[6 with combining macron]](https://www.rsc.org/images/entities/char_0036_0304.gif) |
3.70 |
N/A |
d
11 = 3.24 pm V−1 (cal.) |
N/A |
0.030 (@1.06 μm) |
N/A |
93
|
NaMg3Ga3Se8 |
MgSe6 |
Hexagonal |
P![[6 with combining macron]](https://www.rsc.org/images/entities/char_0036_0304.gif) |
2.77 |
N/A |
1.0 × AGS (@2.09 μm) |
2.3 × AgGaS2 |
0.079 (@0.55 μm) (exp.) |
PM |
93
|
NaMg3Al3S8 |
MgS6 |
Hexagonal |
P![[6 with combining macron]](https://www.rsc.org/images/entities/char_0036_0304.gif) |
4.20 |
N/A |
d
11 = 2.45 pm V−1 |
N/A |
0.010 (@1.06 μm) |
N/A |
93
|
NaMg3Al3Se8 |
MgS6 |
Hexagonal |
P![[6 with combining macron]](https://www.rsc.org/images/entities/char_0036_0304.gif) |
3.72 (cal.) |
N/A |
d
11 = 3.93 pm V−1 |
N/A |
0.038 (@1.06 μm) |
N/A |
93
|
Mg2In3Si2P7 |
(In/Mg)P4 |
Monoclinic |
P21 |
2.21 |
0.56–16.4 |
7.1 × AgGaS2 (@2.05 μm) |
N/A |
0.107 (@2.05 μm) |
PM |
94
|
Ba6Cu1.9Mg1.1Ge4S16 |
(Cu/Mg)S4 |
Cubic |
I 3d |
2.92 |
N/A |
2.3 × AgGaS2 (@2.09 μm) |
6.2 × AgGaS2 |
N/A |
NPM |
95
|
Ba6Cu1.9Mg1.1Sn4S16 |
(Cu/Mg)S4 |
Cubic |
I 3d |
2.4 |
N/A |
2.5 × AgGaS2 (@2.09 μm) |
2.5 × AgGaS2 |
N/A |
NPM |
96
|
2.2.9 Mg2In3Si2P7.
By implementing a “rigidity-flexibility coupling” approach, a quaternary Mg-based phosphide Mg2In3Si2P7 was successfully discovered by Ye's group in 2021.94 Millimeter-level red crystals of Mg2In3Si2P7 (2.5 × 1.5 × 0.5 mm3) were obtained by the solid-state method in sealed silica tubes using the starting materials of Mg, In, Si, P and flux BaBr2 in the molar ratio of 1.33
:
1.5
:
1
:
3.5
:
2.67 at 1073 K.
The Mg2In3Si2P7 crystal structure displays a diamond-like pattern, which is created by the vertex-sharing [(Mg/In)P4], [(In/Si)P4] and [SiP4] ABUs (Fig. 12a). The tetrahedral [SiP4] ABUs are integrated into the 12-MR rings of [(Mg/In)6P6], thereby generating 1D [(Mg/In)6SiP16]∞ chains (Fig. 12a), and the tetrahedral [(In/Si)P4] ABUs interlink through corner-sharing and form zigzag 1D [(In/Si)P3]∞ chains. These two kinds of 1D chains are interconnected to build 2D layers (Fig. 12b), which are arranged in an ABAB fashion, giving rise to a 3D polar structure (Fig. 12a). Additionally, Mg2In3Si2P7 exhibits a hexagonal close-packed structure that closely resembles wurtzite (Fig. 12c), which was confirmed by the experimental results of the selected area electron diffraction patterns (Fig. 12d).
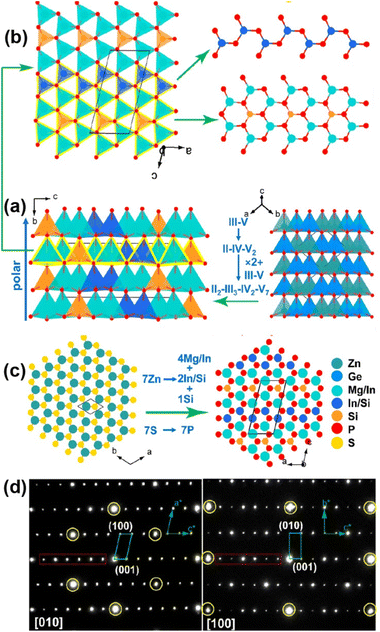 |
| Fig. 12 (a) Structural evolution from ZnGeP2 (right) to Mg2In3Si2P7 (left); (b) single 2D tetrahedral-stacking layer composed of 1D [(Mg/In)6SiP16] and [In/SiP3] chains in Mg2In3Si2P7. (c) Structural evolution from wurtzite ZnS (left) to Mg2In3Si2P7 (right); (d) electron diffraction patterns of Mg2In3Si2P7 along the [010] and [100] zone axes. Copyright 2021 American Chemistry Society. | |
Noticeably, Mg2In3Si2P7 has achieved rare coexistence of huge deff (2 × ZnGeP2 and 7.1 × AgGaS2, granularity range 150–212 μm), a sufficient Eg of 2.21 eV, a large Δn of 0.107, and a wide IR transparent window of 0.56–16.4 μm. Theoretical calculations have revealed that the colossal deff and large Δn can be attributed to the favorable arrangement of tetrahedral [InP4] and [SiP4] ABUs. These findings not only open up a new route for sophisticated IR-NLO crystal design but also have the potential to inspire more discoveries in the quaternary diamond-like families.
