DOI:
10.1039/D3CE00732D
(Paper)
CrystEngComm, 2023,
25, 5887-5892
Crystallisation of organic hydrates by sublimation†
Received
23rd July 2023
, Accepted 15th September 2023
First published on 29th September 2023
Abstract
A series of five organic molecules with known crystalline hydrates were sublimed under vacuum in the presence and absence of water. In two systems, crystals of the hydrate could easily be grown from sublimation. Generally, a mixture of hydrated and anhydrous forms were obtained from sublimation. In several cases, an increase in the quantity of water added to the sublimation system resulted in an increase in water content of the sublimed crystals. Competition studies demonstrated that transfer of water can take place between hydrate and anhydrous forms during a sublimation experiment.
Introduction
Organic multicomponent crystals, such as salts, co-crystals and solvates,1–3 are of interest in many industries, since they often offer improved physiochemical properties in comparison to the parent molecule.4 Hydrates, a subclass of solvates, are of particular interest in, for example, the pharmaceutical industry, because hydrate formation can affect the bioavailability, performance, stability, or solubility of a material.5 Hydrates are often the less desirable form of a drug compound for therapeutic applications, since hydrates are often more thermodynamically stable than their anhydrous form. This makes them less likely to dissolve in water, resulting in lower bioavailability.5 Despite this, hydrates account for a third of active drug forms in the pharmaceutical industry.6
The formation of multicomponent crystals, and specifically hydrates, has been well-explored by common co-crystallisation techniques such as solution crystallisation and mechanochemistry.7,8 Sublimation has not been as well-studied, although the use of sublimation to crystallise polymorphs, co-crystals, and salts from the gas phase has recently been reviewed.9 Our own group has shown that organic salts and co-crystals can selectively be prepared from the gas phase using sublimation.8,10,11 However, prior to the current study, no attempt had been made to investigate the formation of hydrates from the gas phase via sublimation. Therefore, this study aimed to broaden the scope of sublimation as a crystal growth technique by answering the following question: can crystals of the hydrates of organic molecules be grown from the gas phase?
In order to answer this question, a systematic study was carried out on a series of known crystalline hydrates. Molecules were sublimed both in the presence and absence of water, and the effect of the quantity of water added to the sublimation vessel was also investigated. The study focused on five organic molecules (Scheme 1): oxalic acid (OA, 1), isonicotinamide (INAM, 2), theophylline (THE, 3), caffeine (CAF, 4), and 1,4-diazabicyclo[2.2.2]octane (DABCO, 5). Compounds were selected on the basis that they are known to sublime and have at least one crystalline hydrate.
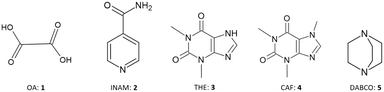 |
| Scheme 1 Molecules used in this study. | |
Results and discussion
Preparation and characterisation of materials
All five compounds selected for this study have multiple crystal forms, including hydrates, anhydrous forms, and polymorphs of each of these. A detailed Cambridge Structural Database (CSD)12 search was carried out to identify known solid-state forms of the selected compounds, including polymorphs. The relevant CSD refcodes are listed in the ESI† (Tables S1–S5). Anhydrous forms are labelled ‘A’, and hydrates labelled ‘H’. Different polymorphs are denoted by suffixes a–f. For example, 1A-a is one anhydrous polymorph of 1. PXRD, TGA and SCXRD were used to verify the crystalline form of each compound as purchased, confirming that compound 1 is in the dihydrate form, 1H-a,132 is 2A-a,14 compound 3 is anhydrous 3A-a,154 is anhydrous 4A-b,16 and 5 is anhydrous 5A-a17 (Fig. S1–S6†). The hydrates of INAM (2B), THE (3B) and CAF (4B) were synthesised mechanochemically, and synthesis of the hydrate was confirmed by PXRD and TGA (Fig. S10–S15†). ‘B’ here indicates the prepared hydrate. Anhydrous OA (1A) was prepared by heating 1 at 80 °C under 4.57 mbar vacuum for a period of five days.
