DOI:
10.1039/D4NR01152J
(Paper)
Nanoscale, 2024,
16, 9361-9366
Construction of novel Ag(0)-containing silver nanoclusters by regulating auxiliary phosphine ligands†
Received
17th March 2024
, Accepted 11th April 2024
First published on 12th April 2024
Abstract
Controlled synthesis of metal clusters through minor changes in surface ligands holds significant interest because the corresponding entities serve as ideal models for investigating the ligand environment's stereochemical and electronic contributions that impact the corresponding structures and properties of metal clusters. In this work, we obtained two Ag(0)-containing nanoclusters (Ag17 and Ag32) with near-infrared emissions by regulating phosphine auxiliary ligands. Ag17 and Ag32 bear similar shells wherein Ag17 features a trigonal bipyramid Ag5 kernel while Ag32 has a bi-icosahedral interpenetrating an Ag20 kernel. Ag17 and Ag32 showed a near-infrared emission (NIR) of around 830 nm. Benefiting from the rigid structure, Ag17 displayed a more intense near-infrared emission than Ag32. This work provides new insight into the construction of novel superatomic silver nanoclusters by regulating phosphine ligands.
Introduction
Metal nanoclusters have attracted wide attention because of their atom-precise structures and quantum size effects, which provide an ideal platform for investigating the connection between simple metal complexes and bulk metal nanoparticles.1–16 In addition, their potential applications in optics,17–20 catalysis,21–25 and biomedicine26–28 encourage us to synthesize novel structured nanoclusters to exploit versatile properties. Among these, nanoclusters with valence electron structures similar to atomic electron structures are considered “superatomic nanoclusters”.29–34 The regulation of superatomic nanocluster structures and nuclearities is beneficial for broadening the diversity of properties and facilitating the exploration of the structure–property correlation at the atomic level. The synthesis of structurally well-defined Ag(0)-containing nanoclusters has been performed for over a decade, but the instability of silver nanoclusters restricts their structural modulation.35–39
Currently, several effective methods have been developed to fine-tune the nanocluster structures, including heterometal doping,40–42 ligand engineering,43–48 counter ion template,49–52 and so on. The ligand engineering strategy is considered one of the most effective ways to regulate the structure and supramolecular assembly of nanoclusters. Ligand engineering strategies have been reported to adjust the structures and properties of nanoclusters by the steric hindrance effect, electron-withdrawing and donating effects, etc.53,54 Surface ligand regulation includes the primary ligands (such as thiolate ligands) and auxiliary ligands. Although manipulating primary ligands is effective for achieving higher nuclearities and electron counts, it is often a time-consuming and laborious process.55–57 In contrast, the use of readily available auxiliary ligands, such as phosphine ligands, has the potential to be more efficient.58,59
Herein, by regulating the auxiliary phosphine ligands, we synthesized two cases of superatomic silver nanoclusters, formulated as [Ag17(L)12(PPh3)6](NO3)3 (L = quinoline-2-thiol (deprotonation)-4-carboxyl, PPh3 = triphenylphosphine, denoted as Ag17) and [Ag32(L)18(P(Ph-pCH3)3)6Cl2](NO3)4 (L = quinoline-2-thiol (deprotonation)-4-carboxyl, P(Ph-pCH3)3 = tri-p-tolylphosphine, denoted as Ag32). The atomically precise structures of two silver nanoclusters were determined by single-crystal X-ray diffraction (SC-XRD) and further confirmed by electrospray ionization mass spectrometry (ESI-MS) measurements. Both Ag17 and Ag32 feature Ag4L4P2 unit-based surface structures, but Ag17 has an Ag5 trigonal bipyramid kernel while Ag32 has the Ag20 inner core with the fusion of two classical Ag13 icosahedrons. Both of them have emissions in the near-infrared region under ambient temperature. The quantum yield of the Ag17 solution is 3.09%, while the quantum yield of the Ag32 solution is below 0.1%. Ag17 shows superior luminescence properties compared to Ag32 due to its rigid surface structure.
Experimental section
Materials and reagents
All chemicals and solvents obtained from suppliers were used without further purification. All solvents were analytical-grade reagents. Silver nitrate (AgNO3, 98%), triphenylphosphine (PPh3, 97%), tri-p-tolylphosphine (P(Ph-pCH3)3, 97%), 2-chloroquinoline-4-carboxylic acid (2-Cl-4-COOH-QL, 96%), thiourea (CH4N2S, 99%), sodium borohydride (NaBH4, 98%), sodium hydroxide (NaOH, 98%), and hydrochloric acid (HCl, 38%) were used.
