DOI:
10.1039/D1NA00119A
(Review Article)
Nanoscale Adv., 2021,
3, 3730-3745
An overview on the investigation of nanomaterials' effect on plasma components: immunoglobulins and coagulation factor VIII, 2010–2020 review
Received
14th February 2021
, Accepted 16th May 2021
First published on 18th May 2021
Abstract
FVIII and immunoglobulins (Igs) are the most prominent plasma proteins, which play a vital role in plasma hemostasis. These proteins have been implemented frequently in protein therapy. Therefore, their maintenance, durability, and stability are highly essential. Herein, various approaches to improve protein functions have been investigated, such as using recombinant protein replacement. In comparison, advances in nanotechnology have provided adequate context to boost biomaterial utilization. In this regard, the applications of various nanoparticles such as polymeric nanomaterials (PEG and PLGA), metal nanoparticles, dendrimers, and lipid based nanomaterials (liposomes and lipid nanoparticles) in stability and the functional improvement of antibodies and coagulation factor VIII (FVIII) have been reviewed from 2010 to 2020. Reviewing related articles has shown that not only can nanomaterials adequately protect the structure of proteins, but have also improved proteins' functions in some cases. For example, the high rate of FVIII instability has been successfully enhanced by bio-PEGylation. Also, utilizing PEGylated liposomes, using the PEG-lip technique for coating nanostructures, leads to FIIIV half-life prolongation. Hence, PEGylation had most impact on the stability of FVIII. Likewise, PEG-coated liposome nano-carriers also presented such a good effect on stability improvements for FVIII due to their ability to tune the immune system by reducing FVIII immunogenicity. Similarly, Ig PEGylation and conjugation to magnetic nanoparticles resulted in increased half-life and better purification of Igs, respectively, without any loss in structural or functional features. Consequently, metal–organic frameworks and recent hybrid systems have been introduced as promising nanomaterials in biomedical applications. As far as we know, this is the first study in this field, which considers the applications of nanoparticles for improving the storage and stability of antibodies and coagulation FVIII.
1. Introduction
Coagulation factors and immunoglobulins (Igs) are two main orderly plasma proteins that play crucial role in blood hemostasis, which stop bleeding through the coagulation cascade, and develop immunogenicity against foreign external components. They are also significant components that are frequently used in protein therapy. In this regard, maintaining the efficiency and improving the stability of these two essential proteins is strongly being followed. However, the objective has been under-researched by scientists.
Advances in nanotechnology in therapeutic and medical applications could open new horizons to employ nanomaterials in protein therapy. Using nanocarriers has improved the efficacy of various therapeutics1–5 (Fig. 1). Here we are going to review the applications of nanoparticles in the improvement of storage of antibodies and coagulation factor VIII from 2010 to 2020. As far as we know, this is this first study in this field, which considers the applications of nanoparticles for improving the storage of antibodies and coagulation factor VIII in order to introduce the most efficient strategies for sustainability and consequently improving the quality of the life of patients.
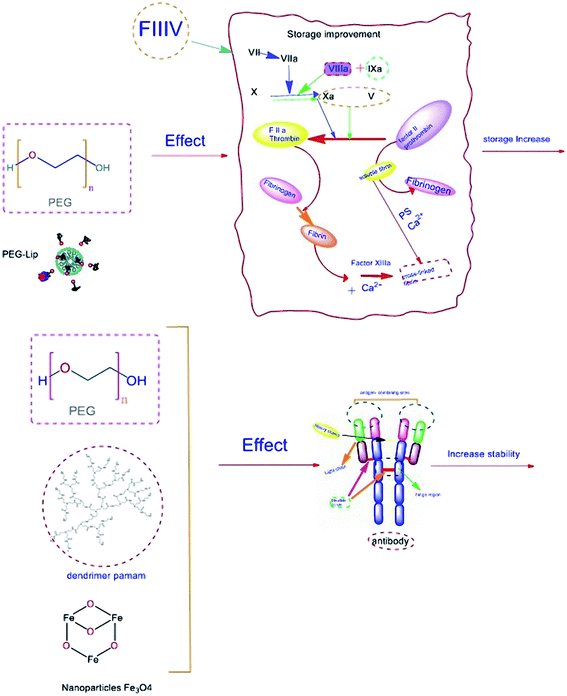 |
| Fig. 1 Overview of nanoparticle effects on two essential plasma proteins. | |
2. Results and discussion
Here, the results of studies focusing on nanomaterials which were conjugated with protein factors such as FVIII and immunoglobulins will be highlighted.
2.1 Coagulation factor VIII
Coagulation factor VIII (FVIII) is a non-enzymatic glycoprotein composition containing six domains, NH2–A1–A2–B–A3–C1–C2–COOH, which serves as a critical component of the intrinsic coagulation pathway.6–9 Coagulation pathways are illustrated in Fig. 2.
