DOI:
10.1039/D0RA05610C
(Review Article)
RSC Adv., 2020,
10, 38379-38403
Heavy metal induced stress on wheat: phytotoxicity and microbiological management
Received
27th June 2020
, Accepted 17th September 2020
First published on 19th October 2020
Abstract
Among many soil problems, heavy metal accumulation is one of the major agronomic challenges that has seriously threatened food safety. Due to these problems, soil biologists/agronomists in recent times have also raised concerns over heavy metal pollution, which indeed are unpleasantly affecting agro-ecosystems and crop production. The toxic heavy metals once deposited beyond certain permissible limits, obnoxiously affect the density, composition and physiological activities of microbiota, dynamics and fertility of soil leading eventually to reduction in wheat production and via food chain, human and animal health. Therefore, the metal induced phytotoxicity problems warrant urgent and immediate attention so that the physiological activities of microbes, nutrient pool of soils and concurrently the production of wheat are preserved and maintained in a constantly deteriorating environment. To mitigate the magnitude of metal induced changes, certain microorganisms have been identified, especially those belonging to the plant growth promoting rhizobacteria (PGPR) group endowed with the distinctive property of heavy metal tolerance and exhibiting unique plant growth promoting potentials. When applied, such metal-tolerant PGPR have shown variable positive impact on wheat production, even in soils contaminated with metals, by supplying macro and micro nutrients and secreting active biomolecules like EPS, melanins and metallothionein (MTs). Despite some reports here and there, the phytotoxicity of metals to wheat and how wheat production in metal-stressed soil can be enhanced is poorly explained. Thus, an attempt is made in this review to better understand the mechanistic basis of metal toxicity to wheat, and how such phytotoxicity can be mitigated by incorporating microbiological remediation strategies in wheat cultivation practices. The information provided here is likely to benefit wheat growers and consequently optimize wheat production inexpensively under stressed soils.
1 Heavy metals in soils: source and availability
Soil is considered a natural habitat of heterogenous microbial communities, and may become contaminated by the deposition of heavy metals (HMs): metals and metalloids having densities greater than 5 g cm−3. Hence, the cultivable land around the world is declining due to soil pollution, which has become a serious problem in many countries.1 Heavy metals occur naturally in soil ecosystems,2,3 resulting from the pedogenetic processes of weathering of parent materials at levels that are regarded as trace (<1000 mg kg−1) and rarely toxic.4,5 Other sources of HM pollution to agronomic soils include emissions from volcanoes, dispersal of metal-containing dusts, and the breakdown product of rocks enriched with HM.6 Apart from these natural sources, HM can also be added extensively to cultivable soils emanating from anthropogenic activities (Fig. 1), such as those involving rapidly expanding industries, mines and smelters,7,8 disposal of HM wastes, gasoline and paints, land application of fertilizers,9,10 biosolids (e.g. livestock manure, compost, and municipal sewage sludge),11,12 pesticides for example, copper-containing fungicidal sprays such as Bordeaux mixture (copper sulphate) and copper oxychloride and lead arsenate,13 unprocessed wastewater used in irrigation,14 coal combustion residues, spillage of petrochemicals, and atmospheric deposition.15,16
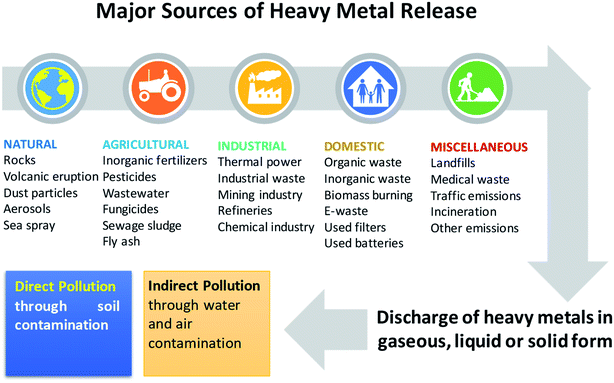 |
| Fig. 1 Sources of heavy metal pollution in the environment. | |
Heavy metals also enter the soil through road traffic and road dust,17–19 and the burning of tires and brake linings.20,21 Some other notable sources that can also add adequate quantities of HM to soils include fly ash originating from coal-fired power plants,22 PVC products, colour pigment, and several alloys and chargeable Ni–Cd batteries.23
All of the anthropogenic activities causing soil contamination have broadly been grouped into five categories: (i) mining and smelting, (ii) industries (e.g., As, Cd, Cr, Co, Cu, Hg, Ni and Zn), (iii) agriculture (e.g., As, Cd, Cu, Pb, Se, U and Zn), (iv) atmospheric deposition (As, Cd, Cr, Cu, Pb, Hg and U), and (v) waste disposal (e.g., As, Cd, Cr, Cu, Pb, Hg and Zn). Heavy metals added to soils from anthropogenic sources are generally more mobile and therefore, easily bioavailable.24,25 However, the total pool of HMs in soils does not provide any information regarding its mobility and availability. Yet, the fate and movement of HMs in soil depends significantly on the composition, concentration and speciation of metals. The distribution of HMs in soils is controlled by: (i) precipitation and dissolution, (ii) ion exchange, adsorption and desorption, (iii) aqueous complexation, (iv) biological immobilization and mobilization, and (v) plant uptake.26 Based on these properties, soil metals have been grouped into five key geochemical categories: (i) exchangeable, (ii) metal bound to carbonate phase, (iii) metal attached to Fe and Mn oxides, (iv) bound to organic matter, and (v) residual metal. Metals found in one or the simultaneous category in soils, however, differ significantly in bioavailability, mobility and speciation largely due to their ability to react rapidly with low-molecular organic acids, carbohydrates, and enzymes excreted by soil microbiota.27 In addition, the surface of microbial communities inhabiting the soil has charges which help them to interact very strongly with metal ions in soil solution.28 For example, symbiotic PGPR have been found to adsorb Cu and Cd in soil, when applied as inoculant.29 However, how soil organisms affect the speciation and distribution of metals in soils are inadequately explained. In order to better understand these, scientists working in different areas have employed numerous strategies, like soil column leaching experiments, sequential extraction and the single extraction approach. However, none of these methods have been completely successful in predicting/establishing the complexation of variable soil metals. Hence, it did not pinpoint the mobility and bioavailability of elements.30,31 Still, among these methods, the chemical extraction method is frequently used to determine the bioavailability or mobility of heavy metals,32,33 which also provides information regarding metal availability and transport of metals to plants.34 In summary, the availability of heavy metals in soils is affected by factors such as: (1) metal species and influence of environmental factors,35 (2) structure and compositions of soil, (3) genotypes and plant photosynthates, (4) soil–crop–microbes interactions, and (5) use of agrochemicals in cultivation practices, water management, and crop rotation systems.36,37
2 Heavy metal–plant interactions
2.1 Heavy metal toxicity to plants: impact on physiological processes
The rapidly growing industries, uncontrolled and untreated discharge of xenobiotic pollutants, and use of poor-quality waters (wastewater) for irrigation in agricultural practices pose severe unbearable danger to the sustainability of agroecological niches.38,39 However, the availability of metals for plants is governed by several soil factors, such as pH, cation exchange capacity (CEC), organic matter content and adsorption by clays.40,41 Heavy metals, following accumulation within soil, enter the food chain38,42,43 and are subsequently transferred to the end consumers, leading to human health problems.44 The toxicity of heavy metals that enter vegetal tissues, however, can inhibit multiple physiological processes of plants,45,46 including wheat47,48 and eventually human health (Fig. 2). Briefly, metals at exceedingly higher concentrations damage plants by: (i) altering membrane permeability,49 (ii) inhibiting physiologically active enzymes,50 (iii) inactivating photosystems,51 and (iv) disturbing mineral metabolism.52 Apart from these, the metal toxicity causes oxidative stress, disruption of pigment function and alteration in protein activity.53 The hyper generation of ROS under metal pressure may cause significant damage to cell structures in plants, such as: (i) oxidation of proteins and lipids, (ii) nucleic acid damage, (iii) enzyme inhibition, and ultimately (iv) cell death.54,55 Some of the physiological processes of plants impacted adversely by heavy metals are briefly discussed in the following section.
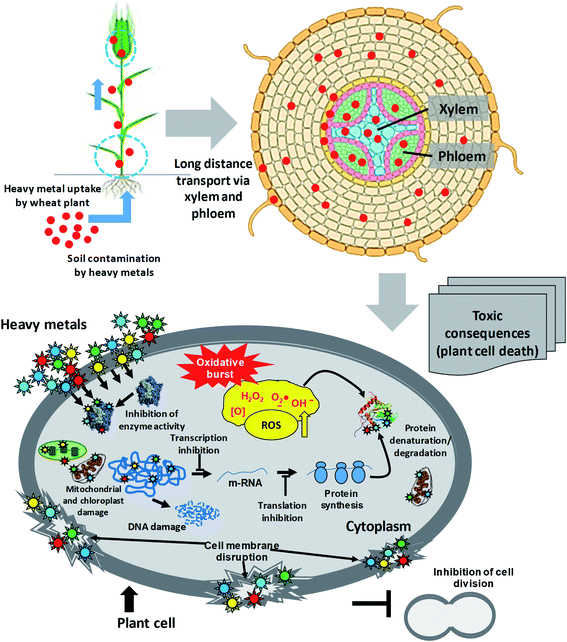 |
| Fig. 2 Sequence of events from metal entry into a plant cell to the death of the plant. | |
2.1.1 Germination and seedling growth.
The majority of studies have been conducted to assess the impact of different heavy metals on live plants,56,57 using seedlings or adult plants. However, in some studies, seeds have also been exposed to metals.58 Hence, the seed germination process becomes an important aspect among many plant physiological processes. Since seed germination is the first physiological process affected by metals, the ability of seeds to germinate in a polluted environment indicates its level of tolerance to metals.59 Seeds possess certain sensing mechanisms, which allow them to germinate under favourable environmental conditions and to complete the developmental process. Despite these properties, seed germination, growth and the production of many plants have been found to be adversely affected when exposed to metals.60,61 For example, metals, such as Hg, Cd, Co, Cu, Pb, Zn, Ni, Ag, have shown variable impact on the germination of seeds of many plants,62 including wheat.48 In a prior study, the phytotoxic effect of Cr on seed germination and seedling growth of some wheat cultivars (HD2956, HD2932, DBW14, KO512, WH775) individually treated with 25, 50, 75, 100 and 125 ppm of Cr(VI) was variable. A consistent increase in Cr(VI) concentration significantly inhibited seed germination, and the percentage phytotoxicity increased with the gradual increase in the Cr(VI) level for all wheat cultivars. Among the measured parameters, root growth was maximally reduced.63
After seeds, the emerging roots are the first organ that comes in direct contact with the various rhizosphere constituents.64 When a root absorbs water or nutrients from soil, ions (including those of HMs) and molecules move toward this organ both by mass flow (along with soil water) and by diffusion process. Root growth is more sensitive to heavy metal contamination.65,66 An immediate effect of the high concentration of metal results in root growth inhibition, which may be shorter, very ramified and without a solid structure.67,68 The inhibition of root elongation is accompanied by alteration in the architecture and morphology of roots. For instance, many heavy metals, including Pb and Cr, have been reported to rapidly inhibit the root growth, leading to biomass reduction of wheat,65 probably due to the inhibition of cell division in the root tip.69,70 In other experiments, wheat71,72 has shown a decrease in length and in the dry mass of roots when grown under Pb stress.73 Mechanistically, the distention and lesions in the cell wall of wheat roots occur due to the activation of certain wall-degrading enzymes in response to Pb exposure.74
2.1.2 Cell wall and plasma membrane.
The cell walls are the first structures of the roots that are exposed to metals, if present in soils. From the soil, it enters the root tissues and forms a complex with the carboxylic groups of the pectin constituents of cell walls.75 The binding of metals in the cell wall and/or to the apoplastic face of the plasma membrane may impair apoplasmic and symplasmic cell metabolism, leading to metal-induced inhibition of root elongation.76 The inhibition of root elongation is accompanied by changes in the architecture and morphology of the roots. A reduction in the formation of the lateral roots and root hairs, changes in colour, thickening, atrophy and curvature of the roots are common symptoms.77 The duration of exposure and concentration of metals may influence the cell wall rigidity, causing the rupture of the rhizodermis and outer cortex of the meristem, which may inhibit the elongation of root tips.78,79 The plasma membrane is yet other site to which any metal can bind and disrupt membrane functions.80 After interaction, the metal induces changes in the membrane constituents, especially lipids, resulting in an altered structure and physiological functions of the membrane and other cellular processes. For instance, the variation in the composition and fluidity of membrane lipids, oxidation and the cross-linking of protein thiols, and the destruction of some important membrane proteins are some of the toxic consequences of metals.81 Among different metals, the effect of Cr on the transport activities of the plasma membrane has been reported.82 The inhibition of ATPase activity is suggested to be due to the disruption of the membrane by free radicals generated under metal stress.83 The decrease in ATPase activity reduces proton extrusion, and ultimately decreases the transport activities of the root plasma membrane. As a result, the uptake of nutrients by roots is limited. Moreover, it is also reported that Cr interferes with the mechanism controlling intracellular pH.84 Mechanistically, Cr alters the metabolic activities of plants as it: (i) modifies the production of photosynthetic pigments (like chlorophyll), (ii) increases the production of metabolites (for example glutathione85 and ascorbic acid86) as a direct response to metal stress, which may damage the plants. Moreover, Cd treatment also reduces the ATPase activity of the plasma membrane fraction of roots.87
2.1.3 Peroxidation of membrane lipids.
The alteration in membrane functions due to metal action leads to changes both in the structure and peroxidation of membrane lipids.88,89 For example, Pb contamination has led to the excessive production of ROS (radical O2−) and hydrogen peroxide (H2O2) in plant cells.90,91 However, the excessive generation of ROS under metal stress is a common feature of plants, which may react with lipids, proteins, photosynthetic pigments, and other cellular organelles. It can cause lipid peroxidation and membrane damage, eventually leading to cell death.92,93 Malondialdehyde (MDA) is one of the ultimate products of lipid peroxidation, whose level is influenced by the degree of membrane lipid peroxidation.94 Malondialdehyde, synthesized in plants as a result of the oxidation of polyunsaturated fatty acids (PUFAs), has a potential role in damaging the cell membrane.95 However, the concentration of MDA increases when plants suffer from excessive oxidative stress, and are unable to scavenge the ROS.96 Since lipid peroxidation may serve as a biomarker for oxidative stress in plants, the MDA content in plants (including those in wheat leaves) is generally determined to assess the extent of metal toxicity. Some ROS even alter gene expression and modulate the activity of specific proteins involved in the plant defense system.97 The increased lipid peroxidation also changes the membrane properties, such as fluidity and permeability, and modulates the activities of membrane-bound ATPases.98 Undeniably, peroxidation is a chain reaction, wherein unsaturated fatty acids are transformed into different small hydrocarbon fragments, such as malondialdehyde.99 The lipid peroxidation processes and ensuing substances, in effect, sternly affect the physiological functions of the plasma membrane, leading eventually to the death of the cells.100,101 In a similar study,45 confirmed Cr-induced membrane damage, lipid peroxidation reduction in plant growth and yield, emulation of oxidative stress, and anti-oxidative defense. In addition, the overproduction of ROS exhausts ATP, reduces respiration rates and affects growth.45,102 In another experiment, the gradual accumulation of Cu in the plant tissues caused certain specific changes in the composition of lipids, for instance: (i) the content of sulfolipids in chloroplasts was declined, (ii) the content of monogalactosyl diacylglycerols, digalactosyl diacylglycerols and phosphatidyl glycerols in chloroplasts and mitochondria grew after an hour of Cu exposure, (iii) and the content of all lipids except phosphatidic acids decreased after 3 h of exposure.100 Other metals (like Fe and Cu compounds) have been found to generate more free radicals, and consequently increased the peroxidation.103
2.1.4 Photosynthesis.
Among various metabolic processes, photosynthesis is one of the most significant physiological traits of plants. However, it has been reported to be deleteriously impacted by numerous heavy metals.104 The toxic metals attack different photosynthetic apparatus and cause: (i) the deposition of metals in the plant foliage,105 (ii) a change in the physiological activity of the chloroplast membrane and distribution of metals in leaf tissues, such as the stomata, mesophyll and bundle sheath,106 (iii) reduction in the formation of photosynthetic pigments,107,108 (iv) alteration in the cytosolic enzymes and organics,109 (v) variation in the supra-molecular level action, especially on photosystem I, photosystem II, membrane acyl liquids and the carrier proteins of the vascular tissues,110 and (vi) the destruction of enzymes associated with photosynthetic carbon reduction (PCR) and the xanthophyll cycle.50,110 The decrease in the chlorophyll ratio under metal stress could possibly be due to the destabilization and destruction of peripheral proteins.111 Among metals, Cd and Zn, for example, have been reported to induce various components of photosynthesis, such as pigments and light capture centre, thylakoid ultrastructure and photosynthetic electron transport, stomatal conductance and access of CO2, and activities of the Calvin cycle enzymes.112,113 Both Cd and Zn significantly decreased the activities of photosystem II (PSII) and, to a lesser extent, also of photosystem I (PSI), as well as the rate of photosynthetic electron transport.114 However, the effects of high Cd and excess Zn concentrations on the light-dependent photosynthetic processes are still not fully understood. Due to the altered photosynthetic activity, plants generally exhibit leaf chlorosis115 and stunted growth.116 As a result, HMs decrease the plant biomass by disturbing photosynthesis, respiration and other metabolic processes.51 As an example, the exposure of wheat to high Cd2+ (50 μM) and Zn2+ (600 μM) concentrations resulted in similar relative growth rate (RGR) inhibitions by about 50% and comparable retardations of the CO2 assimilation rates (about 30%) in the second developed leaf of the wheat seedlings. Moreover, the fluorescence analysis of chlorophyll a indicated that both metals disturbed photosynthetic electron transport processes, causing a 4- to 5-fold suppression of the efficiency of energy transformation in photosystem II. The non-specific toxic effects of Cd and Zn, which prevailed, were an inactivation of part of the photosystem II reaction centres and their transformation into excitation-quenching forms, as well as disturbed electron transport in the oxygen-evolving complex.104
Since heavy metals trigger the oxidative damage within the plant cell, it is very likely that the metals could inhibit the synthesis of certain enzymes involved in chlorophyll synthesis. Hence, the chlorophyll concentration could be reduced under heavy metal stress.