All compounds except DABCO have a hydrate of only one stoichiometry, i.e., OA dihydrate, INAM monohydrate, THE monohydrate, and CAF monohydrate. The monohydrate of INAM has two polymorphs. DABCO has two known hydrates, a monohydrate that has two polymorphs (QOHYAO, 5H-a18 and QOHYAO01, 5H-b19) and a hexahydrate (QOHYES, 5H-c18). Despite numerous attempts using a variety of methods, we were unable to prepare either pure DABCO monohydrate or pure DABCO hexahydrate. ‘DABCO hydrate (5B)’ in this work thus refers to our prepared hydrate, which is a mixture of monohydrate and hexahydrate.
Sublimation experiments
In order to assess the crystallisation of the hydrates of 1–5 from sublimation, a series of sublimation experiments were carried out in both the presence and the absence of water. Sublimations were performed using approximately 50 mg of each compound, which was placed under static vacuum in a glass tube. The bottom of the evacuated tube was submerged in a pre-heated oil bath until the oil level was just higher than the sample height. Co-sublimations with water were carried out by adding the dry sample to the bottom of the tube, followed by water added using a micropipette. Two different quantities of water were used (30 or 40 μL) in order to assess the effect of increasing water content on the product of the sublimation. Sublimations were continued until sufficient material was obtained for analysis, and all sample at the bottom of the tube had sublimed. Sublimation products were identified using PXRD and TGA. Details are given in the ESI.† The results of the sublimation experiments are summarised in Table 1.
Table 1 Summary of crystalline forms of 1–5 obtained from sublimations of anhydrous and hydrate forms both in the presence and the absence of water. Dark shading indicates experiments where only hydrate was obtained from sublimation. Light shading indicates that a mixture of hydrate and anhydrous forms was obtained
1 was also sublimed with 20 μL water, yielding a mixture of anhydrous and hydrate forms.
One peak indicates possible minor contamination with anhydrous material.
PXRD was inconclusive, but unit cell determination on several crystals confirmed the presence of 2A-a.
Unit cell determination confirmed the presence of 2A-b.
No mass loss from TGA, indicating no hydrate present.
PXRD matched prepared hydrate 5B.
|
|
In several cases, we found that crystals of hydrate could easily be grown from sublimation. With the addition of water, crystals of hydrate could be produced from sublimation using either anhydrous or hydrate starting material.
OA is the only one of the compounds studied that exists as its hydrate, 1H-a, as purchased. Subliming 1H-a in the absence of water yielded a mixture of hydrated and anhydrous forms. It was found that 30 μL water is the optimal amount to add to the sublimation of 1H-a in order to yield pure hydrate 1H-a as the product of the sublimation. When 20 μL was used, a mixture of 1A-b20 and 1H-a resulted (Fig. 1).
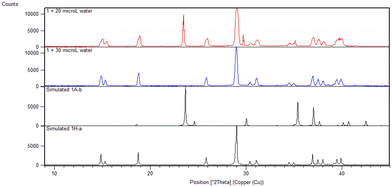 |
| Fig. 1 PXRD pattern of 1 sublimed with 20 μL water (red) and 30 μL water (blue), compared to simulated patterns (black) for the anhydrous and hydrate crystals. | |
Sublimation of the anhydrous form of OA, 1A, in the absence of water yielded a mixture of anhydrous forms 1A-a21 and 1A-b, and hydrate form 1H-a. The presence of hydrate in this product, which was confirmed by TGA (Fig. S52†), must be as a result of the extremely hygroscopic nature of 1A: extensive attempts to prevent the uptake of water by this sample were unsuccessful. These observations agree with the findings of Adams et al.,21 who showed using FTIR that anhydrous OA is extremely hygroscopic. Co-sublimation of 1A with 30 μL water yielded crystals of hydrate 1H-a. Preparation of pure 1H-a from sublimation is straightforward.