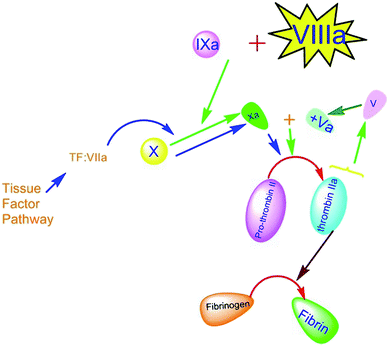 |
| Fig. 2 Schematic image of extrinsic coagulation pathways and the role of FVIII. | |
The coagulopathy resulting from a deficiency in synthesis or activity of FVIII leads to type A hemophilia.10–13 This bleeding disorder is characterized by spontaneous hemorrhage, particularly in joints and muscles due to the congenital x-linked disorder that occurs in 1 of 5000 male births.14,15 Less prevalent hemophilia (type B) can also occur in 1 of 30
000 male births, resulting from FIX deficiency. FVIII is carried in plasma, associated with a protein factor, called von Willebrand factor (vWF), which plays a chaperone-like role in plasma to stabilize FVIII against dendritic cells' clearance and degradation.16,17 Free FVIII is a labile component that just remains less than 2 hours in circulation. However, formation of the vWF-FVIII complex increases the FVIII circulation time to approximately 8–12 h.18 Fresh frozen plasma (FFP) is the best and most accessible resource of FVIII. The temperature susceptibility of FVIII storage could restrict getting access to the high amount of FFP resource in a difficult situation.19–22 Therefore, improving the half-life of the FVIII factor while trying to maintain its activity and efficacy has a crucial impact on life-threatening disorders such as hemophilia.23 Various types of therapy for extending protein maintenance have been established for more than seven decades for hemophilia disorders including albumin-fusion FVIII and PEGylated factors, and some of them were commercialized and entered the market.24–29 In recent studies, nanomaterial application in extending protein maintenance attracted several interests. Therefore, we will review related articles focusing on nano-based content. Prophylactic treatments, including standard FVIII infusion, basically could prevent frequent bleeding episodes. However, harmful consequences and disadvantages like pain, irreversible joint damage, and difficulties due to short interval infusion could significantly impact patients' life quality since prophylaxis for hemophilia A typically needs at least three times per week to provide a sufficient circulating level of FVIII. There is a critical point related to the immunogenicity of FVIII infusion and producing anti-drug antibodies that are called inhibitors, which neutralize the FVIII activity.30 Evidence shows that plasma-derived FVIIIs are less immunogenic but have more exposure to contamination than recombinant FVIIIs.31 However, recent studies have established the third generation of (recombinant FVIII) rFVIII that is both more efficient and less immunogenic than previous generations. Also, neutralization can be controlled by bypassing the inhibitory activity with activated prothrombin complex concentrates (APCC).32–35
Current hemophilia research is focusing on the emergence of new or developed factor replacement therapies to extend proteins' half-lives. Among these recent studies, covalent binding of FVIII to polyethylene glycol (PEG; PEGylation) had the most citation, and then liposomes and rarely PLGA have been used in related experiments which have been brought out in order.
2.1.1 Poly ethylene glycol (PEG).
Basically, the first reaction of the body against nanoparticle entrance, as a foreign component, is forming a protein complex in a way that NPs are totally surrounded by proteins which is called “protein corona”. Opsonins are proteins that are responsible for opsonization and consequently evoke the antigen presenting cells (APCs) like macrophages for phagocytosis and plasma clearance.36 Poly Ethylene Glycol (PEG) is a hydrophilic and biocompatible polymer that has been successfully established in biotech pharma to modify therapeutic proteins for inducing biocompatibility by anti-opsonization activity and prolonging the circulatory half-life of proteins. Herein, native FVIIIs have been investigated, using enzymatically new (PEG) conjugates (ranging from 10 to 80 kDa), to specifically attach a unique O-linked glycan to the FVIII B-domain. Therefore, the cleaving process, which is done by thrombin, leads to B-domain associated O-glycan PEG release. The activated FVIII (FVIIIa) can generally enter into the coagulation cascade. In vivo and in vitro potency of 10, 20, 40, and 80 kDa PEGylated FVIII have been tested. The results revealed a positive correlation between the half-life of FVIII factor and size of PEG. The study also illustrated that FVIII conjugated with a single-chain 40 kDa turoctocog alfa (N8-GP) had the most efficiency on the prolongation of FVIII in mice and rabbits and less in rats. The biochemical functionality of N8-GP was unchanged while the cellular uptake and in vivo clearance reduced.37
A patent was then investigated, using a B-domain of the factor VIII molecule which has been truncated and particularly coated with a hydrophilic polymer, such as a PEG group.38
Furthermore, similar research studies have been conducted on N8GP-rfVIII in order to prolong the half-life of the protein factor that showed similar results. However, there were no statistical reports in these studies.39,40 Also, in another research, a glyco-PEGylated recombinant FVIII (N8GP) was first tested in a human trial to evaluate the safety pharmacokinetic profiles of N8-GP in comparison with previous FVIII products. Assessments illustrated that the terminal half-life of N8-GP averagely extended to 19.0 h (range: 11.6–27.3 h) or a 1.6-fold increase in plasma survival occurred. It could also be well tolerated without any noticeable safety concern.41
According to the nearly similar studies in 2016 and 2017, in addition to the native FVIII, rFVIII has also been examined to improve the biocompatibility and long-lasting circulation, employing novel PEG derivatives. The recombinant FVIII PEGylated by rurioctacog alfa pegol (BAX 855) in which approximately 60% of the 20 kDa PEG chains were localized to the B-domain showed a development in reducing the injection frequency per week without receiving FVIII inhibitory antibodies or unexpected adverse events. Moreover, turoctocog alfa pegol (N8-GP) was used, pursuing the trial phase III of the previous study. Two groups of patients including typical rFVIII injection and N8-GP treated rFVIII have been assessed, and prophylactic medication was also compared in two groups. According to the research, a single dose of up to 75 IU per kg N8-GP and two intravenous injections were well tolerated by 83.6% and 95.5% of the patients. In conclusion N8-GP could extend the half-life of FVIII (mean, 18.4 h) and prolong the factor plasma activity compared to the patients of previous study and favorably showed safety. No clinically severe concern was reported as well.42–47 Herein, BAY rFVIII has been also implemented.48
Also, PEG-FVIII innovation has been patented by an American assignee with similar developing results.49
