DOI:
10.1039/D4QI02645D
(Research Article)
Inorg. Chem. Front., 2025,
12, 253-260
Magneto-optical response and luminescence properties of lanthanide–titanium–oxo clusters Eu2Ti7 and Sm2Ti7†
Received
20th October 2024
, Accepted 14th November 2024
First published on 21st November 2024
Abstract
The study of magneto-optical effects based on the f–f emission and absorption of lanthanide ions has attracted considerable interest. In this work, we present a series of isostructural lanthanide–titanium–oxo clusters (LTOCs) Ln2Ti7 (Ln = La, Sm, Eu) using 3,5-di-tert-butylbenzoic acid as the ligand. A detailed comparison of the luminescence properties of Sm2Ti7 and Eu2Ti7 shows that Eu2Ti7 displays superior luminescence intensity, higher color purity red light, longer lifetime, and significantly higher quantum yield. These properties, along with its high stability in solution, make Eu2Ti7 an excellent candidate for magnetic circularly polarized luminescence (MCPL) studies. Under an external magnetic field, Eu2Ti7 exhibited strong MCPL signals, with the maximum |gMCPL| value being 0.04 T−1 from the 5D0 → 7F4 transition. In contrast, the weaker luminescence of Sm2Ti7 rendered MCPL analysis ineffective; however, its strong near-infrared absorption allowed for magnetic circular dichroism (MCD) studies. The MCD spectra of Sm2Ti7 revealed significant signals corresponding to f–f transitions in the 900–1600 nm range, with the maximum |gMCD| value observed at 1102 nm. This work provides valuable insights into the magneto-optical properties of Ln-based clusters, emphasizing the role of energy-level analysis for further research into their potential applications in magneto-optical devices.
10th anniversary statement
It is a great honor to contribute to the 10th anniversary collection of this esteemed journal. I had the privilege of publishing a paper on lanthanide-oxo clusters in the Emerging Investigator themed collection of Inorganic Chemistry Frontiers in 2016 (Inorg. Chem. Front., 2016, 3, 320–325). Over the years, this journal has provided an invaluable platform for reporting groundbreaking research and encouraging collaborations within China, across Asia, and internationally. Inorganic Chemistry Frontiers has played a vital role in advancing the field of inorganic chemistry. I look forward to the journal's continued growth and influence.
|
Introduction
Most trivalent lanthanide ions possess a rich array of uniquely arranged electron energy levels, which result in characteristic f–f absorption and emission transitions.1 These features make Ln(III) highly valuable in spectroscopy, particularly in the unique magneto-optical properties.2 An external magnetic field can induce optical activity and magneto-optical effects in both achiral and optically inactive substances, such as the Faraday effect, Zeeman effect, and polar Kerr effect.3 The interaction of light with matter in an external magnetic field is particularly noteworthy because it can lead to magnetic circular dichroism (MCD) in the ground state and magnetic circularly polarized luminescence (MCPL) in the excited state. This phenomenon arises from the Zeeman splitting in their degenerate ground and excited states of achiral and optically inactive substances.4
The design and synthesis of molecules containing Ln(III) with well-defined structure are essential for studying the magneto-optical effect of these ions.5 In the selected molecular model for investigating the magneto-optical behavior of Ln(III), the MCPL and MCD signals of Ln(III) can be obtained by choosing suitable ligands, regulating the coordination environment of Ln(III), and minimizing interference with f–f transitions.6 The 3d–4f clusters have the characteristics of multiple metal centers, allowing for synergistic interactions between different metal ions.7 However, 3d–4f clusters with both absorption and emission magneto-optical effects are extremely rare. Current studies of MCPL in lanthanide systems have mostly focused on mononuclear complexes,8 with the exception of the Ln20 cluster,9 and investigations into MCD in the near-infrared range remain limited.10
Lanthanide–titanium–oxo clusters (LTOCs) represent a distinct class of 3d–4f clusters. Their core structures, due to the presence of Ti(IV) with strong Lewis acidity, allow the metal ions to be commonly interconnected via O2− bridges, which helps mitigate the negative impact of O–H stretching vibrations on Ln(III) luminescence.11 By selecting efficient photosensitive ligands, LTOCs with excellent luminescence properties can be achieved.12 These clusters have recently emerged as promising candidates for Ln(III)-based luminescent materials. Furthermore, since Ti(IV) lacks d-electrons, there are no d–d transitions in the absorption spectra, ensuring no interference with the f–f transitions of the lanthanide ions.13 LTOCs provide new alternatives for the study of MCPL and MCD.
Herein, based on bis-tert-butyl modified carboxylic acid ligands, we report a series of lanthanide–titanium–oxo clusters, formulated as Ln2Ti7(μ3-O)6(L)14(EtO)8 (Ln2Ti7, Ln = La, Sm, Eu; HL = 3,5-di-tert-butylbenzoic acid). The introduction of tert-butyl groups enhances the ligand sensitization effect and improves the solubility of the cluster. Luminescence studies reveal that Eu2Ti7 exhibits superior luminescence properties compared to Sm2Ti7, with a longer lifetime and higher quantum yield. The excellent solubility and stability of the cluster in dichloromethane make it suitable for further MCPL study of Eu2Ti7 and near-infrared MCD study of Sm2Ti7. Notably, achiral Eu2Ti7 displays distinct and well-defined CPL signals in the external magnetic field. The energy level analysis prompted the study of the MCD of Sm2Ti7 in the near-infrared region, which also yielded a signal with a near-ideal peak shape. This work marks the first investigation of both emission and absorption magneto-optical effects within the same 3d–4f cluster system.