The results of sublimations involving 2, 3 and 4 were quite different. Sublimation of 4 as either the anhydrous form or the hydrate yielded anhydrous 4A-b under all conditions investigated. This was confirmed using TGA for some sublimation products. Compound 2 gave hydrate from sublimation only when the hydrate was sublimed in the absence of water. Unexpectedly, subliming the hydrate in the presence of water gave anhydrous product (confirmed by TGA). Sublimation of 3 produced hydrate only when starting with anhydrous material and subliming together with 30 μL water. Subliming with 40 μL water gave anhydrous crystals. These experiments were repeated, with the same results.
Sublimation of 5 gave more varied results. Co-sublimation of the anhydrous form with water gave a mixture of hydrate and anhydrous forms. It was difficult to determine which hydrate was produced, but the PXRD pattern matched that of the synthesised hydrated material (5B). Sublimation of the hydrated material 5B yielded a mixture of hydrated and anhydrous forms under all conditions. TGA showed that the water content of the sublimed crystals increased as the amount of water in the sublimation was increased. An extended study was carried out with 5, where the quantity of water added to the sublimation vessel was increased to 56 or 70 μL. This resulted in an increase in the water content of crystals obtained from the sublimation above that observed with 30 μL, as confirmed by TGA. The 40 μL sublimation resulted in the highest water content (Fig. S47†).
It is clear that in several cases, as the water content in a sublimation is increased, there is an increase in the water content of crystals formed. The specific amount of water required to form purely the hydrate of 1 was successfully established, but this was not obtained for the other systems.
Intermolecular interactions
An analysis of the hydrogen bonding in the hydrates of 1–5 helps to explain the results obtained in the sublimation experiments (Table 2, Fig. 2). Table 2 lists the shortest hydrogen bond between the molecule and water for each hydrate structure, as well as these distances expressed as a fraction of the sum of the van der Waals radii for the atoms involved, to allow straightforward comparison.22Fig. 2 presents this data visually. There is a clear correlation between the length, and by inference, the strength of the hydrogen bond to water and the likelihood of crystallising a hydrate from sublimation. The exception to this trend is the hydrate 5H-b.
Table 2 Shortest hydrogen bond distances from molecules to water in the hydrates of 1–5. The final column is each hydrogen bond distance (O⋯O or N⋯O) expressed as a fraction of the sum of the van der Waals radii for the atoms involved22
Structure |
Hydrogen bond interaction |
Interaction distance (Å) |
Fraction of sum of vdW radii |
1H-a
|
O1⋯O3 |
2.513 |
0.827 |
2H-a
|
O4⋯O2 |
2.780(2) |
0.914 |
2H-b
|
O9⋯N14 |
2.788 (5) |
0.908 |
3H-a
|
N3⋯O2 |
2.757(2) |
0.898 |
4H-a
|
O3⋯N4 |
2.815(5) |
0.917 |
5H-a
|
O1⋯N2 |
2.973(2) |
0.968 |
5H-b
|
O1⋯N2 |
2.928(3) |
0.954 |
5H-c
|
O4⋯N5 |
2.708(3) |
0.882 |
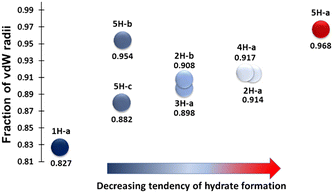 |
| Fig. 2 Tendency of hydrate formation as a function of length of the hydrogen bond to water (expressed as a fraction of the sum of the van der Waals radii of the atoms involved) for the hydrates of 1–5. 1H-a is the most stable from of OA, and it is often observed from sublimation. In contrast, 5H-a is never observed, even by mechanochemistry. 2H-b and 3H-a are seldom observed by sublimation, but are the hydrate forms obtained from mechanochemistry. 2H-a was only observed by sublimation. 4H-a is the hydrate form obtained by mechanochemistry, but is not observed by sublimation. 5H-b and 5H-c are observed both from sublimation and by mechanochemistry. | |
To further investigate the interactions within these hydrates, the intermolecular interaction energies within the hydrate crystal structures were calculated using the UNI force field in Mercury.23,24
In the crystal structure of OA dihydrate 1H-a, there is one dominant interaction between OA and water, with an interaction energy of −20.5 kJ mol−1. The second-highest interaction, with an energy of −12.4 kJ mol−1, is also between OA and water. The interactions between two OA molecules are weaker, with an energy of −11.6 kJ mol−1 (Fig. S76†). For all three DABCO hydrates (5H-a/b/c), the most favourable interactions are also between DABCO and water. For the hydrates of INAM, THE and CAF, however, the situation is quite different. In all three of these materials, the interactions between the molecules are substantially more favourable than any interactions to water. For example, in 4H-a,25 the most favourable interaction of −62.9 kJ mol−1 is between CAF molecules, and the interaction between CAF and water is only the 12th highest interaction, with an energy of −1.2 kJ mol−1.