2.1.2 Liposomes.
Liposomes are nano-to micro-sized lipid vesicles (approximately 100 nm to upper μm in diameter) composed of two spaces where a phospholipid bilayer has encapsulated the hydrophilic interior.50 Although liposomes have different applications in therapeutic agent delivery, they are rapidly detected by dendritic cells in plasma circulation. In this regard, PEGylation is frequently being used in recent progress to improve the biocompatibility and extend the circulation half-life in bloodstream. Phosphatidyl derivatives are decent candidates used to increase the plasma circulation time in interaction with or without vWF in order to improve pharmacokinetics. For example, synthetic phosphocholine based liposomes (POPC) have been investigated in some research studies, using PEGLip, a new technology for PEGylation. The results revealed that PEGLip specifically bound to plasma-derived FVIII (pd-FVIII) that strongly depended on the presence of PEG polymer.51,52 Besides, it has been proven in two other related investigations that PEGLip pd-FVIII significantly induced faster thrombin generation in preclinical studies and also shown that using PEGLip FVIII led to reducing the prophylactic infusion dose to once-weekly administration (Fig. 4), and it was well tolerated, which resulted in lower bleeding frequency as well.53,54
Phosphatidyl derivatives like phosphatidylserine and phosphatidylinositol are two anionic phospholipids that can convert the immunogenic epitope to immune-tolerogen epitope that prolongs protein circulation against inhibitor development. The promising result of using these materials to reduce the immunostimulatory activity of inhibitors could extend the half-life of FVIII. However, the results indicate that liposomes are being exposed to rapid uptake by the reticuloendothelial system (RES). Hence, PEGylation is a strategy that has been investigated to make longer-acting FVIII.55 PEGylation has beneficial effects on making a barrier against opsonin protein by surface hydration and neutralization of the surface to inhibit opsonin and RES cell attraction. Despite all benefits observed in subcutaneous administration, no positive therapeutic effects have been demonstrated in an intravenous infusion due to liposome PEGylation, using passive PEG transfer.56–58
Moreover, joint research studies presented the efficacy of using B-domain deleted (BDD)-rFVIII conjugated soy phosphatidylinositol lipid nanoparticles in reducing inhibitor development and 1.5-fold and 1.4-fold increases in the terminal half-life of BDD-rFVIII to 18 hours in mouse clinical trials and dogs, respectively. Simultaneously, the immunogenicity assay has been evaluated in the presence of labeled anti IgG as well.59–61 Furthermore, in similar research in 2018, it has been demonstrated that subcutaneous administration of Lys-phosphatidylserine could extend the half-life to 1.6 fold by reducing the immunogenicity.62
There are also several reports on employing different types of lipid nanoparticles (LNP), like lipid nanotubes (LNT) and lipid nanodiscs (LND), to investigate and screen the influence of modifications on the protein structure and function. For example, in empirical research, LNTs, helical organization of FVIII on lipid nanotubes, were used to characterize how a difference in sequence can correlate with structural and functional changes. As a result, the methods described in this article can identify the sequences, which will improve the recombinant FVIII function when modified. Therefore, this can lead to significant clinical implications for drug discovery in both thrombosis and hemostasis fields.63 Also, similar studies were conducted using lipid nanodiscs as delivery systems for FVIII, in which rFVIII was assembled on lipid nanodiscs. The results indicated that this complex had a synergistic effect on hemophilic mice's clotting process.64,65
2.1.3 PLGA.
Poly lactic-co-glycolic acid (PLGA) is a biocompatible copolymer that has been classified as a safe component and licensed by FDA. PLGA has been used in the same PEG related applications in therapeutic procedures. Herein, a research article was published in 2015. Tolerogenic nanoparticles (NPs) were introduced, using developed tolerogenic synthetic vaccine particles (SVP) by incorporating rapamycin in the self-assembling of PLGA to induce immunologic tolerance and avoid the inhibitor development process in protein therapy. Rapamycin is commonly used as an immunosuppressant drug to prevent transplant rejection and immune response, as well as induce tolerance to FIX and FVIII in mouse models. The nanoparticle delivery of rapamycin and antigens to the same APC may facilitate tolerogenic antigen presentation, leading to induction of antigen-specific response of regulatory T cells. Since viral vesicles are quickly taken up by dendritic cells, biocompatible and biodegradable PLGA has been used to prolong the circulation half-life. Tolerogenic nanoparticles instead of FVIII replacement therapy showed potential to improve the efficacy and safety profile of FVIII in the treatment of hemophilia patients, either for managing or prevention of bleeding.66 The results are illustrated in Table 1.
Table 1 FVIII and studied nanomaterials
R
|
Types of NPs |
Size (nm) |
Electrostatic charge |
Effects on plasma derivatives' viability |
Effects on plasma derivatives' function |
Ref. |
(1) Polymeric nanomaterial: PEGylation
|
1 |
O-Glycan PEG-FVIII |
40 kDa |
|
Unfolding effect on vWF, reduced activation in presence of vWF |
Reduce degradation and increase circulation time |
37
|
2 |
B-domain truncated FVIII-PEG |
PEG variants |
|
|
(O-Glycan) BDD-FVIII-280 13 to 19 hours, 1.9–2.1 fold increased |
38
|
2 × 40 kDa PEG BDD-FVIII 40 kDa PEG 280 16 to 20–22 hours, 1.7 fold |
(O-Glycan) BDD-FVIII 80 kDa PEG 280 15 to 21 hours, 1.9 fold |
3 |
PEGylated FVIII variants |
Domain variant PEG |
|
Improved pharmacokinetics and activity, attenuated immune-response |
Extended half-life with 95% efficiency, 2 fold increased |
40
|
4 |
Glyco-PEGylated recombinant FVIII (N8GP) |
|
|
Noticeable reduction in cell clearance without any safety concerns |
Half-life of N8-GP extended to an averagely of 19.0 h (range: 11.6–27.3 h) or a 1.6-fold increase in plasma survival |
41
|
5 |
Rurioctocog alfa pegol (BAX 855, Adynovate®) |
Full-length factor VIII, 20 kDa PEG |
|
|
95.9% bleeds controlled with one or two doses and extended to 1.4–1.5 fold increased |
42
|
6 |
Turoctocog alfa pegol (N8-PG) |
B-domain truncated, 40 kDa PEG |
