DOI:
10.1039/D4QI02452D
(Research Article)
Inorg. Chem. Front., 2025,
12, 161-170
In situ construction of donor–acceptor structured g-C3N4 nanotubes incorporated with pyridine heterocyclic rings for efficient photocatalytic water splitting†
Received
28th September 2024
, Accepted 11th November 2024
First published on 13th November 2024
Abstract
Polymeric carbon nitride (PCN) materials, as an emerging class of metal-free photocatalysts, have demonstrated significant potential in the field of solar energy conversion, particularly in areas of water splitting. But the utilization of PCN is restricted by its high carrier recombination rate and low charge transfer efficiency. In order to address these challenges, this work involves choosing pyridyl organic small molecules of nicotinic acid (NA) and melamine to construct donor–acceptor (D–A)-structured carbon nitride nanotubes. Pyridine heterocyclic rings are converged at the edge of the PCN structure via supramolecular self-assembly, facilitating the fabrication of donor–acceptor-structured carbon nitride nanotubes. The pyridine heterocyclic rings, with their strong electronic ability, create a preferred pathway for electronic transfer. This effectively mitigates carrier recombination within the molecular plane. In addition, the unique hollow tubular structure of carbon nitride nanotubes enhances their visible light absorption ability, expands the surface area of the catalyst, and then increases the number of catalytically active sites, which consequently enhances photocatalytic performance. The H2 production rates of one-dimensional tubular carbon nitride doped with 100 mg of NA (designated as NA100-CN) is 2584.2 μmol g−1 h−1, which is 4.7 times that of pristine PCN. This investigation elucidates the mechanism of charge transfer from D to A, describing the response mechanism of photocatalysis, with profound implications for advancing clean energy, environmental preservation and sustainable development.
1. Introduction
At present, the global energy landscape is still dominated by fossil energy, such as petroleum, coal, natural gas and so on.1 These sources occupy a large proportion of global energy consumption and have been excessively exploited, leading to serious environmental pollution and energy shortages.2 Due to its superior energy density and low carbon emissions, hydrogen (H2) is one of the most promising energy sources for the future.3 Among various hydrogen production technologies, converting solar energy into chemical energy through photocatalysis is a significant method for utilizing renewable energy.4 This photocatalytic technology relies on photocatalysts to split water into hydrogen under sunlight. Low carrier separation efficiency and high carrier recombination rates have led to low quantum efficiency and low solar energy conversion efficiency, impeding the practical application of photocatalytic water splitting.5 Despite the vast potential of this technology, its large-scale implementation is still hindered. Therefore, it is particularly important to choose the appropriate photocatalytic material and design high-efficiency photocatalytic systems to improve the conversion efficiency of solar energy utilization.
Among semiconductors, polymeric carbon nitride (PCN) materials have emerged as excellent candidates due to their low cost, environmental friendliness and robust thermal stability.6 Due to its special electronic structure, PCN offers facile regulation of its structure and bandgap, rendering it a promising photocatalyst that has garnered extensive research attention. However, PCN still has problems such as low solar energy utilization efficiency and so on.7 Various strategies have been explored to address these issues and enhance the photocatalytic performance of PCN, encompassing doping with non-metallic elements or organic molecules, morphological engineering, crystallization and the construction of heterostructures.8 Despite optimization efforts, the photocatalytic activity of PCN still falls short of industrial standards.9 Currently, studies have revealed that the n → π* electron transition in carbon nitride (the absorption peak is located near 490 nm) can effectively broaden the visible light response wavelength of PCN to more than 550 nm, which in turn improves the visible photocatalytic activity of PCN and has attracted extensive attention.10,11 However, the n → π* electron transition is forbidden in the plane-symmetric heptazine structure. In order to excite the n → π* electron transition, it is necessary to destroy this plane-symmetric structure and distort it. Researchers have succeeded in realizing the n → π* electron transition in PCN by means of creating defects, breaking hydrogen bonds, etc., which have improved its visible photocatalytic activity.12 However, these methods also destroy the integrity of the original PCN structure, which is not conducive to the rapid separation and transfer of photogenerated carriers. It can be expected that it would be more effective to improve the visible photocatalytic activity of PCN if the n → π* electron transition can be excited under the premise of ensuring the rapid separation and transfer of photogenerated carriers. At the same time, several investigations have demonstrated that aromatic organic rings can be effectively grafted onto PCN edges, which can realize the n → π* electron transition in PCN, thus changing the electron distribution on the PCN surface and promoting charge separation.6,13 Recently, Bhoyar et al. induced the formation of donor–acceptor (D–A) networks by doping PCN with 2,3-diaminopyridine (DAP).13 This resulted in a notable enhancement in photocatalytic hydrogen production activity, reaching 6.56 mmol g−1 h−1, which is 4.5 times that of pristine PCN, and it is found that photogenerated electrons are transferred from PCN (donor) to DAP (acceptor), elucidating the mechanism behind this enhancement.
Here, nicotinic acid (NA) was selected as the precursor. During the self-assembly stage of the supramolecular structure, pyridine heterocyclic rings were precisely grafted onto the edge of the PCN network through the method of conjugated polymerization. The π-electrons of NA are delocalized on the pyridine heterocyclic rings because the N atom has a strong electron-accepting ability, while the hydroxyl groups carried by NA are compatible with the heptazine ring structure. Consequently, NA can coordinate with melamine, and subsequent hydrothermal treatment and calcination result in the successful grafting of the pyridine heterocyclic rings onto the edges of the PCN network, forming one-dimensional tubular carbon nitride (designated as NAX-CN). Experimental results demonstrate a significant enhancement in the photocatalytic activity of NA100-CN. The photocatalytic H2-production activity of NA100-CN is 2584.2 μmol g−1 h−1, which is 4.7 times that of pristine PCN. The successful introduction of pyridine heterocyclic rings promoted the migration and separation of photogenerated electron–hole pairs, enabled more electrons to participate in the proton reduction response, and improved the photocatalytic hydrogen evolution performance.