These simple calculations confirm that those compounds which are most likely to give crystals of hydrate from sublimation are those where the interaction between water and the molecule is more favourable than the interactions between the molecules.
A series of stability studies (see ESI†) confirmed that the hydrates of 1 and 5 (5B) are relatively stable under ambient conditions. The hydrate of 2, however, slowly loses water under ambient conditions, implying that 2 is more stable in its anhydrous form. The anhydrous form of 5 has a water content that slowly increases from zero under ambient conditions. These results align with the results of our sublimation experiments: it is easier to form a hydrate from sublimation if the hydrate form of a material is more stable than the anhydrous form.
Competition experiments
A series of competition experiments were also conducted in order to establish whether water would transfer between forms in a predictable manner during a sublimation experiment, i.e. would the water transfer to the molecule with the more favourable hydrogen bond to water. Co-sublimations were carried out with equimolar quantities of one hydrate and one anhydrous material, and the sublimation temperatures were selected to be at a temperature above the sublimation temperature of both hydrate and anhydrous component to ensure both components entered the gas phase. Three systems were investigated: hydrate 1 + anhydrous 4, hydrate 1 + anhydrous 5, and hydrate 2B + anhydrous 5. A schematic summary of these co-sublimation results is presented in Fig. 3, and experimental details are given in Table S8.†
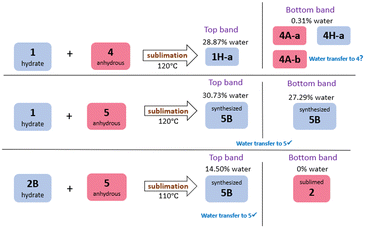 |
| Fig. 3 Summary of results from competition experiments based on 1 : 1 mole-ratio co-sublimations. | |
In all three sublimation experiments, two separate bands of crystals formed in the sublimation tube, which we refer to as the ‘top band’ and the ‘bottom band’. When co-subliming hydrate 1 and anhydrous 4 at 120 °C (Fig. S66–S69†), the top band contained 1H-a, whilst the bottom band appeared to contain a mixture of 4A-a,264A-b, and 4H-a, i.e. there is some evidence for the presence of crystalline caffeine monohydrate. Although the PXRD was not conclusive, TGA confirmed the presence of a small amount of water in this sublimed material. This is an extremely unexpected result, which we are currently investigating further.
The co-sublimation with 1H-a and 5 gave two bands of product. Both of these have PXRD patterns that match the synthesised hydrate of 5, 5B (Fig. S70–S72†). Co-sublimation of the hydrate of 2, 2B, with anhydrous 5 gave two products: the top band had a PXRD pattern matching 5B, and the bottom band matched the PXRD of sublimed anhydrous 2. The transfer of water from 2B to 5 is expected, and aligns with what would be predicted based on the relative hydrogen bond strengths to water. The transfer of water from 1 to 5 in fact is also in keeping with the hydrogen bond strengths: although the hydrogen bonds between 5 and water are not always particularly short, the calculations showed that in all three hydrates of 5, these hydrogen bonds are very favourable.