|
|
Extended the half-life of rFVIII to 18.4 hours |
43
|
7 |
BAX 855 (PEGylated ADVATE™ (rFVIII)) |
20 kDa PEG reagent to FVIII-octocog alfa |
|
No adverse safety concern, no subject developed antibodies to CHO proteins |
1.3–1.5 fold half-life extension, twice-weekly prophylaxis with BAX 855 was safe and efficacious in paediatric with severe haemophilia A |
45
|
8 |
B-domain replaced by sialic acid FVIII conjugated (PEG) |
Branched 40 kDa N8-GP (turoctocog alfa pegol) |
|
|
Subcutaneous administration, prolonged 19 h stability in patients with severe hemophilia A, corresponding to a 1.6-fold prolongation versus standard FVIII |
47
|
9 |
BAY 94-9027 BDDFVIII-PEG |
60 kDa (PEG) molecule site specifically attached via a maleimide linker to a cysteine amino acid |
|
No relevant changes in PK characteristics after multiple doses |
1.4 fold half-life increased |
48
|
![[thin space (1/6-em)]](https://www.rsc.org/images/entities/char_2009.gif) |
(2) Liposomes
|
1 |
PEGLip/pd-FVIII |
DSPE-PEG 2000 (2 kDa) |
|
Reduce the affinity of inhibitor |
Extended circulation and improve survival rate in mice |
51
|
2 |
Conserved peptide associated PEGLip FVIII |
80–100 nm |
|
|
Reduced endothelial uptake |
52
|
3 |
PEGLip pdFVIII |
80–100 |
|
Faster and more efficient thrombin generation |
Enhanced FVIIa haemostatic efficacy 2 h extended half-life |
53 and 54 |
4 |
FVIII–PI |
|
Neg. (−) |
The C2 domain of FVIII; similar binding region with von Willebrand factor within immunodominant epitope |
Protected FVIII from degradation |
56
|
Reduce immunogenicity |
5 |
(FVIII–PI/PEG) |
DMPE-PEG2000 |
|
|
Adverse effect on immunogenicity in intravenous administration due to PEG passive transfer |
57
|
6 |
Soy-PI linked BDD-rFVIII |
|
Negative |
Decrease the electrorepulsion due to B-domain deletion, decrease the inhibitor affinity to attach |
Extended plasma survival and hemostatic efficacy prolong circulation and decrease the immunogenicity |
59–61
|
7 |
Lyso-phosphatidylserine particle |
193.9 ± 69.2 nm |
−27.64 ± 4.00 mV (negative) |
Converting an immunogen to a tolerogen, induction of T cell regulators by |
Reduced anti-FVIII antibody responses, by means of inducing tolerance towards therapeutic proteins. It is not reported quantitatively |
62
|
![[thin space (1/6-em)]](https://www.rsc.org/images/entities/char_2009.gif) |
(3) Lipid nanoparticle (LNP)
|
1 |
FVIII-nanodisc complex |
15 nm |
Negatively charged ND rich in phosphatidylserine (PS) |
Improving procoagulant activity due to shielding effect of ND on FVIII binding site to lip-protein receptors |
Increased the stability and reduce the amount of required FVIII, thus lower the treatment cost |
64 and 65 |
![[thin space (1/6-em)]](https://www.rsc.org/images/entities/char_2009.gif) |
(4) Polymeric nanomaterial: PLGA
|
1 |
PLGA coated SVP-rapamycin encapsulated |
|
|
Inhibited expansion of antigen-specific T cells and induced antigen-specific regulatory T cells or, antigen-specific immune tolerance |
Prevent the immunogenic response and reverse inhibitor formation |
66
|
To sum up, comparing reviewed nanostructures showed that nanosystem-conjugated recombinant FVIII which was covered by derivatives of biocompatible PEG polymers like N8-GP has showed the most effective structure in increasing the circulation time in blood. For example, an international patent (WO) of the o-glycoPEG system and some other research studies have shown the increase of stability up to 19 hours and successful reduction of infusion frequency to just twice a week.40,42,43,49
Generally, improving the stability is related to two mechanisms: first, increasing the circulation time by limiting cell uptake by dendritic cells and hiding from the reticuloendothelial system can be possibly accompanied by PEGylation. Next, the implementation of phosphatidylserine and inositol derivatives such as Lys-PS throughout the liposome PEGylated systems can attenuate immune response by converting immunogenic compounds into tolerogenic and increasing the durability of protein factors.62
Regardless of the current protein function improvement approaches, the state of the art method using mRNA-based gene delivery has been currently investigated. However, probable mutations, high costs, and unknown adverse drawbacks are intensely challenging that restricts its utilization in clinical therapies, and improving the current prophylactic treatment is preferable.67,68
2.2 Immunoglobulins
In 1890, von Behring and Kitasato discovered antibodies in the serum of a rabbit infected by tetanus, claiming that the antibodies had specific functions.17,69–71 Human blood plasma contains an excessive number of globular proteins, specifically Igs known as gamma globulins.72,73 This protein family consists of a wide range of autologous molecules that constitute immune responses in diverse physiological and pathological processes in vertebrate animals.74 Igs or antibodies are glycoprotein molecules with a Y form-like structure that includes two similar domains for recognizing antigens and two similar domains with effector functions (Fig. 3).75 This family is divided into five classes: immunoglobulin G (IgG), IgA, IgM, IgD and IgE, considering their concentration in blood.17,76
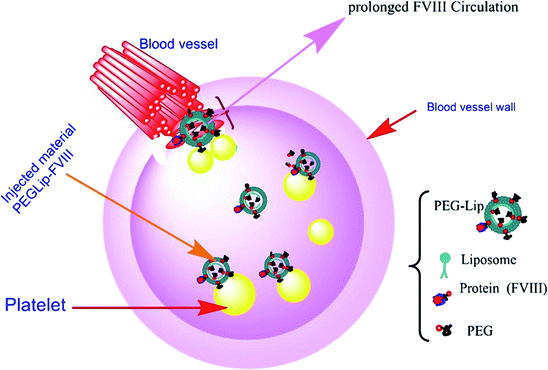 |
| Fig. 3 Image of PEG-linked liposome conjugated rFVIII structure and function. | |
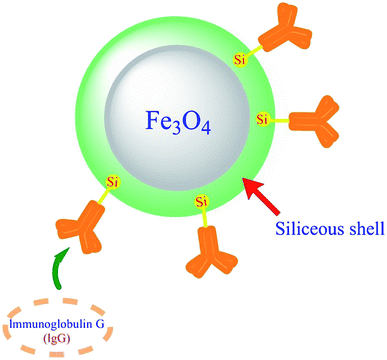 |
| Fig. 4 Schematic image of IgG adsorption and purification from rabbit serum using hybrid magnetic nanoparticles. | |
Igs play a prominent role in medical conditions. They are essential in the diagnostic field and have considerable importance in diverse therapeutic approaches like replacement therapy and immunomodulatory therapy.17,77–79 Moreover, because of their useful characteristics such as high specificity, selectivity, good stability, and solubility, they have been adopted to treat cancer, autoimmune disease, and targeted drug delivery (extracellular or intracellular delivery) in rheumatic, infectious, inflammatory, and neurological conditions.80–83 Human Ig can be used in various modes, for instance, subcutaneous (SCIG), intravenous (IVIG), and intramuscular (IMIG) administration.84–87 However, all administrations mentioned above have their advantages and disadvantages.72,78,88,89 Although there are wide Ig therapeutic approaches for clinical use, applying them in clinical trials is not quite as simple as it might seem. Difficulties are due to challenges in Ig purification and preserving them against aggregation, having a cytotoxic effect in some cases, short half-life, and structural and functional modifications.81,82,90,91 Consequently, since a diverse range of nanostructures have been implemented to optimize the use of Igs, like improving the maintenance and functionality of Ig, protein stabilization, and preventing aggregation, it is intended to gather some reports whereby conjugation and interactions between different Igs and nanostructures were experimented with obtaining a better view of this administration. As we know, any interaction or adsorption might lead to conformational and functional changes in proteins.81,82,92