2.1.5 Antioxidant defense system in plants.
Plants, while growing in the metal-enriched environment, suffer heavily from oxidative damage.117 In addition, the increased concentrations of heavy metals decrease the activity of antioxidant enzymes.118,119 Mechanistically, auto-oxidation and the Fenton reaction may cause the oxidative loss of the defense enzymes. For example, in a previous study, O2− was found to directly inhibit the catalase activity.120 However, the overproduction of ROS under metal stress is a common reaction of plants toward stressor molecules, which sequentially lead to the death of plants by first altering the photosynthetic pigments, lipids, proteins, and other cellular organelles, resulting in lipid peroxidation and membrane damage.93,94 The excessive secretion of ROS also depletes ATP and hence, adversely affects the respiration rates, eventually leading to weakened plant growth. However, to protect cells and tissues from injury and dysfunction, plants have evolved to have a broad range of antioxidative defense systems (both constitutive or induced one) to quench ROS and consequently, to prevent oxidative damage.121,122 Some notable antioxidants providing protection to plants include guaiacol peroxidase (GPx), ascorbate peroxidase (APx), superoxide dismutase (SOD), catalase (CAT), peroxidase (POX), and glutathione reductase.123,124 Apart from these antioxidants, plants also synthesize and secrete low molecular weight non-enzymatic antioxidants, such as proline,125 cysteine,126 non-protein thiol,127 ascorbic acid,128 and glutathione,129 which reduce oxidative stress by scavenging ROS130,131 and protect the cellular structures.132 However, the release of these active molecules differs with plant genotypes, types of tissues and metal speciation.133 For example, an increase in the H2O2 content upon Pb exposure caused a substantial increase in CAT activity in Triticum aestivum.74 However, ref. 134 and 74 reported a decline in the activity of POXs in Elsholtzia argyi and Triticum aestivum roots, respectively, upon Pb exposure. Therefore, from these studies, it was concluded that a higher concentration of Pb or its longer exposure inhibited cell metabolism and H2O2 production, which in turn, decreased the CAT activity.130
2.1.6 Induction of proline.
Among various non enzymatic molecules, proline (a multifunctional amino acid) is reported to accumulate in higher concentrations in plant tissues exposed to various stressor molecules.135,136 Physiologically, proline helps plants maintain osmotic regulation and homeostasis.137 Proline also protects plants from the destructive effects of ROS138 since proline acts as a singlet oxygen quencher and as a scavenger of OH radicals.139,140 Thus, proline is not only an important molecule in redox signaling, but also an effective quencher of reactive oxygen species formed in all plants against abiotic stress. In other plants, proline was produced as an indicator of stress.132 Similarly, ref. 141 reported that proline did not contribute to a great extent in conferring tolerance to plants against Cd stress. This could have been due to the fact that endogenous levels of proline were negatively correlated to the shoot (r = −0.397) and root dry weights (r = −0.432), chlorophyll a (r = −0.361) and H2O2 (r = −0.194). In summary, the damaging effects of toxic heavy metals on the performance of plants and soil health are illustrated in Fig. 3.
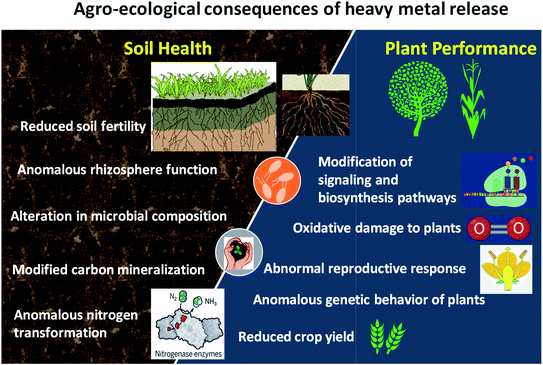 |
| Fig. 3 Agro-ecological consequences of heavy metals on soil and plant health. | |
3 Wheat: production, nutritional composition and importance in human health
Common wheat (Triticum aestivum L. em Thell.), also known as bread wheat, is a major strategic cereal crop that plays an important role in the traditional human health system, particularly in developing countries. Wheat is a major staple food crop for about 36% of the world population, including those of people from Asian countries. Among various wheat producing countries, India ranks second after China followed by United States, Russian Federation, France, Australia, Germany, Ukraine, Canada, Turkey, Pakistan, Argentina, Kazakhstan and United Kingdom.142 India contributes approximately 11.9% to the world wheat production from about 12% of global area.143 Nutritionally, wheat is a major source of energy (carbohydrate), but it also contains a significant amount of other important nutrients, including proteins, fiber, and minor components such as lipids, vitamins, minerals, and phytochemicals.144,145 Worldwide, wheat provides nearly 55% of the carbohydrates and 20% of the food calories consumed globally.146 Even though wheat acts as a major constituent of human dietary systems, about 10% of wheat is retained for seed and industry (for production of starch, paste, malt, dextrose, gluten). Due to the nutritive ingredients, consumption of wheat is increasing globally as wheat-based foods provide a range of essential and beneficial components to the human diet. In regards to health, cereal dietary fiber reduces the risk of cardiovascular disease, type 2 diabetes and forms of cancer, notably colorectal cancer.147
4 Heavy metal–wheat interaction: an overview
Like many plants, wheat is also sensitive to heavy metals.148,149 Once heavy metals enter the plants, the stress triggers different responses in plants, ranging from germination to growth,150 to biochemical responses104 and yield losses in wheat.151,152 Among various toxic metals, Cd for instance has been found highly dangerous to wheat,153,154 resulting in stunted growth and altered membrane permeability, leading eventually to the production of ROS.155 The accumulation of ROS within plant tissues, in turn, causes the leakage of electrolytes and disrupts the integrity of the cell membrane.156 As a result, the oxidation of membrane proteins and lipids is usually disrupted, which results in cell death.157 In a similar study, ref. 158 evaluated the toxicity of six experimental concentrations (0.03; 0.06; 0.12; 0.6; 1.2; 2.4; 4.8 mM) of Cd on seed germination and seedling growth (index of velocity of germination (IVG), length of aerial section and root of seedlings (green and dry mass of the seedlings) of wheat. Results suggested that Cd induced a decrease in the normal germination percentage and IVG. The inhibitory effect of Cd on the initial growth of seedlings influenced the growth of the roots and aerial parts, and also reduced the production of green and dry mass of seedlings. Conclusively, the accumulation of Cd in the soil affected the viability and production of wheat largely due to the absorption of this metal by the plant roots. Ref. 159, in a pot experiment conducted at the Old Botanical Garden, University of Agriculture Faisalabad, assessed the effect of ZnSO4 on the morphological, physiological and yield attributes of two wheat varieties (W-141 and W-142) grown in sandy loam textured field soils. The toxic concentration of Zn adversely affected the morphological, physiological and yield attributes of wheat. Of the two varieties, W-141 was less sensitive to Zn than W-142. In a similar study, ref. 104 assessed the effects of varying rates of Cd2+ (50 μM) and excess Zn2+ (600 μM) on the photosynthetic contents of hydroponically grown durum wheat seedlings. The results revealed that 7 days after exposure, both Cd and Zn had similar inhibitory effect and reduced the relative growth rate (RGR) and CO2 assimilation rates by 50% and 30%, respectively, in the second developed leaf of the wheat seedlings. Furthermore, both metals disrupted the photosynthetic electron transport processes and caused a four- to five-fold decrease in the energy transformation efficiency of photosystem II.
Chromium is yet another highly toxic metal that hampers the growth and yield of wheat.160 Some of the toxicity symptoms associated with chromium include: (i) chlorosis of leaves, (ii) impaired growth of both roots and shoots, and (iii) wilting.161 Once chromium enters plant tissues, it also disrupts the lamellar system. As an example, ref. 160 reported the inhibition of growth parameters due to alteration in the metabolism of plant cells in response to Cr toxicity. Chromium toxicity reduced the active reaction centres of PSII, rate of electron transport, and change in PSII heterogeneity. However, Cr did not cause any change in the heterogeneity of the reducing side, but a significant change in the antenna size heterogeneity of PSII was observed due to Cr toxicity. Ref. 162, in a study, evaluated the effects of interactions between high temperature and Cr(VI) and Cu on wheat (cv. Dagdas 94) seedlings. High concentrations of Cr and Cu at 40 °C decreased the root and shoot length and dry biomass, while the total chlorophyll content was decreased at 30 μM Cr at 40 °C. In contrast, the higher rates of Cr and Cu increased the carotenoid and proline contents, but decreased the soluble protein relative to the control plants. Among the metals, Cr exhibited greater toxic effects on the growth and biochemical parameters compared to Cu. Similar reduction in the chlorophyll a and b contents of leaves and protein content of two wheat varieties (WH-711, C-306) while growing under different regimes of arsenic (As) has been reported.151 Of the two varieties, WH-711 was found more sensitive to heavy metal than C-306. Cobalt is another metal that had less mobility in the leaf tissues compared to the vascular system, but had greater inhibitory impact on wheat.163 For example, different concentrations of Co (100, 200, 300, 400 and 500 ppm) have shown a variable influence on the wheat performance when grown in a sand culture medium.164 Cobalt at 200 ppm reduced the germination percentage, while the vigour index decreased with increasing Co concentration. However, 300 ppm of Co did not show any negative effect on the germination index, while the seed germination decreased beyond 300 ppm. Interestingly, at Co concentrations up to 200 ppm, Co enhanced the plant height, leaf number, leaf area and dry matter accumulation. Conversely, higher Co concentrations resulted in the destructive effect on the measured parameters. Also, chlorophyll a/b increased while the chlorophyll stability index decreased with Co concentration from 300 ppm onwards. Cobalt was accumulated in both above ground plant biomass and wheat grains. It was concluded from these findings that lower concentrations of Co (up to 200 ppm) had stimulatory effect on the growth of wheat. Hence, it could serve as a good phyto-extracting agent, allowing the wheat to grow under mild Co concentrations. In contrast, nickel adversely affects plant growth at higher concentrations,165 yet it regulates N metabolism at lower concentrations,166,167 and consequently affects germination and other vital physiological plant processes. Similarly, different concentrations (0, 40 and 60 ppm) of Pb had variable adverse effects on the growth and development of two different varieties, i.e., Chakwal-97 and Sehar-2006, of wheat when tested in a pot trial experiment.168 In general, Pb reduced the morphological parameters, such as length (shoot and root), fresh and dry biomass of shoots and number of tillers per plant. The photosynthetic pigments (chl a, chl b) also decreased under Pb stress, while the carotene contents of the wheat plants were considerably increased. Sodium and K contents were also decreased by Pb.
Mercury (Hg) is yet another major toxicant that persists in soils and hence, is a major global problem.169 Mercury mainly remains in the solid phase, and the predominant form of Hg in agricultural soils is the ionic form (Hg2+).170 The interaction of Hg with plant systems is extremely vital since Hg has largely been used as seed disinfectants, in fertilizers and in herbicides.171 Following interaction with plants, Hg has been found to generate ROS (such as superoxide radical, H2O2 and hydroxyl radicals) in plants.169,172 From a toxicity point of view, Hg has the greatest inhibitory effects on seed germination, root elongation, and hypocotyl and coleoptile growth in wheat compared to other heavy metals.73 Due to these properties, ref. 90, in an experiment, investigated the effect of Hg on chlorophyll content in winter wheat var. jinan no. 17. Also, the level of Ca and the bioaccumulation of Hg in wheat leaves were assayed, employing an inductively coupled plasma sector field mass spectrometer (ICP-SF-MS). The results revealed that both low and high Hg concentrations stimulated chlorophyll formation at the early stages, while it inhibited chlorophyll generation at the later stages of wheat growth. Also, the Ca and Hg concentrations in the wheat foliage enhanced with consistently increasing Hg concentration with advancing age of plants, as demonstrated by ICP-SF-MS.
5 Wheat–microbe interactions: impact on growth and yield
The constantly increasing costs of synthetic fertilizers, together with its harmful influence on soil nutrient pool (fertility) and indirectly to human health, is a major threat to wheat cultivation across the globe.173,174 In order to solve such daunting problems, microbial formulations often called “biofertilizers” have provided sound and inexpensive solutions to the toxic chemicals in sustainable wheat production systems.175–177 Indeed, soil microbiota that have been applied once will aggregate in the cultivable habitat, and following colonization, enhances wheat production by different mechanisms.
Wheat, among cereals, is highly sensitive to N and insufficiency of this element results in yellowing (chlorosis) of leaves, which is attributed to poor chlorophyll formation, reduced tillering, disturbance of normal cell growth division, and a reduction in the rate and extent of protein synthesis.178 To obviate such deficiency, numerous microflora have been used in wheat cultivation practices. Chief among them is Azotobacter, which asymbiotically supply approx. 20 kg N/ha per year to wheat. Apart from N, inoculation of free living Azotobacter benefits wheat plants by providing various physiologically active growth hormones, like gibberellin, auxin and cytokinin,179,180 ammonia, vitamins and other substances that influence seed germination,181 protection against root pathogens,182,183 stimulation of beneficial rhizospheric microorganisms and plant yield.184 For example, Azotobacter inoculation has been reported to replace up to 50% of urea-N for wheat grown in a greenhouse trial under aseptic conditions,185 and has been found to increase the plant height, tillers, ear length and grain yield over the non-inoculated control.186 The grain and straw yield of wheat markedly increased from 39.4 q ha−1 to 41.8 q ha−1 and 54.3 q ha−1 to 57.2 q ha−1, respectively, because of seed inoculation with Azotobacter.187Ref. 176 in a pot experiment reported that Azotobacter used either alone or in combination with NPK (nitrogen, phosphorous and potassium) and FYM (farm yard manure) significantly enhanced the root length, biomass of roots, shoots and whole plants, height, panicle weight and grain yield and other biological yields of wheat (var. Gautam). The sole application of Azotobacter increased the grain yield by 16.5–19.42% over the control, while with other fertilizers, the grain yield was augmented between 19–63%. The increase in yield was 23% with NPK alone relative to the control. So, Azotobacter was suggested as a biofertilizer, which could effectively be used to optimize the yield of wheat substantially, along with FYM and NPK. In yet another study, ref. 188 investigated the response of mineral phosphatic fertilizer and phosphate solubilizing bacteria [PSB (Pseudomonas sp. and Klebsiella sp.)] alone or as a mixture on the growth, yield, nutrient uptake and P use efficiency of wheat grown in the field soils treated with varying levels of inorganic P (triple super phosphate: TSP) fertilizer. The maximum grain and straw yield (2.13 and 2.84 t ha−1) were recorded when Pseudomonas sp. was applied with 15 kg P ha−1 and Klebsiella sp. with 15 kg P ha−1. Pseudomonas sp. for Pabna and Klebsiella sp. for Rajshahi, along with TSP, showed better performance than other applications in terms of the yield, nutrient uptake and quality of soil. When used alone, the PSB increased the P use efficiency during crop production. A positive significant correlation was observed between the yield-contributing characters and the grain yield of wheat. From this study, it was suggested that the increase in the nutrient uptake and yield of wheat occurred largely due to the inoculation of PSB, which supplied enough of the available P to the growing wheat plants. In another experiment, ref. 177 observed a significant increment in the growth and nutrient uptake of Pseudomonas inoculated wheat plants. Following Pseudomonas sp. inoculation, the dry biomass of shoots increased significantly over the un-inoculated control. Additionally, the maximum concentration of macronutrients, like N, P, and K, in root and shoot tissues were found in the inoculated wheat plants. The application of the sulfur oxidizing bacterium Thiobacillus thiooxidans in the presence of Tilemsi rock phosphate (TRP) caused an increase in wheat yields. The formulation of RP fertilizers, along with T. thiooxidans AHB411 and T. thiooxidans AHB417, increased the yield upto 33.3% and 11.9%, respectively. Other biological parameters, like the number of tillers per plants, panicle length and seed attributes (such as grains per panicle and 1000 grain weight) were dramatically enhanced. A mixed inoculation of T. thiooxidans and Bio TRP1 increased the grain yield of wheat by 46%, whereas the straw yield was enhanced by 74% relative to the control.189 In a follow up study, the plant growth-promoting rhizobacterial strains Bacillus amyloliquefaciens GB03 (BamGB03), B. megaterium SNji (BmeSNji), and A. brasilense 65B (Abr65B) significantly increased the biomass and N content of wheat plants.190 In addition, elongation in the roots and shoots, and the increased root and shoot dry biomass of wheat plants inoculated with Piscibacillus salipiscarius E5 and Halomonas sp. G11 compared to uninoculated control plants was reported.191 Moreover, a significant increase in the plant height, root length, leaf area, total dry matter, total chlorophyll content, and relative water content of wheat plants was observed following inoculation with Pseudomonas aeruginosa strain 2CpS1.192 Inoculation of PSB, together with RP, optimized the shoot height, shoot and root dry biomass, grain yield and total P uptake of wheat plants relative to other treatments. Also, soil available P, enzyme activities and PSB populations were significantly improved due to the inoculation of PSB with RP compared to DAP (diammonium phosphate) treatment alone. Still, the mixture of PSB and RP was found to be more economical compared to fertilizer application.193 Some examples of how PSB inoculants facilitate wheat production are summarized in Table 1.