Conclusions
In summary, we have shown that crystals of molecular hydrates can be grown from the gas phase via co-sublimation in the presence of water. The stronger the interaction between the molecule in question and water within the hydrate crystal, the more likely it is that crystalline hydrate can easily be produced from sublimation. An increased quantity of water in the sublimation vessel results in an increased water content of the sublimed crystals. Competition experiments have shown that water transfer can take place between compounds during co-sublimation. The crystallisation of caffeine hydrate crystals by sublimation indicates that sublimation may be a route to obtaining crystals of otherwise unstable hydrates, a potentially significant advantage of this crystallisation technique that warrants further investigation. In general, these results highlight that sublimation is an important technique to consider when trying to produce crystals of molecular hydrates. Hydrates are essentially a subclass of multi-component crystals, and the results here could also be applied to other multicomponent systems such as co-crystals and salts. Co-sublimation of two single components, or of a mixture of multicomponent materials (competition experiments) may uncover new forms of multicomponent materials.
Experimental
Compounds 1–5 were purchased from Sigma-Aldrich/Merck South Africa and used without additional purification. All of these materials have multiple crystal forms. The forms encountered in this study are listed with their corresponding CSD refcodes in Tables S1–S5.†
Characterisation
Room temperature Powder X-ray diffraction (PXRD) was performed using a Bruker D2 Phaser benchtop diffractometer which was equipped with a copper X-ray radiation source (λ = 1.54184 Å), operating at a voltage of 30 kV and a current of 10 mA. Samples were loaded onto a zero-background holder and data were collected in the 2θ range of 5–45° at a speed of 0.500 s per step, with a set step size of 0.016°, and with a variable rotation of 30 rpm. The data were processed and analysed with the aid of X'Pert HighScore Plus.
TGA analyses were carried out using a TA Instruments Q500 analyser. Sample weights were in the range of 1–5 mg. Samples were placed in a 6.7 mm aluminium sample pan and heated to a temperature of 600 °C with a ramp rate of 10 °C min−1, starting at room temperature. A dry N2 gas flow rate of 50 ml min−1 was used in order to purge the furnace. The thermograms collected were analysed with the aid of TA Instruments Universal Analysis software.
Preparation of materials
Anhydrous OA synthesis.
The method employed to synthesise anhydrous OA was adapted from a study by Okazaki et al.27 Anhydrous OA was successfully synthesised from OA dihydrate 1H-a13 (white crystals), by adding ca. 50 mg of compound 1 to a clean dry thin Schlenk tube, which was evacuated with a 4.57 mbar line pressure. The tube was placed in a pre-heated oil bath at 80 °C and left to dehydrate over a period of 5 days. White/clear block-like crystals were obtained. PXRD was performed on the crystals to confirm the formation of anhydrous OA in the form of 1A-a21 and 1A-b2 (Fig. S7†).
DABCO hydrate synthesis.
The method employed to synthesise DABCO hydrate was adapted from a study by Laus et al.18 Anhydrous DABCO as purchased (0.56 g, clear block-shaped crystals), was stirred for 5 minutes in 8 ml THF with 540 μL water added, and then refrigerated for a period of three days. PXRD (Fig. S8†) was performed on the crystals, confirming that a mixture of monohydrate and hexahydrate was obtained, with some anhydrous crystals (5H-b,195H-c,185A-a18). A TGA analysis was carried out and a mass loss of 26.77% water was observed (Fig. S9†). Several attempts to synthesise either purely DABCO monohydrate or DABCO hexahydrate were unsuccessful.
Mechanochemistry.