2. Results and discussion
2.1 Characterization analysis of morphology and chemical structure
Fig. 1a illustrates the flowchart depicting the preparation of NAX-CN (X = 50, 100, 150 mg). Using NA and melamine as raw materials, a supramolecular intermediate was formed through hydrothermal treatment at 200 °C for 12 h via the supramolecular self-assembly method. During this process, thanks to the carboxyl groups of NA and the amino groups of melamine, the NA assembles with melamine and cyanuric acid into the supramolecular intermediate through hydrogen bonds. The interaction of the hydrogen bonds controls the crystal growth of the supramolecular intermediate, ultimately leading to the generation of ordered rod-like intermediates through the supramolecular assembly approach.14 Subsequently, the conjugated polymeric intermediate was placed in a muffle furnace and heated up to 500 °C at a rate of 2 °C min−1. It was then calcined at 500 °C for 4 h to form an ultrathin tubular polymer (Fig. 1b–f). During this process, the pyridine moiety of NA was connected to the edges of the polymer network through π-conjugation, allowing the pyridine heterocyclic rings with unique conjugated structure to modify the edge of the PCN framework.15 Consequently, D–A (donor–acceptor)-type one-dimensional nanotubes were created.
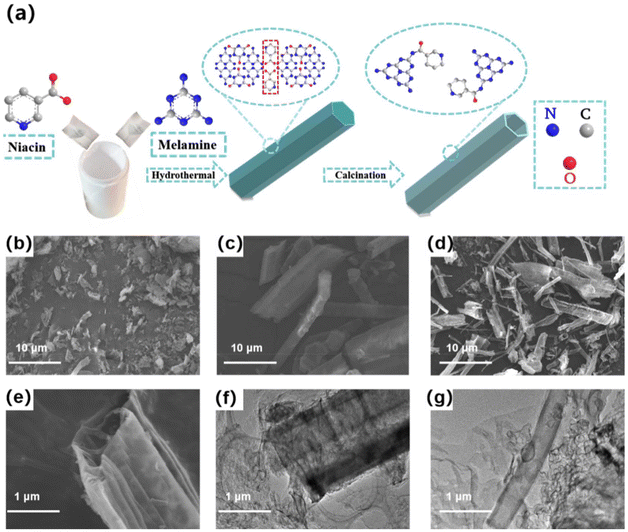 |
| Fig. 1 (a) Schematic diagram of preparing NA100-CN, (b) SEM image of PCN, (c) supramolecular intermediate, (d and e) SEM images of NA100-CN, and (f and g) TEM images of NA100-CN. | |
To gain a deeper understanding of the morphology and structure of the nanotube samples, the prepared samples were analyzed by transmission electron microscopy (TEM) and scanning electron microscopy (SEM) (Fig. 1b–g). PCN obtained by direct calcination exhibited a stacked block structure with a large volume and irregular block size (Fig. 1b). Fig. 1c clearly shows the formation of rod-like structures through supramolecular self-assembly. Fig. 1d–g reveals that NA100-CN exhibits a hollow tubular structure, characterized by multiple folds and accumulations at the tube edges. Partial peeling and subtle pores were evident on the surface of both the tube mouth and walls. This phenomenon can be attributed to the grafting of pyridine heterocyclic rings from NA onto the edges of the PCN, disrupting the in-plane heptazine ring balance.16 The introduction of pyridine heterocyclic rings leads to interlayer stripping, indirectly corroborating the embedding of pyridine heterocyclic rings.10 Furthermore, Fig. 1d demonstrates that the prepared NA100-CN material has a remarkably stable hollow tubular structure, which is attributed to the meticulous control of experimental factors, such as temperature and pressure during the supramolecular preparation process.17 To provide a more comprehensive understanding of the structural variations between NA100-CN and PCN, we acquired high-resolution TEM images (Fig. S3†), which enable a clearer observation of the microstructures and potential doping morphologies of the materials. This reveals an irregular arrangement of structures on the surface, devoid of discernible lattice fringes, indicating that the formation of NA100-CN does not involve a straightforward carbon doping process.
To further validate the structural characteristics of NAX-CN and PCN, nitrogen adsorption–desorption and pore size measurements were conducted on the prepared photocatalysts. As shown in Fig. 2a, the N2 adsorption–desorption isothermal plot of NA100-CN exhibits a type IV curve, with a specific surface area of 16.8 m2 g−1, which is 3.7 times larger than that of PCN (4.6 m2 g−1). Additionally, the presence of an H3-type hysteresis loop at p/p0 = 0.6–1.0 indicates the existence of a mesoporous surface structure.18Fig. 2b depicts the pore size distribution of NA100-CN and PCN, revealing a significant number of pores distributed within the range of 2–5 nm, which is consistent with the observations made from the TEM images. The formation of this porous structure not only increases the specific surface area of the sample, providing more reactive sites, but also shortens the diffusion paths for reactants and photogenerated charge carriers.19 Furthermore, it reduces the recombination rate of photogenerated electrons and holes, thereby enhancing the hydrogen evolution activity of the photocatalyst.20
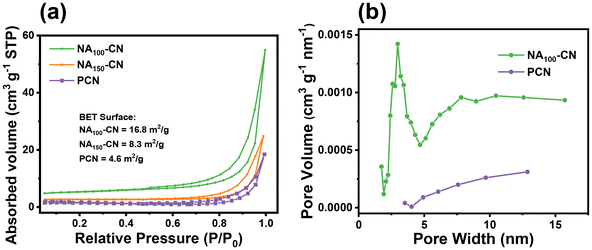 |
| Fig. 2 (a) N2 adsorption–desorption isothermal curves and (b) the pore size distribution of PCN and NA100-CN. | |
2.2 Crystal structure and chemical composition