Table 1 Inoculation effects of P-solubilizing bacteria on the performance of wheat
PSB inoculants |
Growth promotory effect |
References |
Bacillus strain MWT14 |
Increased growth rate, higher chlorophyll contents, straw yield and grain yield |
194
|
B. polymyxa
|
Enhancement in plant height, number of spikelets per spike, grain yield, grains per spike, 100-grain weight |
195
|
Enterobacter cloacae strain B1 |
Increased plant height, fresh and dry weight, flag leaf area, chlorophyll content, spike length, spikelets number number of grains per spike, 1000 grain weight, spike weight, biological weight |
196
|
Pantoea allii strain BD 390, Stenotrophomonas maltophilia strain IAM 12423, Pseudomonas frederiksbergensis strain DSM 13022 |
Shoot length and dry weight |
197
|
Azotobacter + phosphobacteria |
Plant height, number of tillers per plant, number of spikes per plant, spike length, number of grains per spike, grain yield, straw yield |
198
|
Pseudomonas fluorescens
|
Growth traits and yield |
199
|
B. megaterium BHU1 + Arthrobacter chlorophenolicus BHU3 |
Plant height, grain yield, straw yield and nutrient acquisition |
200
|
Pseudomonas sp. |
Nutrient uptake and seedling growth |
177
|
B. megaterium var. phosphaticum |
No. of kernels per spike, grain yield, grain protein ratio |
201
|
PSB strain MR1 |
Grain and straw yield |
202
|
Apart from a traditional and unstressed environment, plant growth promoting rhizobacteria have been reported to enhance the performance of wheat, while growing under stressed conditions.203 As an example, endophytic strain Bacillus subtilis 10-4, capable of producing IAA and siderophores exhibited a protective effect on wheat plants grown under salinity (2% NaCl) stress. As expected, the exposure to salt stress resulted in a considerable increase in the proline (Pro) and MDA levels in wheat seedlings. However, inoculation of B. subtilis 10-4 decreased the level of stress-induced Pro and MDA contents. Moreover, both B. subtilis 10-4 inoculation and salinity caused an increase in the endogenous salicylic acid (SA) content in wheat seedlings as compared to the SA content of the control, while B. subtilis 10-4 suppressed the stress-induced SA accumulation. The water storage capacity (WSC) in the leaf tissues was increased. In addition, the stress-induced hydrolysis of the statolite starch in the root cap cells of the germinal roots was reduced by B. subtilis 10-4. The data indicated that the activation of the defense reactions induced by B. subtilis 10-4 might be related to their ability to decrease the level of stress-induced oxidative damage and osmotic stress in seedlings. The increased level of endogenous SA might also have played an important protective role against salinity stress, vis-à-vis improving plant growth. Overall, the enhancement in the growth and yield of wheat due to microbial inoculations could be attributed to the expression and signaling of various growth promoting activities: (i) P-solubilization, (ii) N2 fixation, (iii) phytohormone secretion, (iv) secretion of antagonist secondary metabolites (e.g., HCN, siderophores, antifungal antibiotics), and (v) secretion of polymeric substances (EPS). The impact of different active biomolecules synthesized by PGPR on the growth of wheat is depicted in Table 2.
Table 2 Plant growth promoting active biomolecules released by soil microbiota affecting wheat growth
Soil microbiota |
Source |
PGP activities |
References |
Pseudomonas fluorescens, P. putida |
Wheat rhizosphere and rhizoplane |
IAA, siderophore, P solubilization |
204
|
Pantoea sp. |
Wheat seeds |
IAA, siderophore, N2 fixation |
205
|
Burkholderia sp., Enterobacter sp. |
Wheat rhizosphere |
IAA, siderophore |
206
|
P. fluorescens
|
Wheat rhizosphere |
Siderophore, IAA |
207
|
Serratia marcescens, P. aeruginosa |
Vegetables rhizosphere |
IAA production, NH4, HCN production |
208
|
Psychrobacter maritimus, S. proteomaculans, Bacillus anthracis |
Wheat rhizosphere |
IAA, siderophore production |
209
|
S. grimessii, S. marcescens |
Wheat rhizosphere |
N2 fixation, zinc solubilization, EPS activity, ACC deaminase, biocontrol activity, IAA production |
210
|
Stenotrophomonas rhizophila, Acetobactor pasteurianus |
Wheat rhizosphere and endosphere |
N2 fixation, IAA production, Zn and P solubilization |
211
|
Azotobacter sp. |
Rhizospheric soil |
IAA production |
200
|
The toxic impacts of heavy metals on wheat and the subsequent growth improvement following PGPR inoculations through various direct and indirect mechanisms of plant growth promotion have been demonstrated in Fig. 4.
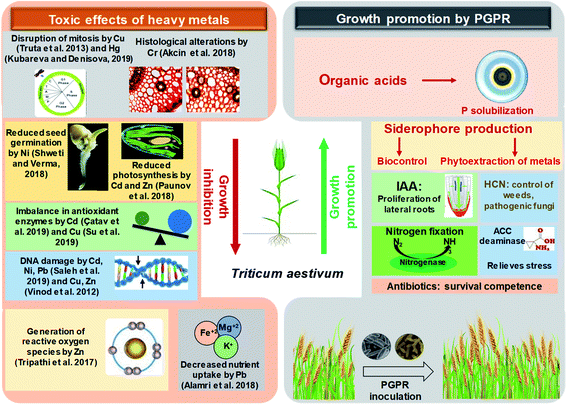 |
| Fig. 4 A schematic representation depicting the toxicity of heavy metals to wheat plants and growth improvement by plant growth promoting rhizobacteria. | |
6 How microbial communities overcome metal stress?
Microbial communities inhabiting various environmental habitats have developed many efficient strategies to clean up the metal contaminated environment.222–224 Approaches adopted by useful soil microflora to combat metal toxicity include: (a) exclusion, (b) active transport of metals away from the cell, (c) intracellular and extracellular sequestration, (d) enzymatic degradation of toxic metals to lesser toxic forms, and (e) reduction in metal sensitivity of cellular targets. One or more simultaneous mechanisms can be adopted at one time by soil microbes to detoxify the contaminated environment. However, the metal clearing approaches might depend on factors, such as type of microorganisms,225 concentration and species of metals, and environmental variables. Many workers have isolated and identified different species of HM-resistant bacteria from various sources, like water and soil.226,227 As an example, the resistance of Bacillus subtilis isolated from different water resources in Taif towards different concentrations (100–1200 g mL−1) and metal species, such as Pb, Cd and Ag, was variable.228 Likewise, ref. 229 revealed that 12 strains of A. chroococcum isolated from wheat, corn and asparagus rhizospheres were sensitive to 50 ppm concentration of Zn, and that the optimum concentration of Zn for the growth of these bacteria was 20 ppm. Accordingly, it was observed that the maximum activity and growth of A. chroococcum strains was achieved when they were grown in the presence of Zn (6–20 ppm). All A. chroococcum strains also carried the nifH gene, an indicator of the nitrogenase enzyme system, and nitrogen fixing capability. Genetic determinants of HMs can be localized both on bacterial chromosomes and on extra-chromosomal genetic elements,230,231 which could provide resistance to toxic HMs. Of these, plasmid-mediated HM resistance determinants have been found to be inducible.232 For example, a Pb-tolerant E. faecalis showed resistance towards many HMs and antibiotics. The metal-tolerant characters were found located on four plasmids of E. faecalis, which were 1.58, 3.06, 22.76 and 28.95 kb in size. Interestingly, the Pb resistance ability of E. faecalis was retained even when all the plasmids were eliminated, as demonstrated by the plasmid profile of the cured bacterial derivatives.233 Similarly, free living nitrogen fixing Gram negative A. chroococcum recovered from contaminated soils exhibited higher resistance to Hg, Cd, Cu, Cr, Co, Ni, Zn and Pb.234 Likewise, the HM resistance characteristics have also been found on bacterial chromosomes.235 For example, Hg2+ resistance in Bacillus, Cd2+ efflux in Bacillus and As efflux in E. coli have been reported.236 Efflux pumps, determined by plasmid and chromosomal systems, are either ATPases or chemiosmotic systems. Both can sometimes function in an identical manner even in different bacterial species. As an example, Cd resistance may involve: (i) an efflux ATPase in Gram-positive bacteria, (ii) cation-H+ antiport in Gram-negative bacteria, and (iii) intracellular metallothionein (MT) in cyanobacteria.237 Likewise, the As-resistant Gram-negative bacteria have an arsenite efflux ATPase and an arsenate reductase.238 Similar systems for Hg2+ resistance were found located on plasmids of Gram-positive and Gram-negative bacteria. Those genes located on plasmids were transcribed to produce the detoxifying enzyme, mercuric reductase, which reduces Hg2+ to elemental Hg0.239 Conclusively, the identification of a metal resistant/tolerant feature might be a useful strategy to develop inexpensive potential bacterial cultures as bioremediation agents for detoxifying the metal contaminated sites.
7 Bioremediation of heavy metal toxicity: a general perspective
In order to make derelict soils cultivable again, various methods such as coagulation, chemical precipitation, electrodialysis, evaporative recovery, floatation, flocculation, ion exchange, nanofiltration, reverse osmosis, and ultrafiltration as well as physico-chemical methods such as extraction, stabilization, immobilization, soil washing of landfills, and excavation have been used. Most of these methods are, however, generally expensive, troublesome for the soil ecosystem, and cannot be used over a large area.240,241 Therefore, to overcome these issues, bioremediation, has emerged in recent times as a magical alternative method to make polluted soils cultivable again. Broadly, bioremediation is “a process used to treat contaminated media, including water, soil and subsurface material, by altering environmental conditions to stimulate the growth of microorganisms and degrade target pollutants”, and has been categorized into two types: (a) in situ bioremediation: the treatment of xenobiotics at origin site and (b) ex situ bioremediation: transportation of contaminated soil from a poisoned site and then treating it.242 Of these, the ex situ approach is expensive, environmentally unsafe and labour-intensive. Due to these issues, the in situ approaches are generally preferred. The commonly employed microbiological metal remediation strategies are summarized in Table 3.
Table 3 Different metal detoxifying strategies adopted by metal tolerant bacteria
Mechanism |
Organism |
Description/effectiveness |
References |
Bioaccumulation |
Delftia sp. B9 |
Intracellular dissolution of Cd, reduction of Cd and accumulation in rice grain |
243
|
Biotransformation and bioaccumulation |
Micrococcus KUMAs 15 |
Arsenite oxidation and accumulation |
244
|
Biosorption |
Bacillus sp. MC3B-22, Microbacterium MC3B-10 |
EPS mediated sorption of Cd2+ |
245
|
Bioreduction |
Bacillus sp. MNU16 |
Reduction of Cr(VI) and growth improvement of plants |
246
|
Bioadsorption |
Pseudomonas aeruginosa PSK1, PSK2, PSK3 and PSK4 |
Removal of Cd from contaminated soil and industrial wastewater |
247
|
8 Microbe-based metal detoxification strategies: current perspectives
Soil microbial populations generally belonging to the metal-tolerant PGPR group have been found to be the most suitable choice for alleviating metal toxicity.248 The metal tolerant bacterial strains evolved to have multiple strategies249 to remediate metal-contaminated soils. Among many bioremediation strategies, about 35% people prefer the use of microbial remediations, whereas only 16% people prefer phytoremediation approach for metal clean-up.250,251 For example, biosorption, extracellular precipitation, conversion of toxic metal ions into less toxic forms and flush out (efflux pumping) of metals to an exterior environment are some of the approaches adopted by bacteria to thrive well even under metal-stressed conditions.252,253 Microbes also enhance the bioavailability of metals from the soil by chelation, acidification, and precipitation. For example, organic acids released by microbes and plant roots lower the soil pH and help in sequestration of metal ions.254
Broadly, the determination of the metal toxicity and detoxification of metals by microbes are indeed the real challenge for the scientists. It is always interesting to see the extent of damage caused by metals and the level of remediation of contaminated environment by microbial communities. To answer these questions, various sensitive techniques have been developed, which very precisely reveal the extent of damage caused by metals to microbes, and also decipher the location and stabilization of metals in various structural components of bacterial cells (Fig. 5). Certain metal detoxification mechanisms adopted by microbes are discussed briefly in the following section.
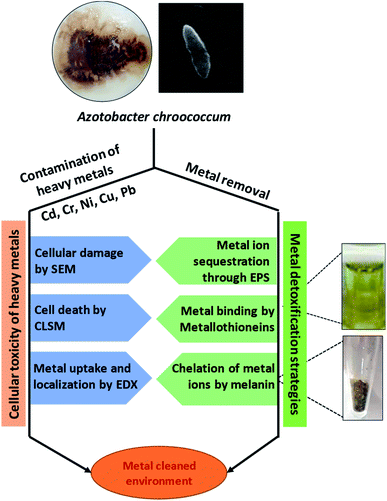 |
| Fig. 5 Examples of most sensitive tools to detect metal toxicity and strategies adopted by soil bacteria to clean up metal contaminated environment [This figure has been adapted and modified from Rizvi et al. (2019)267 with permission from Ecotoxicology, Springer, Copyright 2019]. | |
9 Metal biosorption: biosorbents used in metal clean up
Extracellular polymeric substances (EPSs) are a complex mixture of macro-molecular electrolytes excreted by bacteria that play critical roles in the adsorption of heavy metals.255 For example, Azotobacter,256Bacillus,257Achromobacter258 and Pseudomonas259 have been found to secrete EPS, which allows them to: (i) survive even in the presence of stressor molecules, like heavy metals260 and pesticides261 by masking their toxic impact262,263 and (ii) form complexes with stressor molecules (chelation/sequestration). While doing these functions, the EPS positive strains serve as an important detoxifying agent.264 In this context, as an example, the EPS secretion by the Cd-resistant strain P. aeruginosa265 and A. chroococcum strain XU1 (ref. 266) have been reported.
9.1 Understanding the role of EPS in metal sequestration by SEM, EDX and FTIR
The elemental analysis of EPS, after binding with metal ions and various functional groups involved in metal binding, can be determined using microscopic techniques like SEM (scanning electron microscopy), EDX (energy dispersive X-ray spectroscopy), FTIR (Fourier-transform infrared) spectroscopy and three-dimensional excitation-emission matrix (EEM) fluorescence spectroscopy.268,269 Generally, the adsorption of metal ions by EPS is energy-independent and occurs due to the proteins, polysaccharides and humic substance fractions found in EPS.270 In addition, the shift/disturbances in the wavenumber of a particular spectrum corresponds to the metal binding process taking place either on the bacterial cell surface or the surface of EPS. This could be attributed to the fact that the oxygen of the polysaccharides complexes with metal ions during adsorption in order to reduce the electron cloud density of the functional groups containing oxygen, and to change the vibration frequency and intensity of the participating electrons.271 Due to these properties, the EPS released by many aerobic soil bacteria have been reported to participate in metal removal from polluted environment.245 As an example,272 explained the structural properties and metal biosorption behaviour of a novel EPS secreted by a thermophilic bacterium Anoxybacillus sp. R4-33. This study further revealed the heteropolysaccharide nature of EPS-II, which principally comprised monosaccharide (D-mannose and D-glucose) units present in the ratio of 1
:
0.45. Furthermore, it has been established that EPS secreted by asymbiotic N2 fixers, like Azotobacter and Pseudomonas, also participate in the binding and immobilization of metals.273 After binding, the EPS of Azotobacter creates a microenvironment in the soil, which is composed of metal ions essentially required for regulating the soil ecology. It thereby promotes the normal growth and development of plants.274 In a similar experiment, EPS secreted by Pseudomonas sp. W6 formed a complex with Pb. This relieved the Pb pressure from the contaminated soils, which in turn allowed the crops to grow normally in metal polluted regions.275 Furthermore, SEM coupled with EDX can help to better understand the morphological architecture and composition of bacterial cells besides EPS.276 In this context, ref. 262 reported the tolerance of P. agglomerans towards various metals (for instance, Hg, Cu, Ag, and As), wherein EDX analysis revealed the metal accumulation, while FTIR showed the presence of diverse functional groups influencing the metal attachment. Also, the effect of metals on the surface of bacterial cell employing SEM and TEM showed that heavy metals damaged the cell surface, and accumulated on cell-bound EPS with some intracellular deposition. Thus, this study established that EPS-producing P. agglomerans exhibited the remarkable potential of metal sequestration, and could be used as a potential candidate for heavy metal bioremediation.
9.2 Bacterial biomass
Heavy metal biosorption is an innate and entirely non-enzymatic process found among microorganisms, which they employ to remove metals from contaminated environments by binding/chelating the metal ions even from very dilute solutions.277,278 The biosorption by living or dead microbial biomass involves ion exchange, chelation, adsorption and diffusion through cell walls and membranes. The heavy metal ions are adsorbed on the bacterial surface by an active or passive process,279 both of which may either work independently or in unison. The active process of biosorption is quite slow, and depends mainly on cellular metabolism of the microorganisms involved. It is also affected by various factors like metabolic inhibitors, uncouplers and temperature. In contrast, the passive process does not involve the cellular metabolism of microbes. Instead, metals bind to the cell walls through an ion exchange process. Additionally, the exchange process is not influenced by environmental variables such as pH and ionic strength. However, this process is swift and the complete adsorption of metals occurs within 5–10 min. The passive process of biosorption is also reversible, and involves the biomass of both living and dead microbial cells. However, whatever may be the mode of uptake of metal ions, the adsorption occurs as a result of nonspecific binding of heavy metal ions to the microbial cell surface or extracellular polysaccharides and proteins.264 While comparing both Gram negative and Gram positive bacteria, the cell wall of Gram positive bacteria binds larger quantities of toxic metals in general as compared to the cell envelopes of Gram-negative bacteria.280
Ref. 277, in an experiment, demonstrated the biosorptive ability of live and dead biomass of a novel strain of Bacillus for chromium. Here, both live and dead bacterial biomasses exhibited the monolayer biosorption, where the best fit adsorption isotherm model was the Langmuir isotherm. The results showed that the maximum biosorption potential for Cr was 20.35 mg g−1, which was achieved at 25 °C, pH 3, and the contact time was 50 min. Moreover, SEM and FTIR studies of the metal-loaded bacterial biomass demonstrated the maximum impact of dead bacterial cells on the biosorption of Cr. Also, about 92% and 70% desorption efficiencies were obtained using dead and live cells, respectively. Similarly, in another study, the maximum removal efficiency of Zn(II) by live and dead cell biomasses of V. paradoxus was found to be 92.7 and 91.3%, respectively. In contrast, the live and dead cells of A. viscosus showed a maximum Zn(II) removal efficiency computed as 89.4 and 90.8%, respectively. The results also showed that the biosorption process followed a pseudo-second-order reaction, wherein the best-fit isotherm was the Freundlich isotherm model.281 The FTIR analysis of the bacterial biomass showed that the functional groups present on the bacterial cell surface, such as hydroxyl, amino, carboxylate, and phosphoryl, contributed towards metal-complexing, thereby assisting the process of metal biosorption.282 Therefore, when used as inoculants, such microbes endowed with the property of metal biosorption are likely to circumvent metal toxicity vis-à-vis enhancing the production of agronomically important crops, including cereals grown in soils variously contaminated with heavy metals.
