DOI:
10.1039/C8SC03226B
(Edge Article)
Chem. Sci., 2018,
9, 8207-8212
Ratiometric fluorescent probes for capturing endogenous hypochlorous acid in the lungs of mice†
Received
20th July 2018
, Accepted 4th September 2018
First published on 5th September 2018
Abstract
Hypochlorous acid (HClO) is a promising diagnostic marker for inflammation and relevant diseases. Although many probes were previously developed for HClO imaging, the development of organ targeting probes is still lacking. Herein, we designed and synthesized a series of cyanine derivatives as ratiometric fluorescent probes to detect endogenous HClO in the lungs with inflammation. By installing diverse lipid chains and amino groups on cyanine, we identified that ClO1, with one n-octadecane chain and two 2-[[2-(dimethylamino)ethyl]methylamino]-ethyl groups, is a superior probe to target the lungs over other major organs in mice. ClO1 was able to sense both exogenous and endogenous HClO in A549 (human lung epithelial) cells through fluorescence ratiometric imaging. In a lipopolysaccharide (LPS)-induced lung inflammation mouse model, ClO1 effectively captured endogenous HClO in the lungs after intravenous administration. Overall, these cyanine-derived probes merit further development as organ targeting HClO sensors.
Introduction
Reactive oxygen species (ROS) and reactive nitrogen species (RNS) play critical roles in biological pathways and stress responses.1–10 Hypochlorous acid (HClO), as one of the ROS molecules, is involved in multiple processes of the immune system, such as counteracting pathogens, suppressing inflammation and regulating cellular apoptosis.11–14 Evidence has shown that an increased level of HClO is often found to be associated with human disorders, including inflammatory diseases.15 Due to the important functions of HClO, previous studies have investigated a wide variety of fluorescent probes for detecting HClO. For example, probes established based on naphthalimides, acedan, cyanine dyes and other types of fluorophores were used to image HClO in living cells (Table S1†).5,6,15–34 Many of these probes exhibited favorable features such as two-photon excitability or organelle targetability.6,15,16,18,21,22,25,27,29–31,35 Among these previously reported HClO probes, a number of them were fabricated with cyanine dyes due to their high reactivity towards HClO.17,23,36–38 A mechanism of reaction was previously proposed as shown in Scheme 1A.23 In the presence of HClO, heptamethine cyanine dye (Cy7) undergoes an addition reaction to produce an intermediate. This intermediate then loses a HCl molecule and affords a Cy7 oxirane derivative, which exhibits shorter absorption and emission wavelengths compared to the original Cy7 molecule (Scheme 1A). This structural change enables Cy7 derivatives to be suitable ratiometric probes for HClO. The probes mentioned above are mainly applied in cell studies; however, it is urgent to develop probes for sensing HClO at the organ level for the potential diagnosis of diverse diseases. Previously, phenothiazine was modified with functional groups and a probe termed PMN-TPP was produced. This probe enabled the ratiometric fluorescence imaging of HClO in mice with skin inflammation.6 Most recently, a probe derived from 2-methyl-1H-benzo[de]isoquinoline-1,3(2H)-dione was conjugated onto a diblock copolymer forming polymer micelles, which were used to image liver injury in zebrafish.5 Despite these significant advances of diverse probes in the in vivo imaging of HClO, it is still of great demand to develop new types of organ targeting probes.