2.2.1 PEGylation.
In 1977, the first adopted PEG was in conjugation with bovine serum albumin and its immunoprotective effect was confirmed. Since then, applying PEGylation in therapeutic and pharmaceutical approaches has been boosted. It can be used to block enzymatic degradation, increase the safety of therapeutics, and improve the half-life of biomolecules.93,94 It has also been implemented to improve the solubility and reduce the mAb fragments' immunogenicity.94,95 Below, some of these research studies have been reviewed.
PEGylation was applied to improve the stability of antibodies by Roque and coworkers in 2015. The antibody fragment (Fab) was conjugated to 30 kDa PEG and was tested within the aggregating condition which was induced by temperature and mechanical stress. They also considered the structural change of Fab upon its interaction with PEG. The result indicated that PEGylation was an effective method to preserve antibodies in stressed conditions. Although the secondary structure of the protein did not change in the presence of PEG, tertiary structure stability experienced moderate disturbance.95
In addition, an empirical research study has been conducted by Reichard and coworkers in 2016 to enhance the circulation time of antibodies in targeted organs. In order to achieve this purpose, a single chain variable fragment (scFv) has been developed with a terminal cysteine (ScFv7F9Cys) which showed higher affinity for conjugation. In another research ScFv7F9Cys was conjugated to three deferent sizes of PEG: PEG5K, PEG20K, and PEG40K. Also, according to analysis via SDS-PAGE, only one PEG was conjugated to each antibody fragment. Although regarding past research studies all three PEGs could increase the half-life of ScFv, in the mentioned research only 20 kDa PEG was used for conjugation due to the highest binding activity to the experimented drug. In conclusion, PEGylation successfully increased the half-life of ScFv7F9Cys. Similar to previous research, any conformational or functional change of immunoglobulin was not reported. The colloidal stability of the solution illustrated the stability of Igs upon conjugation.93
In 2017, PEG conjugated to an antibody fragment via a site-selective thiol approach was studied to increase the remaining time within the lungs. In this research, the effect of the two-armed 40 kDa PEG conjugate to a murine anti-IL-17A Fab's monomer on the antibody's local half-life in mouse lungs was measured and compared with that in rats and rabbits. As a result, conjugation dramatically increased the residence time of anti-IL-17A Fab' monomer in all three species' lungs and did not affect Ab's binding activity. However, conjugation markedly raised Ab's inhibitory ability in cell culture. Since no noticeable changes in Ig usability have been observed, we can conclude that no considerable alteration happened in the antibody fragment structure.96
2.2.2 Metal NPs.
Metal NPs have been applied in various therapeutic and pharmaceutical approaches, such as anti-bacterial agents, drug-delivery vectors, and biosensors. Due to their particular characteristics like the ease of synthesis, chemical stability, and good conductivity, gold NPs and silver NPs have been applied in biochemistry, medicine, and analytical chemistry. Biological implementation of metal NPs due to their large surface area and free surface energy faces a challenge in maintaining nano-systems' colloidal stability. NPs, as meta-stable systems, excessively tend to aggregate upon physical or chemical stresses. NPs tend to decrease their high surface energy via interaction with other molecules to form protein corona shells or even aggregation. In this respect, a wide range of materials including surfactants, polymers, and proteins have been used to boost particles' colloidal stability in various conditions.97–100
Magnetic particles (MN) are among the metal NPs with a metallic core and various shells. Polymer shells preserve naked magnetic particles against air and aggregation.101 Ig conjugation to MNs due to its specificity has been used to improve Ig purification and recovery or cell detection and isolation.90,102–105 In 2020 a hybrid magnetic NP with an Fe3O4 core coating with a siliceous shell, which was enriched in the polysaccharide κ-carrageenan (HMNPs), has been implemented to achieve the high volume of Ig purification from rabbit serum (Fig. 5). Fortunately, the hybrid achieved its goal and did not alter the secondary structure of immunoglobulin neither upon adsorption on HMNPs nor after the desorption step.106
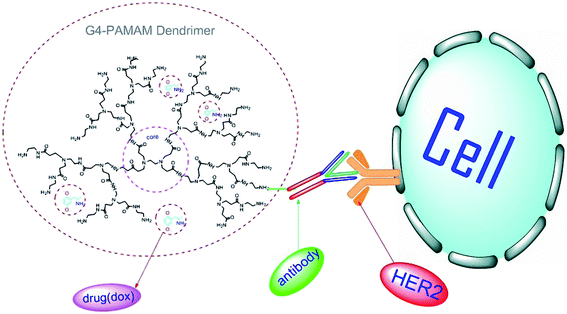 |
| Fig. 5 PAMAM-dox-trastuzumab conjugate, attached to HER-2 receptor. | |
As for other metallic NPs, in 2015 monodispersed IgG-folinate functionalized silver NPs were obtained as bio-nano composites for biological experiments. IgG was selected for protecting silver NPs from aggregation or macrophage recognition in the body. The results showed that AgNP-IgG-CF (AgNP-IgG-calcium folinate) conjugation was successfully functionalized, and no structural/functional changes have been reported.99
Another research on metal nanoparticle-Ab conjugation was published in 2020 in which stable conjugates of antibodies with Au-NPs (GNPs) have been proposed. Mab 2H3C6 is a monoclonal antibody against the protein MPT64 of Mycobacterium tuberculosis, and Mab2H2F6, a monoclonal antibody 2H2F6, against the structural protein of the Sharkey plum virus has been conjugated to GNPs. The results showed that GNPs might aggregate upon interaction with antibodies because of their light chain decay during preparation, storage or conjugation. Although conjugation of GNPs to the fragment antibody which was purified from unstable fragments introduced a stable compound, there is no evidence to support that antibody fragment conjugation with GNPs has negative consequences on Ig stability.97