9.3 Metal-induced synthesis of metallothioneins: insights into heavy metal clean up
Metallothioneins (MTs) are a group of low molecular weight (approx. 3500–14
000 Da), cysteine-rich proteins. Chemically, MTs are composed of small polypeptide chains with approximately 30% cysteine residues, which have a strong binding affinity for metals. As a result, MTs sequester various toxic heavy metals by means of thiolate bonds of cysteine groups, and thus make the metal ions unavailable to microbes.283,284 The suggested roles of MTs include: (a) regulation of homeostasis of essential metal ions within cells, (b) chelation of toxic metal ions, and (c) protection of bacterial and plant cells against oxidative damage induced by metal stress.285–287 The synthesis of MTs by soil microbiota under stressed environment has been reported. For example, the induction of MTs in Bacillus cereus cells while growing in the presence of varying concentrations of Pb288 and P. aeruginosa and P. putida cells upon exposure to Cu and Cd has been reported.283 It has therefore been argued that MTs synthesized by bacterial communities under a metal-stressed environment could be valuable in the efficient detoxification of metals.289 Hence, the bacteria possessing this property of secreting MTs could be explored as a cost-effective approach in bioremediation strategies, which could eventually be employed in the growth enhancement of cereal crops growing in metal-contaminated soils.
9.4 Melanin: importance in metal detoxification
Melanin, the name derived from melons (Greek dark), is a dark brown to black coloured indolic polymer and amorphous substance that is usually attributed to the Swedish chemist Berzelius in 1840. Melanins are synthesized by many prokaryotic organisms, including nitrogen-fixing organisms.290 Based on the colour and structural classes, there are primarily three types of melanin: (i) eumelanins: black to brown colour pigments produced by melanization by classic Mason–Rapper pathway, which produce tyrosine intermediates or metabolites by the action of tyrosinases, (ii) pheomelanins: brown, red or yellow colour pigments, which are produced due to the oxidation of tyrosine and/or phenylalanine to dihydroxyphenylalanine (DOPA) and dopaquinone. Pheomelanin results from cysteinylation of DOPA and these are sulphur containing compounds, and (iii) allomelanins: include nitrogen free heterogeneous group of polymers formed from catechol precursors.
The production of melanin by some bacterial strains, for example A. chroococcum,291 and its ability to chelate metals is yet other mechanism by which the toxicity of metals can be reduced.292–294 Melanin also protects organisms from UV radiation, chemical stresses and high temperatures,295 thermoregulation and camouflage.296 Melanin is an amorphous substance with no definite structure. The structure and property of metal sequestration by melanin could be confirmed by SEM and EDX analysis, respectively. In an experiment, ref. 297 confirmed similar features of melanin extracted from Pseudomonas sp. The adsorption of toxic hexavalent Cr onto melanin pigment isolated from the ink sac of squids was confirmed by SEM and EDX.298 Furthermore, FT-IR spectroscopy of melanin extracted from Bacillus weihenstephanensis displayed a broad peak in the range of 3268–3278 cm−1, which is attributed to –OH stretching. The absorbance peaks detected in the region 1511–1729 cm−1, 2926–2970 cm−1, 1045 cm−1 and 1220 cm−1 indicated the bonding vibration of the C
C and C
O aromatic ring stretching, the presence of double bonds in the COOH group, and the presence of saturated carbon and stretching vibrations in the carbonyl, alcoholic and phenolic groups, respectively.299 Due to these features, bacterial melanin could serve as an excellent metal detoxification mechanism adopted by metal-tolerant microbes. Such melanin synthesizing bacteria, when applied to derelict soils as bioinoculants, could substantially improve the growth of plants, including cereal crops like wheat and maize growing in soils polluted or deliberately treated with toxic heavy metals by sequestrating metal ions and rendering them unavailable for uptake by plants. Heavy metal detoxification by soil bacteria employing various mechanisms has been summarized in Fig. 6.
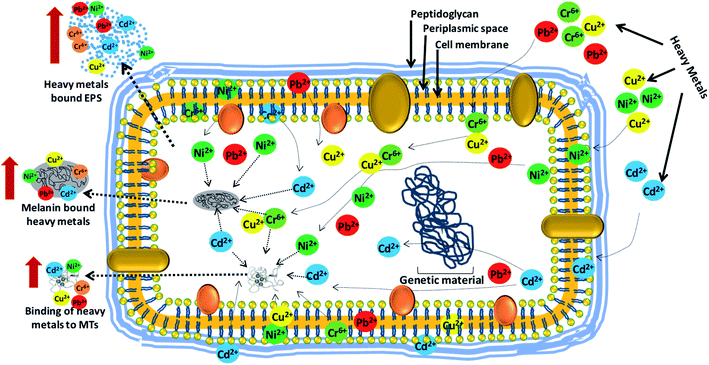 |
| Fig. 6 Interaction of bacterial cells with heavy metal ions and their removal/detoxification by active biomolecules (EPS, MTs and melanin) secreted by bacterial strains when exposed to metal stress [This figure has been adapted and modified from Rizvi et al. (2019)267 with permission from Ecotoxicology, Springer, Copyright 2019]. | |
10 PGPR assisted growth improvement of wheat plants under metal stress
The viability, colonizing efficiency and functionality of metal-tolerant PGPR determines the success of metal removal microbiological strategies used to alleviate contamination, and consequently to enhance the production of wheat growing in polluted soils. Plant growth-promoting rhizobacteria, apart from their normal growth promoting traits, facilitates plant growth by mitigating the toxic impact of metals.300,301 Considering these features, the effects of some of the metal-tolerant PGPR on the overall growth and yield of wheat plants grown in metal polluted soils are discussed briefly in the following section.
In an experiment, an improvement in the germination rate, root and shoot length and other growth parameters of wheat plants grown in Cd-treated soils was recorded following inoculation with Pseudomonas strains SNA5 and PBB1.302 Similarly, the growth of roots (208%), shoots (67%) and root (140%) and shoot (71%) dry biomass of wheat plants grown under Cr stress following inoculation with ACC deaminase positive and P-solubilizing Pseudomonas fluorescens Q14 and Bacillus thuringiensis strain KAP5 has been reported.303 Likewise, ref. 304 found a substantial increase in leaf photosynthetic pigments and other vital growth parameters of P. aeruginosa inoculated wheat plants grown under the influence of various doses of Zn. Furthermore, lowered MDA levels and antioxidant enzyme activity were observed following inoculation with P. aeruginosa under Zn stress. Similarly, the grain yield and membrane integrity of various wheat genotypes were found to be enhanced following inoculation with Azotobacter and Azospirillum strains even under Pb stress. Conversely, such bioinoculants significantly declined the production of MDA, proline and H2O2 under the influence of heavy metal stress.305 The stress alleviation and a simultaneous growth promotion of wheat plants by IAA-synthesizing Bacillus sp. strain USTB-O grown under Cu stress was also reported.306 The beneficial bacterium also improved the antioxidant defense mechanism in metal-stressed wheat plants. In this context, a decline in the levels of proline and other stresses within A. chroococcum and other PGPR-inoculated wheat plants grown under metal stress has been reported.307,308 Moreover, a decline in oxidative stress in PGPR inoculated wheat plants was recorded. Also, Bacillus subtilis SU47 and Arthrobacter sp. SU18 has been found to minimize the accumulation of antioxidant enzymes in wheat plants by alleviating the stress.309 In another study, the dry biomass of wheat plants was increased and a reduction in oxidative stress was recorded following inoculation with Pseudomonas gessardii and Brevundimonas intermedia when wheat was grown in As-polluted soil.310 Also, a similar reduction in antioxidant enzyme activity was recorded in wheat plants inoculated with Planomicrobium chinense and Bacillus cereus under stressed conditions.311
11 Conclusion
Despite the toxicity conferred by heavy metals onto various parameters of plants in general, the introduction of metal-tolerant PGPR in polluted soils greatly diminishes the ruinous effects of metals, and enhances the growth and yield of wheat. The metal-tolerant bacteria, through biosorptive ability and capability to secrete EPS, can remove significant amounts of metal ions from the contaminated environment. The release of EPS by metal-tolerant strains also protects plants from other challenges, like pathogen attacks and desiccation. This consequently allows them to survive, and perform normal physiological and biochemical activities in stressed environments. Moreover, the secretion of MTs and melanin by viable cells under the influence of heavy metals could be considered another vital strategy evolved within bacterial strains to mitigate metal toxicity. Overall, the microbial management strategy to detoxify/remediate the contaminated environment through biosorption, secretion of MTs, melanin and EPS secretion makes metal-tolerant bacterial strains a promising and most suitable choice for heavy metal clean up from contaminated soils. The novel and fascinating traits of metal-tolerant bacteria could serve as an inexpensive yet environmentally viable approach in the metal clean-up program vis-a-vis, the growth and yield enhancement of wheat growing in metal-enriched soils.
Conflicts of interest
The authors declare no competing interests.
Acknowledgements
This research was funded by the Deanship of Scientific Research at Princess Nourah Bint Abdulrahman University through the Fast-track Research Funding Program. One of the authors, Asfa Rizvi, is also thankful to Department of Science and Technology, New Delhi, India for providing financial assistance through the INSPIRE fellowship.
References
- T. Kamitani, H. Oba and N. Kaneko, Microbial biomass and tolerance of microbial community on an aged heavy metal polluted floodplain in Japan, Water, Air, Soil Pollut., 2006, 172, 185–200 CrossRef.
-
P. B. Tchounwou, C. G. Yedjou, A. K. Patlolla and D. J. Sutton, Heavy metal toxicity and the environment, in Molecular, clinical and environmental toxicology, Springer, Basel, 2012, pp. 133–164 Search PubMed.
- M. Algreen, A. Rein, C. N. Legind, C. E. Amundsen and U. G. Karlson, Test of tree core sampling for screening of toxic elements in soils from a Norwegian site, Int. J. Phytoremediation, 2012, 14, 305–319 CrossRef CAS PubMed.
-
A. Kabata-Pendias and H. Pendias, Trace elements in plants, in Trace elements in soils and plants, 2001, pp. 83–34 Search PubMed.
-
G. M. Pierzynski, J. T. Sims and G. Vance, Soils and environmental quality, CRC Press LLC, Boca Raton, FL, 2000 Search PubMed.
-
P. K. Gautam, R. K. Gautam, S. Banerjee, M. C. Chattopadhyaya and J. D. Pandey, Heavy metals in the environment: fate, transport, toxicity and remediation technologies, in Heavy Metals, ed. D. Pathania, Nova Science Publishers, Inc., 2016, ISBN: 978-1-63484-740-7 Search PubMed.
- V. Ettler, Soil contamination near non-ferrous metal smelters: a review, Appl. Geochem., 2016, 64, 56–74 CrossRef CAS.
- P. S. DeVolder, S. L. Brown, D. Hesterberg and K. Pandya, Metal bioavailability and speciation in a wetland tailings repository amended with biosolids compost, wood ash, and sulfate, J. Environ. Qual., 2003, 32, 851 CrossRef CAS PubMed.
- M. Munir, Z. I. Khan, K. Ahmad, K. Wajid, H. Bashir, I. S. Malik, M. Nadeem, A. Ashfaq and I. Ugulu, Transfer of heavy metals from different sources of fertilizers in wheat variety (Galaxy-13), Asian J. Biol. Sci., 2019, 12, 832–841 CrossRef CAS.
-
P. H. Raven, L. R. Berg and G. B. Johnson, Environment, Saunders College Publishing, New York, NY, USA, 2nd edn, 1998 Search PubMed.
- M. S. Khan, A. Zaidi, M. Ahemad, M. Oves and P. A. Wani, Plant growth promotion by phosphate solubilizing fungi-current perspective, Arch. Agron. Soil Sci., 2010, 56, 73–98 CrossRef CAS.
- S. R. Smith, A critical review of the bioavailability and impacts of heavy metals in municipal solid waste composts compared to sewage sludge, Environ. Int., 2009, 35, 142–156 CrossRef CAS PubMed.
-
L. H. P. Jones and S. C. Jarvis, The fate of heavy metals, in The chemistry of soil process, 1981, pp. 593–620 Search PubMed.
- E. Kabir, S. Ray, K. H. Kim, H. O. Yoon and E. C. Jeon, Current status of trace metal pollution in soils affected by industrial activities, Sci. World J., 2012, 916705, DOI:10.1100/2012/916705.
- M. K. Zhang, Z. Y. Liu and H. Wang, Use of single extraction methods to predict bioavailability of heavy metals in polluted soils to rice, Commun. Soil Sci. Plant Anal., 2010, 41, 820–831 CrossRef CAS.
- S. Khan, Q. Cao, Y. M. Zheng, Y. Z. Huang and Y. G. Zhu, Health risks of heavy metals in contaminated soils and food crops irrigated with wastewater in Beijing, China, Environ. Pollut., 2008, 152, 686–692 CrossRef CAS PubMed.
- P. C. Ogbonna and N. Okezie, Heavy metal level and macronutrient contents roadside soil and vegetation in Umuahia, Nigeria, Terr. Aquat. Environ. Toxicol., 2011, 5, 35–39 Search PubMed.
- K. Suzuki, T. Yabuki and Y. Ono, Roadside Rhododendron pulchrum leaves as bioindicators of heavy metal pollution in traffic areas of Okayama, Japan, Environ. Monit. Assess., 2009, 149, 133–141 CrossRef CAS PubMed.
- I. Fernandez-Olmo, C. Lasa and A. Irabien, Modeling of zinc solubility in stabilized/solidified electric arc furnace dust, J. Hazard. Mater., 2007, 144, 720–724 CrossRef CAS PubMed.
- K. Iijima, T. Otake, J. Yoshinaga, M. Ikegami and E. Suzuki, Cadmium, lead, and selenium in cord blood and thyroid hormone status of newborns, Biol. Trace Elem. Res., 2007, 119, 10–18 CrossRef CAS PubMed.
- K. Adachi and Y. Tainosho, Characterization of heavy metal particles embedded in tire dust, Environ. Int., 2004, 30, 1009–1017 CrossRef CAS PubMed.
- R. Ochoa-Gonzalez, A. F. Cuesta, P. Córdoba, M. Díaz-Somoano and O. Font, Study of boron behaviour in two Spanish coal combustion power plants, J. Environ. Manage., 2011, 92, 2586–2589 CrossRef CAS PubMed.
- X. Tang, C. Shen, D. Shi, S. A. Cheema and M. I. Khan, Heavy metal and persistent organic compound contamination in soil from Wenling: an emerging e-waste recycling city in Taizhou area, China, J. Hazard. Mater., 2010, 173, 653–660 CrossRef CAS PubMed.
- M. Kaasalainen and M. Yli-Halla, Use of sequential extraction to assess metal partitioning in soils, Environ. Pollut., 2003, 126, 225–233 CrossRef CAS PubMed.
- S. Kuo, P. E. Heilman and A. S. Baker, Distribution and forms of copper, zinc, cadmium, iron, and manganese in soils near a copper smelter, Soil Sci., 1983, 135, 101–109 CrossRef CAS.
-
M. Alamgir, The effects of soil properties to the extent of soil contamination with metals, in Environmental remediation technologies for metal-contaminated soils, ed. Hasegawa Hiroshi,M. M. Rahman Ismail and M. Rahman Azizur, Springer, Tokyo, 2016, pp. 1–19 Search PubMed.
- D. K. Patel, G. Archana and G. N. Kumar, Variation in the nature of organic acid secretion and mineral phosphate solubilization by Citrobacter sp. DHRSS in the presence of different sugars, Curr. Microbiol., 2008, 56, 168–174 CrossRef CAS PubMed.
-
T. J. Beveridge, S. Schultze-Lam and J. B. Thompson, Detection of anionic sites on bacterial walls, their ability to bind toxic heavy metals and form sedimentable flocs and their contribution to mineralization in natural freshwater environments, Metal Speciation and Contamination of Soil, Lewis Publishers, 1995, pp. 183–200 Search PubMed.
- Q. Y. Huang, J. Wu and W. Chen, Adsorption of Cd on soil colloids and minerals in presence of rhizobia, Pedosphere, 2000, 10, 299–307 CAS.
- E. M. Cukrowska, K. Govender and M. Viljoen, Ion mobility based on column leaching of South African gold tailings dam with chemometric evaluation, Chemosphere, 2004, 56, 39–50 CrossRef CAS PubMed.
- A. Voegelin, K. Barmettler and R. Kretzschmar, Heavy metal release from contaminated soils: comparison of column leaching and batch extraction results, J. Environ. Qual., 2003, 32, 865–875 CAS.
- J. J. Zhang, T. Y. Liu, W. F. Chen, E. T. Wang and X. H. Sui,
Mesorhizobium muleiense sp. nov., nodulating with Cicer arietinum L, Int. J. Syst. Evol. Microbiol., 2012, 62, 2737–2742 CrossRef CAS PubMed.
- X. H. Huang, Z. B. Wei, X. F. Guo, X. F. Shi and Q. T. Wu, Metal removal from contaminated soil by co-planting phytoextraction and soil washing, Huanjing Kexue, 2010, 31, 3067–3074 CAS.
- S. P. McGrath and J. Cegarra, Chemical extractability of heavy metals during and after long-term applications of sewage sludge to soil, J. Soil Sci., 1992, 43, 313321 Search PubMed.
- R. Khlifi and A. Hamza-Chaffai, Head and neck cancer due to heavy metal exposure via tobacco smoking and professional exposure: a review, Toxicol. Appl. Pharmacol., 2010, 248, 71–88 CrossRef CAS PubMed.
- Y. Chen, J. Xu, M. Yu, X. Chen and J. Shi, Lead contamination in different varieties of tea plant (Camellia sinensis L.) and factors affecting lead bioavailability, J. Sci. Food Agric., 2010, 90, 1501–1507 CrossRef CAS PubMed.
- E. I. Chopin, B. Marin, R. Mkoungafoko, A. Rigaux and M. J. Hopgood, Factors affecting distribution and mobility of trace elements (Cu, Pb, Zn) in a perennial grapevine (Vitis vinifera L.) in the Champagne region of France, Environ. Pollut., 2008, 156, 1092–1098 CrossRef CAS PubMed.