As shown in Fig. 3a, both NAX-CN and PCN exhibit similar X-ray diffraction (XRD) patterns, featuring two standard diffraction peaks located at 13.2° and 27.1°, respectively. The peak at 13.2° represents the typical characteristic peak of the triazine ring structure, corresponding to the (100) plane of carbon nitride. The peak at 27.1° corresponds to the interlayer stacking of conjugated aromatic rings, representing the (002) plane of carbon nitride.21 Notably, the prepared NAX-CN materials do not exhibit any new diffraction peaks, indicating that the basic phase structure of the synthesized NAX-CN remains consistent with that of carbon nitride. Furthermore, it is observed that the diffraction peak intensity at 27.1° gradually decreases with the increase of the incorporation of pyridine heterocyclic rings, indicating that the intercalation of pyridine heterocyclic rings reduces the interlayer stacking strength of carbon nitride.13 Additionally, the diffraction peaks of XRD gradually shift to a lower angle with the embedding of pyridine heterocyclic rings, indicating that the interlayer accumulation of NAX-CN increases compared with PCN.22Fig. 3b presents the Fourier transform infrared (FT-IR) spectra of all samples. The spectra of NAX-CN and PCN are generally consistent, exhibiting stretching vibration peaks at 3000–3700 cm−1, 1200–1750 cm−1 and 810 cm−1. These peaks correspond to the O–H stretching vibration of water, the typical C–N or C
N stretching vibration of carbon–nitrogen heterocycles, and the breathing vibration of the triazine ring, respectively.23 As shown in Fig. 3c, the peak vibration signals of NAX-CN in the range of 1270–1750 cm−1 are significantly stronger than those of PCN. This is attributed to the stretching vibration of aromatic C–C bonds with a pyridine structure, further indicating the introduction of the pyridine heterocycle.24 As shown in Fig. S4,† we observed peaks as depicted in Fig. S4a,† located near 840 cm−1, which can be attributed to the breathing vibration of the pyridine heterocycle. Similarly, the peak appearing in Fig. S4b† around 1100 cm−1 can be ascribed to the in-plane bending vibration of C–H bonds within the pyridine heterocycle. The peak located near 1400 cm−1 is indicative of the C
N heterocyclic skeletal vibration of the pyridine heterocycle.10 Additionally, the peak around 1500 cm−1 corresponds to the aromatic C
C stretching vibration of the pyridine molecule in NA-CN, while the C–N stretching vibration of the pyridine ring falls within the range of approximately 1335–1250 cm−1, thus confirming the presence of pyridine rings within the doped PCN catalyst. To verify the light absorption capability of the materials, diffuse reflectance spectroscopy (DRS) was conducted on NAX-CN and PCN, as shown in Fig. 3d. The tubular structure of NA100-CN exhibits a pronounced quantum confinement effect, resulting in a distinct blue shift in its absorption edge. The UV-Vis DRS spectrum of NA100-CN shows an absorption edge at 430 nm, which is attributed to the grafting of a pyridine heterocycle at the edge of the polymer structure, resulting in an n → π* electronic transition.25 The absorbance of NA100-CN within the visible light absorption range (λ ≥ 450 nm) is significantly enhanced, which potentially contributes to the improvement of the photocatalytic reduction activity.
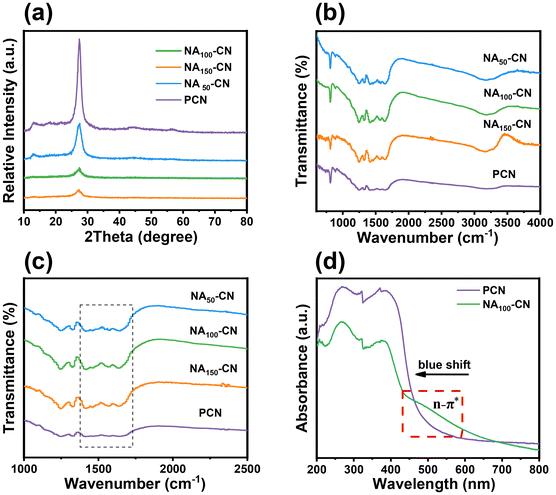 |
| Fig. 3 (a) XRD patterns of NAX-CN and PCN, (b) and (c) FT-IR spectra of NAX-CN and PCN, and (d) DRS spectra of NAX-CN and PCN. | |
Through X-ray photoelectron spectroscopy (XPS) analysis of the elemental states and chemical composition of PCN and NA100-CN materials (Fig. 4a), it was found that they are composed of C and N elements. The fitted high-resolution C 1s spectrum of the PCN catalyst (Fig. 4b) exhibits three main peaks located at 284.74 eV, 286.74 eV and 287.95 eV, corresponding to the C
C, C–NHX and C
N–C in the PCN framework, respectively.26–28 Notably, the C 1s spectrum of the NA100-CN catalyst after the introduction of pyridine heterocyclic rings also exhibits three peaks with binding energies of 284.75 eV, 286.8 eV and 288.05 eV. Compared to PCN, the binding energy of NA100-CN shifts towards higher values, which is attributed to the increased electron density of C atoms in NA100-CN resulting from the introduction of pyridine heterocyclic rings.29 Simultaneously, compared to PCN, the percentage of the C
N–C peak area relative to the total carbon atom peak area in NA100-CN decreases from 85.2% to 57.6%, while the C
C percentage increases from 10.4% to 35.6% (Table S1†). This is due to the introduction of the structural characteristics of the pyridine unit into the framework. The high-resolution N 1s spectrum of PCN exhibits three peaks with binding energies located at 398.40 eV, 399.09 eV and 400.86 eV, which correspond to C
N–C, N–(C)3 and C–NH2, respectively (Fig. 4c).30 In contrast, the N 1s spectrum of NA100-CN exhibits a fourth peak. Among them, three peaks at 398.61 eV, 400.01 eV and 401.06 eV are consistent with PCN. As depicted in Fig. S5a,† when comparing the O 1s spectrum of NA with that of NA100-CN, the latter exhibits a peak near 532.8 eV, which is indicative of the formation of C
O bonds. This confirms the presence of C
O bonds in NA100-CN and further substantiates the successful incorporation of pyridine rings into NA100-CN.31 In this study we conducted the elemental analysis of PCN and NA100-CN, respectively, and the element ratios are presented in Table S2.† The increase in the O and N content may be the result of the embedding of C
O bonds and C–N
C bonds in the pyridine molecules.