Synthesis of quinoline-2-thiol (deprotonation)-4-carboxyl (L)
L was synthesized according to the procedures described in the literature.60 2-Cl-4-COOH-QL (1 mol) was added to the EtOH solution of thiourea (1.2 mol) and the mixture was refluxed for 15–30 min. Then the solution was diluted to twice its volume with H2O, and the yellow product obtained was dissolved in 10% NaOH. The yellow filtrate was acidified with 10% HCl and the yellow precipitate obtained was collected by filtration, washed with H2O, and dried. It was recrystallized from EtOH to give yellowish-orange needles. The 1H NMR spectra confirmed the structure (Fig. S1, ESI†). δ = 13.99 (s, 1H), 8.34–8.33 (d, 1H), 7.69–7.68 (d, 2H), 7.58 (d, 1H), 7.43–7.40 (m, 1H).
Synthesis of [Ag17(L)12(PPh3)6](NO3)3 (Ag17)
AgNO3 (12 mg, 0.07 mmol) was dissolved in a mixed solvent of 4 mL of methanol and 4 mL of dichloromethane at room temperature. Then L (10 mg, 0.05 mmol) and PPh3 (13 mg, 0.05 mmol) were added under vigorous stirring to afford Ag–S–P complex. About 5 min later, freshly prepared NaBH4 (2 mg, 0.052 mmol) in 1 mL of methanol was added dropwise under stirring. The color of the solution changed from yellow to brown–black. Five hours later, the brown–black solution was collected after centrifuging. The mixture evaporated slowly at room temperature and orange crystals were obtained for five days. Yield: 15% (based on Ag). Elemental analysis (found (calcd.), %; based on C228H162Ag17N15O33P6S12): C, 44.86 (45.31); H, 2.62 (2.70); N, 3.19 (3.48); S, 6.48 (6.37); O 8.54 (8.74).
Synthesis of [Ag32(L)18(P(Ph-pCH3)3)6Cl2](NO3)4 (Ag32)
AgNO3 (17 mg, 0.1 mmol) was dissolved in a mixed solvent of 4 mL of methanol and 4 mL of dichloromethane at room temperature. Then L (12 mg, 0.06 mmol) and P(Ph-pCH3)3 (25 mg, 0.082 mmol) were added under vigorous stirring. About 5 min later, freshly prepared NaBH4 (2 mg, 0.052 mmol) in 1 mL of methanol was added dropwise under stirring. The color of the solution changed from yellow to brown–black. Five hours later, the brown–black solution was collected after centrifuging. The mixture evaporated slowly at room temperature and black block crystals were obtained for five days. Yield: 27% (based on Ag). Elemental analysis (found (calcd.), %; based on C306H234Ag32N22O48P6S18Cl2): C, 39.91 (39.64); H, 2.32 (2.54); N, 3.41 (3.32); S, 6.39 (6.22); O, 8.35 (8.28).
Characterization
1H NMR spectroscopy was carried out on a Bruker 400 spectrometer operating at 600 MHz with DMSO-d6 as the solvent. Electrospray ionization mass spectrometry (ESI-MS) was performed on a X500R QTOF spectrometer. Powder X-ray diffraction (PXRD) patterns of the samples were recorded on a D/MAX-3D diffractometer. Elemental analyses (EA) were carried out with a PerkinElmer 240 elemental analyzer. Fourier transform infrared (FT-IR) spectra were recorded on a Bruker ALPHA II spectrometer. UV-vis absorption spectra were obtained by means of a Hitachi UH4150 UV-visible spectrophotometer. The NIR luminescence spectra were measured with an Edinburgh FLS 980 fluorescence spectrometer. The luminescence lifetime was measured on an Edinburgh FLS 980 fluorescence spectrometer operating in time-correlated single-photon counting (TCSPC) mode. The quantum yield (QY) was measured using an integrating sphere on an Edinburgh FLS 980 fluorescence spectrometer. X-ray photoelectron spectroscopy (XPS) was conducted using a Thermo ESCALAB 250XI with Al Kα radiation as the excitation source. C 1s calibrated binding energies at 284.8 eV. Energy-dispersive spectroscopy and elemental mapping measurements were performed via a Zeiss Sigma 500.