- J. J. Liu, Z. Wei and J. H. Li, Effects of copper on leaf membrane structure and root activity of maize seedling, Bot. Stud., 2014, 55, 47, DOI:10.1186/s40529-014-0047-5.
- Q. H. Wang, X. Liang, Y. J. Dong, L. L. Xu, X. W. Zhang, J. Hou and Z. Y. Fan, Effects of exogenous nitric oxide on cadmium toxicity, element contents and antioxidative system in perennial ryegrass, Plant Growth Regul., 2013, 69, 11–20 CrossRef CAS.
- L. S. Macedo and W. B. B. Morril, Origem e Comportamento dos Metais Fitotoxicos: Revisao da Literatura, Tecnologia & Ciência Agropecuária, 2008, 2, 29–38 Search PubMed.
- A. Karaca, Effect of organic wastes on the extractability of cadmium, copper, nickel, and zinc in soil, Geoderma, 2004, 122, 297–303 CrossRef CAS.
- G. Guo, M. Lei, Y. Wang, B. Song and J. Yang, Accumulation of As, Cd, and Pb in sixteen wheat cultivars grown in contaminated soils and associated health risk assessment, Int. J. Environ. Res. Publ. Health, 2018, 15, 2601 CrossRef CAS PubMed.
- L. M. Sandalio, H. C. Dalurzo, M. Gomez, M. C. Romero-Puertas and L. A. del Rio, Cadmium-induced changes in the growth and oxidative metabolism of pea plants, J. Exp. Bot., 2001, 52, 2115–2126 CrossRef CAS PubMed.
- P. K. Rai, S. S. Lee, M. Zhang, Y. F. Tsang and K. H. Kim, Heavy metals in food crops: health risks, fate, mechanisms and management, Environ. Int., 2019, 125, 365–385 CrossRef CAS PubMed.
- S. Singh, P. Parihar, R. Singh, V. P. Singh and S. M. Prasad, Heavy metal tolerance in plants: role of transcriptomics, proteomics, metabolomics, and ionomics, Front. Plant Sci., 2015, 6, 1143, DOI:10.3389/fpls.2015.01143.
- M. F. López-Climent, V. Arbona, R. M. Pérez-Clemente and A. Gómez-Cadenas, Effects of cadmium on gas exchange and phytohormone contents in citrus, Biol. Plant., 2011, 55, 187–190 CrossRef.
- A. Rizvi, B. Ahmed, A. Zaidi and M. S. Khan, Heavy metal mediated phytotoxic impact on winter wheat: oxidative stress and microbial management of toxicity by Bacillus subtilis BM2, RSC Adv., 2019, 9, 6125–6142 RSC.
-
O. Pintilie, M. Zaharia, A. Cosma, A. Butnaru, M. Murariu, G. Drochioiu and I. Sandu, Effect of heavy metals on the germination of wheat seeds: Enzymatic Assay, The Annals of “Dunarea De Jos”, University of Galati Fascicle Ix, Metallurgy Mater Sci 1, 2016, ISSN 1453-083X Search PubMed.
- T. Mahmood, K. J. Gupta and W. M. Kaiser, Cd stress stimulates nitric oxide production by wheat roots, Pak. J. Bot., 2009, 41, 1285–1290 CAS.
- J. K. Mohammad, T. Muhammad and K. Khalid, Effect of organic and inorganic amendments on the heavy metal content of soil and wheat crop irrigated with wastewater, Sarhad J. Agric., 2013, 29, 145–152 Search PubMed.
- D. Pizzeghello, O. Francioso, A. Ertani, A. Muscolo and S. Nardi, Isopentenyl adenosine and cytokinin-like activity of different humic substances, J. Geochem. Explor., 2013, 129, 70–75 CrossRef CAS.
- G. M. Gadd, Geomycology: biogeochemical transformations of rocks, minerals, metals and radionuclides by fungi, bioweathering and bioremediation, Mycol. Res., 2007, 111, 3–49 CrossRef CAS PubMed.
- A. Alemzadeh, L. Rastgoo, A. Tale, S. Tazangi and T. Eslamzadeh, Effects of copper, nickel and zinc on biochemical parameters and metal accumulation in gouan, Aeluropus littoralis, Plant Knowledge J, 2014, 3, 31–38 Search PubMed.
- M. Adrees, S. Ali, M. Rizwan, M. Ibrahim, F. Abbas, M. Farid, M. Zia-Ur-Rehman, M. K. Irshad and S. A. Bharwana, The effect of excess copper on growth and physiology of important food crops: a review, Environ. Sci. Pollut. Res., 2015, 22, 8148–8162 CrossRef CAS PubMed.
- M. E. Pérez-Pérez, S. D. Lemaire and J. L. Crespo, Reactive oxygen species and autophagy in plants and algae, Plant Physiol., 2012, 160, 156–164 CrossRef PubMed.
- S. Gholamabbas, A. Majid, M. Sayed-Farhad, A. C. Karim, R. K. Brian and S. Rainer, Transport of Cd, Cu, Pb and Zn in a calcareous
soil under wheat and safflower cultivation- A column study, Geoderma, 2010, 154, 311–320 CrossRef.
- S. Bose and A. K. Bhattacharyya, Heavy metal accumulation in wheat plant grown in soil amended with industrial sludge, Chemosphere, 2008, 70, 1264–1272 CrossRef CAS PubMed.
- J. Y. He, Y. F. Ren, C. Zhu and D. A. Jiang, Effects of cadmium stress on seed germination, seedling growth and seed amylase activities in rice (Oryza sativa), Rice Sci., 2008, 15, 319–325 CrossRef.
- J. R. Peralta, G. Torresday, J. L. Tiemann, K. J. E. Gomez, S. Arteaga and E. Rascon, Uptake and effects of five heavy metals on seed germination and plant growth in alfalfa (Medicago sativa L.), Bull. Environ. Contam. Toxicol., 2001, 66, 727–734 CAS.
- S. A. Bhalerao and A. S. Sharma, Toxicity of nickel in plants, Indian J. Pure Appl. Biosci., 2015, 3, 345–355 Search PubMed.
- S. Sethy and S. Ghosh, Effect of heavy metals on germination of seeds, J. Nat. Sc. Biol. Med., 2013, 4, 272–275 CrossRef PubMed.
- W. Li, M. A. Khan, S. Yamaguchi and Y. Kamiya, Effects of heavy metals on seed germination and early seedling growth of Arabidopsis thaliana, Plant Growth Regul., 2005, 46, 45–50 CrossRef CAS.
- J. K. Datta, A. Bandhyopadhyay, A. Banerjee and N. K. Mondal, Phytotoxic effect of chromium on the germination, seedling growth of some wheat (Triticum aestivum L.) cultivars under laboratory condition, Journal of Agricultural Technology, 2011, 7, 395–402 Search PubMed.
- A. Lareen, F. Burton and P. Schäfer, Plant root-microbe communication in shaping root microbiomes, Plant Mol. Biol., 2016, 90, 575–587 CrossRef CAS PubMed.
- F. Rees, T. Sterckeman and J. L. Morel, Root development of non-accumulating and hyperaccumulating plants in metal-contaminated soils amended with biochar, Chemosphere, 2016, 142, 48–55 CrossRef CAS PubMed.
-
N. Lal, Molecular mechanisms and genetic basis of heavy metal toxicity and tolerance in plants, in Plant Adaptation and Phytoremediation, 2010, pp. 35–58 Search PubMed.
- Z. A. Al-Othman, R. Ali, A. M. Al-Othman, J. Ali and M. A. Habila, Assessment of toxic metals in wheat crops grown on selected soils, irrigated by different water sources, Arab. J. Chem., 2016, 9, S1555–S1562 CrossRef CAS.
- M. F. S. Guilherme, H. M. de Oliveira and E. da Silva, Cadmium toxicity on seed germination and seedling growth of wheat Triticum aestivum, Acta Sci., Biol. Sci., 2015, 37, 499–504 CrossRef CAS.
- S. Samardakiewicz and A. Wozny, Cell division in Lemna minor roots treated with lead, Aquat. Bot., 2005, 83, 289–295 CrossRef CAS.
- S. O. Eun, H. S. Youn and Y. Lee, Lead disturbs microtubule organization in the root meristem of Zea mays, Physiol. Plant., 2000, 103, 665–702 Search PubMed.
- G. Kaur, H. P. Singh, D. R. Batish and R. K. Kohli, Growth, photosynthetic activity and oxidative stress in wheat (Triticum aestivum) after exposure of lead Pb to soil, J. Environ. Biol., 2012, 33, 265–269 CAS.
- S. K. Dey, J. Dey, S. Patra and D. Pothal, Changes in the antioxidative enzyme activities and lipid peroxidation in wheat seedlings exposed to cadmium and lead stress, Braz. J. Plant Physiol., 2007, 19, 53–60 CrossRef CAS.
- O. Munzuroglu and H. Geckil, Effects of metals on seed germination, root elongation, and coleoptile and hypocotyl growth in Triticum aestivum and Cucumis sativus, Arch. Environ. Contam. Toxicol., 2002, 43, 203–213 CrossRef CAS PubMed.
- G. Kaur, H. P. Singh, D. R. Batish and R. K. Kohli, Lead (Pb)-induced biochemical and ultrastructural changes in wheat (Triticum aestivum) roots, Protoplasma, 2013, 250, 53–62 CrossRef CAS PubMed.
- J. L. Yang, Y. Y. Li, Y. J. Zhang, S. S. Zhang, Y. R. Wu, P. Wu and S. J. Zheng, Cell wall polysaccharides are specifically involved in the exclusion of aluminum from the rice root apex, Plant Physiol., 2008, 146, 602–611 CrossRef CAS PubMed.
- W. J. Horst, Y. X. Wang and D. Eticha, The role of the root apoplast in aluminium-induced inhibition of root elongation and in aluminum resistance of plants: a review, Ann. Bot., 2010, 106, 185–197 CrossRef CAS PubMed.
- M. Čiamporová, Diverse responses of root cell structure to aluminium stress, Plant Soil, 2000, 226, 113–116 CrossRef.
- P. M. Kopittke, F. P. C. Blamey and N. W. Menzies, Toxicities of soluble Al, Cu, and La include ruptures to rhizodermal and root cortical cells of cowpea, Plant Soil, 2008, 303, 217–227 CrossRef CAS.
- J. D. G. Jones and J. L. Dang, The plant immune system, Nature, 2006, 444, 323–329 CrossRef CAS PubMed.
- M. A. Llamas, M. J. Mooij, M. Sparrius, M. J. E. Christina, Vandenbroucke-rauls and C. Ratledge, Characterization of five novel Pseudomonas aeruginosa cell-surface signalling systems, Mol. Microbiol., 2008, 67, 458–472 CrossRef CAS PubMed.
- M. A. Hossain, P. Piyatida, J. A. T. da Silva and M. Fujita, Molecular mechanism of heavy metal toxicity and tolerance in plants: central role of glutathione in detoxification of reactive oxygen species and methylglyoxal and in heavy metal chelation, J. Bot., 2012 DOI:10.1155/2012/872875.
- K. Kabala, M. Janicka-Russak, M. Burzynski and G. Klobus, Comparison of heavy metal effect on the proton pumps of plasma membrane and tonoplast in cucumber root cells, Plant Physiol., 2008, 165, 278–288 CrossRef CAS PubMed.
- A. Zaidi, D. Fernandes, J. L. Bean and M. L. Michaelis, Effects of paraquat induced oxidative stress on the neuronal plasma membrane Ca (2+)-ATPase, Free Radic. Biol. Med., 2009a, 47, 1507–1514 Search PubMed.
- P. Zaccheo, M. Cocucci and S. Cocucci, Effects of Cr on proton extrusion, potassium uptake and transmembrane electric potential in maize root segments, Plant Cell Environ., 1985, 8, 721–726 CAS.
- P. A. Wani, M. S. Khan and A. Zaidi, Chromium reduction, plant growth-promoting potentials, and metal solubilizatrion by Bacillus sp. isolated from alluvial soil, Curr. Microbiol., 2007, 54, 237–243 CrossRef CAS PubMed.
- G. Quievryn, E. Peterson, J. Messer and A. Zhitkovich, Genotoxicity and mutagenicity of chromium (VI)/ascorbate-generated DNA adducts in human and bacterial cells, Biochem, 2003, 42, 1062–1070 CrossRef CAS PubMed.
- M. Janicka-Russak, K. Kabała and M. Burzyński, Different effect of cadmium and copper on H+-ATPase activity in plasma membrane vesicles from Cucumis sativus roots, J. Exp. Bot., 2012, 63, 4133–4142 CrossRef CAS PubMed.
- C. N. Meisrimler, S. Planchon, J. Renaut, K. Sergeant and S. Luthje, Alteration of plasma membrane-bound redox systems of iron deficient pea roots by chitosan, J. Proteom., 2011, 74, 1437–1449 CrossRef CAS PubMed.
- S. A. Nasim and B. Dhir, Heavy metals alter the potency of medicinal plants, Rev. Environ. Contam. Toxicol., 2010, 203, 139–149 CAS.
- D. X. Liu, Z. Wang, L. Chen, H. Xu and Y. Wang, Influence of mercury on chlorophyll content in winter wheat and mercury bioaccumulation, Plant Soil Environ., 2010, 56, 139–143 CrossRef CAS.
- A. M. Reddy, S. G. Kumar, G. Jyothsnakumari, S. Thimmanaik and C. Sudhakar, Lead induced changes in antioxidant metabolism of horsegram (Macrotyloma uniflorum (Lam.) Verdc.) and bengalgram (Cicer arietinum L.), Chemosphere, 2005, 60, 97–104 CrossRef CAS PubMed.
- S. S. Sharma and K. J. Dietz, The relationship between metal toxicity and cellular redox imbalance, Trends Plant Sci., 2009, 14, 43–50 CrossRef CAS PubMed.
- Y. Yamamoto, Y. Kobayashi and H. Matsumoto, Lipid peroxidation is an early symptom triggered by aluminum, but not the primary cause of elongation inhibition in pea roots, Plant Physiol., 2001, 125, 199–208 CrossRef CAS PubMed.
- S. A. Anjum, X. Xie, L. Wang, M. F. Saleem, C. Man and W. Lei, Morphological, physiological and biochemical responses of plants to drought stress, Afr. J. Agric. Res., 2011, 6, 2026–2032 Search PubMed.
- X. Song, Y. Wang and X. Lv, Responses of plant biomass, photosynthesis and lipid peroxidation to warming and precipitation change in two dominant species (Stipa grandis and Leymus chinensis) from North China Grasslands, Ecol. Evol., 2016, 6, 1871–1882 CrossRef PubMed.
-
M. Labudda, Lipid peroxidation as a biochemical marker for oxidative stress during drought: an effective tool for plant breeding, E-wydawnictwo, Poland, 2013, http://www.e-wydawnictwo.eu/document/documentpreview/3342 Search PubMed.
- P. Sharma and R. Dubey, Lead toxicity in plants, Braz. J. Plant Physiol., 2005, 17, 35–52 CrossRef CAS.
- R. I. Shewfelt and M. C. Erickson, Role of lipid peroxidation in the mechanism of membrane associated disorders in edible plant tissue, Trends Food Sci. Technol., 1991, 2, 152–154 CrossRef CAS.
- G. Witz, N. J. Lawrie, A. Zaccaria, H. E. Ferran, Jr and B. D. Goldstein, The reaction of 2-thiobarbituric acid with biologically active alpha, beta-unsaturated aldehydes, Free Radicals Biol. Med., 1986, 2, 33–39 CAS.
- O. A. Rozentsvet, V. N. Nesterov and N. F. Sinyutina, The effect of copper ions on the lipid composition of subcellular membranes in Hydrilla verticillata, Chemosphere, 2012, 89, 108–113 CrossRef CAS PubMed.
- S. Panda and U. C. Biswal, Effect of magnesium and calcium ions on photoinduced lipid peroxidation and thylakoid breakdown of cell-free chloroplasts, Indian J. Biochem. Biophys., 1990, 27, 159–163 CAS.
- Y. Yamamoto, Y. Kobayashi, S. R. Devi, S. Rikiishi and H. Matsumoto, Aluminum toxicity is associated with mitochondrial dysfunction and the production of reactive oxygen species in plant cells, Plant Physiol., 2002, 128, 63–72 CrossRef CAS PubMed.
- K. M. Janas, J. Zielinska-Tomaszewska, D. Rybaczek, J. Maszewski and M. M. Posmyk, The impact of copper ions on growth, lipid peroxidation, and phenolic compound accumulation and localization in lentil (Lens culinaris Medic.) seedlings, J. Plant Physiol., 2010, 167, 270–276 CrossRef CAS PubMed.
- M. Paunov, L. Koleva, A. Vassilev, J. Vangronsveld and V. Goltsev, Effects of different metals on photosynthesis: cadmium and zinc affect chlorophyll fluorescence in durum wheat, Int. J. Mol. Sci., 2018, 19, 787, DOI:10.3390/ijms19030787.
- S. S. Gill, N. A. Khan and N. Tuteja, Cadmium at high dose perturbs growth, photosynthesis and nitrogen metabolism while at low dose it up regulates sulfur assimilation and antioxidant machinery in garden cress (Lepidium sativum L.), Plant. Sci., 2011, 182, 112–120 CrossRef PubMed.
- E. Romanowska, W. Wasilewska, R. Fristedt, A. V. Vener and M. Zienkiewicz, Phosphorylation of PSII proteins in maize thylakoids in the presence of Pb ions, J. Plant Physiol., 2012, 169, 345–352 CrossRef CAS PubMed.
- K. Shah, A. U. Mankad and M. N. Reddy, Cadmium accumulation and its effects on growth and biochemical parameters in Tagetes erecta L, J. Pharmacogn. Phytochem., 2017, 6, 111–115 CAS.
- R. Chandra and H. Kang, Mixed heavy metal stress on photosynthesis, transpiration rate, and chlorophyll content in poplar hybrids, For. Sci. Technol., 2016, 12, 55–61 Search PubMed.