Experimental section
Materials and physical measurements
All reagents were of analytical grade, commercially sourced, and were used without further purification. The crystallographic data were collected on a Rigaku Oxford diffraction XtaLAB synergy diffractometer with micro-focus sealed X-ray Cu Kα radiation (λ = 1.54184 Å) at 100 K. The excitation and emission spectra of the room-temperature luminescence, the time-resolved PL decay curves and the absolute quantum yields were measured on the steady-state and transient fluorescence spectrometer (FLS-1000, Edinburgh). Dynamic light scattering analysis was conducted using the laser particle size analyzer (Malvern, ZSU3100). The MCPL and MCD spectra were measured on the JASCO CPL-300 and JASCO J-1700 equipped with a JASCO PM-491 compact permanent magnet (1.6 T).
Synthesis of Ln2Ti7
Clusters Ln2Ti7 (Ln = La, Sm, Eu) were synthesized through solvothermal crystallization by reacting Ln(OAc)3·xH2O, Ti(OiPr)4, and 3,5-di-tert-butylbenzoic acid in ethanol. The solid reactants were fully dissolved by sonication in the sealed vials, followed by solvothermal reactions at 80 °C for 24–48 h. The colorless block crystals were obtained without cooling to room temperature, with yields exceeding 40% (calculated based on Ln(OAc)3·xH2O).
Results and discussion
Single crystal structures
Single-crystal X-ray diffraction (SCXRD) analysis revealed that the three obtained clusters, Ln2Ti7(μ3-O)6(EtO)8(L)14 (Ln2Ti7, Ln = La, Sm, Eu; HL = 3,5-di-tert-butylbenzoic acid), are electrically neutral and isostructural clusters, though their cell parameters differ. La2Ti7 and Sm2Ti7 crystallize in the triclinic crystal system with the space group P
, while Eu2Ti7 crystallizes in the monoclinic crystal system with the space group C2/c. To illustrate their structural features, we discuss in detail only the molecular structure of La2Ti7. As shown in Fig. 1a, the metal-oxo core [La2Ti7(μ3-O)6]22+ is stabilized by 14 L− anions and 8 EtO− anions. The metal-oxo core consists of two eight-coordinated La3+ ions and seven six-coordinated Ti4+ ions, connected by 6 μ3-O2− bridges (Fig. 1b and c). Interestingly, the previously reported cluster LaTi4, obtained using benzoic acid ligands, features a [LaTi4(μ3-O)3]13+ metal-oxo core (Fig. S2†).14 The La2Ti7 can be envisioned as two [LaTi4(μ3-O)3] units sharing a Ti4+ ion in a “hand in hand” arrangement. In each [LaTi4(μ3-O)3] unit, the four Ti4+ ions form a non-linear circular arc, with the La3+ surrounded by Ti4+ bridged through O2− bridges. The bond distances and angles (Tables S5–S12†) are consistent with literature values. Notably, the molecular model of Ln2Ti7 lacks OH− bridges and features fourteen photosensitive ligands, making it suitable for luminescence studies.
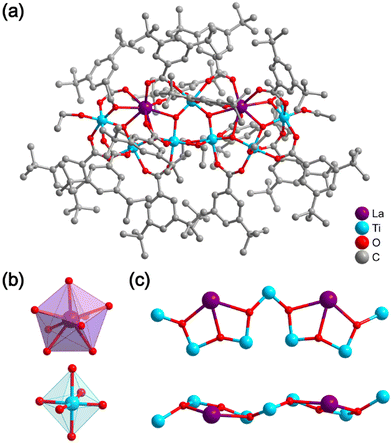 |
| Fig. 1 (a) Crystal structure of La2Ti7. (b) Coordination configurations of La3+ ion and Ti4+ ion. (c) Ball-and-stick depiction of metal-oxo core [La2Ti7(μ3-O)6]22+. | |
Luminescence properties
Sm2Ti7 and Eu2Ti7 were selected for luminescence studies due to energy levels of Sm(III) and Eu(III) favorable match with the chosen photosensitive ligands. Room-temperature luminescence measurements of Sm2Ti7 and Eu2Ti7 at room temperature were performed, with the results presented in Fig. 2. Fig. 2a displays the excitation and emission spectra of Sm2Ti7, which exhibits Sm(III) characteristic emission peaks at 562 nm (4G5/2 → 6H5/2), 597 nm (4G5/2 → 6H7/2), 644 nm (4G5/2 → 6H9/2), and 706 nm (4G5/2 → 6H11/2) upon irradiation at 339 nm or 405 nm (6H5/2 → 6P3/2).15Fig. 2b presents the excitation and emission spectra of Eu2Ti7, where irradiation at 339 nm or 395 nm (7F0 → 5L6) leads to characteristic Eu(III) emissions at 580 nm (5D0 → 7F0), 593 nm (5D0 → 7F1), 618 nm (5D0 → 7F2), 650 nm (5D0 → 7F3) and 701 nm (5D0 → 7F4).16 In Fig. 2c, the relative emission intensities of Sm2Ti7 and Eu2Ti7 are compared, along with a reference for emission peak widths. The luminescence of Sm2Ti7 is primarily dominated by two f–f transitions: 4G5/2 → 6H7/2 and 4G5/2 → 6H9/2, whereas the luminescence of Eu2Ti7 is predominantly driven by the 5D0 → 7F2 transition. The emission peak widths of Sm2Ti7 are broader compared to Eu2Ti7, indicating higher color purity for Eu2Ti7.