2.3 Quinary Mg-based chalcogenides
2.3.1 Ba6Cu1.9Mg1.1Ge4S16 and Ba6Cu1.94Mg1.06Sn4S16.
Ba6Cu1.9Mg1.1Ge4S16 and Ba6Cu1.94Mg1.06Sn4S16 were reported by Wang's group in 2021 and 2022, respectively.95,96 Both of them can be obtained through the traditional high-temperature solid-state method using the stoichiometric amounts of the reagents at 1073 K, with the difference being that the former requires the use of KI salt as the flux, while the latter does not.
Ba6Cu1.9Mg1.1Ge4S16 and Ba6Cu1.94Mg1.06Sn4S16 are both members of the MII6MI4MIV4Q16 (MII = Sr, Ba; MI = Li, Cu, Ag; MIV = Ge, Sn; Ch = S, Se) family.101,102 These two compounds feature mixed locations at the Li/Cu/Ag atomic sites, where MI metals are replaced by MII metals. Since both compounds belong to the same non-centrosymmetric I
3d space group and exhibit similar structural features, Ba6Cu1.9Mg1.1Ge4S16 has been chosen as the representative. The 3D framework is formed by tetrahedral [(Cu/Mg)S4] and [GeS4] ABUs through corner-sharing S atoms, where the charge-balanced Ba2+ packing in the empty spaces. The polyhedral and ball–stick modes of Ba6Cu1.9Mg1.1Ge4S16 are displayed in Fig. 13a and b, respectively. Besides, the coordination environment between the tetrahedral [(Cu/Mg)S4] and [GeS4] ABUs is highlighted in Fig. 13c.
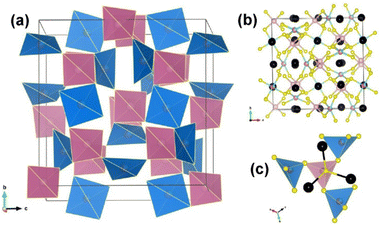 |
| Fig. 13 Crystal structural of Ba6Cu1.9Mg1.1Sn4S16: (a) polyhedral packing model; (b) ball-and-stick model; (c) coordination environment of [(Cu/Mg)S4] (pink) and [SnS4] (blue) tetrahedra. Black: Ba; red: Mg; blue: Cu; pink: Sn; yellow: S. Copyright 2021 American Chemistry Society. | |
The experimental Eg was determined to be 2.92 and 2.24 eV for Ba6Cu1.9Mg1.1Ge4S16 and Ba6Cu1.94Mg1.06Sn4S16, respectively. Through the placement of monovalent Cu+ with divalent Mg2+, the optical properties can be shifted to promote a good balance between deff and LIDT. For instance, Ba6Cu1.9Mg1.1Ge4S16 possesses a strong non-phase-matching deff and a high LIDT of 2.3 × AgGaS2 and 6.2 × AgGaS2 within the particle size range of 28–55 μm, respectively. Theoretical calculations confirmed that the [(Cu/Mg)S4] and [GeS4] ABUs are the major role of the SHG responses.
3. Conclusions and perspectives
Research interest in new IR-NLO candidates has stimulated the rapid development of new NCS Mg-based chalcogenides and pnictides. In this review, we presented a brief introduction to the solid-state synthesis, structural design, and NLO properties together with the structure–property relationship of the recently reported Mg-based IR-NLO materials. The distribution of Eg (eV) in relation to deff (× AgGaS2) and detailed performance comparison for previously reported Mg-based IR-NLO materials are illustrated in Fig. 14, 15 and Table 1, respectively. From this, certain characteristics can be inferred:
(i) The currently reported Mg-based IR-NLO materials (including 36 chalcogenides and 4 pnictides) are mainly quaternary compounds (87.5%), with a small amount of ternary (7.5%) and pentagonal (5%) compounds (Fig. 14a and b). Moreover, they mainly belong to the highly symmetrical crystal systems, such as the hexagonal space group of P63 (27.5%) and P
(30%) (Fig. 14c).
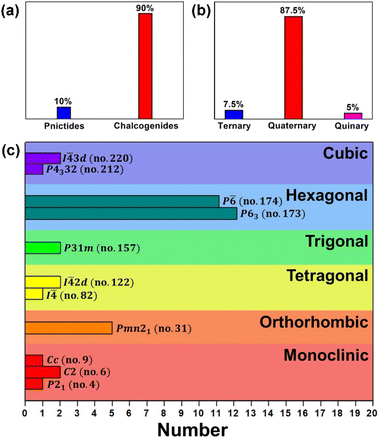 |
| Fig. 14 Distributions of the Mg-based IR-NLO materials according to (a) material type, (b) chemical component and (c) the space groups in different crystal systems. | |
(ii) The Mg-based compounds mentioned above are synthesized using traditional high-temperature solid-state methods. Some of them require the use of fluxes to assist in the reaction, including Bi, NaCl, NaI, KI, CsCl, CaCl2, BaCl2, and BaBr2. Furthermore, it is worth noting the synthesis of RE6MgSi2S14, which utilizes a boron chalcogen mixture method. It is anticipated that this method can be expanded to other chalcogenide systems, allowing for the exploration of further captivating physical properties.
(iii) As displayed in Fig. 15, most of them are stronger than commercial AgGaS2 (Eg = 2.56 eV and deff = 1 × AgGaS2). Remarkably, quaternary pnictide Mg2In3Si2P7 exhibits the largest deff (7.1 × AgGaS2) due to the most favorable combination and arrangement of ABUs, while NaMg3Al3S8 possesses the widest Eg of 4.20 eV in this system.