Results and discussion
Ag17 and Ag32 were prepared by a one-pot reduction method. Briefly for Ag17, AgNO3 was dissolved in a mixed solvent of methanol and dichloromethane at room temperature followed by the addition of L and PPh3 under vigorous stirring to afford the Ag–S–P complex. Subsequently, freshly prepared NaBH4 in methanol was added dropwise under stirring. The color of the solution changed from yellow to brown–black. The raw product solution was collected after centrifuging. Orange crystals were obtained by evaporating slowly in darkness for five days. Ag32 was prepared by a similar method but with P(Ph-pCH3)3 instead of PPh3, and the black block crystals of Ag32 were obtained (Scheme 1).
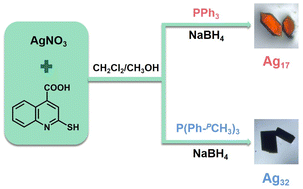 |
| Scheme 1 Synthetic routes for Ag17 and Ag32. | |
The chemical compositions of Ag17 and Ag32 were confirmed by electrospray ionization mass spectroscopy (ESI-MS). As shown in Fig. 1a, the ESI-MS spectrum of Ag17 shows a prominent signal at m/z 1952.70, which corresponds to the [Ag17(PPh3)6(L)12]3+ species (calcd. m/z 1952.69). Ag17 exhibits a 2-electron configuration (17 − 12 − 3 = 2e). As shown in Fig. 1b, the ESI-MS spectrum of Ag32 shows a prominent signal at m/z 2256.26, which corresponds to the [Ag32(P(Ph-pCH3)3)6(L)18Cl2]4+ species (calcd. m/z 2256.24). It bears an eight-electron closed-shell (32 − 18 − 2 − 4 = 8e) structure. The presence of NO3− in Ag17 and Ag32 was also confirmed by Fourier transform infrared (FT-IR) spectra and negative-ion mode ESI-MS spectra (Fig. S2 and S3, ESI†). Powder X-ray diffraction (PXRD) further verified the phase purity of Ag17 and Ag32 (Fig. S4, ESI†). X-ray photoelectron spectroscopy (XPS) and energy dispersive X-ray spectrometry (EDS) elemental mapping of Ag17 and Ag32 revealed all of the expected elements and the presence of Ag(0) in Ag17 and Ag32 (Fig. S5–S8, ESI†).
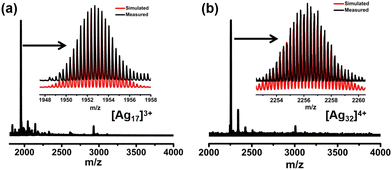 |
| Fig. 1 (a) Positive-ion mode ESI-MS of Ag17 in CH3OH. (Inset) Measured (black line) and simulated (red line) isotopic distribution patterns of the molecular ion peak. (b) Positive-ion mode ESI-MS of Ag32 in CH3OH. (Inset) Measured (black line) and simulated (red line) isotopic distribution patterns of the molecular ion peak. | |
Single-crystal X-ray diffraction (SC-XRD) analysis revealed that Ag17 crystallized in the P
1c space group. The overall structure of Ag17 shows a 3-fold rotational symmetry (Fig. 2a and Fig. S9, ESI†). Ag17 has an Ag5 trigonal bipyramid (Fig. 2b) covered by the Ag12(L)12(PPh3)6 shell (Fig. S10a, ESI†). The 3-fold axis passes through the top and bottom of the two vertexes of the kernel. Thus, the Ag12(L)12(PPh3)6 shell could be regarded as a trimeric structure of the Ag4L4(PPh3)2 unit (Fig. 2c and d). Each Ag4L4(PPh3)2 is located on one side of the trigonal bipyramid. The thiolates in the “equatorial” position of the kernel adopt a μ4 bridging mode and the other thiolates near the vertexes of the kernel adopt a μ3 bridging mode. The Ag atoms located above the triangular Ag3 face of the Ag5 trigonal bipyramid are quadruply coordinated to three sulfur atoms of three L ligands and one phosphorus atom of PPh3. The other Ag atoms located near vertexes of the kernel are quadruply coordinated to two sulfur atoms and two nitrogen atoms of the neighboring two L ligands. In addition, the free carboxylic acid functional groups impart potential for post-modification to Ag17.