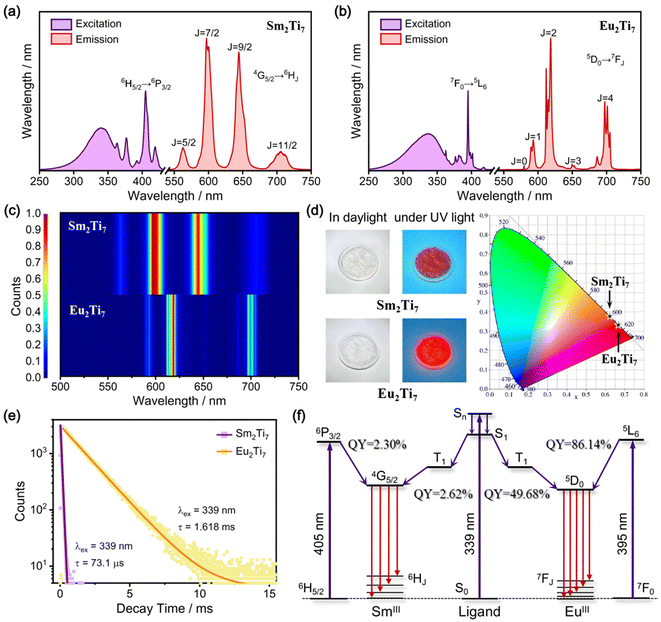 |
| Fig. 2 (a) Excitation and emission spectra of Sm2Ti7. (b) Excitation and emission spectra of Eu2Ti7. (c) Relative emission intensities of Sm2Ti7 and Eu2Ti7. (d) Physical photographs and CIE color coordinates of Sm2Ti7 and Eu2Ti7. (e) Excited-state decay curves of Sm2Ti7 and Eu2Ti7. (f) Quantum yield results of Sm2Ti7 and Eu2Ti7. | |
Under daylight, the crystal samples of Sm2Ti7 and Eu2Ti7 appear colorless, but exhibit red luminescence when exposed to ultraviolet light. The CIE color coordinates for Sm2Ti7 are (x = 0.6179, y = 0.3815), and for Eu2Ti7 are (x = 0.6632, y = 0.3365). Both coordinates fall within the orange-red region of the CIE chromaticity diagram, consistent with the observed luminescence. Notably, there is a visible difference in luminous intensity between the two samples, with Eu2Ti7 exhibiting significantly stronger emission than Sm2Ti7 (Fig. 2d). The excited-state decay curves, presented in Fig. 2e, indicate lifetimes of 72.6 μs (χ2 = 1.1564) for Sm2Ti7 and 1.618 ms (χ2 = 1.0182) for Eu2Ti7.
The photo-luminescence process in lanthanide coordination system generally involves three stages: energy absorption, energy transfer to the Ln(III) emission level, and the subsequent f–f transitions that produce luminescence. Energy absorption occurs primarily through photosensitive ligands or intrinsic f–f transitions of Ln(III). Different absorption modes correspond to distinct energy transfer pathways.17 In the first case, upon the ligand's absorption of energy, the energy populates the excited singlet energy level (Sn) of the ligand. Subsequently, it relaxes to the lowest singlet energy level (S1) of the ligand. Then, intersystem crossing (ISC) takes place, leading to the energy populating the lowest triplet energy level (T1) of the ligand. Afterwards, energy is transferred from the T1 level to the excited state energy level of Ln(III). Once the energy reaches the emission energy level of Ln(III), radiative emission occurs. In the second case, Ln(III) absorbs energy via its intrinsic f–f transition, attaining a high excited state energy level of Ln(III). Subsequently, the energy relaxes to the emission level of Ln(III) through a non-radiative decay process and then radiative emission takes place. In the excitation spectra of Sm2Ti7 and Eu2Ti7, strong and broad excitation peaks are attributed to ligand-based energy absorption, while strong but narrow excitation peaks arise from direct f–f transitions. Quantum yields (QYs) for Sm2Ti7 and Eu2Ti7 were measured for the two excitation modes at their strongest excitation wavelengths (Fig. 2f). Under ligand-sensitized excitation (339 nm), the QYs of Sm2Ti7 and Eu2Ti7 are 2.62% and 49.68%, respectively. For Sm2Ti7, when excited by the strongest f–f transition 6H5/2 → 6P3/2 (excitation wavelength at 405 nm), the QY is 2.30%. For Eu2Ti7, when excited by the strongest f–f transition 7F0 → 5L6 (excitation wavelength at 395 nm), the QY is 86.14%.