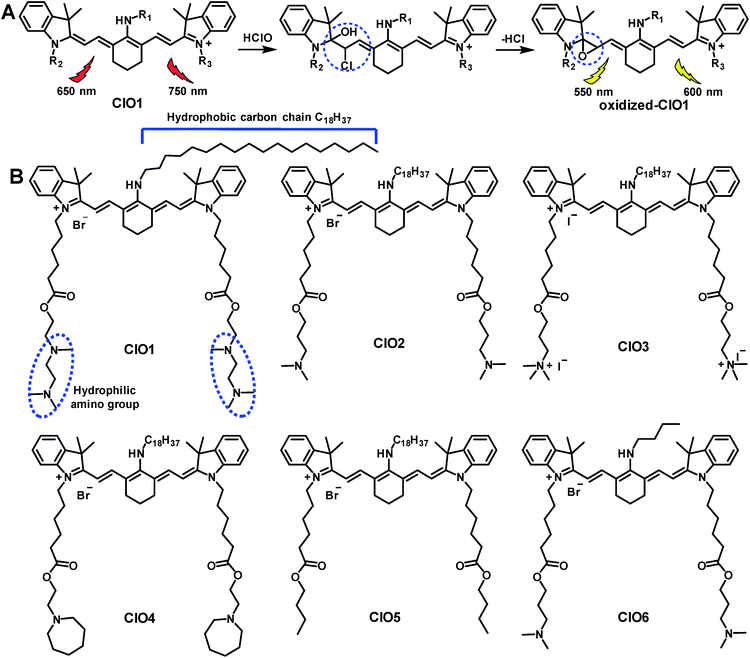 |
| Scheme 1 (A) Mechanism of heptamethine Cy7 for the ratiometric fluorescence imaging of HClO.23 (B) Structures of probes ClO1–ClO6. | |
Results and discussion
In this study, we aim to design lung-targeting probes and detect HClO molecules in the event of inflammation. To date, numerous studies have explored lipid and polymer based nanoparticles for the delivery of therapeutic cargo to the lungs.39–42 For example, researchers employed cationic or ionizable lipids to deliver DNA or siRNA to the lungs of mice.40,42 Based on these studies, we speculate that lipid chains and amino groups may contribute to the accumulation of delivery materials along with their cargo in the lungs. Therefore, we conceived a series of heptamethine Cy7 based cyanine derivatives, ClO1–ClO6, as fluorescent ratiometric HClO probes (Scheme 1B). According to the methods reported previously,23ClO1–ClO6 were synthesized through two-step reactions. The synthetic routes to ClO1–ClO6 are described in the ESI.† The structures of ClO1–ClO6 were confirmed by 1H NMR and high-resolution mass spectrometry (ESI†). To confirm the mechanism shown in Scheme 1, we treated ClO1 with HClO and measured the resulting product through mass spectra. A new peak of m/z +16 was found (ESI, Fig. S1†), which corresponds to the epoxides produced by the oxidation of the double bonds in ClO1 (Scheme 1A). This result is in accordance with the reported mechanism for the response of cyanine dyes towards HClO.23 In order to study the effects of different functional groups, we installed long (n-octadecyl alkane, C18H37) and short (n-butyl, C4H9) lipid chains. Meanwhile, we incorporated diverse amino groups including 2[[2-(dimethylamino)ethyl]methylamino]-ethyl groups (ClO1), 3-(dimethylamino)-propyl groups (ClO2 and ClO6), propyl-trimethylammonium groups (ClO3), and hexahydro-1H-azepine-1-ethanyl (ClO4). ClO5 was synthesized as a control compound without amino groups.
In order to study the sensing ability of ClO1–ClO6 towards HClO, we first performed a spectral study in solution with serial concentrations of HClO. Briefly, we investigated the absorption and emission spectra of ClO1–ClO6 in phosphate buffered saline (PBS) by using a NaClO solution as the HClO source. Upon titration with NaClO, ClO1 displayed a significant decrease in the absorption peak at 638 nm and a gradual increase in the absorption peak at 540 nm (Fig. S2†). Meanwhile, we observed an evident decrease in the peak at 760 nm and an increase in the peak at 605 nm in the emission spectrum of ClO1 with increasing concentration of NaClO (Fig. 1A, excited at 530 nm). Consequently, ClO1 exhibited a dramatic fluorescence ratio (I605/I760) enhancement from 0.1 to 6.9 in a NaClO concentration range of 0–14 μM (Fig. 1B). The detection limit of ClO1 was calculated to be 0.1 μM (Fig. S3†). In order to investigate the effects of different reactive oxygen species (ROS) and reactive nitrogen species (RNS) on ClO1, we performed a selectivity study. As shown in Fig. S4 and S5,†ClO1 demonstrated a minimal absorption change at 638 nm and a minimal fluorescence change at 760 nm upon the addition of a wide variety of ROS/RNS, metal ions and amino acids, while an apparent decrease was seen after the addition of HClO. Therefore, the reactivity of ClO1 towards HClO is much higher than that towards other ROS and RNS. Also, ClO1 displayed a significant decrease in absorption at 638 nm and a decrease in fluorescence at 760 nm upon the addition of NaClO in the presence of Fe2+ and H2O2, but showed low reactivity with reduced glutathione (GSH) (Fig. S4 and S5†). Yet, local concentrations of these analytes may be different in both cells and tissues. In addition, we found that ClO2, ClO4 and ClO6 showed similar patterns of changes towards NaClO in both the absorption and emission spectra (Fig. S6, S8 and S10†). However, ClO3 and ClO5 exhibited strong fluorescence peaks at both 560 nm and 750 nm without the presence of NaClO, which makes them unsuitable for ratiometric fluorescence imaging (Fig. S7 and S9†). Therefore, ClO1, ClO2, ClO4 and ClO6 were selected for cell imaging studies.