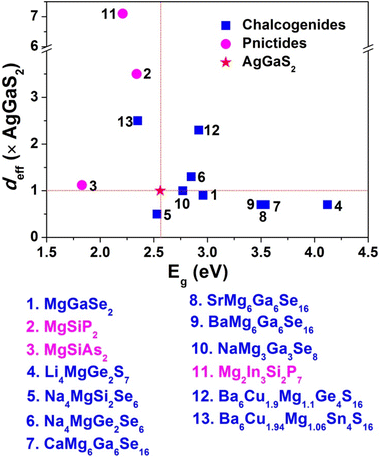 |
| Fig. 15 Comparison of the deff (× AGS) and Eg (eV) for selected Mg-based materials with IR NLO properties. | |
(iv) Due to the incompatibility of optical parameters, there are currently no reports of IR-NLO Mg-based materials that can achieve a balance between large Eg (>3.0 eV) and strong phase-matching deff (>1.0 × AgGaS2).
Many distinguished researchers have made significant contributions in exploring and verifying the potential of Mg-based IR NLO materials. However, more research is required to fully uncover the potential of these materials. Some possible avenues for further progress are outlined below:
(1) The pnictide system shows promise for further exploration. However, only a few Mg-based pnictides have been reported, and their usability has been limited by their narrow Eg. To increase the Eg, an effective solution could be to introduce high electronegativity “structure scissors” ions, such as alkali metals, alkaline earth metals, and halogens.
(2) Efforts are underway to synthesize high-performance Mg-based IR-NLO compounds by combining other ABUs. Currently, distorted [MQ4] tetrahedra (M = group 13 and 14 metal elements) are the most commonly used second ABUs, but it would be worthwhile to experiment with introducing other ABUs, such as, lone-pair-cation-based [MQn] ABUs, distorted [REQn] ABUs, or mixed-anion [MOxQy] ABUs.
(3) The theoretical calculation system for IR-NLO materials requires further study. Researchers can gain crucial properties through first-principles calculations, reducing the experimental blind spots and deepening the understanding of structure–activity relationships. Due to the development of anionic group theory in the research of oxide systems, there is still a significant difference between the calculation results and single crystal tests when applied to chalcogenides and pnictides. Therefore, there is an urgent need to establish a theoretical system that is suitable for IR-NLO materials.
(4) The study of large-sized crystal growth still requires further enhancement. After identifying crystals with exceptional properties, it is crucial for their scientific and technological development to investigate the possibility of growing them into large-sized crystals for commercial purposes.
Author contributions
Jia-Xiang Zhang: investigation and writing – original draft. Mao-Yin Ran: investigation and formal analysis. Xin-Tao Wu: conceptualization and formal analysis. Hua Lin: supervision, conceptualization and writing – review and editing. Qi-Long Zhu: supervision and writing – review and editing.
Conflicts of interest
There are no conflicts to declare.
Acknowledgements
This work was sponsored by the National Natural Science Foundation of China (21771179), Fujian Science & Technology Innovation Laboratory for Optoelectronic Information of China (2021ZR118), and the Natural Science Foundation of Fujian Province (2022L3092 and 2023H0041).
References
- U. Keller, Recent developments in compact ultrafast lasers, Nature, 2003, 424, 831–838 CrossRef CAS PubMed
.
-
T. Schneider, Nonlinear optics in telecommunications, Springer Science & Business Media, 2004 Search PubMed
.
-
D. N. Nikogosyan, Nonlinear Optical Crystals: A Complete Survey, Springer, New York, 1st edn, 2005 Search PubMed
.
-
F. J. Duarte, Tunable Laser Applications, CRC Press, Boca Raton, 2008 Search PubMed
.
- V. A. Serebryakov, E. V. Boiko, N. N. Petrishchev and A. V. Yan, Medical applications of mid-TR lasers: problems and prospects, J. Opt. Technol., 2010, 77, 6–17 CrossRef CAS
.
-
Structure-Property Relationships in Nonlinear Optical Crystals II The IR Region, in Structure and Bonding, ed. X.-T. Wu and L. Chen, Spring, New York, Series ed. D. M. Mingos, 2012 Search PubMed
.
- I. Chung and M. G. Kanatzidis, Metal Chalcogenides: A Rich Source of Nonlinear Optical Materials, Chem. Mater., 2013, 26, 849–869 CrossRef
.
- V. Petrov, Frequency down-conversion of solid-state laser sources to the mid-infrared spectral range using non-oxide nonlinear crystals, Prog. Quantum Electron., 2015, 42, 1–106 CrossRef
.
- A. Autere, H. Jussila, Y. Dai, Y. Wang, H. Lipsanen and Z. Sun, Nonlinear Optics with 2D Layered Materials, Adv. Mater., 2018, 30, e1705963 CrossRef PubMed
.
- P. S. Halasyamani and J. M. Rondinelli, The must-have and nice-to-have experimental and computational requirements for functional frequency doubling deep-UV crystals, Nat. Commun., 2018, 9, 2972 CrossRef PubMed
.
-
A. Newell, Nonlinear optics, CRC Press, Boca Raton, 2018 Search PubMed
.
- C. Wu, G. Yang, M. G. Humphrey and C. Zhang, Recent advances in ultraviolet and deep-ultraviolet second-order nonlinear optical crystals, Coord. Chem. Rev., 2018, 375, 459–488 CrossRef CAS
.
- M. Mutailipu, M. Zhang, Z. Yang and S. Pan, Targeting the Next Generation of Deep-Ultraviolet Nonlinear Optical Materials: Expanding from Borates to Borate Fluorides to Fluorooxoborates, Acc. Chem. Res., 2019, 52, 791–801 CrossRef CAS PubMed
.