2.2.3 Dendrimers.
Dendrimers are a relatively new class of highly branched polymers, which have been firstly introduced in the middle of 1970. Their high monodispersity, 3D poly-branched, core–shell like structure, internal cavities to entrap molecules like drugs, and different functional groups on the surface are the main properties that allow them to be employed in various applications as drug delivery, imaging, antiviral and transfecting agents.107–111
PAMAM (polyamidoamine) or PPI (polypropyleneimine) dendrimers are the most prominent dendrimers. Dendrimers are categorized into cationic (e.g., PAMAM-NH2), anionic (e.g., PAMAM-COOH), and neutral (e.g., PAMAM-OH) dendrimers based on their surface functional groups. Surface charge modifications can significantly diminish polymers' toxicity and improve their biocompatibility: the molecule size and number of terminal groups are directly related to dendrimers' generation. Moreover, different protonation patterns will mainly make different structures. PAMAM G4 (4th generation) with a diameter of 45 Å has been demonstrated to have lower cytotoxicity among other larger generations of PAMAM-NH2. So, it is the first candidate as a drug delivery system in comparison with other PAMAM generations.108
In empirical studies, dendrimers have been investigated in pharmacy and medicine. The results illustrated their potential for enhancing the solubility of hydrophobic components in drug-delivery systems. Besides, cationic dendrimers can be decent non-viral vehicles for gene delivery in therapeutic applications. However, toxicity is the main factor that should be considered. Cationic dendrimers have more toxic activity among others. Positive surface charges or groups (such as amine groups) can interact with negatively charged lipid membranes and lead to hole formation. To reduce the mentioned toxic effects, modifications of dendrimers like surface coating with biocompatible polymers like PEG, an acetyl group, or saccharide moiety can significantly reduce the toxicity. In PAMAM dendrimers, amine group substitution with hydroxyl groups seems to reduce the toxicity. Also, they can interact via possible hydrogen bond formation, which mainly occurs in biological reactions.108,109
Several studies have investigated the effect of PAMAM derivatives on different blood components.
In 2018, a PAMAM based delivery system has been developed, using PAMAM dendrimer linked trastuzumab, delivering doxorubicin (dox) to breast cancer cells in which human epidermal growth receptor 2 (HER-2) had been overexpressed on the cellular surface. Trastuzumab is a humanized monoclonal antibody against HER-2 and provides specificity for conjugated structures. The results showed that PAMAM-dox-trastuzumab conjugates were more effective and had less toxicity than PAMAM-trastuzumab conjugates or free doxorubicin (Fig. 6).112 In 2018 PAMAM dendrimer-trastuzumab conjugates have been tested with different drugs, docetaxel (doc) or paclitaxel (ptx), against HER-2-positive SKBR-3 and HER-2-negative MCF-7 by the same team. The results showed the high selectivity of PAMAM-doc-trastuzumab and PAMAM-ptx-trastuzumab conjugates for HER-2-positive cells and confirmed the unity of trastuzumab.113 PAMAM-dendrimer protected the whole conjugate during the process in both types of research, and binding between the dendrimer and trastuzumab did not change the IgG's characteristics.112,113 In 2016, a PAMAM-dendrimer and humanized anti-HER-2 antibody (herceptin) conjugate have been implemented in a nanohybrid structure, which successfully bound specifically to HER-2 overexpressing cells as a targeted nano-imaging agent. The experiments indicate that the herceptin structure and function remained after covalent binding to dendrimers.114 As a result, the PAMAM-dendrimer-immunoglobulin conjugate had satisfactory usage in the targeted compound delivery.
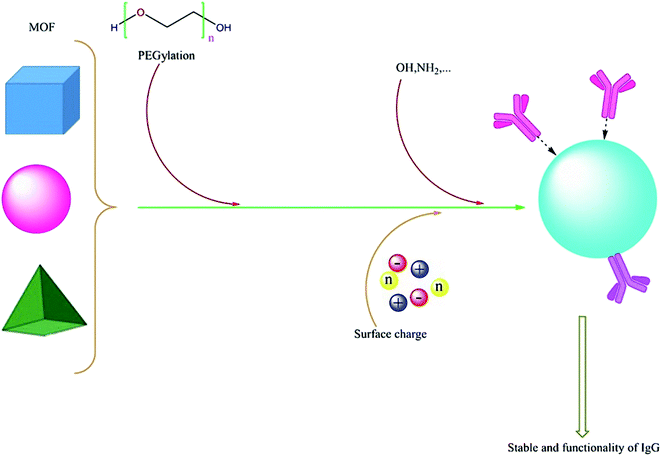 |
| Fig. 6 Co-based MOF system and surface functionalization regulation of the system for specific protein enrichment. | |
2.2.4 PLGA.
As explained before, PLGA has a similar mechanism of action as a coating agent to avoid opsonization and phagocytic attack of macrophages. So, this polymer can be used as a PLGA-PEG dual coating which is capable of enhancing features as well.