- X. Shu, L. Yin, Q. Zhang and W. Wang, Effect of Pb toxicity on leaf growth, antioxidant enzyme activities, and photosynthesis in cuttings and seedlings of Jatropha curcas L, Environ. Sci. Pollut. Res. Int., 2011, 19, 893–902 CrossRef PubMed.
- G. Srivastava, S. Kumar, G. Dubey, V. Mishra and S. M. Prasad, Nickel and ultraviolet-B stresses induce differential growth and photosynthetic responses in Pisum sativum L. seedlings, Biol. Trace Elem. Res., 2012, 149, 86–96 CrossRef CAS PubMed.
-
A. K. Shanker, Physiological, biochemical and molecular aspects of chromium toxicity and tolerance in selected crops and tree species, PhD thesis, Tamil Nadu Agricultural University, Coimbatore, India, 2003.
- A. Vassilev, A. Nikolova, L. Koleva and F. Lidon, Effects of excess Zn on growth and photosynthetic performance of young bean plants, J. Phytol., 2011, 3, 58–62 CAS.
- A. Cuypers, J. Vangronsve and H. Clijsters, The redox status of plant cells (AsA and GSH) is sensitive to zinc imposed oxidative stress in roots and primary leaves
of Phaseolus vulgaris, Plant Physiol. Biochem., 2001, 39, 657–664 CrossRef CAS.
- A. Vassilev, F. C. Lidon, M. D. Matos, J. C. Ramalho and M. G. Bareiro, Shoot cadmium accumulation and photosynthetic performance of barley at high Cd treatments, J. Plant Nutr., 2004, 27, 773–793 CrossRef.
- N. Parsafar and S. Marofi, Investigation of transfer coefficients of Cd, Zn, Cu and Pb from soil to potato under wastewater reuse, Journal of Water and Soil Science, 2013, 17, 199–209 Search PubMed.
- J. Park, L. Dane and P. Periyasamy, Role of organic amendments on enhanced bioremediation of heavy metal (loid) contaminated soils, J. Hazard. Mater., 2011, 185, 549–574 CrossRef CAS PubMed.
- A. T. Gilvanise, G. Helena, D. F. Josely and R. M. Danilo, Effect of copper, zinc, cadmium and chromium in the growth of crambe, Agric. Sci., 2014, 5, 975–983 Search PubMed.
- R. Fryzova, M. Pohanka, P. Martinkova, H. Cihlarova, M. Brtnicky, J. Hladky and J. Kynicky, Oxidative stress and heavy metals in plants, Rev. Environ. Contam. Toxicol., 2017, 245, 129–156 CAS.
- D. Martinez-Dominguez, R. Torronteras-Santiago and F. Cordoba-Garcia, Modulation of the antioxidative response of Spartina densiflora against iron exposure, Physiol. Plant., 2009, 136, 169–179 CrossRef CAS PubMed.
- Y. Kono and I. Fridovich, Superoxide radical inhibits catalase, J. Biol. Chem., 1982, 257, 5751–5754 CrossRef CAS PubMed.
- C. R. Li, D. D. Liang, J. Li, Y. B. Duan, H. Li and Y. C. Yang, Unravelling mitochondrial retrograde regulation in the abiotic stress induction of rice ALTERNATIVE OXIDASE 1 genes, Plant Cell Environ., 2013, 36, 775–788 CrossRef CAS PubMed.
- N. S. Calgaroto, G. Y. Castro, D. Cargnelutti, L. B. Pereira, J. F. Gonçalves, L. V. Rossato, F. V. Antes, V. L. Dressler, E. M. M. Flroes, M. R. C. Schetinger and F. T. Nicoloso, Antioxidant system activation by mercury in Pfaffia glomerata plantlets, BioMetals, 2010, 23, 295–305 CrossRef CAS PubMed.
- D. M. Kasote, S. S. Katyare, M. V. Hegde and H. Bae, Significance of antioxidant potential of plants and its relevance to therapeutic applications, Int. J. Biol. Sci., 2015, 11, 982–991 CrossRef CAS PubMed.
-
M. A. Matilla-Vázquez and A. J. Matilla, Role of H2O2 as signaling molecule in plants, in Environmental adaptations and stress tolerance of plants in the era of climate change, ed. P. Ahmad and M. N. V. Prasad, Springer, New York, 2012, pp. 361–380 Search PubMed.
- M. Zarattini and G. Forlani, Toward unveiling the mechanisms for transcriptional regulation of proline biosynthesis in the plant cell response to biotic and abiotic stress conditions, Front. Plant Sci., 2017, 8, 927, DOI:10.3389/fpls.2017.00927.
- K. Fujishima, K. M. Wang, J. A. Palmer, N. Abe, K. Nakahigashi, D. Endy and L. J. Rothschild, Reconstruction of cysteine biosynthesis using engineered cysteine-free enzymes, Sci. Rep., 2018, 8, 1776, DOI:10.1038/s41598-018-19920-y.
- H. S. El-Beltagi and A. A. Mohamed, Changes in non protein thiols, some antioxidant enzymes activity and ultrastructural alteration in radish plant (Raphanus sativus L.) grown under lead toxicity, Not. Bot. Horti Agrobot. Cluj-Napoca, 2010, 38, 76–85 CAS.
- E. T. Dikkaya and N. Ergun, Effects of cadmium and zinc interactions on growth parameters and activities of ascorbate peroxidase on maize (Zea mays L. MAT 97), Eur. J. Exp. Biol., 2014, 4, 288–295 Search PubMed.
- M. Hasanuzzaman, K. Nahar, T. I. Anee and M. Fujita, Glutathione in plants: biosynthesis and physiological role in environmental stress tolerance, Physiol. Mol. Biol. Plants, 2017, 23, 249–268 CrossRef CAS PubMed.
- A. Malecka, A. Piechalak and B. Tomaszewska, Reactive oxygen species production and antioxidative defense system in pea root tissues treated with lead ions: The whole roots level, Acta Physiol. Plant., 2009, 31, 1053–1063 CrossRef CAS.
- S. Singh, S. Eapen and S. F. D'Souza, Cadmium accumulation and its influence on lipid peroxidation and antioxidative system in an aquatic plant, Bacopa monnieri L, Chemosphere, 2006, 62, 233–246 CrossRef CAS PubMed.
- M. Ashraf and M. R. Foolad, Roles of glycine betaine and proline in improving plant abiotic stress resistance, Environ. Exp. Bot., 2007, 59, 206–216 CrossRef CAS.
- M. Rusin, J. Gospodarek, G. Barczyk and A. Nadgórska-Socha, Antioxidant responses of Triticum aestivum plants to petroleum-derived substances, Ecotoxicol, 2018, 27, 1353–1367 CrossRef CAS PubMed.
- E. Islam, X. Yang, T. Li, D. Liu, X. Jin and F. Meng, Effect of Pb toxicity on root morphology, physiology and ultrastructure in the two ecotypes of Elsholtzia argyi, J. Hazard. Mater., 2007, 147, 806–816 CrossRef CAS PubMed.
- S. C. Chun and M. Chandrasekaran, Proline accumulation influenced by osmotic stress in arbuscular mycorrhizal symbiotic plants, Front. Microbiol., 2018, 9, 2525, DOI:10.3389/fmicb.2018.02525.
- J. Kumchai, J. Z. Huang, C. Y. Lee, F. C. Chen and S. W. Chin, Proline partially overcomes excess molybdenum toxicity in cabbage seedlings grown in vitro, Genet. Mol. Res., 2013, 12, 5589–5601 CrossRef CAS PubMed.
- S. Amini, C. Ghobadi and A. Yamchi, Proline accumulation and osmotic stress: an overview of P5CS gene in plants, Journal of Plant Molecular Breeding, 2015, 3, 44–55 Search PubMed.
- X. Liang, L. Zhang, S. K. Natarajan and D. F. Becker, Proline mechanisms of stress survival, Antioxid. Redox Signal., 2013, 19, 998–1011 CrossRef CAS PubMed.
- P. P. Saradhi, Alia and P. Mohanty, Involvement of proline in protecting thylakoid membranes against free radical-induced photodamage, J. Photochem. Photobiol., B, 1997, 38, 253–257 CrossRef.
- Alia and P. P. Saradhi, Suppression in mitochondrial electron transport is the prime cause behind stress induced proline accumulation, Biochem. Biophys. Res. Commun., 1993, 193, 54–58 CrossRef CAS PubMed.
- A. Hussain, N. Abbas, F. Arshad, M. Akram, Z. I. Khan, K. Ahmad, M. Mansha and F. Mirzaei, Effects of diverse doses of lead (Pb) on different growth attributes of Zea mays L, Agric. Sci., 2013, 4, 262–265 Search PubMed.
- K. Majumdar, M. L. Jat, M. Pampolino, T. Satyanarayana, S. Dutta and A. Kumar, Nutrient management in wheat: current scenario, improved strategies and future research needs in India, J. wheat res., 2013, 4, 1–10 Search PubMed.
-
USDA, Grain Report No. IN1011, Global Agricultural Information Network, Washington, 2010 Search PubMed.
-
V. Piironen, A. M. Lampi, P. Ekholm, M. Salmenkallio-Marttila and K. H. Liukkonen, Micronutrients and phytochemicals in wheat grain, in Wheat: Chemistry and technology, ed. K. Khan and P. R. Shewry, American Association of Cereal Chemists, Inc. (AACC), St. Paul, USA, 2009, pp. 179–222 Search PubMed.
- K. K. Adom, M. E. Sorrells and R. H. Liu, Phytochemical profiles and antioxidant activity of wheat varieties, J. Agric. Food Chem., 2003, 51, 7825–7834 CrossRef CAS PubMed.
- A. Breiman and D. Graur, Wheat evaluation, Isr. J. Plant Sci., 1995, 43, 58–95 Search PubMed.
- R. S. Peter and S. J. Hey, The contribution of wheat to human diet and health, Food Energy Secur., 2015, 4, 178–202 CrossRef PubMed.
- S. M. Stanišić Stojić, L. M. Ignjatović, S. Popov, S. Škrivanj, A. R. Đorđević and A. Stojić, Heavy metal accumulation in wheat and barley: the effects of soil presence and liquid manure amendment, Plant Biosyst., 2016, 150, 104–110 CrossRef.
- A. Ghani, I. Khan, S. Umer, I. Ahmed, I. Mustafa and N. Mohammad, Response of wheat (Triticum aestivum) to exogenously applied chromium: effect on growth, chlorophyll and mineral composition, J. Environ. Anal. Toxicol., 2015, 5, 1 Search PubMed.
- A. Gang, A. L. Vyas and H. Vyas, Toxic effect of heavy metals on germination and seedling growth of wheat, J. Environ. Res. Dev., 2013, 2, 206–213 Search PubMed.
- P. Sindhu, A. Saharma and P. Pooja, Total chlorophyll and total protein content in wheat (Triticum aestivum) grown under arsenic stress, Int. J. Recent Sci. Res., 2015, 6, 5072–5075 Search PubMed.
- L. B. Singh, R. K. Yadav and T. Abraham, Evaluate the different levels of zinc and methods of boron application on growth, yield and protein content of wheat (Triticum aestivum L.), Res. J. Environ. Sci., 2014, 7, 279–282 Search PubMed.
- M. Rizwan, S. Ali, T. Abbas, M. Zia-ur-Rehman, F. Hannan, C. Keller, M. I. Al-Wabel and Y. S. Ok, Cadmium minimization in wheat: a critical review, Ecotoxicol. Environ. Saf., 2016, 130, 43–53 CrossRef CAS PubMed.
- K. Yadav and N. B. Singh, Effects of benzoic acid and cadmium toxicity on wheat seedlings, Chil. J. Agric. Res., 2013, 73, 167–174 Search PubMed.
- M. H. Ibrahim, Y. Chee-Kong and N. A. Mohd Zain, Effect of cadmium and copper exposure on growth, secondary metabolites and antioxidant activity in the medicinal plant Sambung Nyawa (Gynura procumbens (Lour.) Merr), Molecules, 2017, 22, 1623, DOI:10.3390/molecules22101623.
- A. Emamverdian, Y. Ding, F. Mokhberdoran and Y. Xie, Heavy metal stress and some mechanisms of plant defense response, Sci. World J., 2015 DOI:10.1155/2015/756120.
- K. N. Ibrahim, Z. R. Yet, A. M. Som, N. Razali, N. A. M. Rahaizah, E. N. Othman, N. A. Burok, Y. M. Yunos, R. Othman and T. F. T. Yahya, Heavy metal concentration (Pb, Cu, Fe, Zn, Ni) in plant parts of Zea Mays L. cultivated in agricultural area Near Alor Gajah, Melaka, Malaysia, Am. J. Environ. Eng., 2015, 5(3A), 8–12 Search PubMed.
- M. F. S. Guilherme, H. M. de Oliveira and E. da Silva, Cadmium toxicity on seed germination and seedling growth of wheat Triticum aestivum, Acta Sci., Biol. Sci., 2015, 37, 499–504 CrossRef CAS.
- H. F. Kirmani, M. Hussain, F. Ahmad, M. Shahid and A. Asghar, Impact of zinc uptake on morphology, physiology and yield attributes of wheat in Pakistan, Cercetari Agronomice în Moldova, 2018, 51, 29–36 Search PubMed.
- S. Mathur, H. M. Kalaji and A. Jajoo, Investigation of deleterious effects of chromium phytotoxicity and photosynthesis in wheat plant, Photosynthetica, 2016, 54, 185–192 CrossRef CAS.
- V. Kumar and A. K. Chopra, Toxicity of chromium in agricultural crops with respect to its chemical speciation-A review, World Appl. Sci. J., 2015, 33, 944–969 CAS.
- A. Muslu and N. Ergün, Effects of copper and chromium and high temperature on growth, proline and protein content in wheat seedlings, Bangladesh J. Bot., 2013, 42, 105–111 CrossRef.
- R. N. Collins, E. Bakkaus, M. Carriere, H. Khodja, O. Proux, J. L. Morel and B. Gouget, Uptake, localization, and speciation of cobalt in Triticum aestivum L. (Wheat) and Lycopersicon esculentum M. (Tomato), Environ. Sci. Technol., 2010, 44, 2904–2910 CrossRef CAS PubMed.
- B. Sarma, P. Devi, N. Gogoi and Y. M. Devi, Effects of cobalt induced stress on Triticum aestivum L. crop, Asian J. Agric. & Biol., 2014, 2, 137–147 Search PubMed.
- T. Amari, T. Ghnaya, A. Debez, M. Taamali, N. B. Youssef, G. Lucchini, G. A. Sacchi and C. Abdelly, Comparative Ni tolerance and accumulation potentials between Mesembryanthemum crystallinum (halophyte) and Brassica juncea: metal accumulation, nutrient status and photosynthetic activity, J. Plant Physiol., 2014, 171, 1634–1644 CrossRef CAS PubMed.
- C. Fabiano, T. Tezotto, J. L. Favarin, J. C. Polacco and P. Mazzafera, Essentiality of nickel in plants: a role in plant stresses, Front. Plant Sci., 2015, 6, 754, DOI:10.3389/fpls.2015.00754.
- J. Nie, Y. Pan, J. Shi, Y. Guo, Z. Yan, X. Duan and M. Xu, A comparative study on the uptake and toxicity of nickel added in the form of different salts to maize seedlings, Int. J. Environ. Res. Publ. Health, 2015, 12, 15075–15087 CrossRef CAS PubMed.
- K. H. Bhatti, S. Anwar, K. Nawaz, K. Hussain, E. H. Siddiqi, R. U. Sharif, A. Talat and A. Khalid, Effect of heavy metal lead (Pb) stress of different concentration on wheat (Triticum aestivum L.), Middle East J. Sci. Res., 2013, 14, 148–154 CAS.
- M. Patra and A. Sharma, Mercury toxicity in plants, Bot. Rev., 2000, 66, 379–422 CrossRef.
- F. X. Han, Y. Su, D. L. Monts, A. C. Waggoner and J. M. Plodinec, Binding, distribution, and plant uptake of mercury in a soil from oak ridge, Tennessee, USA, Sci. Total Environ., 2006, 368, 753–768 CrossRef CAS PubMed.
- M. J. Mclaughlin, K. G. Tiller, R. Naidu and D. P. Stevens, The behaviour and environmental impact of contaminants in fertilizers, Aust. J. Soil Res., 1996, 34, 1–54 CrossRef CAS.
- M. Israr, S. Sahi, R. Datta and D. Sarkar, Bioaccumulation and physiological effects of mercury in Sesbania drummonii, Chemosphere, 2006, 65, 591–598 CrossRef CAS PubMed.
- A. A. Eman, A. E. Monem, M. M. S. Saleh and E. A. M. Mostafa, Minimizing the quantity of mineral nitrogen fertilization grapevine by using humic acid organic and biofertilizers, Res. J. Agric. Biol. Sci., 2008, 4, 46–50 Search PubMed.
-
R. P. Singh, M. Kumar and P. K. Jaiwal, Improvement in nitrogen use efficiency and yield of crop plants by sustained nutrient supply and enhanced nitrogen assimilation, in Development in physiology, biotechnology and molecular biology of plants, ed. B. Bose and A. Hemantranjan, New Delhi Publishing Agency, New Delhi, India, 2008, pp. 1–31 Search PubMed.
- N. Khan, A. Bano and M. D. A. Babar, The stimulatory effects of plant growth promoting rhizobacteria and plant growth regulators on wheat physiology grown in sandy soil, Arch. Microbiol., 2019, 201, 769–785 CrossRef CAS PubMed.
- S. Mahato and A. Kafle, Comparative study of Azotobacter with or without other fertilizers on growth and yield of wheat in Western hills of Nepal, Ann. Agrar. Sci., 2018, 16, 250–256 CrossRef.
- A. Sarker, N. M. Talukder and M. T. Islam, Phosphate solubilizing bacteria promote growth and enhance nutrient uptake by wheat, Plant Sci. Today, 2014, 1, 86–93 CrossRef.
- S. A. R. Hammad and O. A. M. Ali, Physiological and biochemical studies on drought tolerance of wheat plants by application of amino acids and yeast extract, Ann. Agric. Sci., 2014, 59, 133–145 CrossRef.
-
S. A. Wani, S. Chand, M. A. Wani, M. Ramzan and K. R. Hakeem, Azotobacter chroococcum–a potential biofertilizer in agriculture: an overview, in Soil science: agricultural and environmental prospectives, ed. K. R. Hakeem, J. Akhtar and M. Sabir, Springer, Cham, 2016, pp. 333–348 Search PubMed.