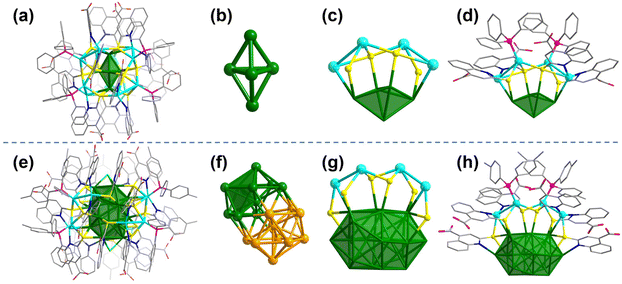 |
| Fig. 2 (a) The overall structure of Ag17. (b) The Ag5 kernel of Ag17. (c) The Ag4S4 motif of Ag17. (d) The Ag17 peripheral Ag4L4(PPh3)2 unit. (e) The overall structure of Ag32. (f) The Ag20 kernel of Ag32 (the polyhedron highlighted is an Ag5 unit). (g) The Ag4S6 motif of Ag32. (h) The Ag32 peripheral Ag4L6(P(Ph-pCH3)3)2 unit. Atom colors: Ag, dark green, turquoise and orange; P, purple; N, blue; S, yellow; Cl, light green; C, gray; O, red. All hydrogen atoms are omitted for clarity. | |
Ag32 adopts the P
3n space group with a formula [Ag32(P(Ph-pCH3)3)6(L)18Cl2]. The overall structure of Ag32 is shown in Fig. 2e. Structurally, Ag32 features an Ag20 kernel, which is the fusion of two classical Ag13 icosahedrons (Fig. 2f). Alternatively, the Ag20 kernel could also be regarded as the fusion of the Ag5 trigonal bipyramid by sharing the icosahedral central Ag atom. Probably due to Ag17 and Ag32 having an Ag5 trigonal bipyramid-based kernel, they have similar surface structures (Fig. S10b, ESI†). Compared to Ag17 with a trimeric structure of Ag4L4(PPh3)2 units, Ag32 has three monomeric Ag4L4(P(Ph-pCH3)3)2 units (Fig. 2g and h). The additional two L ligands capping the Ag4L4(P(Ph-pCH3)3)2 units prevent their polymerization. There are two Cl-terminated atoms at both ends of Ag20, and each Cl atom caps the Ag3 triangular face (Fig. S11, ESI†). Each silver atom of the Ag3 triangular face is not only coordinated with Cl but also protected by S and N (deprotonation) from two quinoline ligands. The distances between Ag and Cl atoms in Ag32 were 2.589 and 2.606 Å (average 2.60 Å), which were not only much shorter than the sum of van der Waals radii of Ag and Cl atoms (1.72 Å + 1.80 Å = 3.52 Å), but also shorter than the sum of the atomic radii of Ag and Cl atoms (1.44 Å + 1.62 Å = 3.06 Å). Accordingly, the interactions between Ag and Cl atoms in Ag32 could be considered covalent interactions. The Ag–Ag bond distances of Ag3 are 3.276 Å and 3.288 Å. The average Ag–Ag distance between the Ag atoms in the outer shell to the core in Ag32 is 2.9575 Å, which is shorter than that of Ag17 (3.3109 Å). All the thiolates in Ag32 are μ3 coordination modes. Twelve of the -SR groups were co-coordinated with two Ag atoms in the core and one Ag atom in the shell layer, and the remaining six -SR groups were coordinated with one Ag atom in the core and two Ag atoms in the shell layer. Due to the change of the phosphine ligand (replace PPh3 with P(Ph-pCH3)3), the π⋯π interactions (Fig. S12, ESI†) and the change in steric hindrance caused by the alteration of the phosphine ligand, which affected the arrangement of the peripheral ligands, ultimately resulted in two different structures of Ag17 and Ag32.
As illustrated in Fig. 3a and b, Ag17 and Ag32 in CH3OH exhibit distinct UV-vis absorption spectra with molecule-like optical transitions. The UV-vis spectrum of Ag17 shows optical absorption bands at 330, 387, 487, and 597 nm. Likewise, Ag32 has absorptions at 357, 499, and 653 nm. The similar absorption in the high-energy region may derived from their similar surface structures. To gain an insight into the electronic structures and absorption characteristics of Ag17 and Ag32, time-dependent density functional theory (TD-DFT) calculations were performed to simulate the optical absorptions (Fig. 3, Fig. S13 and S14, ESI†). The calculated UV-vis absorption spectra of Ag17 and Ag32 match well with the experimental ones with slight shifts. For Ag17, the transition for the peaks of 423 nm is mainly from the outer shell. The lowest-energy absorption band at 618.2 nm is attributed to electronic transition from the highest occupied molecular orbital (HOMO) to the lowest unoccupied molecular orbital (LUMO). For the Ag32, the transitions for the peaks of 362.5 nm and 451.0 nm are from the outer shell. The lowest-energy absorption band at 597.7 nm is attributed to the electronic transition from the HOMO to the LUMO. The two nanoclusters of 2e Ag17 and 8e Ag32 exhibit clear super-atomic orbital characteristics with 1S2 and 1S21P6 (Fig. S15, ESI†).