- J. Chen, C. L. Hu, F. Kong and J. G. Mao, High-Performance Second-Harmonic-Generation (SHG) Materials: New Developments and New Strategies, Acc. Chem. Res., 2021, 54, 2775–2783 CrossRef CAS PubMed
.
- W. Huang, S. Zhao and J. Luo, Recent Development of Non-π-Conjugated Deep Ultraviolet Nonlinear Optical Materials, Chem. Mater., 2021, 34, 5–28 CrossRef
.
- X. Chen and K. M. Ok, Metal oxyhalides: an emerging family of nonlinear optical materials, Chem. Sci., 2022, 13, 3942–3956 RSC
.
- L. Kang and Z. Lin, Deep-ultraviolet nonlinear optical crystals: concept development and materials discovery, Light: Sci. Appl., 2022, 11, 201 CrossRef CAS PubMed
.
- Y. Li, J. Luo and S. Zhao, Local Polarity-Induced Assembly of Second-Order Nonlinear Optical Materials, Acc. Chem. Res., 2022, 55, 3460–3469 CrossRef CAS PubMed
.
- Y. Xia, C. Chen, D. Tang and B. Wu, New nonlinear optical crystals for UV and VUV harmonic generation, Adv. Mater., 1995, 7, 79–81 CrossRef CAS
.
- C. Chen, B. Wu, A. Jiang and G. You, A new-type ultraviolet SHG crystal β-BaB2O4, Sci. China, Ser. B: Chem., Life Sci., Earth Sci., 1985, 28, 235–243 Search PubMed
.
- C. T. Chen, Y. C. Wu, A. D. Jiang, B. C. Wu, G. M. You, R. K. Li and S. J. Lin, New nonlinear-optical crystal: LiB3O5, J. Opt. Soc. Am. B, 1989, 6, 616–621 CrossRef CAS
.
- P. S. Peercy, “Soft” mode and coupled modes in the ferroelectric phase of KDP, Solid State Commun., 1975, 16, 439–442 CrossRef CAS
.
- D. Z. Shen and C. Huang, A new nonlinear optical-crystal KTP, Prog. Cryst. Growth Charact., 1985, 11, 269–274 CrossRef CAS
.
- A. Harasaki and K. Kato, New Data on the Nonlinear Optical Constant, Phase-Matching,
and Optical Damage of AgGaS2, Jpn. J. Appl. Phys., 1997, 36, 700–703 CrossRef CAS
.
- G. C. Catella, L. R. Shiozawa, J. R. Hietanen, R. C. Eckardt, R. K. Route, R. S. Feigelson, D. G. Cooper and C. L. Marquardt, Mid-IR absorption in AgGaSe2 optical parametric oscillator crystals, Appl. Opt., 1993, 32, 3948–3951 CrossRef CAS PubMed
.
- G. D. Boyd, E. Buehler and F. G. Storz, Linear and nonlinear optical properties of ZnGeP2 and CdSe, Appl. Phys. Lett., 1971, 18, 301–304 CrossRef CAS
.
- M. C. Ohmer, R. Pandey and B. H. Bairamov, Emergence of chalcopyrites as nonlinear optical materials, MRS Bull., 1998, 23, 16–20 CrossRef CAS
.
- L. Bai, Z. Lin, Z. Wang, C. Chen and M. H. Lee, Mechanism of linear and nonlinear optical effects of chalcopyrite AgGaX2 (X=S, Se, and Te) crystals, J. Chem. Phys., 2004, 120, 8772–8778 CrossRef CAS PubMed
.
- L. Kang, M. Zhou, J. Yao, Z. Lin, Y. Wu and C. Chen, Metal Thiophosphates with Good Mid-infrared Nonlinear Optical Performances: A First-Principles Prediction and Analysis, J. Am. Chem. Soc., 2015, 137, 13049–13059 CrossRef CAS PubMed
.
- S.-P. Guo, Y. Chi and G.-C. Guo, Recent achievements on middle and far-infrared second-order nonlinear optical materials, Coord. Chem. Rev., 2017, 335, 44–57 CrossRef CAS
.
- F. Liang, L. Kang, Z. Lin, Y. Wu and C. Chen, Analysis and prediction of mid-IR nonlinear optical metal sulfides with diamond-like structures, Coord. Chem. Rev., 2017, 333, 57–70 CrossRef CAS
.
- J.-R. Xiao, S.-H. Yang, F. Feng, H.-G. Xue and S.-P. Guo, A review of the structural chemistry and physical properties of metal chalcogenide halides, Coord. Chem. Rev., 2017, 347, 23–47 CrossRef CAS
.
- M.-M. Chen, H.-G. Xue and S.-P. Guo, Multinary metal chalcogenides with tetrahedral structures for second-order nonlinear optical, photocatalytic, and photovoltaic applications, Coord. Chem. Rev., 2018, 368, 115–133 CrossRef CAS
.
- P. Gong, F. Liang, L. Kang, X. Chen, J. Qin, Y. Wu and Z. Lin, Recent advances and future perspectives on infrared nonlinear optical metal halides, Coord. Chem. Rev., 2019, 380, 83–102 CrossRef CAS
.
- L. Kang, F. Liang, X. Jiang, Z. Lin and C. Chen, First-Principles Design and Simulations Promote the Development of Nonlinear Optical Crystals, Acc. Chem. Res., 2020, 53, 209–217 CrossRef CAS PubMed
.
- Q. G. Yue, W. B. Wei, H. Chen, X. T. Wu, H. Lin and Q. L. Zhu, Salt-inclusion chalcogenides: an emerging class of IR nonlinear optical materials, Dalton Trans., 2020, 49, 14338–14343 RSC
.