Luminescence studies reveal that Eu2Ti7 exhibits stronger emission intensity, higher color purity red light, longer luminescence lifetime and higher luminescence efficiency, compared to Sm2Ti7 (Table 1). The difference in luminescence performance between Eu2Ti7 and Sm2Ti7 can be understood by analyzing their energy levels. When considering the ligand sensitization pathway, the emission levels of Sm(III) and Eu(III) are quite close (4G5/2: 17
924 cm−1; 5D0: 17
286 cm−1). This suggests that the ligand absorption and energy transfer processes leading to the emission levels should be similar in both Sm2Ti7 and Eu2Ti7. However, the lower QY of Sm2Ti7 points to significant energy loss during the transition from the emission level (4G5/2) to the lower levels (6HJ). A comparison of energy level diagrams reveals that Eu(III) transitions directly from the 5D0 level to the 7FJ levels without intermediate excited states. In contrast, Sm(III) has additional intermediate states (6FJ) between the 4G5/2 and 6HJ levels. These intermediate states allow for non-radiative decay, contributing to energy loss and negatively impacting the luminescence of Sm(III). Interestingly, f–f transitions from the ground state (6H5/2) to these intermediate excited state levels were observed in the diffuse reflection spectrum of Sm2Ti7, showing strong absorption in the near-infrared region (Fig. S9c†).
Table 1 Results of the luminescence properties of Ln2Ti7
Ln2Ti7 |
f–f transitions & emission peaks |
CIE |
Lifetime |
QY (Ex) |
Sm2Ti7
|
4G5/2 → 6HJ |
(x = 0.6179, y = 0.3815) |
72.6 μs |
2.3% (405 nm), 2.6% (339 nm) |
562 nm (J = 5/2), 597 nm (J = 7/2), 644 nm (J = 9/2), 706 nm (J = 11/2) |
Eu2Ti7
|
5D0 → 7FJ |
(x = 0.6632, y = 0.3365) |
1.618 ms |
86.1% (395 nm), 49.7% (339 nm) |
580 nm (J = 0), 593 nm (J = 1), 618 nm (J = 2), 650 nm (J = 3), 701 nm (J = 4) |
Stability in solution
Eu2Ti7 was chosen as a representative to validate the stability of Ln2Ti7 in dichloromethane (CH2Cl2). Dynamic light scattering (DLS) analysis revealed an average particle size of Eu2Ti7 at 2.1 ± 0.5 nm (Fig. 3a), consistent with the theoretical particle size determined by single crystal structure analysis. To further assess the stability of Eu2Ti7, emission spectra were characterized at various concentrations, ranging from 10–2 to 10–5 M. The large solubility range benefits from the modification of the tert-butyl groups on the ligands. The results showed that the Eu2Ti7 solution emits red light under ultraviolet irradiation, with emission peaks consistent with the characteristic Eu(III) transitions observed in the solid state (Fig. 3b). As the concentration decreased, the emission intensity weakened, but the spectral shape and peak positions remained unchanged. This consistency suggests that Eu2Ti7 retains its structural integrity in CH2Cl2. Interestingly, after allowing the CH2Cl2 solution of Eu2Ti7 to evaporate, colorless block crystals formed. SCXRD analysis confirmed that these crystals were Eu2Ti7, but with a change in space group from C2/c to P
(Fig. 3c), likely due to the incorporation of CH2Cl2 molecules into the crystal lattice (Fig. S1c†). The recrystallized of Eu2Ti7 also highlights the solubility and stability in CH2Cl2.