2.2.5 Liposomes.
Targeted drug delivery in cancer treatment has shown promising future for the next evolution of liposomal drug carriers. Liposomes have been used in different forms such as antibody-targeted PEGylated liposomal drugs that have showed preferable therapeutic effects over their non-targeted correlates. Interacting with antibodies, by covalent or non-covalent bonds, antibody fragments, peptides or small ligands can enhance the selectivity of liposomes for different diseased cells. Antibody-targeted liposomes usually called immunoliposomes (IL) increase specific binding to tumor-associated internalizing antigens on the surface of cancer cells.115
In 2011, IgG antibody was conjugated to the surface of hepatitis B surface antigen (HBsAg)-loaded liposomes. This in vitro study was focusing on the targeting potential, localization pattern, and immunogenicity of the complex. This conjugation was formed by a glutaraldehyde coupling method. The positive zeta potential of optimized liposomes has not been altered after antibody coupling onto the surface. The coupling of antibodies on the surface of liposomes reduced the release rate of encapsulated antigens. In fact, antibody coupling led to the steric barrier on the surface of liposomes. According to the results and practical structure of the hybrid, we can conclude that the structure and function of IgG were maintained as well.116
2.2.6 Metal–organic frameworks (MOFs).
Metal–organic frameworks (MOFs) are the most recent nanomaterials that are widely employed in research due to their special benefits such as tunable porous and crystalline structures and high surface areas. This nano-scale porous structure is mostly used in separation, catalysis, storage, sensing and gas adsorption, as well as drug delivery. Generally, MOFs are generated by chemical coordination of metal ions and organic linkers to form a hybrid structure. They also have been implemented as a host for biomolecule encapsulation in recent years.117–120
In 2019, a hydrophilic MOF-based nanomaterial UiO@GO@PEG was produced from a zirconium-based MOF UiO-66-NH2 coated with a graphene oxide (GO) layer and hydrophilic polyethylene glycol (PEG) to enhance the stability and hydrophilicity of nano-structures. This hybrid was prepared for adsorbing glycoproteins from complex matrices. Three glycoproteins (IgG, γ-Glo and b-Lf) and five non-glycoproteins (α-La, BSA, Mb, Lys and cyt-c) have been used to prove UiO@GO@PEG functionality in protein adsorption under pH 7. The result showed that the adsorption efficiency of the nanostructure is 96.9% for IgG, 75.9% for γ-Glo, 76.8% for b-Lf that were much higher than those of non-glycoproteins which illustrate selective adsorption for glycoproteins. IgG was recovered with 0.5% (m/v) NH4OH solution with 82.6% efficiency and it was also isolated from human serum as a real biological system to investigate adsorption selectivity. No functional or structural change of IgG has been reported.121
In 2020, regulation of the surface properties like hydrophilicity and charge characteristics of Co-based MOF nanostructures by operating various organic building units was reported. Co-MOF-OH, a MOF coated with abundant hydroxyl groups, showed selective adsorption and enriched efficiency toward IgG at physiological pH (7.4). The enrichment factor for IgG from the IgG/human serum albumin mixture with a mass ratio of 1
:
50 was obtained up to 97.7. The result indicated that the structure of IgG did not experience any changes during the enrichment process and practicability of Co-MOF-OH in a complex biological solution was confirmed by the selective enrichment of IgG from human serum samples.122
The results are listed in Table 2.
Table 2 Immunoglobulins and nanomaterials
R
|
Types of NPs |
Size (nm) |
Electrostatic charge |
Effects on structure of Ig |
Effects on function of Ig |
Ref. |
(1) Polymeric NPs: PEG
|
1 |
PEG |
20 kDa |
No charge |
No change |
Increased half-life |
93
|
2 |
PEG |
10.7 nm/40 kDa |
No charge |
No change |
Increased half-life |
96
|
3 |
PEG |
30 kDa |
No charge |
Moderate disturbance in tertiary structure |
Ig increased hybrid affinity |
95
|
![[thin space (1/6-em)]](https://www.rsc.org/images/entities/char_2009.gif) |
(2) Metal NPs
|
1 |
Gold NPs (AuNPs) |
24.9 nm |
Negative (citrate) |
No change |
No change |
97
|
2 |
Silver NPs (AgNPs) |
26 nm |
Negative (citrate) |
No change |
No change |
99
|
3 |
Iron NPs |
Core: 48.9 (±5.9) |
−25 mV |
No change in secondary structure |
High purification of Ig adsorption yield: 90% |
106
|
Shell thickness: 20.7 (±2.3) |
4 |
Iron oxide |
Hydrodynamic size: 33 nm |
−51 mV |
No changes |
No changes |
105
|
![[thin space (1/6-em)]](https://www.rsc.org/images/entities/char_2009.gif) |
(3) Polymeric NPs: dendrimer
|
1 |
PAMAM dendrimers |
PAMAM G4 dendrimer |
Positive |
No change |
No change |
112 and 113 |
2 |
PAMAM dendrimers |
PAMAM G5 dendrimer |
459.0 ± 28 |
No change |
No change |
114
|
![[thin space (1/6-em)]](https://www.rsc.org/images/entities/char_2009.gif) |
(4) Polymeric NPs: PLGA
|
1 |
PLGA |
221–252 nm |
No charge |
No change |
Ig functionality maintained after released |
81
|
2 |
PLGA |
−<10 μm |
No charge |
No change |
Ig functionality maintained after encapsulation |
92
|
![[thin space (1/6-em)]](https://www.rsc.org/images/entities/char_2009.gif) |
(5) Liposome and LNP
|
1 |
Liposome |
360.23 ± 15.11 |
+8.23 ± 0.51 mV |
|
|
116
|
![[thin space (1/6-em)]](https://www.rsc.org/images/entities/char_2009.gif) |
(6) Metal–organic frameworks (MOFs)
|
1 |
MOF (UiO@GO@PEG hybrid) |
500 to 600 nm |
−29.6 mV |
No change |
No change |
121
|
2 |
Co-based MOF |
Hybrid system > 10 μ |
−40 mV |
No change |
No change |
122
|
Herein, we have mainly considered immunoglobulin usage in different therapeutic areas: treatment of cancer, as transporters of toxins or radiolabelled isotopes to cancerous cells, and also as anti-autoimmune agents for treatment of autoimmune diseases and neurological disorders, in which Ig has been conjugated to a nanostructure.106 In this review NPs have been implemented on antibody or antibody fragments upon interaction.