- L. Gao, J. Huang, S. Guo, Z. Yang and S. Pan, Structure-property survey and computer-assisted screening of mid-infrared nonlinear optical chalcohalides, Coord. Chem. Rev., 2020, 421, 213379 CrossRef CAS
.
- H. D. Yang, M. Y. Ran, W. B. Wei, X. T. Wu, H. Lin and Q. L. Zhu, The Rise of Infrared Nonlinear Optical Pnictides: Advances and Outlooks, Chem. – Asian J., 2021, 16, 3299–3310 CrossRef CAS PubMed
.
- H. Chen, M.-Y. Ran, W.-B. Wei, X.-T. Wu, H. Lin and Q.-L. Zhu, A comprehensive review on metal chalcogenides with three-dimensional frameworks for infrared nonlinear optical applications, Coord. Chem. Rev., 2022, 470, 214706 CrossRef CAS
.
- H.-D. Yang, M.-Y. Ran, W.-B. Wei, X.-T. Wu, H. Lin and Q.-L. Zhu, Recent advances in IR nonlinear optical chalcogenides with well-balanced comprehensive performance, Mater. Today Phys., 2023, 35, 101127 CrossRef CAS
.
- H. Lin, W.-B. Wei, H. Chen, X.-T. Wu and Q.-L. Zhu, Rational design of infrared nonlinear optical chalcogenides by chemical substitution, Coord. Chem. Rev., 2020, 406, 213150 CrossRef CAS
.
- G. Zou and K. M. Ok, Novel ultraviolet (UV) nonlinear optical (NLO) materials discovered by chemical substitution-oriented design, Chem. Sci., 2020, 11, 5404–5409 RSC
.
- M.-Y. Ran, A.-Y. Wang, W.-B. Wei, X.-T. Wu, H. Lin and Q.-L. Zhu, Recent progress in the design of IR nonlinear optical materials by partial chemical substitution: structural evolution and performance optimization, Coord. Chem. Rev., 2023, 481, 215059 CrossRef CAS
.
- H. Lin, L. Chen, L. J. Zhou and L. M. Wu, Functionalization based on the substitutional flexibility: strong middle IR nonlinear optical selenides AXII4XIII5Se12, J. Am. Chem. Soc., 2013, 135, 12914–12921 CrossRef CAS PubMed
.
- S. P. Guo, X. Cheng, Z. D. Sun, Y. Chi, B. W. Liu, X. M. Jiang, S. F. Li, H. G. Xue, S. Deng, V. Duppel, J. Kohler and G. C. Guo, Large Second Harmonic Generation (SHG) Effect and High Laser-Induced Damage Threshold (LIDT) Observed Coexisting in Gallium Selenide, Angew. Chem., Int. Ed., 2019, 58, 8087–8091 CrossRef CAS PubMed
.
- K. Wu, Y. Chu, Z. Yang and S. Pan, A2SrMIVS4 (A=Li, Na; MIV=Ge, Sn) concurrently exhibiting wide bandgaps and good nonlinear optical responses as new potential infrared nonlinear optical materials, Chem. Sci., 2019, 10, 3963–3968 RSC
.
- H.-D. Yang, M.-Y. Ran, S.-H. Zhou, X.-T. Wu, H. Lin and Q.-L. Zhu, Rational Design via Dual-Site Aliovalent Substitution Leads to an Outstanding IR Nonlinear Optical Material with Well-Balanced Comprehensive Properties, Chem. Sci., 2022, 13, 10725–10733 RSC
.
- Q. Q. Liu, X. Liu, L. M. Wu and L. Chen, SrZnGeS4: A Dual-Waveband Nonlinear Material With A Transparency Spanning UV-Vis and Far-IR Spectral Regions, Angew. Chem., Int. Ed., 2022, 61, e202205587 CAS
.
- M.-M. Chen, S.-H. Zhou, W.-B. Wei, X.-T. Wu, H. Lin and Q.-L. Zhu, Phase Matchability Transformation in the Infrared Nonlinear Optical Materials with Diamond-Like Frameworks, Adv. Opt. Mater., 2022, 10, 2102123 CrossRef CAS
.
- P. Wang, Y. Chu, A. Tudi, C. Xie, Z. Yang, S. Pan and J. Li, The Combination of Structure Prediction and Experiment for the Exploration of Alkali-Earth Metal-Contained Chalcopyrite-Like IR Nonlinear Optical Material, Adv. Sci., 2022, 9, 2106120 CrossRef CAS PubMed
.
- W. Cai, A. Abudurusuli, C. Xie, E. Tikhonov, J. Li, S. Pan and Z. Yang, Toward the Rational Design of Mid-Infrared Nonlinear Optical Materials with Targeted Properties via a Multi-Level Data–Driven Approach, Adv. Funct. Mater., 2022, 32, 2200231 CrossRef CAS
.
- H. Chen, M.-Y. Ran, S.-H. Zhou, X.-T. Wu, H. Lin and Q.-L. Zhu, Simple yet extraordinary: super-polyhedra-built 3D chalcogenide framework of Cs5Ga9S16 with excellent infrared nonlinear optical performance, Chin. Chem. Lett., 2023, 34, 107838 CrossRef CAS
.
- M.-Y. Li, Z. Ma, B. Li, X.-T. Wu, H. Lin and Q.-L. Zhu, HgCuPS4: An Exceptional Infrared Nonlinear Optical Material with Defect Diamond-like Structure, Chem. Mater., 2020, 32, 4331–4339 CrossRef CAS
.