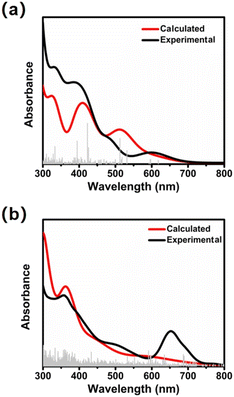 |
| Fig. 3 Experimental (black) and calculated (red) absorption spectra of Ag17 (a) and Ag32 (b) in CH3OH. | |
Both Ag17 and Ag32 have emissions in the near-infrared region in solution (Fig. 4 and Fig. S16, ESI†). The Ag17 NCs in CH3OH show the maximum emission peak remaining at 830 nm. The HOMO of Ag17 mainly lies on the Ag5 kernel, while the LUMO is located on the surface ligands. Thus, the emission is mainly derived from the metal-to-ligand charge transfer (MLCT). The Ag32 nanocluster in CH3OH shows the maximum emission peak at 840 nm, mainly from the mixed MLCT and cluster-centered transition. It can be found that the luminescence intensity of Ag17 is an order of magnitude higher than that of Ag32. The quantum yield (QY) of Ag17 in the CH3OH solution is 3.09%, while the QY of Ag32 is below 0.1%. The lifetimes of Ag17 and Ag32 are 1.47 μs and 1.61 μs (Fig. S17 and S18, ESI†), respectively, indicating phosphorescence. Similarly, the emission peak intensity of Ag17 in the near-infrared region is stronger than that of Ag32 in the solid state (Fig. S19 and S20, ESI†). As mentioned above in the structural analysis section, Ag17 has a trimeric structure of Ag4L4(PPh3)2 units and Ag32 has three discrete monomeric Ag4L4(P(Ph-pCH3)3)2 units. Therefore, the rigid structure of Ag17 decreases the nonradiative decay, resulting in enhanced luminescence. Besides, rotation of the methyl groups on the phosphine ligands of Ag32 also dissipates energy, facilitating nonradiative decay that converts light into heat.61
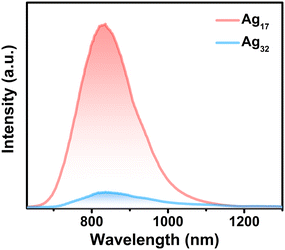 |
| Fig. 4 Emission spectra of Ag17 and Ag32 in CH3OH solution (excited at 400 nm). | |
Conclusions
In conclusion, we synthesized two new superatomic silver nanoclusters Ag17 and Ag32 by adjusting phosphine ligands. The structural anatomy indicated that the kernel of Ag17 is Ag5 and the kernel of Ag32 is Ag20. The inner cores of Ag17 and Ag32 have huge distinctions while the peripheral Ag–S motifs have some similarities. In addition, both the solution states and the solid states of Ag17 and Ag32 have luminescence properties in the near-infrared region. Due to the rigid structure of Ag17 and the high-frequency vibrations of the methyl groups of Ag32, Ag17 shows stronger near-infrared emission intensity and higher QY. This work provides new insight into the construction of novel superatomic silver nanoclusters by co-reduction by regulating phosphine ligands.
Author contributions
Qing-Qing Ma and Xue-Jing Zhai: investigation, data curation, formal analysis and writing – original draft; Jia-Hong Huang: writing – original draft and validation; Yubing Si: theoretical simulation; Xi-Yan Dong: conceptualization, writing – review & editing and funding acquisition; Shuang-Quan Zang: supervision and funding acquisition; and Thomas C. W. Mak: supervision.
Conflicts of interest
There are no conflicts to declare.
Acknowledgements
This work was supported by the National Natural Science Foundation of China (No. U21A20277, 92061201), the Excellent Youth Foundation of Henan Scientific Committee (232300421022) and the Thousand Talents (Zhongyuan Scholars) Program of Henan Province (234000510007).
References
- R. Jin, C. Zeng, M. Zhou and Y. Chen, Chem. Rev., 2016, 116, 10346–10413 CrossRef CAS PubMed.
- Z. Lei, X.-K. Wan, S.-F. Yuan, Z.-J. Guan and Q.-M. Wang, Acc. Chem. Res., 2018, 51, 2465–2474 CrossRef CAS PubMed.
- Y. Jin, C. Zhang, X.-Y. Dong, S.-Q. Zang and T. C. W. Mark, Chem. Soc. Rev., 2021, 50, 2297–2319 RSC.