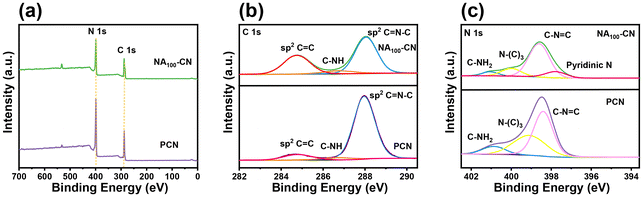 |
| Fig. 4 (a) XPS survey spectra of NA100-CN and PCN, (b) C 1s spectra, and (c) N 1s spectra of NA100-CN and PCN. | |
2.3 Analysis of the photocatalytic hydrogen production performance
Visible light-driven photocatalytic hydrogen evolution tests were conducted on the photocatalysts. As can be seen from Fig. 5a, the amount of photocatalytic hydrogen evolution increases linearly with the extension of the reaction time for all samples. The photocatalytic hydrogen evolution activity of NAX-CN (x = 50, 100, 150 mg) samples is significantly improved compared to PCN. In particular, NA100-CN exhibits the highest photocatalytic hydrogen production amount, reaching 2584.2 μmol g−1 h−1, which is approximately 5 times that of PCN. This allows us to determine the optimal amount of NA introduced. This is due to the fact that low nicotinic acid content can lead to the construction of a D–A-type structure, which can enhance the electron migration efficiency and reduce the recombination rate of photogenerated carriers.32 Too much NA leads to overdoping, and it becomes a recombination centre for photogenerated electrons and inhibits charge migration.33 Meanwhile, the Pt loading content also greatly influences the hydrogen generation performance of NA100-CN (Fig. S6†). The hydrogen generation rate increases when the Pt loading content is increased from 0.75% to 3%, and when the Pt loading content exceeds 6%, the hydrogen generation rate shows a decrease, so the optimized rate is acquired at 3% Pt over NA100-CN. In Fig. 5b, the effects of calcination after hydrothermal treatment and direct calcination without hydrothermal treatment on hydrogen production were studied. It was found that the photocatalytic performance of NA100-CN without the hydrothermal process was significantly lower than that of NA100-CN calcined after hydrothermal treatment. This suggests that the hydroxyl groups of NA can only be polymerized into the PCN framework through hydrogen bonding during the hydrothermal treatment process.34 The long-term stability and photostability of photocatalytic hydrogen evolution are also important indicators for evaluating the performance of photocatalysts.35 In addition, as shown in Fig. 5c, the cycling stability of the NA100-CN photocatalyst was tested continuously 5 times, for a total time of 25 h. NA100-CN demonstrated no significant attenuation during the 25 h of the cycling test, indicating that NA100-CN can maintain stable photocatalytic hydrogen evolution reactions and demonstrating its good stability and reproducibility. In Fig. 5d and Fig. S7,† the SEM images, XRD patterns and FT-IR spectra of NA100-CN before and after cycling are presented. It can be seen that the prepared NA100-CN photocatalyst has consistent patterns before and after the cycling experiment, indicating that there is no significant change in its structure. This demonstrates the stability of the phase structure of NA100-CN before and after the reaction, further proving the stability of the NA100-CN material.36 Meanwhile, hydrogen generation over NA100-CN far surpassed that of most reported donor–acceptor-structured PCN photocatalysts (Table S3†).
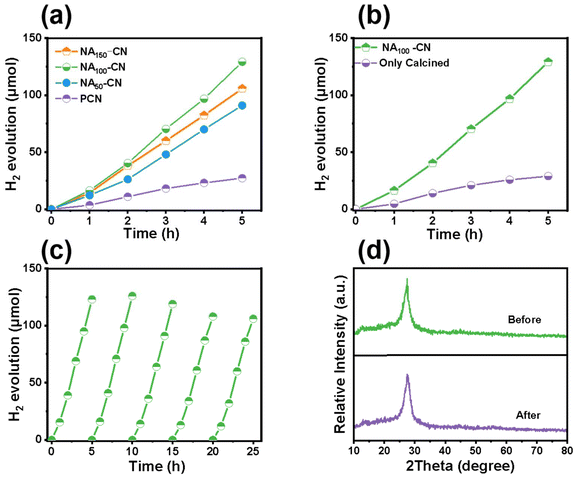 |
| Fig. 5 (a) Performance trends in water splitting for hydrogen production, (b) comparison between direct mixing and calcination versus hydrothermal calcination, (c) time course of H2 evolution for NA100-CN, and (d) XRD patterns of NA100-CN before and after 5 cycles. | |
2.4 Analysis of optical properties and charge dynamics
To further analyze the mechanism of photocatalysis, the separation, transport and recombination behavior of carriers in the samples was evaluated through photoelectrochemical methods. As shown in Fig. 6a, steady-state photoluminescence (PL) spectra indicate that the pristine PCN maintains a strong fluorescence intensity, suggesting recombination quenching of a significant number of photogenerated carriers in PCN.37 The reduction in fluorescence signal intensity for the photocatalyst indicates that the recombination rate of photogenerated electrons and holes can be effectively suppressed.38 Time-resolved PL spectra (Fig. S8†) show that NA100-CN (τavg = 11.89 ns) has the longest lifetime compared with that of PCN (τavg = 9.55 ns) and NA150-CN (τavg = 10.37 ns), illustrating NA100-CN offers the longest-lived charges for surface reactions.39 Compared to PCN, the NA100-CN exhibits significantly lower fluorescence intensity, attributed to the improved photogenerated carrier recombination in the constructed D–A polymer network structure.40 The electrochemical impedance spectroscopy (EIS) test (Fig. 6b) further demonstrates that NA100-CN has the smallest arc radius, indicating an enhanced photogenerated charge transport rate and increased electron diffusion mobility in NA100-CN.41,42 As shown in Fig. 6c, the photocurrent of NA100-CN is significantly stronger than that of PCN and NA150-CN, confirming that the electron transport efficiency of NA100-CN is highest.43,44 These experimental results suggest that NA100-CN can effectively improve the separation and transfer efficiency of photogenerated electrons and holes.