The hydrates of INAM, CAF, and THE were synthesised mechanochemically using an FTS1000 Shaker Mill from Form-Tech Scientific. Samples were placed in two 5 ml Teflon lined grinding jars (internal diameter of 10.2 mm) with two 3 mm steel balls per jar (total mass of both balls = 0.520 g). Milling was done using ca. 50 mg anhydrous material as purchased, together with 30 μL water added using a 10–100 μL micropipette. Samples were milled at room temperature at a speed of 1800 rpm; for 10 minutes in the case of THE and INAM, and 30 minutes in the case of CAF. The successful synthesis of the hydrates was confirmed using PXRD (Fig. S10, S12 and S14†) and TGA (Fig. S11, S13 and S15†), which showed that hydrates 2H-b,283H-a29 and 4H-a25 were made using this method.
Sublimations
All sublimations were performed under static vacuum using clean and dry Schlenk tubes (length excluding cap = 155.4 mm, internal diameter = 8 mm). Sublimation experiments were performed with approximately 50 mg sample (specified in Tables S6 and S7†) in a thin glass Schlenk tube which was evacuated with a 4.57 mbar line pressure. The bottom of the tube was placed in a pre-heated oil bath at the appropriate sublimation temperature (see Tables S11 and S12†). When preparing co-sublimations with water, the dry anhydrous or hydrate sample was added to the Schlenk tube first, followed by water added with a micropipette (10–100 μL). The duration of each sublimation experiment varied: in some cases longer sublimation times were required in order to allow a sufficient quantity of material for further analysis to form. In general, sublimations were carried out until all the sample had sublimed. All sublimations were carried out in a laboratory with a regulated temperature of 18 °C. Many sublimation experiments were carried out in duplicate (see ESI† for details). In all cases the results were reproducible, unless explicitly stated otherwise.
Intermolecular potentials
The interaction energies present within the crystal structure of the hydrate form(s) of compounds 1–5 were calculated using the UNI23 intermolecular potential calculator in Mercury24 (Fig. S76–S82†).
Author contributions
DAH conceptualised the project, assisted with data analysis supervised ALV and edited the manuscript. ALV carried out the investigation and wrote the first draft.
Conflicts of interest
There are no conflicts to declare.
Acknowledgements
ALV thanks the National Research Foundation of South Africa for a bursary. This work is based on the research supported in part by the National Research Foundation of South Africa (Ref Number SRUG2204224316).
References
- L. Casali, L. Mazzei, O. Shemchuk, L. Sharma, K. Honer, F. Grepioni, S. Ciurli, D. Braga and J. Baltrusaitis, ACS Sustainable Chem. Eng., 2019, 7, 2852–2859 CrossRef CAS.
-
S. R. Byrn, G. Zografi and X. Chen, Solid State Properties of Pharmaceutical Materials, John Wiley & Sons, Inc., Hoboken, 2017 Search PubMed.
- R. V. Kent, R. A. Wiscons, P. Sharon, D. Grinstein, A. A. M. Frimer and A. J. Matzger, Cryst. Growth Des., 2018, 18, 219–224 CrossRef CAS.
- C. Aakeröy, Acta Crystallogr., Sect. B: Struct. Sci., Cryst. Eng. Mater., 2015, 71, 387–391 CrossRef PubMed.
- E. Jurczak, A. H. Mazurek, L. Szeleszczuk, D. M. Pisklak and M. Zielińska-Pisklak, Pharmaceutics, 2020, 12, 959–960 CrossRef CAS PubMed.
- S. R. Vippagunta, H. G. Brittain and D. J. Grant, Adv. Drug Delivery Rev., 2011, 48, 3–26 CrossRef PubMed.
- P. M. J. Szell, S. A. Gabriel, E. Caron-Poulin, O. Jeannin, M. Fourmigué and D. L. Bryce, Cryst. Growth Des., 2018, 18, 6227–6238 CrossRef CAS.
- L. Lombard, D. A. Haynes and T. le Roex, Cryst. Growth Des., 2020, 20, 7840–7849 CrossRef.