- X. Kang, Y. Li, M. Zhu and R. Jin, Chem. Soc. Rev., 2020, 49, 6443–6514 RSC.
- Y.-M. Su, Z. Wang, C.-H. Tung, D. Sun and S. Schein, J. Am. Chem. Soc., 2021, 143, 13235–13244 CrossRef CAS PubMed.
- X.-Y. Dong, Y. Si, J.-S. Yang, C. Zhang, Z. Han, P. Luo, Z.-Y. Wang, S.-Q. Zang and T. C. W. Mak, Nat. Commun., 2020, 11, 3678 CrossRef CAS PubMed.
- M. S. Bootharaju, S. Lee, G. Deng, S. Malola, W. Baek, H. Häkkinen, N. Zheng and T. Hyeon, Angew. Chem., Int. Ed., 2021, 60, 9038–9044 CrossRef CAS PubMed.
- J.-H. Huang, Z.-Y. Wang, S.-Q. Zang and T. C. W. Mak, ACS Cent. Sci., 2020, 6, 1971–1976 CrossRef CAS PubMed.
- A. C. Templeton, W. P. Wuelfing and R. W. Murray, Acc. Chem. Res., 2000, 33, 27–36 CrossRef CAS PubMed.
- R. H. Adnan, J. M. L. Madridejos, A. S. Alotabi, G. F. Metha and G. G. Andersson, Adv. Sci., 2022, 9, 2105692 CrossRef CAS PubMed.
- F. Hu, R.-L. He, Z.-J. Guan, C.-Y. Liu and Q.-M. Wang, Angew. Chem., Int. Ed., 2023, 62, e202304134 CrossRef CAS PubMed.
- K. H. Wijesinghe, N. A. Sakthivel, T. Jones and A. Dass, J. Phys. Chem. Lett., 2020, 11, 6312–6319 CrossRef CAS PubMed.
- K. L. D. M. Weerawardene, P. Pandeya, M. Zhou, Y. Chen, R. Jin and C. M. Aikens, J. Am. Chem. Soc., 2019, 141, 18715–18726 CrossRef CAS PubMed.
- N. A. Sakthivel, M. Shabaninezhad, L. Sementa, B. Yoon, M. Stener, R. L. Whetten, G. Ramakrishna, A. Fortunelli, U. Landman and A. Dass, J. Am. Chem. Soc., 2020, 142, 15799–15814 CrossRef CAS PubMed.
- O. Lopez-Acevedo, H. Tsunoyama, T. Tsukuda, H. Häkkinen and C. M. Aikens, J. Am. Chem. Soc., 2010, 132, 8210–8218 CrossRef CAS PubMed.
- C. P. Joshi, M. S. Bootharaju, M. J. Alhilaly and O. M. Bark, J. Am. Chem. Soc., 2015, 137, 11578–11581 CrossRef CAS PubMed.
- X.-J. Zhai, J.-H. Hu, J. Guan, Y. Si, X.-Y. Dong, P. Luo, F. Pan, Z. Yu, R. Han and S.-Q. Zang, Nano Res., 2023, 16, 11366–11374 CrossRef CAS.
- S. Biswas and Y. Negishi, J. Phys. Chem. Lett., 2024, 15, 947–958 CrossRef CAS PubMed.
- R.-W. Huang, Y.-S. Wei, X.-Y. Dong, X.-H. Wu, C.-X. Du, S.-Q. Zang and T. C. W. Mark, Nat. Chem., 2017, 9, 689–697 CrossRef CAS PubMed.
- W.-M. He, Z. Zhou, Z. Han, S. Li, Z. Zhou, L.-F. Ma and S.-Q. Zang, Angew. Chem., Int. Ed., 2021, 60, 8505–8509 CrossRef CAS PubMed.
- S. Biswas, S. Das and Y. Negishi, Nanoscale Horiz., 2023, 8, 1509–1522 RSC.
- T. Kawawaki, T. Okada, D. Hirayama and Y. Negishi, Green Chem., 2024, 26, 122–163 RSC.
- X.-K. Wan, J.-Q. Wang, Z.-A. Nan and Q.-M. Wang, Sci. Adv., 2017, 3, e1701823 CrossRef PubMed.
- Y. Lei, F. Mehmood, S. Lee, J. Greeley, B. Lee, S. Seifert, R. E. Winans, J. W. Elam, R. J. Meyer, P. C. Redfern, D. Teschner, R. Schlögl, M. J. Pellin, L. A. Curtiss and S. Vajda, Science, 2010, 328, 224–228 CrossRef CAS PubMed.