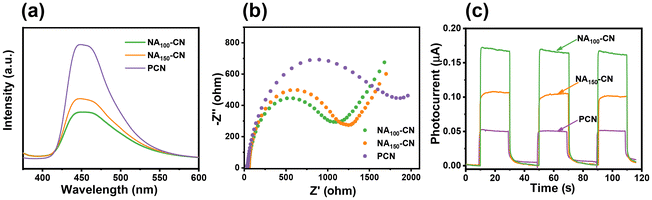 |
| Fig. 6 (a) Photoluminescence spectra, (b) EIS spectra in 0.1 M phosphate buffer and 5 mM K3[Fe(CN)6]/K4[Fe(CN)6] (pH = 7.0) and (c) visible light transient photocurrent response of PCN and NAX-CN in 0.1 M phosphate buffer (pH = 7.0) under visible-light irradiation. | |
2.5 Energy band structure and potential reaction mechanisms
As shown in Fig. 7a, Tauc plots were obtained from the DRS spectra using the Kubelka–Munk function to determine the bandgap width of the NA100-CN and PCN materials. The band gaps of NA100-CN and PCN are 2.69 eV and 2.63 eV, respectively. Additionally, the absorption edge of NAX-CN exhibits a blue shift compared to PCN, primarily due to the quantum confinement effect arising from the formation of a smaller tubular structure.45 The VB positions of PCN and NA100-CN were analyzed using XPS VB spectra (Fig. 7b and c). The VB value of PCN is 1.81 V, while the VB value of NA100-CN is 1.78 V. Based on the band gap and VB values, the CB value of PCN is calculated to be −0.82 V, and the CB value of NA100-CN is −0.91 V. An energy band structure diagram (Fig. 7d) was constructed based on the CB and VB positions. It is found that NA100-CN exhibits a stronger absolute value of the CB, indicating its excellent reductive capacity for hydrogen evolution, which is beneficial for enhancing the performance of photocatalytic hydrogen evolution.46
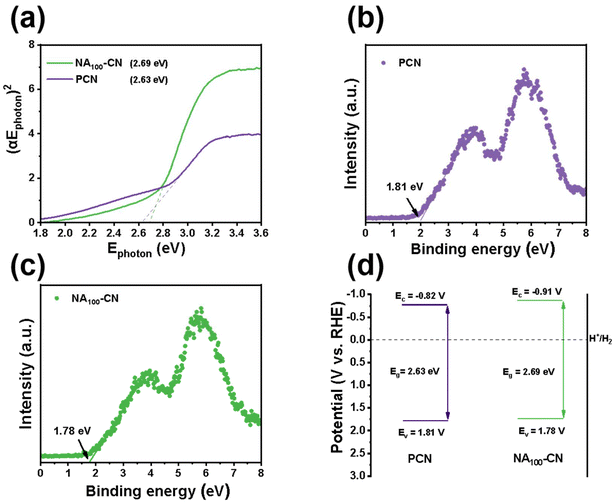 |
| Fig. 7 (a) Band gap spectra of PCN and NA100-CN, (b and c) VB spectra of PCN and NA100-CN, and (d) electronic band structure. | |
Based on the results of the aforementioned characterization studies, the reaction mechanism of NA100-CN can be inferred. Under visible light irradiation, the active sites on the surface of the catalyst are quickly occupied by the separated photogenerated carriers. Upon light excitation, electrons are generated at the conduction band (CB) of NA100-CN and holes are left behind at the valence band (VB) of NA100-CN. The photoelectrons are then involved in the photocatalytic hydrogen evolution and the remaining holes are consumed by triethanolamine (TEOA).31 As shown in Fig. 8, the absolute value of the reduction-terminal potential of NA100-CN is greater than that of PCN, endowing NA100-CN with a strong reductive capacity, which is more conducive to light excitation, thereby promoting electron transfer and the separation of photogenerated carriers. Additionally, the edge grafting of pyridine heterocyclic moieties leads to the construction of a D–A structure, establishing a directional electron transfer pathway that can effectively reduce the recombination of photogenerated carriers within the plane, contributing to improved photocatalytic hydrogen evolution performance.
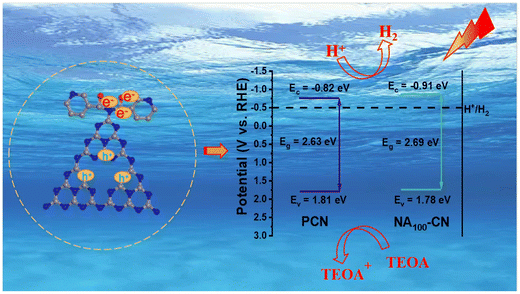 |
| Fig. 8 Photocatalytic hydrogen evolution mechanism of NA100-CN and PCN. | |
3. Conclusion
In summary, the precise grafting of pyridine heterocyclic rings onto the edges of the PCN framework through supramolecular self-assembly led to the formation of a D–A structure, effectively reducing the recombination rate of photogenerated electrons and holes. The one-dimensional tubular structure exhibited a larger surface area compared to PCN, providing more photocatalytically active sites on its surface and shortened diffusion paths for reactants and photogenerated carriers. These two factors synergistically enhanced the hydrogen evolution activity of the photocatalyst. Detailed analysis of the experimental results revealed that the introduction of pyridine heterocyclic rings increased the π-electron density, effectively promoting the separation of photogenerated electrons and holes, thereby enabling more electrons to participate in the reduction reaction. The results indicated that NAX-CN exhibited significantly enhanced photocatalytic activity under visible light irradiation, with a hydrogen evolution rate nearly 4.7 times higher than that of PCN, specifically 2584.2 μmol g−1 h−1 for NA100-CN. Based on these findings, it can be concluded that NAX-CN possesses effective carrier separation, high π-electron density and excellent light-capturing capabilities. This research provides insights into the grafting of organic small molecules onto the edges of the PCN framework and offers new possibilities for the development of efficient polymeric PCN-based photocatalysts.