- K. Kukreja, S. Suneja, S. Goyal and N. Narula, Phytohormone production by Azotobacter- a review, Agric. Rev., 2004, 25, 70–75 Search PubMed.
- J. Gonzalez-Lopez, V. Salmeron, M. V. Martinez-Toledo, F. Ballesteros and A. Ramos-Cormenzana, Production of auxins, gibberellins and cytokinins by Azotobacter vinelandii ATCC12837 in chemically-defined media and dialized soil media, Soil Biol. Biochem., 1986, 18, 119–120 CrossRef CAS.
- S. S. Sindhu, Y. S. Rakshiya and G. Sahu, Biological control of soil-borne plant pathogens with rhizosphere bacteria, Pest Technol., 2009, 3, 10–21 Search PubMed.
- S. Verma, V. Kumar, N. Narula and W. Merbach, Studies on in vitro production of antimicrobial substances of Azotobacter chroococcum isolates/mutants, J. Plant Dis. Prot., 2001, 108, 152–165 CAS.
- C. H. Wu, S. M. Bernard, G. L. Anderson and W. Chen, Developing microbe-interactions for applications in plant growth promotion and disease control, production of useful compounds, remediation and carbon sequestration, Microb. Biotechnol., 2009, 2, 428–440 CrossRef CAS PubMed.
- S. Soliman, M. A. Seeda, S. S. M. Aly and A. M. Gadalla, Nitrogen fixation by wheat plants as affected by nitrogen fertilizer levels and non-symbiotic bacteria, Egypt. J. Soil Sci., 1995, 35, 401–413 Search PubMed.
- R. C. Sharma, Yield and yield components responses of wheat cultivars to seeding rate, J. Inst. Agric. Anim. Sci., 1987, 8, 99–110 Search PubMed.
- J. Singh and C. M. Singh, Effect of potassium application in rice-wheat cropping system, Indian J. Agron., 2000, 45, 12–20 Search PubMed.
- M. B. Hossain and M. A. Sattar, Effect of inorganic phosphorus fertilizer and inoculants on yield and phosphorus use efficiency of wheat, J. Environ. Sci. Nat. Resour., 2014, 7, 75–79 Search PubMed.
- A. H. Babana, A. Kassogué, A. H. Dicko, K. Maîga, F. Samaké, D. Traoré, R. Fané and F. A. Faradji, Development of a biological phosphate fertilizer to improve wheat (Triticum aestivum L.) production in Mali, Procedia Eng., 2016, 138, 319–324 CrossRef CAS.
- M. L. Nguyen, S. Spaepen, P. du Jardin and P. Delaplace, Biostimulant effects of rhizobacteria on wheat growth and nutrient uptake depend on nitrogen application and plant development, Arch. Agron. Soil Sci., 2018, 65, 58–73 CrossRef.
- F. Naili, M. Neifar, D. Elhidri, H. Cherif, B. Bejaoui and M. Aroua, Optimization of the effect of PGPR–based biofertlizer on wheat growth and yield, Biom. Biostat. Int. J., 2018, 7, 226–232 Search PubMed.
- H. Meena, M. A. Ahmed and P. Prakash, Amelioration of heat stress in wheat, Triticum aestivum by PGPR (Pseudomonas aeruginosa strain 2CpS1), Biosci. Biotech. Res. Comm., 2015, 8, 171–174 Search PubMed.
- G. Kaur and M. S. Reddy, Effects of phosphate-solubilizing bacteria, rock phosphate and chemical fertilizers on maize-wheat cropping cycle and economics, Pedosphere, 2015, 25, 428–437 CrossRef CAS.
- M. Tahir, U. Khalid, M. Ijaz, G. M. Shah, M. A. Naeem, M. Shahid, K. Mahmood, N. Ahmad and F. Kareem, Combined application of bio-organic phosphate and phosphorus solubilizing bacteria (Bacillus strain MWT 14) improve the performance of bread wheat with low fertilizer input under an arid climate, Braz. J. Microbiol., 2018, 49, 15–24 CrossRef CAS PubMed.
- N. A. Sial, S. A. Abro, M. Abbas, M. Irfan and N. Depar, Growth and yield of wheat as affected by phosphate solubilizing bacteria and phosphate fertilizer, Pak. J. Biotechnol., 2018, 15, 475–479 Search PubMed.
- A. Borham, E. Belal, M. Metwaly and Sh. El-Gremy, Phosphate solubilization by Enterobacter cloacae and its impact on growth and yield of wheat plants, Journal of Sustainable Agricultural Sciences, 2017, 43, 89–103 CrossRef.
- F. El Habil-Addas, S. Aarab, A. Rfaki, A. Laglaoui, M. Bakkali and A. Arakrak, Screening of phosphate solubilizing bacterial isolates for improving growth of wheat, European J. Biotechnol. Biosci., 2017, 5, 7–11 Search PubMed.
- S. C. MeCarty, D. S. Chauhan, A. D. MeCarty, K. M. Tripathi and T. Selvan, Effect of Azotobacter and phosphobacteria on yield of wheat (Triticum aestivum), Vegetos, 2017, 130, 2, DOI:10.4172/2229-4473.1000225.
- A. T. S. Zia-ul-Hassan, A. N. Shah, G. M. Jamro and I. Rajpar, Biopriming of wheat seeds with rhizobacteria containing ACC deaminase and phosphate solubilizing activities increases wheat growth and yield under phosphorus deficiency, Pak. J. Agric. Agric. Eng. Vet. Sci., 2015, 31, 24–32 Search PubMed.
- A. Kumar, B. R. Maurya and R. Raghuwanshi, Isolation and characterization of PGPR and their effect on growth, yield and nutrient content in wheat (Triticum aestivum L.), Biocatal. Agric. Biotechnol., 2014b, 3, 121–128 Search PubMed.
- S. Bulut, Evaluation of yield and quality parameters of phosphorous-solubilizing and N-fixing bacteria inoculated in wheat (Triticum aestivum L.), Turk. J. Agric. For., 2013, 37, 545–554 CrossRef CAS.
- M. A. Haque, M. A. Sattar, M. R. Islam, M. A. Hashem and M. K. Khan, Performance of phosphate solubilizing bacteria with various phosphorus levels on wheat in pot culture, J. Environ. Sci. Nat. Resour., 2013, 6, 221–226 Search PubMed.
- T. Hassan, A. Bano and I. Naz, Alleviation of heavy metals toxicity by the application of plant growth promoting rhizobacteria and effects on wheat grown in saline sodic field, Int. J. Phytoremediation, 2017, 19, 522–529 CrossRef PubMed.
- A. Meliani, A. Bensoltane, L. Benidir and K. Oufdou,
Plant growth-promotion and IAA secretion with Pseudomonas fluorescens and Pseudomonas putida, Res. Rev.: J. Bot. Sci., 2017, 6, 16–24 CAS.
- S. D. Herrera, C. Grossi, M. Zawoznik and M. D. Groppa, Wheat seeds harbour bacterial endophytes with potential as plant growth promoters and biocontrol agents of Fusarium graminearum, Microbiol. Res., 2016, 186, 37–43 CrossRef PubMed.
- F. D. Moreira, P. B. Costa, R. D. Souza, A. Beneduzi, B. B. Lisboa, L. K. Vargas and L. M. Passaglia, Functional abilities of cultivable plant growth promoting bacteria associated with wheat (Triticum aestivum L.) crops, Genet. Mol. Biol., 2016, 39, 111–121 CrossRef CAS PubMed.
- D. Safari, F. Jamali, H. R. Nooryazdan and F. Bayat, Screening fluorescent pseudomonads isolated from wheat rhizosphere for plant growth- promoting and salt tolerance properties, Biol. Forum Int. J., 2016, 8, 35–42 CAS.
- A. Kumar, B. R. Maurya and R. Raghuwanshi, Characterization of bacterial strains and their impact on plant growth promotion and yield of wheat and microbial populations of soil, Afr. J. Agric. Res., 2015, 10, 1367–1375 CrossRef.
- U. Amara, Y. X. Wang, X. L. Cui, R. Khalid, S. Ali, G. Shabbir and R. Hayat, Screening and identification of soil bacteria for growth promotion of wheat (Triticum aestivum L.), J. Biodivers. Environ. Sci., 2015, 7, 87–99 Search PubMed.
- M. Abaid-Ullah, M. N. Hassan, M. Jamil, G. Brader, M. K. N. Shah, A. Sessitsch and H. FY, Plant growth promoting rhizobacteria: an alternate way to improve yield and quality of wheat (Triticum aestivum), Int. J. Agric. Biol., 2015, 17, 51–60 Search PubMed.
- A. Majeed, M. K. Abbasi, S. Hameed, A. Imran and N. Rahim, Isolation and characterization of plant growth-promoting rhizobacteria from wheat rhizosphere and their effect on plant growth promotion, Front. Microbiol., 2015, 6, 1–10 Search PubMed.
- E. Truta, G. Vochita, M. M. Zamfirache, Z. Olteanu and C. M. Rosu, Copper-induced genotoxic effects in root meristems of Triticum aestivum L. cv. beti. Carp, Journal of Environment and Earth Science, 2013, 8, 83–92 Search PubMed.
-
M. Kubareva and E. Denisova, Evaluation of the cytogenotoxic activity of mercury by analyzing chromosomal aberrations in wheat root meristem cells, in IOP Conference Series: Earth and Environmental Science, IOP Publishing, 2019, vol. 315, no. 5, p. 052028 Search PubMed.
- T. A. Akcin, A. Akcin and C. Yildirim, Effects of chromium on anatomical characteristics of bread wheat (Triticum aestivum L. cv.“Ekiz”), J. Int. Environ. Appl. Sci., 2018, 13, 27–32 CAS.
- A. K. Shweti and J. S. Verma, Effects of nickel chloride on germination and seedling growth of different wheat (Triticum aestivum L. em Thell.) cultivars, J. Pharmacogn. Phytochem., 2018, 7, 2227–2234 Search PubMed.
- Ş. S. Çatav, T. O. Genç, M. K. Oktay and K. Küçükakyüz, Cadmium toxicity in wheat: impacts on element contents, antioxidant enzyme activities, oxidative stress, and genotoxicity, Bull. Environ. Contam. Toxicol., 2020, 104, 71–77 CrossRef PubMed.
- Z. Su, G. Wang, L. Xu, J. Zhang and X. Liu, Effects of Cu stress on physiological, biochemical, and spectral properties of wheat at different growth stages, Int. J. Agric. & Biol. Eng., 2019, 12, 147–153 Search PubMed.
- S. R. Saleh, M. M. Kandeel, D. Ghareeb, T. M. Ghoneim, N. I. Talha, B. Alaoui-Sossé, L. Aleya and M. M. Abdel-Daim, Wheat biological responses to stress caused by cadmium, nickel and lead, Sci. Total Environ., 2020, 706, 136013 CrossRef CAS PubMed.
- K. Vinod, G. Awasthi and P. K. Chauchan, Cu and Zn tolerance and responses of the biochemical and physiochemical system of wheat, J. Stress Physiol. Biochem., 2012, 8 Search PubMed.
- D. K. Tripathi, R. K. Mishra, S. Singh, S. Singh, V. P. Singh, P. K. Singh, D. K. Chauhan, S. M. Prasad, N. K. Dubey and A. C. Pandey, Nitric oxide ameliorates zinc oxide nanoparticles phytotoxicity in wheat seedlings: implication of the ascorbate-glutathione cycle, Front. Plant Sci., 2017, 8, 1 Search PubMed.
- S. A. Alamri, M. H. Siddiqui, M. Y. Al-Khaishany, M. Nasir Khan, H. M. Ali, I. A. Alaraidh, A. A. Alsahli, H. Al-Rabiah and M. Mateen, Ascorbic acid improves the tolerance of wheat plants to lead toxicity, J. Plant Interact., 2018, 13, 409–419 CrossRef CAS.
- N. A. Gurave, V. V. Korde, S. S. Dhas and M. Disale, Isolation and identification of heavy metal resistant bacteria from petroleum soil of Loni, Ahmednagar, Eur. J. Exp. Biol., 2015, 5, 6–11 CAS.
- A. A. L. De Silva, M. A. R. de Carvalho, A. L. de S. Sérgio, P. M. T. Dias, R. G. da Silva Filho, S. de M. S. Carmen, A. de M. B. Cleonice and E. Hofer, Heavy metal tolerance (Cr, Ag and Hg) in bacteria isolated from sewage, Braz. J. Microbiol., 2012, 43, 1620–1631 CrossRef.
- M. S. Khan, A. Zaidi, P. A. Wani and M. Oves, Role of plant growth promoting rhizobacteria in the remediation of metal contaminated soils, Environ. Chem. Lett., 2009, 7, 1–9 CrossRef.
- O. D. Ianeva, Mechanisms of bacteria resistance to heavy metals, Mikrobiol. Z., 2009, 71, 54–65 CAS.
- A. E. Abo-Amer, E.-S. R. Abd El-Raheem and M. A. Othman, Isolation and molecular characterization of heavy metal resistant Alcaligenes faecalis from sewage waste water and synthesis of silver nanoparticles, Geomicrobiology, 2015 DOI:10.1080/01490451.2015.1010754.
- A. Khusro, J. P. Preetam Raj and S. G. Panicker, Multiple heavy metals response and antibiotic sensitivity pattern of Bacillus subtilis strain KPA, Int. J. Res. Pharm. Chem., 2014, 6, 532–538 CAS.
- O. M. Alzahrani and N. A. Thoufeek, Isolation and characterization of heavy metal resistant Bacillus subtilis spp. collected from water sources of Taif province of Saudi Arabia, Int. J. Curr. Microbiol. Appl. Sci., 2015, 4, 350–357 CAS.
- M. Dadook, S. Mehrabian, M. Salehi and S. Irian, Morphological, biochemical and molecular characterization of twelve nitrogen-fixing bacteria and their response to various zinc concentration, Jundishapur J. Microbiol., 2014, 7, 9415 Search PubMed.
- E. Shoeb, U. Badar, J. Akhter, H. Shams and M. Sultana,
et al., Horizontal gene transfer of stress resistance genes through plasmid transport, World J. Microbiol. Biotechnol., 2012, 28, 1021–1025 CrossRef CAS PubMed.
- D. H. Nies, Microbial heavy-metal resistance, Appl. Microbiol. Biotechnol., 1999, 51, 730–750 CrossRef CAS PubMed.
- N. Khunajakr, C. Q. Liu, P. Charoenchai and N. W. Dunn, A plasmid-encoded two-component regulatory system involved in copper-inducible transcription in Lactococcus lactis, Gene, 1999, 229, 229–235 CrossRef CAS PubMed.
- Y. Aktan, S. Tan and B. Icgen, Characterizations of lead-resistant river isolate Enterococcus faecalis and assessment of its multiple metal and antibiotic resistance, Environ. Monit. Assess., 2013, 185, 5285–5293 CrossRef CAS PubMed.
- A. E. Abo-Amer, M. A. Abu-Gharbia, E. S. M. Soltan and W. M. Abd El-Raheem, Isolation and molecular characterization of heavy metal-resistant Azotobacter chroococcum from agricultural soil and their potential application in bioremediation, Geomicrobiol. J., 2014, 31, 551–561 CrossRef CAS.
- C. Pal, J. Bengtsson-Palme, E. Kristiansson and D. J. Larsson, Co-occurrence of resistance genes to antibiotics, biocides and metals reveals novel insights into their co-selection potential, BMC Genom., 2015, 16, 964, DOI:10.1186/s12864-015-2153-5.
- S. Silver and L. T. Phung, Bacterial heavy metal resistance: new surprises, Annu. Rev. Microbiol., 1996, 50, 753–789 CrossRef CAS PubMed.
- J. Xu, Y. S. Tian, R. H. Peng, A. S. Xiong and B. Zhu, Cyanobacteria MT gene SmtA enhance zinc tolerance in Arabidopsis, Mol. Biol. Rep., 2010, 37, 11051110 Search PubMed.
- R. E. Macur, C. R. Jackson, L. M. Botero, T. R. McDermott and W. P. Inskeep, Bacterial populations associated with the oxidation and reduction of arsenic in an unsaturated soil, Environ. Sci. Technol., 2004, 38, 104–111 CrossRef CAS PubMed.
- S. K. Kannan and R. Krishnamoorthy, Isolation of mercury resistant bacteria and influence of abiotic factors on bioavailability of mercury- a case study in Pulicat Lake North of Chennai, South East India, Sci. Total Environ., 2006, 367, 341–353 CrossRef CAS PubMed.
- V. Srivastava, A. Sarkar, S. Singh, P. Singh, A. S. de Araujo and R. P. Singh, Agroecological responses of heavy metal pollution with special emphasis on soil health and plant performances, Front. Environ. Sci., 2017, 5, 64, DOI:10.3389/fenvs.2017.00064.
- P. Gupta and B. Diwan, Bacterial exopolysaccharide mediated heavy metal removal: a review on biosynthesis, mechanism and remediation strategies, Biotechnol. Rep., 2017, 13, 58–71 CrossRef PubMed.
- C. C. Azubuike, C. B. Chikere and G. C. Okpokwasili, Bioremediation techniques-classification based on site of application: principles, advantages, limitations and prospects, World J. Microbiol. Biotechnol., 2016, 32, 180, DOI:10.1007/s11274-016-2137-x.
- J. Liu, J. Wang, S. Lee and R. Wen, Copper-caused oxidative stress triggers the activation of antioxidant enzymes via ZmMPK3 in maize leaves, PloS One, 2018, 13, e0203612, DOI:10.1371/journal.pone.0203612.
- T. Paul, A. Chakraborty, E. Islam and S. K. Mukherjee, Arsenic bioremediation potential of arsenite-oxidizing Micrococcus sp. KUMAs15 isolated from contaminated soil, Pedosphere, 2018, 28, 299–310 CrossRef CAS.