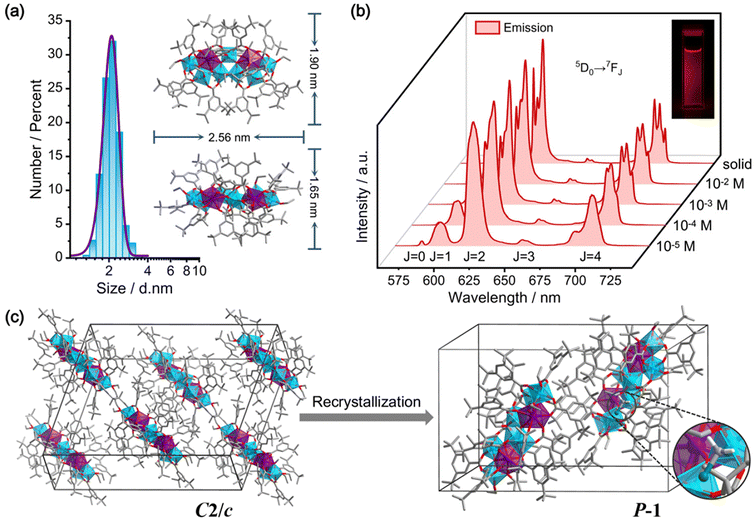 |
| Fig. 3 (a) Dynamic light scattering analysis of Eu2Ti7. (b) Emission spectra of Eu2Ti7 solutions at various concentrations. (c) The space group transitioned from C2/c to P after recrystallization of Eu2Ti7. | |
Magneto-optical response
Given the remarkable luminescence properties and high stability of Eu2Ti7, the magnetic circularly polarized luminescence (MCPL) properties were studied in CH2Cl2 solution under an external magnetic field of 1.6 T. A CH2Cl2 solution of Eu2Ti7 with a concentration of 1 × 10–3 M was prepared. As illustrated in Fig. 4a, Eu2Ti7 displays distinct MCPL spectra (5D0 → 7FJ) upon excitation at 328 nm (Fig. S7†), with significant CPL signals (ΔI) observed in the wavelength ranges of 585–605 nm (J = 1), 605–630 nm (J = 2) and 675–715 nm (J = 4), corresponding to the overall emission (I). The signals are primarily dominated by Faraday A-term.18 In contrast, the Eu2Ti7 in solution did not show the CPL signal in the absence of an external magnetic field, confirming that the external magnetic field induces the CPL signal of achiral Eu2Ti7. The MCPL spectra under the N-up and S-up magnetic fields are nearly mirror images of each other.
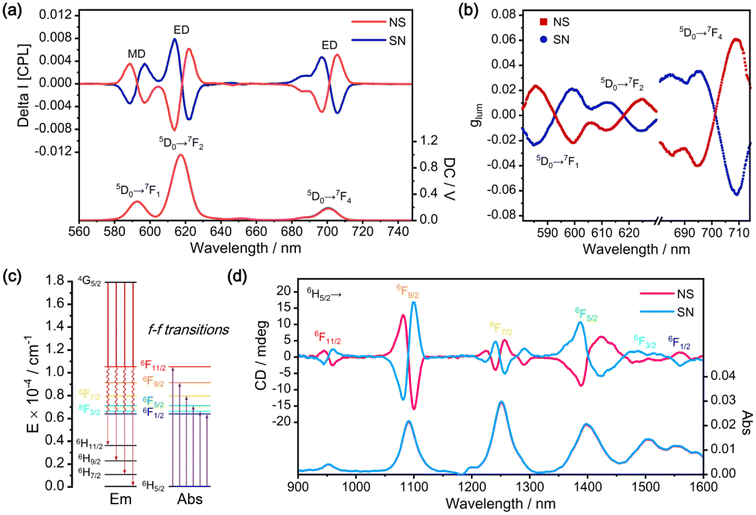 |
| Fig. 4 (a) The MCPL (upper) and PL (lower) spectra of Eu2Ti7 in solution state under the 1.6 T external magnetic field. (b) Correspondence between glum value and wavelength in MCPL of Eu2Ti7. (c) Energy level diagram of emission and absorption f–f transitions for Sm(III). (d) The near-infrared MCD (upper) and absorption (lower) spectra of Sm2Ti7 in solution state under the 1.6 T external magnetic field. | |
To quantitatively analyze the MCPL results for different f–f transitions of Eu2Ti7, the luminescence asymmetry factor glum (glum = ΔI/I) is used.19 In MCPL, the glum value should be normalized by the magnetic flux density (B) of the external magnetic field, giving gMCPL = glum/B (T−1). The glum values for Eu2Ti7 are presented in Fig. 4b, with the peak values summarized in Table S3.† For chiral Eu(III)-contained systems without an external magnetic field, the largest glum value typically occurs during the magnetic dipole transition 5D0 → 7F1. However, in Eu2Ti7, the magnetic field has the greatest influence on the electric dipole transition 5D0 → 7F4, followed by the magnetic dipole transition 5D0 → 7F1, and then the electric dipole transition 5D0 → 7F2. The maximum |gMCPL| for the 5D0 → 7F4 transition is observed at 709 nm with a value of 4.0 × 10–2 T−1. For the 5D0 → 7F1 transition, the maximum |gMCPL| is 1.5 × 10–2 T−1 at 586 nm. Lastly, for the 5D0 → 7F2 transition, the maximum |gMCPL| is 0.8 × 10–4 T−1 at 625 nm. In contrast, accurate MCPL analysis for Sm2Ti7 was not feasible due to its weak emission intensity and the interference of background noise. This highlights the necessity of strong luminescence intensity as a prerequisite for effective MCPL measurement.
Although the suboptimal luminescence of Sm2Ti7 hinders obtaining a clear MCPL signal, its strong absorption (arising from 6H5/2 → 6F1/2–11/2 transitions) in the near-infrared region is of highly significant for magnetic circular dichroism (MCD) studies. As shown in Fig. 4d, the CD signals (ΔA) for Sm2Ti7 were recorded at the external magnetic field strength of 1.6 T, corresponding to the overall absorption (A). When the magnetic field direction was reversed, the CD signals maintain the magnitude but exhibit a mirrored distribution. The MCD results were further analyzed by calculating the gMCD factor, defined as gMCD = gabs/B (where gabs = ΔA/A is the absorption asymmetry factor20). For Sm2Ti7, the maximum |gMCD| value is 2.1 × 10–2 T−1 at 1102 nm, corresponding to the electric dipole transition 6H5/2 → 6F9/2. Additionally, for the electric dipole transition 6H5/2 → 6F11/2, the magnetic dipole transition 6H5/2 → 6F5/2 and the magnetic dipole transition 6H5/2 → 6F3/2, the maximum |gMCD| values of these transitions are on the order of 10–2 T−1.