Data availability
The data supporting this article have been included as part of the ESI.†
Conflicts of interest
There are no conflicts to declare.
Acknowledgements
This study was financially supported by the National Natural Science Foundation of China (22202086, 22208129), and the Huaian City Science and Technology Plan Project (HAG202303).
References
- S. Qin, J. Sha, P. Yang, S. Li, C. Liu and C. Shen, Graphene oxide/graphitic carbon nitride/polyamide oxime nanofibers for adsorption and photocatalytic reduction of uranium from seawater, Inorg. Chem. Front., 2024, 11, 6156–6167 RSC
.
- K. Zhong, P. Sun and H. Xu, Advances in Defect Engineering of Metal Oxides for Photocatalytic CO2 Reduction, Small, 2024, 2310677 CrossRef PubMed
.
- H. Zhang, Y. Wang, B. Zhang, S. Zhang, Y. Ma, Z. Wu, Y. Zhu, F. Liu, Z. Xiao and L. Wang, Interwoven N-doped carbon nanotubes with capped Ni-doped FeP as double-functional electrocatalysts for overall seawater electrolysis, Sci. China Mater., 2023, 66, 4630–4638 CrossRef CAS
.
- J. Pan, D. Wang, D. Wu, J. Cao, X. Fang, C. Zhao, Z. Zeng, B. Zhang, D. Liu, S. Liu, G. Liu, S. Jiao, Z. Xu, L. Zhao and J. Wang, Rational Design of Three Dimensional Hollow Heterojunctions for Efficient Photocatalytic Hydrogen Evolution Applications, Adv. Sci., 2024, 11, e2309293 CrossRef PubMed
.
- X. Lu, I. Ullah, J. Li, S. Chen, C. Yuan and A. Xu, A bimetallic CoZn metal-organic-framework derived CoZnS@NSC Co-catalyst loaded on g-C3N4 for significantly augmented photocatalytic H2 evolution, Inorg. Chem. Front., 2024, 11, 3435 RSC
.
- J. Dong, J. Zhao, X. Yan, L. Li, G. Liu, M. Ji, B. Wang, Y. She, H. Li and J. Xia, Construction of carbonized polymer dots/potassium doped carbon nitride nanosheets van der Waals heterojunction by ball milling method for facilitating photocatalytic CO2 reduction performance in pure water, Appl. Catal., B, 2024, 351, 123993 CrossRef CAS
.
- M. Ren, J. Meng, Y. Yang, X. Zhang, G. Yang, L. Qin and Y. Guo, Synergy between palladium single atoms and small nanoparticles co-anchored on carbon atom self-doped graphitic carbon nitride boosting photocatalytic H2 generation, Appl. Catal., B, 2024, 345, 123680 CrossRef CAS
.
- D. Wen, Y. Su, J. Fang, D. Zheng, Y. Xu, S. Zhou, A. Meng, P. Han and C.-P. Wong, Synergistically boosted photocatalytic production of hydrogen peroxide via protonation and oxygen doping on graphitic carbon nitride, Nano Energy, 2023, 117, 108917 CrossRef CAS
.
- P. Wu, P. Sui, G. Peng, Z. Sun, F. Liu, W. Yao, H. Jin and S. Lin, Designable Photo–Responsive Micron–Scale Ultrathin Peptoid Nanobelts for Enhanced Performance on Hydrogen Evolution Reaction, Adv. Mater., 2024, 36, e2312724 CrossRef PubMed
.
- H. Guo, L. Zhou, K. Huang, Y. Li, W. Hou, H. Liao, C. Lian, S. Yang, D. Wu, Z. Lei, Z. Liu and L. Wang, Nitrogen-Rich Carbon Dot-Mediated n→π* Electronic Transition in Carbon Nitride for Superior Photocatalytic Hydrogen Peroxide Production, Adv. Funct. Mater., 2024, 34, 2402650 CrossRef CAS
.
- X. Lin, H. Du, D. Jiang, P. Zhang, Z. Yu, H. Bi and Y. Yuan, Microwave awakening the n-π* electronic transition in highly crystalline polymeric carbon nitride nanosheets for photocatalytic hydrogen generation, J. Energy Chem., 2022, 65, 541–547 CrossRef CAS
.
- S. An, G. Zhang, K. Li, Z. Huang, X. Wang, Y. Guo, J. Hou, C. Song and X. Guo, Self-Supporting 3D Carbon Nitride with Tunable n→π* Electronic Transition for Enhanced Solar Hydrogen Production, Adv. Mater., 2021, 33, 2104361 CrossRef CAS PubMed
.
- T. Bhoyar, D. Kim, B. Abraham, S. Tonda, N. Manwar, D. Vidyasagar and S. Umare, Tailoring photoactivity of polymeric carbon nitride via donor-π-acceptor network, Appl. Catal., B, 2022, 310, 121347 CrossRef CAS
.
- M. Gao, F. Tian, X. Zhang, Z. Chen, W. Yang and Y. Yu, Improved Plasmonic Hot-Electron Capture in Au Nanoparticle/Polymeric Carbon Nitride by Pt Single Atoms for Broad-Spectrum Photocatalytic H2 Evolution, Nano-Micro Lett., 2023, 15, 129 CrossRef CAS PubMed
.