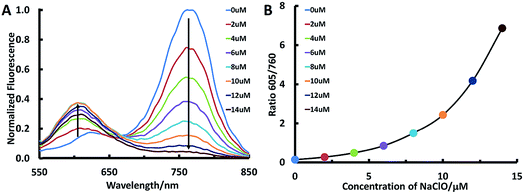 |
| Fig. 1 (A) Emission spectra of ClO1 (5 μM) upon treatment with various concentrations of NaClO (0–14 μM); (B) fluorescence ratio (I605/I760) of ClO1 (5 μM) upon treatment with various concentrations of NaClO (0–14 μM). | |
Next, we applied ClO1, ClO2, ClO4 and ClO6 to detect exogenous HClO (NaClO solution) in live A549 cells (a human lung adenocarcinoma cell line). We found that these probes were able to stain A549 cells (Fig. 2 and S11–S13†). Consistent with the spectral study in solution, ClO1 showed weak fluorescence in the green channel (Fig. 2A, pseudo color green) and intense fluorescence in the red channel (Fig. 2B, pseudo color red) from the normal cell imaging. After treatment with NaClO, the cells displayed a remarkable enhancement in the fluorescence of the green channel (Fig. 2D) and a slight decrease in the fluorescence of the red channel (Fig. 2E). The ratio of the intensity in the two channels (green/red channel) increased dramatically from the baseline (Fig. 2C) to up to 5.0 (Fig. 2F), which indicates that ClO1 possesses a robust ratiometric imaging property. ClO6 displayed similar responses towards HClO in A549 cells to ClO1 (Fig. S13†). ClO2 and ClO4 exhibited a much lower fluorescence enhancement in the green channel than ClO1 and ClO6 (Fig. S11 and S12†). Due to the high ratio enhancement in both the spectral study (0 to 6.9) and cell imaging (0 to 5.0), we selected ClO1 for further in vitro and in vivo experiments.
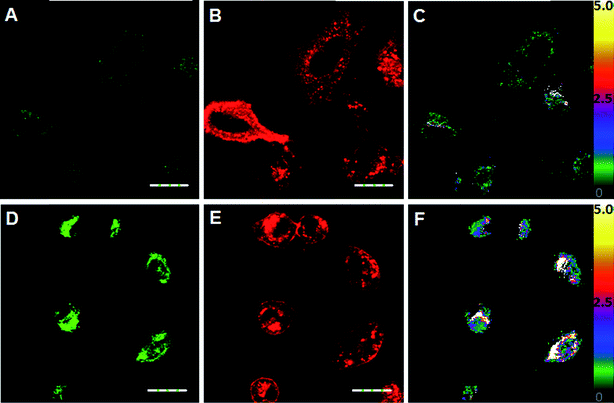 |
| Fig. 2 Detection of exogenous HClO in A549 cells by ClO1. Cells were imaged with ClO1 in the absence (A–C) or presence (D–F) of NaClO. (A) and (D) are green channels (560–660 nm); (B) and (E) are red channels (655–755 nm); (C) and (F) are the ratio images of the green/red channel. Scale bar = 20 μm. | |
In order to evaluate the ability of ClO1 to detect in situ HClO production, we used lipopolysaccharides (LPS, endotoxins that cause the release of HClO in cells and animals43) to induce endogenous HClO production in A549 cells. Briefly, cells were further incubated with or without LPS for 24 h after staining with ClO1. We observed an apparent fluorescence increase in the green channel of cells treated with LPS (Fig. 3D) as compared to the control group without the treatment with LPS (Fig. 3A). An enhancement of the fluorescence ratio (green/red channel) was detected from 0 (Fig. 3C) to 2.5 (Fig. 3F) upon treatment with LPS. These results indicated that ClO1 was able to capture HClO molecules generated from LPS treated cells. Furthermore, in order to study the cytotoxicity of ClO1, an MTT assay was performed using a series of concentrations of ClO1. As shown in Fig. S14,† the survival rate was higher than 90%, suggesting low cytotoxicity of ClO1 at the tested concentrations (0.5–10 μM).