- H. Chen, Y.-Y. Li, B.-X. Li, P.-F. Liu, H. Lin, Q.-L. Zhu and X.-T. Wu, Salt-Inclusion Chalcogenide [Ba4Cl2][ZnGa4S10]: Rational Design of an IR Nonlinear Optical Material with Superior Comprehensive Performance Derived from AgGaS2, Chem. Mater., 2020, 32, 8012–8019 CrossRef CAS
.
- H. Chen, W.-B. Wei, H. Lin and X.-T. Wu, Transition-metal-based chalcogenides: A rich source of infrared nonlinear optical materials, Coord. Chem. Rev., 2021, 448, 214154 CrossRef CAS
.
- C. Li, X. Meng, Z. Li and J. Yao, Hg-based chalcogenides: An intriguing class of infrared nonlinear optical materials, Coord. Chem. Rev., 2022, 453, 214328 CrossRef CAS
.
- W. Zhou, J. Wu, W. Liu and S.-P. Guo, Ag-based chalcogenides and derivatives as promising infrared nonlinear optical materials, Coord. Chem. Rev., 2023, 477, 214950 CrossRef CAS
.
- Y.-F. Shi, Y.-K. Chen, M.-C. Chen, L.-M. Wu, H. Lin, L.-J. Zhou and L. Chen, Strongest Second Harmonic Generation in the Polar R3MTQ7 Family: Atomic Distribution Induced Nonlinear Optical Cooperation, Chem. Mater., 2015, 27, 1876–1884 CrossRef CAS
.
- Q. G. Yue, S. H. Zhou, B. Li, X. T. Wu, H. Lin and Q. L. Zhu, Quaternary Noncentrosymmetric Rare-Earth Sulfides Ba4RE2Cd3S10 (RE=Sm, Gd, or Tb): A Joint Experimental and Theoretical Investigation, Inorg. Chem., 2022, 61, 1797–1804 CrossRef CAS PubMed
.
- Z. X. Chen, C. Y. Zhao, X. H. Li, W. D. Yao, W. Liu and S. P. Guo, KREP2Se6 (RE=Sm, Gd, Tb): The First Rare-Earth Selenophosphates with Remarkable Nonlinear Optical Activities Realized by Synergistic Effect of RE- and P-Based Motifs, Small, 2023, 19, e2206910 CrossRef PubMed
.
- M.-Y. Ran, S.-H. Zhou, W.-B. Wei, B.-X. Li, X.-T. Wu, H. Lin and Q.-L. Zhu, Rational Design of a Rare-Earth Oxychalcogenide Nd3[Ga3O3S3][Ge2O7] with Superior Infrared Nonlinear Optical Performance, Small, 2023, 19, 2300248 CrossRef CAS PubMed
.
- M. C. Chen, L. M. Wu, H. Lin, L. J. Zhou and L. Chen, Disconnection enhances the second harmonic generation response: synthesis and characterization of Ba23Ga8Sb2S38, J. Am. Chem. Soc., 2012, 134, 6058–6060 CrossRef CAS PubMed
.
- H. Lin, Y.-Y. Li, M.-Y. Li, Z. Ma, L.-M. Wu, X.-T. Wu and Q.-L. Zhu, Centric-to-acentric structure transformation induced by a stereochemically active lone pair: a new insight for design of IR nonlinear optical materials, J. Mater. Chem. C, 2019, 7, 4638–4643 RSC
.
- M.-Y. Li, B. Li, H. Lin, Z. Ma, L.-M. Wu, X.-T. Wu and Q.-L. Zhu, Sn2Ga2S5: A Polar Semiconductor with Exceptional Infrared Nonlinear Optical Properties Originating from the Combined Effect of Mixed Asymmetric Building Motifs, Chem. Mater., 2019, 31, 6268–6275 CrossRef CAS
.
- Y. Xiao, M.-M. Chen, Y.-Y. Shen, P.-F. Liu, H. Lin and Y. Liu, A3Mn2Sb3S8 (A=K and Rb): A new type of multifunctional infrared nonlinear optical material based on unique three-dimensional open frameworks, Inorg. Chem. Front., 2021, 8, 2835–2843 RSC
.
- M. Yan, H.-G. Xue and S.-P. Guo, Recent Achievements in Lone-Pair Cation-Based Infrared SecondOrder Nonlinear Optical Materials, Cryst. Growth Des., 2021, 21, 698–720 CrossRef CAS
.
- M.-M. Chen, Z. Ma, B.-X. Li, W.-B. Wei, X.-T. Wu, H. Lin and Q.-L. Zhu, M2As2Q5 (M=Ba, Pb; Q=S, Se): a source of infrared nonlinear optical materials with excellent overall performance activated by multiple discrete arsenate anions, J. Mater. Chem. C, 2021, 9, 1156–1163 RSC
.
- C. Liu, S.-H. Zhou, Y. Xiao, C. Zhang, H. Lin and Y. Liu, Aliovalent-cation-substitution-induced structure transformation: a new path toward high-performance IR nonlinear optical materials, J. Mater. Chem. C, 2021, 9, 15407–15414 RSC
.
- X.-H. Li, Z.-H. Shi, M. Yang, W. Liu and S.-P. Guo, Sn7Br10S2: The First Ternary Halogen-Rich Chalcohalide Exhibiting a Chiral Structure and Pronounced Nonlinear Optical Properties, Angew. Chem., Int. Ed., 2022, 61, e202115871 CAS
.
- M.-M. Chen, S.-H. Zhou, W. Wei, M.-Y. Ran, B. Li, X.-T. Wu, H. Lin and Q.-L. Zhu, RbBiP2S6: A Promising IR Nonlinear Optical Material with a Giant Second-Harmonic Generation Response Designed by Aliovalent Substitution, ACS Mater. Lett., 2022, 4, 1264–1269 CrossRef CAS
.