- Y. Wang, F. Dai, Y. Tao, K. Zhang, B. Li, M. Zhang, K. Liu, J. Xu, L. Wang and J. Xing, Understanding the synthesis mechanism, chemical structures and optical properties of aromatic carbon nitride, Inorg. Chem. Front., 2024, 11, 2346 RSC
.
- Y. Yao, Y. Wang, T. Lu, J. Zhang, K. Hu, H. Zhang, T. Pukala, Y. Liu, X. Duan and S. Wang, In-plane implanting carbon rings into carbon nitride to intrigue nonradical photodegradation, Appl. Catal., B, 2024, 342, 123363 CrossRef CAS
.
- Z. Xie, Y. Jia, Y. Huang, D. Xu, X. Wu, M. Chen and W. Shi, Near-Infrared Light-Driven Photocatalytic Reforming Lignocellulose into H2 and Chemicals over Heterogeneous Carbon Nitride, ACS Catal., 2023, 13, 13768–13776 CrossRef CAS
.
- S. Lv, Y. Deng, Q. Liu, Z. Fu, X. Liu, M. Wang, Z. Xiao, B. Li and L. Wang, Carbon-quantum-dots-involved Fe/Co/Ni phosphide open nanotubes for high effective seawater electrocatalytic decomposition, Appl. Catal., B, 2023, 326, 122403 CrossRef CAS
.
- X. Hao, Y. Fan, W. Deng and Z. Jin, In situ XPS demonstrated efficient charge transfer of ohmic junctions based on graphdiyne (g-CnH2n−2) nanosheets coupled with porous nanoflowers Ni5P4 for efficient photocatalytic H2 evolution, Carbon, 2024, 218, 118752 CrossRef CAS
.
- Z. Yang, T. Huang, M. Li, X. Wang, X. Zhou, S. Yang, Q. Gao, X. Cai, Y. Liu, Y. Fang, Y. Wang, S. Zhang and S. Zhang, Unveiling the Synergistic Role of Frustrated Lewis Pairs in Carbon–Encapsulated Ni/NiOx Photothermal Cocatalyst for Enhanced Photocatalytic Hydrogen Production, Adv. Mater., 2024, e2313513 CrossRef PubMed
.
- Y. Chen, Y. Ge, Y. Yan, L. Xu, X. Zhu, P. Yan, P. Ding, H. Li and H. Li, Photoinduced Zn-Air Battery-Assisted Self-Powered Sensor Utilizing Cobalt and Sulfur Co-Doped Carbon Nitride for Portable Detection Device, Adv. Sci., 2024, 2408293 CrossRef PubMed
.
- J. Yang, L. Jing, X. Zhu, W. Zhang, J. Deng, Y. She, K. Nie, Y. Wei, H. Li and H. Xu, Modulating electronic structure of lattice O-modified orange polymeric carbon nitrogen to promote photocatalytic CO2 conversion, Appl. Catal., B, 2024, 340, 122005 Search PubMed
.
- X. Hao, W. Deng, Y. Fan and Z. Jin, Engineering of a hierarchical S-scheme 2D/3D heterojunction with graphdiyne (g-CnH2n−2) coated 3D porous CoAl2O4 nanoflowers for highly efficient photocatalytic H2 evolution, J. Mater. Chem. A, 2024, 12, 8543–8560 RSC
.
- D. Jiang, S. Hu, Y. Qu, X. Tian, H. Du, C. Zhu, Z. Li, L. Yin, Y. Yuan and G. Liu, Infrared Irradiation–Lattice Vibration Coupling–Initiated n→π* Electronic Transition in Carbon Nitride Nanosheets for Increased Photocatalysis, Adv. Funct. Mater., 2024, 34, 2311803 CrossRef CAS
.
- C. Xue, P. Wang, H. Che, W. Liu, B. Liu and Y. Ao, Simultaneous organic pollutant degradation and hydrogen peroxide production by molecular-engineered carbon nitride, Appl. Catal., B, 2024, 340, 123259 CrossRef CAS
.
- G. Wu, Z. He, Q. Wang, H. Wang, Z. Wang, P. Sun, Z. Mo, H. Liu and H. Xu, Non-metal inducing charge rearrangement in carbon nitride to promote photocatalytic hydrogen production, J. Mater. Sci. Technol., 2024, 195, 1–8 CrossRef
.
- X. Chen, Y. Shi, Y. Cai, J. Xie, Y. Yang, D. Hou and Y. Fan, Effect of non–thermal plasma injection flow rate on diesel particulate filter regeneration at room temperature, Carbon Lett., 2024, 34, 1075–1089 CrossRef
.
- Y. Luo, Y. Shi, K. Zhuang, R. Ji, X. Chen, Y. Huang, Z. Wang, Y. Cai and X. Li, Study on the Evolution of Physicochemical Properties of Carbon Black at Different Regeneration Stages of Diesel Particulate Filters Regenerated by Non-Thermal Plasma, Processes, 2024, 12, 1113 CrossRef CAS
.
- M. Zhou, H. Wang, R. Liu, Z. Liu, X. Xiao, W. Li, C. Gao, Z. Lu, Z. Jiang, W. Shi and Y. Xiong, Construction of Frustrated Lewis Pairs in Poly(heptazine Imide) Nanosheets via Hydrogen Bonds for Boosting CO2 Photoreduction, Angew. Chem., Int. Ed., 2024, 202407468 Search PubMed
.
- P. Sun, Z. Chen, J. Zhang, G. Wu, Y. Song, Z. Miao, K. Zhong, L. Huang, Z. Mo and H. Xu, Simultaneously tuning electronic reaction pathway and photoactivity of P,O modified cyano-rich carbon nitride enhances the photosynthesis of H2O2, Appl. Catal., B, 2024, 342, 123337 CrossRef CAS
.
- A. Sikandaier, Y. Zhu and D. Yang, In-situ decorated cobalt phosphide cocatalyst on Hittorf's phosphorus triggering efficient photocatalytic hydrogen production, Chin. J. Struct. Chem., 2024, 43, 100242 CrossRef CAS
.