- M. Cao, R. Pang, Q.-Y. Wang, Z. Han, Z.-Y. Wang, X.-Y. Dong, S.-F. Li, S.-Q. Zang and T. C. W. Mak, J. Am. Chem. Soc., 2019, 141, 14505–14509 CrossRef CAS PubMed.
- J. Yang, F. Yang, C. Zhang, X. He and R. Jin, ACS Mater. Lett., 2022, 4, 1279–1296 CrossRef CAS.
- Y. Hua, Z.-H. Shao, A. Zhai, L.-J. Zhang, Z.-Y. Wang, G. Zhao, F. Xie, J.-Q. Liu, X. Zhao, X. Chen and S.-Q. Zang, ACS Nano, 2023, 17, 7837–7846 CrossRef CAS PubMed.
- Z. Yang, R. Shi, X. Liu, Q. Zhang, M. Chen, Y. Shen, A. Xie and M. Zhu, ACS Mater. Lett., 2023, 5, 2361–2368 CrossRef CAS.
- T.-H. Huang, F.-Z. Zhao, Q.-L. Hu, Q. Liu, T.-C. Wu, D. Zheng, T. Kang, L.-C. Gui and J. Chen, Inorg. Chem., 2020, 59, 16027–16034 CrossRef CAS PubMed.
- P. Luo, S. Bai, X. Wang, J. Zhao, Z.-N. Yan, Y.-F. Han, S.-Q. Zang and T. C. W. Mark, Adv. Opt. Mater., 2021, 9, 2001936 CrossRef CAS.
- M. Zhu, C. M. Aikens, F. J. Hollander, G. C. Schatz and R. Jin, J. Am. Chem. Soc., 2008, 130, 5883–5885 CrossRef CAS PubMed.
- M. W. Heaven, A. Dass, P. S. White, K. M. Holt and R. W. Murray, J. Am. Chem. Soc., 2008, 130, 3754–3755 CrossRef CAS PubMed.
- R. S. Dhayal, J.-H. Liao, Y.-C. Liu, M.-H. Chiang, S. Kahlal, J.-Y. Saillard and C. W. Liu, Angew. Chem., Int. Ed., 2015, 54, 3702–3706 CrossRef CAS PubMed.
- L. G. AbdulHalim, M. S. Bootharaju, Q. Tang, S. Del Gobbo, R. G. AbdulHalim, M. Eddaoudi, D.-E. Jiang and O. M. Bark, J. Am. Chem. Soc., 2015, 137, 11970–11975 CrossRef CAS PubMed.
- A. Desireddy, B. E. Conn, J. Guo, B. Yoon, R. N. Barnett, B. M. Monahan, K. Kirschbaum, W. P. Griffith, R. L. Whetten, U. Landman and T. P. Bigioni, Nature, 2013, 501, 399–402 CrossRef CAS PubMed.
- M. Bodiuzzaman, E. Khatun, K. S. Sugi, G. Paramasivam, W. A. Dar, S. Antharjanam and T. Pradeep, J. Phys. Chem. C, 2020, 124, 23426–23432 CrossRef CAS.
- L. Pen, P. Yuan, H. Su, S. Malola, S. Lin, Z. Tang, B. K. Teo, H. Häkkinen, L. Zheng and N. Zheng, J. Am. Chem. Soc., 2017, 139, 13288–13291 CrossRef PubMed.
- G.-X. Duan, L. Tian, J.-B. Wen, L.-Y. Li, Y.-P. Xie and X. Lu, Nanoscale, 2018, 10, 18915–18919 RSC.
- F. Hu, J.-J. Li, Z.-J. Guan, S.-F. Yuan and Q.-M. Wang, Angew. Chem., Int. Ed., 2020, 59, 5312–5315 CrossRef CAS PubMed.
- W. Fei, S. Antonello, T. Dainese, A. Dolmella, M. Lahtinen, K. Rissanen, A. Venzo and F. Maran, J. Am. Chem. Soc., 2019, 141, 16033–16045 CrossRef CAS PubMed.
- W.-Q. Shi, Z.-J. Kuan, J.-J. Li, X.-S. Han and Q.-M. Wang, Chem. Sci., 2022, 13, 5148–5154 RSC.
- S. Hossain, D. Suzuki, T. Iwasa, R. Kaneko, T. Suzuki, S. Miyajima, Y. Iwamatsu, S. Pollitt, T. Kawawaki, N. Barrabés, G. Rupprechter and Y. Negishi, J. Phys. Chem. C, 2020, 124, 22304–22313 CrossRef CAS.