- H.-J. Zhao, H.-D. Yang, P.-F. Liu and H. Lin, From Cc to P63mc: Structural Variation in La3S2Cl2[SbS3] and La3OSCl2[SbS3] Induced by the Isovalent Anion Substitution, Cryst. Growth Des., 2022, 22, 1437–1444 CrossRef CAS
.
- J. K. Harada, N. Charles, K. R. Poeppelmeier and J. M. Rondinelli, Heteroanionic Materials by Design: Progress Toward Targeted Properties, Adv. Mater., 2019, 31, 1805295 CrossRef PubMed
.
- Y.-Y. Li, W.-J. Wang, H. Wang, H. Lin and L.-M. Wu, Mixed-Anion Inorganic Compounds: A Favorable Candidate for Infrared Nonlinear Optical Materials, Cryst. Growth Des., 2019, 19, 4172–4192 CrossRef CAS
.
- M.-Y. Ran, Z. Ma, H. Chen, B.-X. Li, X.-T. Wu, H. Lin and Q.-L. Zhu, Partial Isovalent Anion Substitution to Access Remarkable Second-Harmonic Generation Response: A Generic and Effective Strategy for Design of Infrared Nonlinear Optical Materials, Chem. Mater., 2020, 32, 5890–5896 CrossRef CAS
.
- Y. F. Shi, W. B. Wei, X. T. Wu, H. Lin and Q. L. Zhu, Recent progress in oxychalcogenides as IR nonlinear optical materials, Dalton Trans., 2021, 50, 4112–4118 RSC
.
- M.-Y. Ran, S.-H. Zhou, B. Li, W. Wei, X.-T. Wu, H. Lin and Q.-L. Zhu, Enhanced Second-Harmonic-Generation Efficiency and Birefringence in Melillite Oxychalcogenides Sr2MGe2OS6 (M=Mn, Zn, and Cd), Chem. Mater., 2022, 34, 3853–3861 CrossRef CAS
.
- Y.-F. Shi, Z. Ma, B.-X. Li, X. Wu, H. Lin and Q.-L. Zhu, Phase matching achieved by isomorphous substitution in IR nonlinear optical material Ba2SnSSi2O7 with an undiscovered [SnO4S] functional motif, Mater. Chem. Front., 2022, 6, 3054–3061 RSC
.
- Y. Zhang, H. Wu, Z. Hu and H. Yu, Oxychalcogenides: A Promising Class of Materials for Nonlinear Optical Crystals with Mixed-Anion Groups, Chem. – Eur. J., 2023, 29, e202203597 CAS
.
- Y. F. Shi, S. H. Zhou, B. Li, Y. Liu, X. T. Wu, H. Lin and Q. L. Zhu, Ba5Ga2SiO4S6: a Phase-Matching Nonlinear Optical Oxychalcogenide Design via Structural Regulation Originated from Heteroanion Introduction, Inorg. Chem., 2023, 62, 464–473 CrossRef CAS PubMed
.
- J. Xu and K. Wu, Comprehensive review on multiple mixed-anion ligands, physicochemical performances and application prospects in metal oxysulfides, Coord. Chem. Rev., 2023, 486, 215139 CrossRef
.
- H.-D. Yang, S.-H. Zhou, M.-Y. Ran, X.-T. Wu, H. Lin and Q.-L. Zhu, Melillite oxychalcogenide Sr2FeGe2OS6: a phase-matching IR nonlinear optical material realized by isomorphous substitution, Inorg. Chem. Front., 2023, 10, 2030–2038 RSC
.
- P. Wang, Y. Chu, A. Tudi, C. Xie, Z. Yang, S. Pan and J. Li, The Combination of Structure Prediction and Experiment for the Exploration of Alkali-Earth Metal-Contained Chalcopyrite-Like IR Nonlinear Optical Material, Adv. Sci., 2022, 9, 2106120 CrossRef CAS
.
- J. Xiao, S. Zhu, B. Zhao, B. Chen, H. Liu and Z. He, Computational assessment of promising mid-infrared nonlinear optical materials Mg–IV–V2 (IV=Si, Ge, Sn; V=P, As): a first principles study, Mater. Res. Express, 2018, 5, 035907 CrossRef
.
- K. E. Woo, J. Wang, K. Wu, K. Lee, J.-A. Dolyniuk, S. Pan and K. Kovnir, Mg-Si-As: An Unexplored System with Promising Nonlinear Optical Properties, Adv. Funct. Mater., 2018, 28, 1801589 CrossRef
.
- J. Chen, Q. N. Wu, H. Tian, X. Jiang, F. Xu, X. Zhao, Z. Lin, M. Luo and N. Ye, Uncovering a Vital Band Gap Mechanism of Pnictides, Adv. Sci., 2022, 9, 2105787 CrossRef CAS PubMed
.
- A. Abudurusuli, J. Huang, P. Wang, Z. Yang, S. Pan and J. Li, Li4MgGe2S7:TheFirst Alkali and Alkaline-Earth Diamond-Like Infrared Nonlinear Optical Material with Exceptional Large Band Gap, Angew. Chem., Int. Ed., 2021, 60, 24131–24136 CrossRef CAS PubMed
.
- A. T. Barton, M. Liang, A. J. Craig, W. Zhang, S. S. Stoyko, A. N. Radzanowski, D. Fingerlow, P. S. Halasyamani, J. H. MacNeil and J. A. Aitken, Li2Mg2Si2S6 and Li2Mg2Ge2S6: Two nonlinear optical sulfides featuring a unique, polar trigonal structure incorporating ethane-like anions, Z. Anorg. Allg. Chem., 2022, 648, e202200071 CrossRef CAS
.