- X. Gong, F. Ma, Y. Zhang, Y. Li, Z. Wang, Y. Liu, P. Wang, H. Cheng, Y. Dai, Y. Fan, B. Huang and Z. Zheng, In Situ Construction of an Intramolecular Donor-Acceptor Conjugated Copolymer via Terephthalic Acid Derived from Plastic Waste for Photocatalysis of Plastic to Hydrogen Peroxide, ACS Catal., 2023, 13, 12338–12349 CrossRef CAS
.
- G. Zhang, W. Huang, Y. Xu, Y. Li, C. He, X. Ren, P. Zhang and H. Mi, Suppressing Defects–Induced Non–Radiative Recombination for Activating the Near–Infrared Photoactivity of Red Polymeric Carbon Nitride, Adv. Funct. Mater., 2023, 33, 2305935 CrossRef CAS
.
- Y. Zhong, W. Dong, S. Ren and L. Li, Oligo(phenylenevinylene)–Based Covalent Organic Frameworks with Kagome Lattice for Boosting Photocatalytic Hydrogen Evolution, Adv. Mater., 2023, 36, e2308251 CrossRef PubMed
.
- J. Ning, B. Zhang, L. Siqin, G. Liu, Q. Wu, S. Xue, T. Shao, F. Zhang, W. Zhang and X. Liu, Designing advanced S-scheme CdS QDs/La-Bi2WO6 photocatalysts for efficient degradation of RhB, Exploration, 2023, 3, 20230050 CrossRef PubMed
.
- Z. Zhu, X. Xing, Q. Qi, W. Shen, H. Wu, D. Li, B. Li, J. Liang, X. Tang, J. Zhao, H. Li and P. Huo, Fabrication of graphene modified CeO2/g-C3N4 heterostructures for photocatalytic degradation of organic pollutants, Chin. J. Struct. Chem., 2023, 42, 100194 CrossRef CAS
.
- G. Wang, C. Zhang, W. Zhao, B. Wang, Y. Liu, T. Zhang, W. Cui, R. Zhang and Z. Zhao, Bonding Interaction of Adjacent Pt and Ag Single-Atom Pairs on Carbon Nitride Efficiently Promotes Photocatalytic H2 Production, CCS Chem., 2024, 6, 1523–1534 CrossRef CAS
.
- Q. Wang, Q. Liu, Y. Ma, H. Bi, J. Du and Z. Han, A photocatalyst with an enhanced Schottky effect and an efficient electron-transfer channel fabricated by assembling Ni-WC heterojunction nanoparticles on g-C3N4 for highly efficient removal of chlorophenols, Inorg. Chem. Front., 2024, 11, 1238 RSC
.
- G. Chen, Z. Zhou, B. Li, X. Lin, C. Yang, Y. Fang, W. Lin, Y. Hou, G. Zhang and S. Wang, S-scheme heterojunction of crystalline carbon nitride nanosheets and ultrafine WO3 nanoparticles for photocatalytic CO2 reduction, J. Environ. Sci., 2024, 140, 103–112 CrossRef CAS PubMed
.
- X. Yang, Q. Shen, W. Cao, B. Xu, Y. Sun and C. Li, In-situ template etching synthesis of BiON/BiOCl0.9I0.1 heterojunction for photocatalytic degradation of tetracycline, ChemPhysMater, 2024, 3, 103–110 CrossRef
.
- Y. Liu, Z. Zheng, S. Jabeen, N. Liu, Y. Liu, Y. Cheng, Y. Li, J. Yu, X. Wu, N. Yan, L. Xu and H. Li, Mechanochemical route to fabricate an efficient nitrate reduction electrocatalyst, Nano Res., 2024, 17, 4889–4897 CrossRef CAS
.
- H. Zhang, Y. Wu, X. Wang, C. Li, Z. Xiao, Y. Liu, Y. Deng, Z. Li and L. Wang, The construction of defect-rich CoP@CoP@(Co/Ni)2P triple-shell hollow nanospheres with boosted electrocatalytic hydrogen evolution performances over a wide pH range, Chem. Eng. J., 2023, 463, 142448 CrossRef CAS
.
- P. Yan, J. Huang, G. Wu, Y. Zhang, Z. Mo, K. Xu, M. Ling, S. Dong, L. Xu and H. Li, Construction of a In2O3/ultrathin g-C3N4 S-scheme heterojunction for sensitive photoelectrochemical aptasensing of diazinon, J. Colloid Interface Sci., 2025, 679, 653–661 CrossRef CAS PubMed
.
- Z. Zhou, W. Guo, T. Yang, D. Zheng, Y. Fang, X. Lin, Y. Hou, G. Zhang and S. Wang, Defect and nanostructure engineering of polymeric carbon nitride for visible-light-driven CO2 reduction, Chin. J. Struct. Chem., 2024, 43, 100245 CrossRef CAS
.
- E. Hu, Q. Chen, Q. Gao, X. Fan, X. Luo, Y. Wei, G. Wu, H. Deng, S. Xu, P. Wang, L. Liu, R. He, X. Chen, W. Zhu and Y. Zhu, Cyano–Functionalized Graphitic Carbon Nitride with Adsorption and Photoreduction Isosite Achieving Efficient Uranium Extraction from Seawater, Adv. Funct. Mater., 2024, 34, 2312215 CrossRef CAS
.
- Z. Mo, Z. Miao, P. Yan, P. Sun, G. Wu, X. Zhu, C. Ding, Q. Zhu, Y. Lei and H. Xu, Electronic and energy level structural engineering of graphitic carbon nitride nanotubes with B and S co-doping for photocatalytic hydrogen evolution, J. Colloid Interface Sci., 2023, 645, 525–532 CrossRef CAS PubMed
.
|
This journal is © the Partner Organisations 2025 |
Click here to see how this site uses Cookies. View our privacy policy here.