- W.-D. Tian, W.-D. Si, S. Havenridge, C. Zhang, Z. Wang, C. M. Aikens, C.-H. Tung and D. Sun, Sci. Bull., 2024, 69, 40–48 CrossRef CAS PubMed.
- C. A. Hosier and C. J. Ackerson, J. Am. Chem. Soc., 2019, 141, 309–314 CrossRef CAS PubMed.
- J. Xin, J. Xu, C. Zhu, Y. Tian, Q. Zhang, X. Kang and M. Zhu, Chem. Sci., 2023, 14, 8474–8482 RSC.
- Y. Wang, Z. Liu, A. Mazumder, C. G. Gianopoulos, K. Kirschbaum, L. A. Peteanu and R. Jin, J. Am. Chem. Soc., 2023, 145, 26328–26338 CrossRef CAS PubMed.
- L. C. McKenzie, T. O. Zaikova and J. E. Hutchison, J. Am. Chem. Soc., 2014, 136, 13426–13435 CrossRef CAS PubMed.
- S. Biswas, S. Das and Y. Negishi, Coord. Chem. Rev., 2023, 492, 215255 CrossRef CAS.
- L. Li, Y. Zhu, B. Han, Q. Wang, L. Zheng, L. Feng, D. Sun and Z. Wang, Chem. Commun., 2022, 58, 9234–9237 RSC.
- R. K. Gupta, L. Li, Z. Wang, B.-L. Han, L. Feng, Z.-Y. Gao, C.-H. Tung and D. Sun, Chem. Sci., 2023, 14, 1138–1144 RSC.
- Y. Horita, S. Hossain, M. Ishimi, P. Zhao, M. Sera, T. Kawawaki, S. Takano, Y. Niihori, T. Nakamura, T. Tsukuda, M. Ehara and Y. Negishi, J. Am. Chem. Soc., 2023, 145, 23533–23540 CrossRef CAS PubMed.
- J.-J. Li, C.-Y. Liu, Z.-J. Guan, Z. Lei and Q.-M. Wang, Angew. Chem., Int. Ed., 2022, 61, e202201549 CrossRef CAS PubMed.
- E. Khatun, A. Ghosh, P. Chakraborty, P. Singh, M. Bodiuzzaman, P. Ganesan, G. Nataranjan, J. Ghosh, S. K. Pal and T. Pradeep, Nanoscale, 2018, 10, 20033–20042 RSC.
- X. Kang, S. Wang and M. Zhu, Chem. Sci., 2018, 9, 3062–3068 RSC.
- Y. Wang, Z. Liu, A. Mazumder, C. G. Gianopoulos, K. Kirschbaum, L. A. Peteanu and R. Jin, J. Am. Chem. Soc., 2023, 145, 26328–26338 CrossRef CAS PubMed.
- Z. Liu, Y. Li, E. Kahng, S. Xue, X. Du, S. Li and R. Jin, ACS Nano, 2022, 16, 18448–18458 CrossRef CAS PubMed.
- N. A. Sakthivel and A. Dass, Acc. Chem. Res., 2018, 51, 1774–1783 CrossRef CAS PubMed.
- X. Wei, Y. Lv, H. Shen, H. Li, X. Kang, H. Yu and M. Zhu, Aggregate, 2023, 4, e246 CrossRef CAS.
- Z. He, Y. Yang, J. Zou, Q. You, L. Feng, M.-B. Li and Z. Wu, Chem. – Eur. J., 2022, 28, e202200212 CrossRef CAS PubMed.
- S. Nakano, T. Yoshida, H. Taniguchi and M. Yasuki, Chem. Pharm. Bull., 1980, 28, 49–56 CrossRef CAS.
- D. Xi, M. Xiao, J. Cao, L. Zhao, N. Xu, S. Long, J. Fan, K. Shao, W. Sun, X. Yan and X. Peng, Adv. Mater., 2020, 32, 1907855 CrossRef CAS PubMed.
Footnote |
† Electronic supplementary information (ESI) available: Fig. S1–S20 and Tables S1–S4 for experimental details, detailed crystallographic structures, PXRD, and UV-vis spectra. CCDC 2327808 and 2327952. For ESI and crystallographic data in CIF or other electronic format see DOI: https://doi.org/10.1039/d4nr01152j |
|
This journal is © The Royal Society of Chemistry 2024 |
Click here to see how this site uses Cookies. View our privacy policy here.