Conclusion
In conclusion, we report a series of isostructural LTOCs Ln2Ti7 (Ln = La, Sm, Eu), using 3,5-di-tert-butylbenzoic acid serving as the ligand. A detailed comparative analysis of the luminescence properties revealed that Eu2Ti7 exhibits significantly superior luminescence characteristics compared to Sm2Ti7, including stronger emission intensity, higher quantum yield, and longer lifetime. We also conducted a study of the magnetic circularly polarized luminescence (MCPL) of Eu2Ti7, demonstrating its responsiveness to external magnetic fields and showcasing its potential for magneto-optical applications. The energy-level analysis explaining the luminescence differences between Eu2Ti7 and Sm2Ti7 further motivated our research into the magnetic circular dichroism (MCD) properties of Sm2Ti7, particularly in the near-infrared region. This study not only contributes to the understanding of magneto-optical effects in lanthanide-based clusters but also emphasizes the importance of analyzing the energy levels of Ln(III) ions for advancing magneto-optical research.
Data availability
All relevant data are within the manuscript and ESI.† The data are available from the corresponding author on reasonable request.
Conflicts of interest
There are no conflicts to declare.
Acknowledgements
We are grateful for the financial support from the National Natural Science Foundation of China (Grant No. 92161104, 92161203, and 92361301).
References
- P. S. Peijzel, A. Meijerink, R. T. Wegh, M. F. Reid and G. W. Burdick, A complete 4fn energy level diagram for all trivalent lanthanide ions, J. Solid State Chem., 2005, 178, 448–453 CrossRef CAS.
-
(a) P. Chen, B. Peng, Z. Liu, J. Liu, D. Li, Z. Li, X. Xu, H. Wang, X. Zhou and T. Zhai, Room-Temperature Magnetic-Induced Circularly Polarized Photoluminescence in Two-Dimensional Er2O2S, J. Am. Chem. Soc., 2024, 146, 6053–6060 CrossRef CAS;
(b) C. Li, L. C. Adi, K. Paillot, I. Breslavetz, L. Long, L. Zheng, G. L. J. A. Rikken, C. Train, X. Kong and M. Atzori, Enhancement of Magneto-Chiral Dichroism Intensity by Chemical Design: The Key Role of Magnetic-Dipole Allowed Transitions, J. Am. Chem. Soc., 2024, 146, 16389–16393 CrossRef CAS;
(c) H. Wang, B. Yin, J. Bai, X. Wei, W. Huang, Q. Chang, H. Jia, R. Chen, Y. Zhai, Y. Wu and C. Zhang, Giant magneto-photoluminescence at ultralow field in organic microcrystal arrays for on-chip optical magnetometer, Nat. Commun., 2024, 15, 3995 CrossRef CAS PubMed;
(d) Y. Li, H. Wang, Z. Zhu, Y. Wang, F. Liang and H. Zou, Aggregation induced emission dynamic chiral europium(III) complexes with excellent circularly polarized luminescence and smart sensors, Nat. Commun., 2024, 15, 2896 CrossRef CAS PubMed.
- H. Yoshikawa, G. Nakajima, Y. Mimura, T. Kimoto, Y. Kondo, S. Suzuki, M. Fujiki and Y. Imai, Mirror-image magnetic circularly polarized luminescence (MCPL) from optically inactive EuIII and TbIIItris(β-diketonate), Dalton Trans., 2020, 49, 9588–9594 RSC.
-
(a) B. Han, X. Gao, J. Lv and Z. Tang, Magnetic Circular Dichroism in Nanomaterials: New Opportunity in Understanding and Modulation of Excitonic and Plasmonic Resonances, Adv. Mater., 2018, 32, 1801491 CrossRef;
(b) B. Han, X. Gao, J. Lv and Z. Tang, Magnetic Circular Dichroism in Nanomaterials: New Opportunity in Understanding and Modulation of Excitonic and Plasmonic Resonances, Adv. Mater., 2018, 32, 1801491 CrossRef PubMed.
-
(a) D. A. Gálico, C. M. S. Calado and M. Murugesu, Lanthanide molecular cluster-aggregates as the next generation of optical materials, Chem. Sci., 2023, 14, 5827–5841 RSC;
(b) Y. Li, H. Wang, Z. Zhu, F. Liang and H. Zou, Recent advances in the structural design and regulation of lanthanide clusters: Formation and self-assembly mechanisms, Coord. Chem. Rev., 2023, 493, 215322 CrossRef CAS;
(c) X. Zheng, J. Xie, X. Kong, L. Long and L. Zheng, Recent advances in the assembly of high-nuclearity lanthanide clusters, Coord. Chem. Rev., 2019, 378, 222–236 CrossRef CAS.