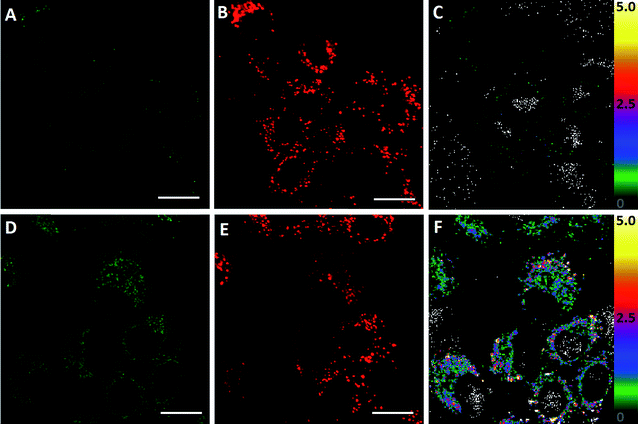 |
| Fig. 3 Detection of endogenous HClO in A549 cells by ClO1. Cells were imaged with ClO1 in the absence (A–C) or presence (D–F) of LPS. (A) and (D) are green channels (560–660 nm); (B) and (E) are red channels (655–755 nm); (C) and (F) are the ratio images of the green/red channel. Scale bar = 20 μm. | |
Following the in vitro studies, we performed a bio-distribution study of ClO1–ClO6 in C57BL/6 mice. Indocyanine green (ICG, an FDA approved cyanine for fluorescence imaging in the clinic44) was used as a control. ClO1–ClO6 and ICG were injected intravenously into mice. 1 h post injection, major organs (liver, lungs, spleen, kidneys, and heart) were harvested and imaged by using the IVIS fluorescence imaging system. ClO1–ClO3 exhibited higher accumulation in the lungs than in other organs. ClO4 and ClO5 displayed higher distribution in both the spleen and lungs. ClO6 and ICG showed a higher signal in the liver (Fig. 4). Based on the structural features of ClO1–ClO6 and their corresponding organ distribution, we found that a long lipid chain was favorable for lung accumulation. Also, amino groups were a critical factor for lung targeting (ClO1vs.ClO4), and might be protonated in cells and produce positive charges. These results are consistent with previous reports that positive charges on nanoparticles affect their lung-targeting ability.40,42
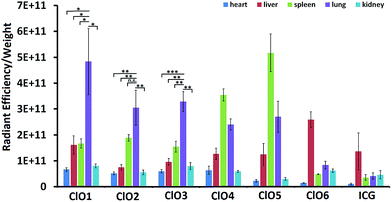 |
| Fig. 4 The ratio of radiant efficiency to the organ weight of mice intravenously injected with ClO1–ClO6 and ICG. (n = 3; *P < 0.05; **P < 0.01; ***P < 0.001; n.s., P > 0.05; t test, double-tailed). | |
Lastly, we studied whether ClO1 was able to detect endogenous HClO at LPS-induced lung inflammation sites in mice. We first treated mice with LPS (intranasal administration) to induce acute lung inflammation. Then, we intravenously injected ClO1 into both untreated and LPS-treated mice. 1 h after the injection, the lungs of the mice were dissected and sectioned into ∼100 μm slices and imaged under a confocal microscope. Strong fluorescence was observed in the red channel of both slices, which confirmed the accumulation of ClO1 in the lungs (Fig. 5B and F). Consistent with the in vitro results, many spots in the lungs treated with LPS produced a strong signal in the green channel (Fig. 5E), while minimal fluorescence was found in the green channel of the untreated lungs (Fig. 5A). The fluorescence ratio (green/red channel) at the inflammation sites was around 5.0 (Fig. 5H), which is much higher than that in the control lungs without inflammation (Fig. 5D).