- J. Camacho-Chab, M. Castañeda-Chávez, M. Chan-Bacab, R. Aguila-Ramírez, I. Galaviz-Villa, P. Bartolo-Pérez, F. Lango-Reynoso, C. Tabasco-Novelo, C. Gaylarde and B. Ortega-Morales, Biosorption of cadmium by non-toxic extracellular polymeric substances (EPS) synthesized by bacteria from marine intertidal biofilms, Int. J. Environ. Res. Publ. Health, 2018, 15, 314, DOI:10.3390/ijerph15020314.
- N. Upadhyay, K. Vishwakarma, J. Singh, M. Mishra, V. Kumar, R. Rani, R. K. Mishra, D. K. Chauhan, D. K. Tripathi and S. Sharma, Tolerance and reduction of chromium (VI) by Bacillus sp. MNU16 isolated from contaminated coal mining soil, Front. Plant Sci., 2017, 8, 778, DOI:10.3389/fpls.2017.00778.
- K. K. Ghaima, A. I. Mohamed, W. Y. Al Meshhdany and A. A. Abdulhassan, Resistance and bioadsorption of cadmium by Pseudomonas aeruginosa isolated from agricultural soil, Int. J. Appl. Environ. Sci., 2017, 12, 1649–1660 Search PubMed.
- E. Abatenh, B. Gizaw, Z. Tsegaye and M. Wassie, Application of microorganisms in bioremediation-review, Journal of Environmental Microbiology, 2017, 1, 2–9 Search PubMed.
- Z. Ahmad, M. Imran, S. Qadeer, S. Hussain, R. Kausar, L. Dawson and A. Khalid, Biosurfactants for sustainable soil management, Adv. Agron., 2018, 150, 81–130 Search PubMed.
- M. A. Ashraf, I. Hussain, R. Rasheed, M. Iqbal, M. Riaz and M. S. Arif, Advances in microbe-assisted reclamation of heavy metal contaminated soils over the last decade: a review, J. Environ. Manage., 2017, 198, 132–143 CrossRef CAS PubMed.
- R. Dixit, Wasiullah, D. Malaviya, K. Pandiyan, U. B. Singh, A. Sahu, R. Shukla, B. P. Singh, J. P. Rai, P. K. Sharma, H. Lade and D. Paul, Bioremediation of heavy metals from soil and aquatic environment: an overview of principles and criteria of fundamental processes, Sustainability, 2015, 7, 2189–2212 CrossRef.
- B. E. Igiri, S. I. Okoduwa, G. O. Idoko, E. P. Akabuogu, A. O. Adeyi and I. K. Ejiogu, Toxicity and bioremediation of heavy metals contaminated ecosystem from tannery wastewater: a review, J. Toxicol., 2018 DOI:10.1155/2018/2568038.
-
S. Jain and D. Arnepalli, Biomineralization as a remediation technique: a critical review, Proceedings of the Indian Geotechnical Conference (IGC2016), Chennai, India, 2016, pp. 15–17 Search PubMed.
- J. Mishra, R. Singh and N. K. Arora, Alleviation of heavy metal stress in plants and remediation of soil by rhizosphere microorganisms, Front. Microbiol., 2017, 8, 1706, DOI:10.3389/fmicb.2017.01706.
- B. Mu'minah, H. Subair, Fahruddin and B. Darwisah, Isolation and screening of exopolysaccharide producing bacterial (EPS) from potato rhizosphere for soil aggregation, Int. J. Curr. Microbiol. Appl. Sci., 2015, 4, 341–349 Search PubMed.
- S. L. Sivapriya and P. R. Priya, Selection of hyper exopolysaccharide producing and cyst forming Azotobacter isolates for better survival under stress conditions, Int. J. Curr. Microbiol. Appl. Sci., 2017, 6, 2310–2320 CrossRef CAS.
- S. A. Moghannem, M. M. Farag, A. M. Shehab and M. S. Azab, Exopolysaccharide production from Bacillus velezensis KY471306 using statistical experimental design, Braz. J. Microbiol., 2018, 49, 452–462 CrossRef CAS PubMed.
- M. M. S. Asker, M. G. Mahmoud, A. Y. Ibrahim and S. S. Mohamed, Inhibitory effect of exopolysaccharide from Achromobacter piechaudii NRC2 against cyclooxygenases and acetylcholinesterase with evaluation of its antioxidant properties and structure elucidation, Der Pharm. Lett., 2015, 7, 129–141 Search PubMed.
- S. Periasamy, H. A. S. Nair, K. W. K. Lee, J. Ong, J. Q. J. Goh, S. Kjelleberg and S. A. Rice,
Pseudomonas aeruginosa PAO1 exopolysaccharides are important for mixed species biofilm community development and stress tolerance, Front. Microbiol., 2015, 6, 851, DOI:10.3389/fmicb.2015.00851.
- N. Nocelli, P. C. Bogino, E. Banchio and W. Giordano, Roles of extracellular polysaccharides and biofilm formation in heavy metal resistance of rhizobia, Materials, 2016, 9, 418, DOI:10.3390/ma9060418.
- A. W. Decho and T. Gutierrez, Microbial extracellular polymeric substances (EPS) in ocean systems, Front. Microbiol., 2017, 8, 922, DOI:10.3389/fmicb.2017.00922.
- B. V. Mohite, S. H. Koli and S. V. Patil, Heavy metal stress and its consequences on exopolysaccharide (EPS)-producing Pantoea agglomerans, Appl. Biochem. Biotechnol., 2018, 186, 199–216 CrossRef CAS PubMed.
- R. Batool, U. Marghoob and A. Kalsoom, Estimation of exopolysaccharides (EPS) producing ability of Cr (VI) resistant bacterial strains from tannery effluent, J. Basic Appl. Sci., 2017, 13, 589–596 CrossRef CAS.
- M. Muthu, H. F. Wu, J. Gopal, I. Sivanesan and S. Chun, Exploiting microbial polysaccharides for biosorption of trace elements in aqueous environments-scope for expansion via nanomaterial intervention, Polymers, 2017, 9, 721, DOI:10.3390/polym9120721.
- L. I. Zivkovic, M. Rikalovic, G. G. Cvijovic, S. Kazazic, M. Vrvic, I. Brceski, V. Beskoski, B. Loncarevic, K. Gopcevic and I. Karadzic, Cadmium specifc proteomic responses of a highly resistant Pseudomonas aeruginosa san ai, RSC Adv., 2018, 8, 10549–10560 RSC.
- B. A. Rasulov, A. Yili and H. A. Aisa, Biosorption of metal ions by exopolysaccharide produced by Azotobacter chroococcum XU1, J. Environ. Prot., 2013, 4, 989–993 CrossRef.
- A. Rizvi, B. Ahmed, A. Zaidi and M. S. Khan, Bioreduction of toxicity influenced by bioactive molecules secreted under metal stress by Azotobacter chroococcum, Ecotoxicol, 2019, 28, 302–322 CrossRef CAS PubMed.
- A. Rizvi and M. S. Khan, Putative role of bacterial biosorbent in metal sequestration revealed by SEM–EDX and FTIR, Indian J. Microbiol., 2019 DOI:10.1007/s12088-019-00780-7.
-
A. Bankar and G. Nagaraja, Recent trends in biosorption of heavy metals by Actinobacteria, in New and future developments in microbial biotechnology and bioengineering, ed. V. Gupta, Elsevier, 2018, pp. 257–275 Search PubMed.
- G. P. Sheng, J. Xu, W. H. Li and H. Q. Yu, Quantification of the interactions between Ca2+, Hg2+ and extracellular polymeric substances (EPS) of sludge, Chemosphere, 2013, 93, 1436–1441 CrossRef CAS PubMed.
- Z. Zhang, R. Cai, W. Zhang, Y. Fu and N. Jiao, A novel exopolysaccharide with metal adsorption capacity produced by a marine bacterium Alteromonas sp. JL2810, Mar. Drugs, 2017b, 15, 175, DOI:10.3390/md15060175.
- S. Zhao, F. Cao, H. Zhang, L. Zhang, F. Zhang and X. Liang, Structural characterization and biosorption of exopolysaccharides from Anoxybacillus sp. R4-33 isolated from radioactive radon hot spring, Appl. Biochem. Biotechnol., 2014b, 172, 2732–2746 Search PubMed.
- P. M. Joshi and A. A. Juwarkar, In vivo studies to elucidate the role of extracellular polymeric substances from Azotobacter in immobilization of heavy metals, Environ. Sci. Technol., 2009, 43, 5884–5889 CrossRef CAS PubMed.
- S. S. Gauri, S. M. Mandal and B. R. Pati, Impact of Azotobacter exopolysaccharides on sustainable agriculture, Appl. Microbiol. Biotechnol., 2012, 95, 331–338 CrossRef CAS PubMed.
- D. Kalita and S. R. Joshi, Study on bioremediation of lead by exopolysaccharide producing metallophilic bacterium isolated from extreme habitat, Biotechnol. Rep., 2017, 16, 48–57 CrossRef PubMed.
- G. Guibaud, D. Bhatia, P. d'Abzac, I. Bourven, F. Bordas, E. D. Van Hullebusch and P. N. Lens, Cd (II) and Pb (II) sorption by extracellular polymeric substances (EPS) extracted from anaerobic granular biofilms: evidence of a pH sorption-edge, J. Taiwan Inst. Chem. Eng., 2012, 43, 444–449 CrossRef CAS.
- A. Dadrasnia, K. S. Chuan Wei, N. Shahsavari, M. S. Azirun and S. Ismail, Biosorption potential of Bacillus salmalaya strain 139SI for removal of Cr (VI) from aqueous solution, Int. J. Environ. Res. Publ. Health, 2015, 12, 15321–15338 CrossRef CAS PubMed.
- S. H. Abbas, I. M. Ismail, T. M. Mostafa and A. H. Sulaymon, Biosorption of heavy metals: a review, Journal of Chemical Science and Technology, 2014, 3, 74–102 Search PubMed.
- A. S. Ayangbenro and O. O. Babalola, A new strategy for heavy metal polluted environments: a review of microbial biosorbents, Int. J. Environ. Res. Publ. Health, 2017, 14, 94, DOI:10.3390/ijerph14010094.
- P. Prabhakaran, M. A. Ashraf and W. S. Aqma, Microbial stress response to heavy metals in the environment, RSC Adv., 2016, 6, 109862–109877 RSC.
- S. Malkoc, E. Kaynak and K. Guven, Biosorption of zinc (II) on dead and living biomass of Variovorax paradoxus and Arthrobacter viscosus, Desalin. Water Treat., 2016, 57, 15445–15454 CrossRef CAS.
- S. Patil and G. Unnikrishnan, Fourier transform infrared spectroscopic characterization of heavy metal-induced metabolic changes in the thermophiles, World J. Pharm. Pharmaceut. Sci., 2017, 7, 592–599 Search PubMed.
- M. Enshaei, A. Khanafari and A. A. Sepahey, Metallothionein induction in two species of Pseudomonas exposed to cadmium and copper contamination, Iran. J. Environ. Health Sci. Eng., 2010, 7, 287–298 CAS.
- V. Diopan, V. Shestivska, V. Adam, T. Macek, M. Mackova, L. Havel and R. Kizek, Determination of content of metallothionein and low molecular mass stress peptides in transgenic tobacco plants, Plant Cell, Tissue Organ Cult., 2008, 94, 291–298 CrossRef CAS.
- M. Si and J. Lang, The roles of metallothioneins in carcinogenesis, J. Hematol. Oncol., 2018, 11, 107 CrossRef PubMed.
- K. S. Vignesh and G. S. Deepe Jr, Metallothioneins: emerging modulators in immunity and infection, Int. J. Mol. Sci., 2017, 18, 2197 CrossRef PubMed.
- M. A. Hossain, P. Piyatida, J. A. da Silva and M. Fujita, Molecular mechanism of heavy metal toxicity and tolerance in plants: central role of glutathione in detoxification of reactive oxygen species and methylglyoxal and in heavy metal chelation, J. Bot., 2012a DOI:10.1155/2012/872875.
- S. Murthy, G. Bali and S. K. Sarangi, Effect of lead on metallothionein concentration in lead resistant bacteria Bacillus cereus isolated from industrial effluent, Afr. J. Biotechnol., 2011, 10, 15966–15972 CAS.
- K. A. Mosa, I. Saadoun, K. Kumar, M. Helmy and O. P. Dhankher, Potential biotechnological strategies for the cleanup of heavy metals and metalloids, Front. Plant Sci., 2016, 7, 303, DOI:10.3389/fpls.2016.00303.
- V. Maru and S. Gadre, Melanin pigment production studies from Azotobacter vinelandii, Int. J. Adv. Life Sci., 2016, 9, 44–49 CAS.
- A. Banerjee, S. Supakar and R. Banerjee, Melanin from the nitrogen-fixing bacterium Azotobacter chroococcum: a spectroscopic characterization, PloS One, 2014, 9, e84574 CrossRef PubMed.
- H. Thaira, K. Raval, V. Manirethan and R. M. Balakrishnan, Melanin nano-pigments for heavy metal remediation from water, Separ. Sci. Technol., 2018, 2, 1–10 Search PubMed.
- R. J. Cordero, R. Vij and A. Casadevall, Microbial melanins for radioprotection and bioremediation, Microb. Biotechnol., 2017, 10, 1186–1190 CrossRef PubMed.
- N. E. El-Naggar and S. M. El-Ewasy, Bioproduction, characterization, anticancer and antioxidant activities of extracellular melanin pigment produced by newly isolated microbial cell factories Streptomyces glaucescens NEAE-H, Sci. Rep., 2017, 7, 42129 CrossRef CAS PubMed.
- M. P. N. Rao, M. Xiao and W. J. Li, Fungal and bacterial pigments: secondary metabolites with wide applications, Front. Microbiol., 2017, 8, 1113, DOI:10.3389/fmicb.2017.01113.
- J. Côte, A. Boniface, S. Blanchet, A. P. Hendry, J. Gasparini and L. Jacquin, Melanin-based coloration and host–parasite interactions under global change, Proc. R. Soc. Ser. B, 2018, 285(1879), 20180285 CrossRef PubMed.
- K. Tarangini and S. Mishra, Production, characterization and analysis of melanin from isolated marine Pseudomonas sp. using vegetable waste, Res. J. Eng. Sci., 2013, 2, 40–46 Search PubMed.
- A. M. Cuong, N. T. Le Na, P. N. Thang, T. N. Diep, L. B. Thuy, N. L. Thanh and N. D. Thang, Melanin-embedded materials effectively remove hexavalent chromium (Cr VI) from aqueous solution, Environ. Health Prev. Med., 2018, 23, 9, DOI:10.1186/s12199-018-0699-y.
- J. M. Drewnowska, M. Zambrzycka, B. Kalska-Szostko, K. Fiedoruk and I. Swiecicka, Melanin-like pigment synthesis by soil Bacillus weihenstephanensis isolates from Northeastern Poland, PloS One, 2015, 10, e0125428 CrossRef PubMed.
- V. G. R. Bhargavi and T. Padmavathi,
Bacillus sp. as potential plant growth promoting rhizobacteria, Int. J. Adv. Life Sci., 2016, 9, 28–36 Search PubMed.
- K. I. Shinwari, A. Shah, M. I. Afridi, M. Zeeshan, H. Hussain, J. Hussain, O. Ahmad and M. Jamil, Application of plant growth promoting rhizobacteria in bioremediation of heavy metal polluted soil, Asian J. Multidiscip. Stud., 2015, 3, 179–185 Search PubMed.
- C. Verma, P. Singh and R. Kumar, Isolation and characterization of heavy metal resistant PGPR and their role in enhancement of growth of wheat plant under metal (cadmium) stress condition, Arch. Appl. Sci. Res., 2015, 7, 37–43 CAS.
- I. Shahzadi, A. Khalid, S. Mahmood, M. Arshad, T. Mahmood and I. Aziz, Effect of bacteria containing ACC deaminase on growth of wheat seedlings grown with chromium contaminated
water, Pak. J. Bot., 2013, 45, 487–494 Search PubMed.
- F. Islam, T. Yasmeen, Q. Ali, S. Ali, M. S. Arif, S. Hussain and H. Rizvi, Influence of Pseudomonas aeruginosa as PGPR on oxidative stress tolerance in wheat under Zn stress, Ecotoxicol. Environ. Saf., 2014, 104, 285–293 CrossRef CAS PubMed.
- M. Janmohammadi, M. R. Bihamta and V. Ghasemzadeh, Influence of rhizobacteria inoculation and lead stress on the physiological and biochemical attributes of wheat genotypes, Cercet. Agron. Mold., 2013, 153, 49–67 Search PubMed.
- H. Wang, R. Xu, L. You and G. Zhong, Characterization of Cu- tolerant bacteria and definition of their role in promotion of growth, Cu accumulation and reduction of Cu toxicity in Triticum aestivum L, Ecotoxicol. Environ. Saf., 2013a, 94, 1–7 Search PubMed.
- A. Silini, H. Cherif-Silini and B. Yahiaoui, Growing varieties durum wheat (Triticum durum) in response to the effect of osmolytes and inoculation by Azotobacter chroococcum under salt stress, Afr. J. Microbiol. Res., 2016, 10, 387–399 CrossRef CAS.
- R. Khalilzadeh, R. Seyed Sharifi and J. Jalilian, Antioxidant status and physiological responses of wheat (Triticum aestivum L.) to cycocel application and bio fertilizers under water limitation condition, J. Plant Interact., 2016, 11, 130–137 CrossRef CAS.
- S. K. Upadhyay, J. S. Singh, A. K. Saxena and D. P. Singh, Impact of PGPR inoculation on growth and antioxidant status of wheat under saline conditions, Plant Biol., 2012, 14, 605–611 CrossRef CAS PubMed.
- J. Soto, J. Ortiz, H. Herrera, A. Fuentes, L. Almonacid, T. C. Charles and C. Arriagada, Enhanced arsenic tolerance in Triticum aestivum inoculated with arsenic-resistant and plant growth promoter microorganisms from a heavy metal-polluted soil, Microorganisms, 2019, 7, 348 CrossRef CAS PubMed.
- N. Khan and A. Bano, Exopolysaccharide producing rhizobacteria and their impact on growth and drought tolerance of wheat grown under rainfed conditions, PloS One, 2019, 14, e0222302, DOI:10.1371/journal.pone.0222302.
|
This journal is © The Royal Society of Chemistry 2020 |
Click here to see how this site uses Cookies. View our privacy policy here.