-
(a) L. Armelao, S. Quici, F. Barigelletti, G. Accorsi, G. Bottaro, M. Cavazzini and E. Tondello, Design of luminescent lanthanide complexes: From molecules to highly efficient photo-emitting materials., Coord. Chem. Rev., 2010, 254, 487–505 CrossRef CAS;
(b) A. de Bettencourt-Dias and J. S. K. Rossini, Ligand Design for Luminescent Lanthanide-Containing Metallopolymers, Inorg. Chem., 2016, 55, 9954–9963 CrossRef CAS;
(c) J.-C. G. Bünzli and C. Piguet, Taking advantage of luminescent lanthanide ions, Chem. Soc. Rev., 2005, 34, 1048–1077 RSC;
(d) Y. Hasegawa, Y. Kitagawa and T. Nakanishi, Effective photosensitized, electrosensitized, and mechanosensitized luminescence of lanthanide complexes, NPG Asia Mater., 2018, 10, 52–70 CrossRef CAS.
-
(a) C. Huang, R. Sun, L. Bao, X. Tian, C. Pan, M. Li, W. Shen, K. Guo, B. Wang, X. Lu and S. Gao, A hard molecular nanomagnet from confined paramagnetic 3d-4f spins inside a fullerene cage, Nat. Commun., 2023, 14, 8443 CrossRef CAS PubMed;
(b) Z. Lu, Z. Zhuo, W. Wang, Y. Huang and M. Hong, {Gd44Ni22}: a gigantic 3d–4f wheel-like nanoscale cluster with a large magnetocaloric effect, Inorg. Chem. Front., 2023, 10, 979–983 RSC;
(c) X. Wang, S. Wang, J. Chen, J. Jia, C. Wang, K. Paillot, I. Breslavetz, L. Long, L. Zheng, G. L. J. A. Rikken, C. Train, X. Kong and M. Atzori, Magnetic 3d–4f Chiral Clusters Showing Multimetal Site Magneto-Chiral Dichroism, J. Am. Chem. Soc., 2022, 144, 8837–8847 CrossRef CAS.
-
(a) K. Mishima, D. Kaji, M. Fujiki and Y. Imai, Remarkable Effects of External Magnetic Field on Circularly Polarized Luminescence of EuIII(hfa)3 with Phosphine Chirality, ChemPhysChem, 2021, 22, 1728–1737 CrossRef CAS PubMed;
(b) F. S. Richardson and H. G. Brittain, A structural study of tris(.beta.-diketonate)europium(III) complexes in solution using magnetic circularly polarized luminescence spectroscopy, J. Am. Chem. Soc., 1981, 103, 18–24 CrossRef CAS.
- D. A. Gálico and M. Murugesu, Magnetic Circularly Polarized Luminescence with Heterometallic Molecular Cluster–Aggregates, Adv. Opt. Mater., 2024, 12, 2401064 CrossRef.
-
(a) J. Chen, K. Huang, P. Cheng, M. Qi, H. Xu, J. Chen, Y. Duan, X. Kong, L. Zheng and L. Long, Strong NIR-II Magneto-Optical Activity of a Chiral Sm15Cu54 Cage, J. Am. Chem. Soc., 2024, 146, 22913–22917 CrossRef CAS;
(b) Y. Kitagawa, S. Wada, K. Yanagisawa, T. Nakanishi, K. Fushimi and Y. Hasegawa, Molecular Design Guidelines for Large Magnetic Circular Dichroism Intensities in Lanthanide Complexes, ChemPhysChem, 2016, 17, 845–849 CrossRef CAS PubMed.
-
(a) C. Artner, S. Kronister, M. Czakler and U. Schubert, Ion-Size-Dependent Formation of Mixed Titanium/Lanthanide Oxo Clusters, Eur. J. Inorg. Chem., 2014, 2014, 5596–5602 CrossRef CAS;
(b) W. Fang, L. Zhang and J. Zhang, Synthetic strategies, diverse structures and tuneable properties of polyoxo-titanium clusters, Chem. Soc. Rev., 2018, 47, 404–421 RSC;
(c) Y. Liu, W. Fang, L. Zhang and J. Zhang, Recent advances in heterometallic polyoxotitanium clusters, Coord. Chem. Rev., 2020, 404, 213099 CrossRef CAS;
(d) S. Wang, H. Su, L. Yu, X. Zhao, L. Qian, Q. Zhu and J. Dai, Fluorescence and energy transfer properties of heterometallic lanthanide-titanium oxo clusters coordinated with anthracenecarboxylate ligands, Dalton Trans., 2015, 44, 1882–1888 RSC;
(e) G. Zhang, S. Wang, J. Hou, C. Mo, C. Que, Q. Zhu and J. Dai, A lanthanide-titanium (LnTi11) oxo-cluster, a potential molecule based fluorescent labelling agent and photocatalyst, Dalton Trans., 2016, 45, 17681–17686 RSC.