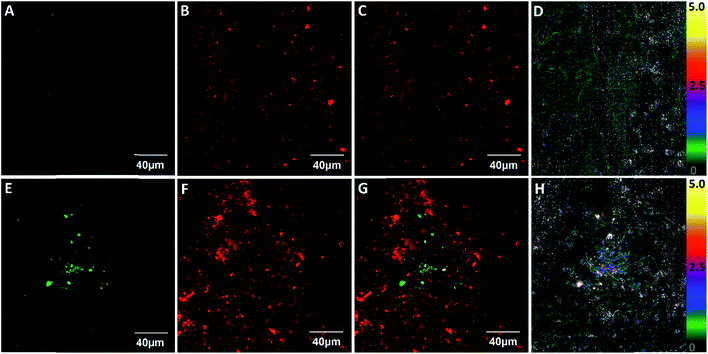 |
| Fig. 5 Fluorescence imaging of the lung histology slides from mice intravenously injected with ClO1. (A–D) control mouse with intravenous injection of ClO1; (E–H) mouse treated with intranasal administration of LPS and intravenous injection of ClO1. (A) and (E) are green channels (560–660 nm); (B) and (F) are red channels (655–755 nm); (C) and (G) are overlay images of green and red channels; (D) and (H) are ratio images of green/red channels. | |
Conclusions
In summary, we designed and synthesized a series of Cy7 based fluorescence ratiometric probes in order to detect HClO in the lungs. ClO1–ClO3 with long hydrophobic lipid chains and hydrophilic amino groups showed high accumulation in mouse lungs after intravenous injections. However, ClO4 with hexahydro-1H-azepine-1-ethanyl amino groups and ClO5 with n-butyl (without amino groups) showed a higher uptake in the spleen than in other organs tested. ClO6 with short lipid chains displayed high distribution in the liver similar to ICG. These results indicated that both lipid chains and amino groups are important moieties for enabling the lung targeting effect of the probes. Moreover, an appropriate combination may dramatically increase the targeting specificity. Among these probes, ClO1, containing one n-octadecane chain and two 2-[[2-(dimethylamino)ethyl]methylamino]-ethyl groups, exhibited a sensitive fluorescence ratio response towards both exogenous and endogenous HClO in live cells. Based on the in vivo results, we demonstrated that ClO1 was able to capture LPS-induced HClO in the mouse lungs. These findings can be applied to design new probes for non-invasive and real-time monitoring of HClO in vivo in the future.
Conflicts of interest
There are no conflicts to declare.
Acknowledgements
Y. D. acknowledges the support from the Maximizing Investigators' Research Award 1R35GM119679 from the National Institute of General Medical Sciences as well as the start-up fund from the College of Pharmacy at The Ohio State University. All animal procedures were performed in accordance with the Guidelines for Care and Use of Laboratory Animals of The Ohio State University and experiments were approved by the Animal Ethics Committee of Institutional Animal Care and Use Committee (IACUC).
References
- B. D'Autreaux and M. B. Toledano, Nat. Rev. Mol. Cell Biol., 2007, 8, 813–824 CrossRef PubMed.
- T. Finkel and N. J. Holbrook, Nature, 2000, 408, 239–247 CrossRef PubMed.
- M. Valko, D. Leibfritz, J. Moncol, M. T. Cronin, M. Mazur and J. Telser, Int. J. Biochem. Cell Biol., 2007, 39, 44–84 CrossRef PubMed.
- C. C. Winterbourn, Nat. Chem. Biol., 2008, 4, 278–286 CrossRef PubMed.
- P. Zhang, H. Wang, Y. Hong, M. Yu, R. Zeng, Y. Long and J. Chen, Biosens. Bioelectron., 2018, 99, 318–324 CrossRef PubMed.
- H. Xiao, J. Li, J. Zhao, G. Yin, Y. Quan, J. Wang and R. Wang, J. Mater. Chem. B, 2015, 3, 1633–1638 RSC.
- P. Zhang, Y. Tian, H. Liu, J. Ren, H. Wang, R. Zeng, Y. Long and J. Chen, Chem. Commun., 2018, 54, 7231–7234 RSC.