- P. McArdle and A. Erxleben, CrystEngComm, 2021, 23, 5267–5283 RSC.
- J. Lombard, V. J. Smith, T. le Roex and D. A. Haynes, CrystEngComm, 2020, 22, 7826–7831 RSC.
- J. Lombard, T. le Roex and D. A. Haynes, Cryst. Growth Des., 2020, 20, 7384–7391 CrossRef CAS.
- C. R. Groom, I. J. Bruno, M. P. Lightfoot and S. C. Ward, Acta Crystallogr., Sect. B: Struct. Sci., Cryst. Eng. Mater., 2016, 72, 171–179 CrossRef CAS PubMed.
- R. G. Delaplane and J. A. Ibers, Acta Crystallogr., Sect. B: Struct. Crystallogr. Cryst. Chem., 1969, 25, 2423–2437 CrossRef CAS.
- C. B. Aakeröy, A. M. Beatty, B. A. Helfrich and M. Nieuwenhyzen, Cryst. Growth Des., 2003, 2, 159–165 CrossRef.
- Y. Ebisuzaki, P. D. Boyle and J. A. Smith, Acta Crystallogr., Sect. C: Cryst. Struct. Commun., 1997, 53, 777–779 CrossRef.
- A. Martin and A. A. Pinkerton, Acta Crystallogr., Sect. B: Struct. Sci., 1998, 54, 471–477 CrossRef.
- J. L. Sauvajol, J. Phys. C: Solid State Phys., 1980, 13, L927 CrossRef CAS.
- G. Laus, V. Kahlenberg, K. Wurst, T. Lörting and H. Schottenberger, CrystEngComm, 2008, 10, 1638–1644 RSC.
- B. Wicher and M. Gdaniec, Acta Crystallogr., Sect. C: Cryst. Struct. Commun., 2010, 66, o270–o273 CrossRef CAS PubMed.
- J. L. Derissen and P. H. Smith, Acta Crystallogr., Sect. B: Struct. Crystallogr. Cryst. Chem., 1974, 30, 2240–2242 CrossRef.
- J. Adams and H. Kim, Spectrochim. Acta, 1973, 29, 675–677 CrossRef CAS.
- see for example R. Puttreddy, O. Jurček, S. Bhowmik, T. Mäkeläa and K. Rissanen, Chem. Commun., 2016, 52, 2338–2341 RSC.
- A. Gavezotti, Acc. Chem. Res., 1994, 27, 309–314 CrossRef CAS; A. Gavezzotti and G. Filippini, J. Phys. Chem., 1994, 98, 4831–4837 CrossRef.
- C. F. Macrae, I. Sovago, S. J. Cottrell, P. T. A. Galek, P. McCabe, E. Pidcock, M. Platings, G. P. Shields, J. S. Stevens, M. Towler and P. A. Wood, J. Appl. Crystallogr., 2020, 53, 226–235 CrossRef CAS PubMed.
- D. J. Sutor, Acta Crystallogr., 1958, 11, 453–458 CrossRef CAS.
- G. D. Enright, V. V. Terskik, D. H. Brouwer and J. A. Ripmeester, Cryst. Growth Des., 2007, 7, 1406–1410 CrossRef CAS.
- N. Okazaki and E. Takemura, Bull. Chem. Soc. Jpn., 1961, 7, 977–982 CrossRef.
- N. B. Báthori, A. Lemmerer, G. A. Venter, S. A. Bourne and M. R. Caira, Cryst. Growth Des., 2011, 11, 75–87 CrossRef.
- S. Majodina, L. Ndima, O. O. Abosede, E. C. Hosten, C. M. A. Lorentino, H. F. Frota, L. S. Sangenito, M. H. Branquinha, A. L. S. Santos and A. S. Ogunlaja, CrystEngComm, 2021, 23, 335–352 RSC.
|
This journal is © The Royal Society of Chemistry 2023 |
Click here to see how this site uses Cookies. View our privacy policy here.