-
(a) R. Chen, Z. Hong, Y. Zhao, H. Zheng, G. Li, Q. Zhang, X. Kong, L. Long and L. Zheng, Ligand-Dependent Luminescence Properties of Lanthanide-Titanium Oxo Clusters, Inorg. Chem., 2019, 58, 15008–15012 CrossRef CAS;
(b) G. Li, L. Long, X. Kong and L. Zheng, Recent Advances in Lanthanide-titanium-oxo Clusters, Chem. J. Chin. Univ., 2020, 40, 2577–2586 CrossRef;
(c) W. Liu, G. Li, H. Xu, Y. Deng, M. Du, L. Long, L. Zheng and X. Kong, Circularly polarized luminescence and performance modulation of chiral europium-titanium (Eu2Ti4)-oxo clusters, Chem. Commun., 2023, 59, 346–349 RSC;
(d) D. Lu, Z. Hong, J. Xie, X. Kong, L. Long and L. Zheng, High-Nuclearity Lanthanide-Titanium Oxo Clusters as Luminescent Molecular Thermometers with High Quantum Yields, Inorg. Chem., 2017, 56, 12186–12192 CrossRef CAS PubMed;
(e) X. Shu, W. Luo, H. Wang, M. Fu, Q. Zhu and J. Dai, Eu-phen Bonded Titanium Oxo-Clusters, Precursors for a Facile Preparation of High Luminescent Materials and Films, Inorg. Chem., 2020, 59, 10422–10429 CrossRef CAS PubMed;
(f) Y. Zhao, H. Zheng, L. Chen, H. Chen, X. Kong, L. Long and L. Zheng, The Effect on the Luminescent Properties in Lanthanide-Titanium OXO Clusters, Inorg. Chem., 2019, 58, 10078–10083 CrossRef CAS PubMed.
-
(a) C. Liu and Y. Wang, Supramolecular Chemistry of Titanium Oxide Clusters, Chem. – Eur. J., 2021, 27, 4270–4282 CrossRef CAS PubMed;
(b) L. Zhang, X. Fan, X. Yi, X. Lin and J. Zhang, Coordination-Delayed-Hydrolysis Method for the Synthesis and Structural Modulation of Titanium-Oxo Clusters, Acc. Chem. Res., 2022, 55, 3150–3161 CrossRef CAS PubMed.
- C. Wang, J. Zhang and X. Zhu, Synthesis of lanthanide-doped titanium-oxo clusters for efficient photocurrent responses, J. Solid State Chem., 2021, 304, 122586 CrossRef CAS.
-
(a) S. S. Mortensen, M. A. M. Nielsen, P. Nawrocki and T. J. Sørensen, Electronic Energy Levels and Optical Transitions in Samarium(III) Solvates, J. Phys. Chem. A, 2022, 126, 8596–8605 CrossRef CAS PubMed;
(b) M. Tang, Z. Zhu, Y. Li, W. Qin, F. Liang, H. Wang and H. Zou, Specific smart sensing of electron-rich antibiotics or histidine improves the antenna effect, luminescence, and photodynamic sterilization capabilities of lanthanide polyoxometalates, J. Colloid Interface Sci., 2025, 680, 235–246 CrossRef CAS.
- K. Binnemans, Interpretation of europium(III) spectra, Coord. Chem. Rev., 2015, 295, 1–45 CrossRef CAS.
-
(a) E. G. Moore, A. P. S. Samuel and K. N. Raymond, From Antenna to Assay: Lessons Learned in Lanthanide Luminescence, Acc. Chem. Res., 2009, 42, 542–552 CrossRef CAS PubMed;
(b) J. Xu and S. Tanabe, Persistent luminescence instead of phosphorescence: History, mechanism, and perspective, J. Lumin., 2019, 205, 581–620 CrossRef CAS;
(c)
A. d. Bettencourt-Dias, Luminescence of Lanthanide Ions in Coordination Compounds and Nanomaterials, WILEY, 2014, ISBN 978-1119950837 CrossRef;
(d) B. Yu, Z. Zhu, W. Qin, H. Wang, Y. Li, F. Liang and H. Zou, Enhancement of Luminescence, Multiple-Sensing, and Differentiated Live-Cell-Imaging Properties of High-Nuclear Lanthanide Nanoclusters via the Zn(II)-Chelate-Controlled Dual Antenna Effect, ACS Mater. Lett., 2024, 6, 3312–3326 CrossRef CAS.
- F. Zinna and G. Pescitelli, Magnetic Circularly Polarized Luminescence of Organic Compounds, Eur. J. Org. Chem., 2023, e202300509 CrossRef CAS.
- Z. Gong, Z. Li and Y. Zhong, Circularly polarized luminescence of coordination aggregates, Aggregate, 2022, 3, e177 CrossRef CAS.
- C. Zhang, S. Li, X. Dong and S. Zang, Circularly polarized luminescence of agglomerate emitters, Aggregate, 2021, 2, e48 CrossRef CAS.
|
This journal is © the Partner Organisations 2025 |
Click here to see how this site uses Cookies. View our privacy policy here.