- P. Zhang, X. F. Jiang, X. Nie, Y. Huang, F. Zeng, X. Xia and S. Wu, Biomaterials, 2016, 80, 46–56 CrossRef PubMed.
- P. Zhang, X. Nie, M. Gao, F. Zeng, A. Qin, S. Wu and B. Z. Tang, Mater. Chem. Front., 2017, 1, 838–845 RSC.
- Y. Huang, P. Zhang, M. Gao, F. Zeng, A. Qin, S. Wu and B. Z. Tang, Chem. Commun., 2016, 52, 7288–7291 RSC.
- C.-H. Sam and H.-K. Lu, Journal of Dental Sciences, 2009, 4, 45–54 CrossRef.
- C. C. Winterbourn, M. B. Hampton, J. H. Livesey and A. J. Kettle, J. Biol. Chem., 2006, 281, 39860–39869 CrossRef PubMed.
- B. Kwaśny-Krochin, M. Bobek, E. Kontny, P. Gluszko, R. Biedroń, B. M. Chain, W. Maśliński and J. Marcinkiewicz, Amino Acids, 2002, 23, 419–426 CrossRef PubMed.
- M. Casciaro, E. Di Salvo, E. Pace, E. Ventura-Spagnolo, M. Navarra and S. Gangemi, Immun. Ageing, 2017, 14, 21 CrossRef PubMed.
- Y. W. Jun, S. Sarkar, S. Singha, Y. J. Reo, H. R. Kim, J.-J. Kim, Y.-T. Chang and K. H. Ahn, Chem. Commun., 2017, 53, 10800–10803 RSC.
- L. Yuan, L. Wang, B. K. Agrawalla, S.-J. Park, H. Zhu, B. Sivaraman, J. Peng, Q.-H. Xu and Y.-T. Chang, J. Am. Chem. Soc., 2015, 137, 5930–5938 CrossRef PubMed.
- H. Li, L. Guan, X. Zhang, H. Yu, D. Huang, M. Sun and S. Wang, Talanta, 2016, 161, 592–598 CrossRef PubMed.
- S.-k. Yao and Y. Qian, Sens. Actuators, B, 2017, 252, 877–885 CrossRef.
- L.-L. Xi, X.-F. Guo, C.-L. Wang, W.-L. Wu, M.-F. Huang, J.-Y. Miao and B.-X. Zhao, Sens. Actuators, B, 2018, 255, 666–671 CrossRef.
- Y.
L. Pak, S. J. Park, D. Wu, B. Cheon, H. M. Kim, J. Bouffard and J. Yoon, Angew. Chem., Int. Ed., 2018, 57, 1567–1571 CrossRef PubMed.
- P. Zhang, H. Wang, D. Zhang, X. Zeng, R. Zeng, L. Xiao, H. Tao, Y. Long, P. Yi and J. Chen, Sens. Actuators, B, 2018, 255, 2223–2231 CrossRef.
- B. Zhang, X. Yang, R. Zhang, Y. Liu, X. Ren, M. Xian, Y. Ye and Y. Zhao, Anal. Chem., 2017, 89, 10384–10390 CrossRef PubMed.
- Z. Lou, P. Li, P. Song and K. Han, Analyst, 2013, 138, 6291–6295 RSC.
- L. Wu, I. C. Wu, C. C. DuFort, M. A. Carlson, X. Wu, L. Chen, C.-T. Kuo, Y. Qin, J. Yu, S. R. Hingorani and D. T. Chiu, J. Am. Chem. Soc., 2017, 139, 6911–6918 CrossRef PubMed.
- M. Ren, B. Deng, K. Zhou, X. Kong, J.-Y. Wang, G. Xu and W. Lin, J. Mater. Chem. B, 2016, 4, 4739–4745 RSC.
- H. Zhu, J. Fan, J. Wang, H. Mu and X. Peng, J. Am. Chem. Soc., 2014, 136, 12820–12823 CrossRef PubMed.
- L. Cao, R. Zhang, W. Zhang, Z. Du, C. Liu, Z. Ye, B. Song and J. Yuan, Biomaterials, 2015, 68, 21–31 CrossRef PubMed.
- J. Fan, H. Mu, H. Zhu, J. Wang and X. Peng, Analyst, 2015, 140, 4594–4598 RSC.