- J. Chen, Y. Zhang, H. Wu, Z. Hu, J. Wang, Y. Wu and H. Yu, AeMg6Ga6S16 (Ae=Ca, Sr, Ba): The First Double Alkaline Earth Metal Chalcogenides with Excellent Performances, Adv. Opt. Mater., 2023, 11, 2202147 CrossRef CAS
.
- A. A. King, L. S. Breton, G. Morrison, M. D. Smith, M. Liang, P. S. Halasyamani and H.-C. zur Loye, Crystal Structures and Property Measurements of Rare Earth Magnesium Thiosilicates Synthesized via Flux Crystal Growth Utilizing the Boron Chalcogen Mixture (BCM) Method, Inorg. Chem., 2023, 62, 7446–7452 CrossRef CAS PubMed
.
- H. Gao, K. Zhang, A. Abudurusuli, C. Bai, Z. Yang, K. Lai, J. Li and S. Pan, Syntheses, Structures and Properties of Alkali and Alkaline Earth Metal Diamond-Like Compounds Li2MgMSe4 (M=Ge, Sn), Materials, 2021, 14, 6166 CrossRef CAS PubMed
.
- K. Wu, Z. Yang and S. Pan, Na4MgM2Se6 (M=Si, Ge): The First Noncentrosymmetric Compounds with Special Ethane-like [M2Se6]6− Units Exhibiting Large Laser Damage Thresholds, Inorg. Chem., 2015, 54, 10108–10110 CrossRef CAS PubMed
.
- B.-W. Liu, M.-J. Zhang, Z.-Y. Zhao, H.-Y. Zeng, F.-K. Zheng, G.-C. Guo and J.-S. Huang, Synthesis, structure, and optical properties of the quaternary diamond-like compounds I2–II–IV–VI4 (I=Cu; II=Mg; IV=Si, Ge; VI=S, Se), J. Solid State Chem., 2013, 204, 251–256 CrossRef CAS
.
- L. Luo, L. Wang, J. Chen, J. Zhou, Z. Yang, S. Pan and J. Li, AIBII3CIII3QVI8: A New Family for the Design of Infrared Nonlinear Optical Materials by Coupling Octahedra and Tetrahedra Units, J. Am. Chem. Soc., 2022, 144, 21916–21925 CrossRef CAS PubMed
.
- J. Chen, H. Chen, F. Xu, L. Cao, X. Jiang, S. Yang, Y. Sun, X. Zhao, C. Lin and N. Ye, Mg2In3Si2P7: A Quaternary Diamond-like Phosphide Infrared Nonlinear Optical Material Derived from ZnGeP2, J. Am. Chem. Soc., 2021, 143, 10309–10316 CrossRef CAS PubMed
.
- G. Cicirello, K. Wu and J. Wang, Synthesis, crystal structure, linear and nonlinear optical properties of quaternary sulfides Ba6(Cu2X)Ge4S16 (X=Mg, Mn, Cd), J. Solid State Chem., 2021, 300, 122226 CrossRef CAS
.
- B. Ji, K. Wu, Y. Chen, F. Wang, A. J. Rossini, B. Zhang and J. Wang, Ba6(CuxZy)Sn4S16 (Z=Mg, Mn, Zn, Cd, In, Bi, Sn): High Chemical Flexibility Resulting in Good Nonlinear-Optical Properties, Inorg. Chem., 2022, 61, 2640 CrossRef CAS PubMed
.
- M. El-Hagary, M. Emam-Ismail, E. R. Shaaban, A. Al-Rashidi and S. Althoyaib, Composition, annealing and thickness dependence of structural and optical studies on Zn1-xMnxS nanocrystalline semiconductor thin films, Mater. Chem. Phys., 2012, 132, 581–590 CrossRef CAS
.
- R. L. Gitzendanner, C. M. Spencer, F. J. DiSalvo, M. A. Pell and J. A. Ibers, Synthesis and structure of a new quaternary rare-earth sulfide, La6MgGe2S14, and the related compound La6MgSi2S14, J. Solid State Chem., 1997, 131, 399–404 CrossRef CAS
.
- L. S. Breton, G. Morrison, M. R. Lacroix, P. S. Halasyamani and H.-C. zur Loye, Lanthanide thioborates, an emerging class of nonlinear optical materials, efficiently synthesized using the boronchalcogen mixture method, Chem. Commun., 2022, 58, 7992–7995 RSC
.
- G. A. Marking and M. G. Kanatzidis, The ethane-like (Ge2S6)6− and (Si2Se6)6− ligands bound to main-group metals in Na8Pb2(Ge2S6)2, Na8Sn2(Ge2S6)2, and Na8Pb2(Si2Se6)2, J. Alloys Compd., 1997, 259, 122–128 CrossRef CAS
.
- Y.-K. Lian, R.-A. Li, X. Liu, L.-M. Wu and L. Chen, Sr6(Li2Cd)A4S16 (A=Ge, Sn): How to Go beyond the Band Gap Limitation via Site-Specific Modification, Cryst. Growth Des., 2020, 20, 8084–8089 CrossRef CAS
.
- R. Duan, H. Lin, Y. Wang, Y. Zhou and L. Wu, Non-centrosymmetric sulfides A2Ba6MnSn4S16 (A=Li, Ag): syntheses, structures and properties, Dalton Trans., 2020, 49, 5914–5920 RSC
.
|
This journal is © the Partner Organisations 2023 |
Click here to see how this site uses Cookies. View our privacy policy here.