- Z. Qu, J. Ding, M. Zhao and P. Li, J. Photochem. Photobiol., A, 2015, 299, 1–8 CrossRef.
- C. Liu, X. Jiao, S. He, L. Zhao and X. Zeng, Talanta, 2017, 174, 234–242 CrossRef PubMed.
- K. Li, J.-T. Hou, J. Yang and X.-Q. Yu, Chem. Commun., 2017, 53, 5539–5541 RSC.
- B. Zhu, L. Wu, M. Zhang, Y. Wang, C. Liu, Z. Wang, Q. Duan and P. Jia, Biosens. Bioelectron., 2018, 107, 218–223 CrossRef PubMed.
- B. Zhu, L. Wu, M. Zhang, Y. Wang, Z. Zhao, Z. Wang, Q. Duan, P. Jia and C. Liu, Sens. Actuators, B, 2018, 263, 103–108 CrossRef.
- Y. Wang, L. Wu, C. Liu, B. Guo, B. Zhu, Z. Wang, Q. Duan, Z. Ma and X. Zhang, J. Mater. Chem. B, 2017, 5, 3377–3382 RSC.
- Z. Mao, M. Ye, W. Hu, X. Ye, Y. Wang, H. Zhang, C. Li and Z. Liu, Chem. Sci., 2018, 9, 6035–6040 RSC.
- J. Peng, A. Samanta, X. Zeng, S. Han, L. Wang, D. Su, D. T. B. Loong, N.-Y. Kang, S.-J. Park, A. H. All, W. Jiang, L. Yuan, X. Liu and Y.-T. Chang, Angew. Chem., Int. Ed., 2017, 56, 4165–4169 CrossRef PubMed.
- X. Jia, Q. Chen, Y. Yang, Y. Tang, R. Wang, Y. Xu, W. Zhu and X. Qian, J. Am. Chem. Soc., 2016, 138, 10778–10781 CrossRef PubMed.
- D. Oushiki, H. Kojima, T. Terai, M. Arita, K. Hanaoka, Y. Urano and T. Nagano, J. Am. Chem. Soc., 2010, 132, 2795–2801 CrossRef PubMed.
- E. R. Lee, J. Marshall, C. S. Siegel, C. Jiang, N. S. Yew, M. R. Nichols, J. B. Nietupski, R. J. Ziegler, M. B. Lane, K. X. Wang, N. C. Wan, R. K. Scheule, D. J. Harris, A. E. Smith and S. H. Cheng, Hum. Gene Ther., 1996, 7, 1701–1717 CrossRef PubMed.
- R. Stribling, E. Brunette, D. Liggitt, K. Gaensler and R. Debs, Proc. Natl. Acad. Sci. U. S. A., 1992, 89, 11277–11281 CrossRef.
- S. Azarmi, W. H. Roa and R. Löbenberg, Adv. Drug Delivery Rev., 2008, 60, 863–875 CrossRef PubMed.
- O. F. Khan, E. W. Zaia, S. Jhunjhunwala, W. Xue, W. Cai, D. S. Yun, C. M. Barnes, J. E. Dahlman, Y. Dong, J. M. Pelet, M. J. Webber, J. K. Tsosie, T. E. Jacks, R. Langer and D. G. Anderson, Nano Lett., 2015, 15, 3008–3016 CrossRef PubMed.
- C.-Y. Chuang, T.-L. Chen, Y.-G. Cherng, Y.-T. Tai, T.-G. Chen and R.-M. Chen, Arch. Toxicol., 2011, 85, 209–218 CrossRef PubMed.
- G. Liberale, P. Bourgeois, D. Larsimont, M. Moreau, V. Donckier and T. Ishizawa, Eur. J. Surg. Oncol., 2017, 43, 1656–1667 CrossRef PubMed.
Footnotes |
† Electronic supplementary information (ESI) available: Materials and methods, details of synthesis, and 1H NMR spectra of the probes ClO1–ClO6 (PDF). See DOI: 10.1039/c8sc03226b |
‡ X. Z. and W. Z. contributed equally to this work. |
|
This journal is © The Royal Society of Chemistry 2018 |
Click here to see how this site uses Cookies. View our privacy policy here.