Noticing the bio-conjugations as mentioned earlier, we can see moderate behavior for Igs upon interaction with NPs. Ig has mostly been used due to its higher specificity in drug delivery systems or cell isolation.104 In conjugation to metal NPs, magnetic NPs had the most common implementation regarding antibodies' most important applications: bio-separation and purification purposes.90
Additionally, using other metal NPs, the well-functionalized structure and good colloidal stability were investigated. Encapsulation of metal nanoparticles via bio-compatible materials plays a critical role in both colloidal and Igs' structural and functional strength.99,123
MOF nanostructures have different applications in interaction with Igs. Purification and enrichment of immunoglobulin G have been explained before, which demonstrated that noncovalent interaction between Igs and MOFs does not change the protein stability and function of Igs during the enrichment process.121,122
In the field of targeted drug delivery, Igs conjugated with dendrimers, particularly PAMAM-dendrimers, were used in various studies. The results illustrated that the stability of antibodies was maintained within conjugation.112–114
Likewise, liposomes are mostly used in targeted drug delivery and their successful function for delivering molecules without affecting their stability has been confirmed. Similar to using dendrimers, Igs have been implemented to targeting liposomes.115,116
PEGylation has shown promising results in increasing the half-life of antibodies and antibody fragments during therapy in the polymeric nanostructure.93,96 PEGylation causes prolonged systemic exposure via decreasing the amount of proteolysis and renal elimination. It also increases solubility and reduces immunogenicity.80 Moreover, it successfully protects the Ig structure in unfavorable conditions.95
3. Conclusion
During the last few decades, bio-system applications in medical and pharmaceutical approaches have been developed. Nowadays, several types of research are conducted based on macromolecules containing antibodies, recombinant proteins, and nucleic-acid-based products after a long time focusing on small molecules as biopharmaceuticals.
According to the innovation aspect of the article, it is worth mentioning that this is the first time that reports from 2010 to 2020, dealing with the nanostructures' effect on plasma protein stability, have been reviewed and collected. To conclude, the results revealed that nano-based systems with PEG derivatives have the best improvement. Also, utilizing PEGylated liposomes, using the PEG-lip technique for coating nanostructures, leads to FIIIV half-life prolongation. This however is the only research that has been reported about PLGA coating.
Among different nanostructures reviewed in this study, including metal NPs (GNPs, AgNPs, and magnetic NPs), dendrimers, PEG, and PLGA, choosing the best nanoparticle for conjugation with diverse Igs depends on the impact of nanomaterials on the stability of the structure and consequently function of Igs indeed. We should select a suitable nanostructure according to the implementation of Ig conjugated to the nanoparticle. Reviewing the last decade's papers, iron oxide magnetic nanoparticles have demonstrated proper function in the separation and isolation of stem cells or other cells and Ig purification from plasma. Liposomes and dendrimers are suitable vehicles for targeted drug delivery. PLGA and PEG have been used for encapsulating Ig and protect it against aggregation, phagocytosis, and reduce toxicity or immunogenicity. These properties make PEG and PLGA suitable for coating other nanostructures and nano-hybrid structures regardless of their application. Moreover, PEG has been considered as a stabilizer for Ig in the stressed situation to enhance Ig's half-life in targeted tissues. Fortunately, no dramatic structural changes and loss of Ig functionality in conjugation with NPs have already been reported. MOFs are the recent applicable nanomaterials that have been frequently used in different studies. However, further investigations should be implemented for safety validation in biomedical applications. In conclusion, the results showed that MOFs are promising hybrid systems that can promote the usage of nanomaterials in the near future.
Conflicts of interest
There are no conflicts to declare.
References
- T. Zadeh Mehrizi, A. Khamesipour, M. Shafiee Ardestani, H. Ebrahimi Shahmabadi, M. Haji Molla Hoseini and N. Mosaffa,
et al., Comparative analysis between four model nanoformulations of amphotericin B-chitosan, amphotericin B-dendrimer, betulinic acid-chitosan and betulinic acid-dendrimer for treatment of Leishmania major: real-time PCR assay plus, Int. J. Nanomed., 2019, 14, 7593 CrossRef PubMed
.
- T. Zadeh Mehrizi, N. Mosaffa, M. Shafiee Ardestani, A. Khamesipour, H. Ebrahimi Shahmabadi and M. Pirali Hamedani,
et al., In vivo therapeutic effects of four synthesized antileishmanial nanodrugs in the treatment of Leishmaniasis, Arch. Clin. Infect. Dis., 2018, 13(5), e80314 Search PubMed
.
- T. Zadeh Mehrizi, M. Pirali Hamedani, H. Ebrahimi Shahmabadi, M. Mirzaei, M. Shafiee Ardestani and M. Haji Molla Hoseini,
et al., Effective materials of medicinal plants for leishmania treatment in vivo environment, J. Med. Plants, 2020, 19, 39–62 CrossRef
.
- T. Zadeh Mehrizi, M. Shafiee Ardestani, M. Haji Molla Hoseini, A. Khamesipour, N. Mosaffa and A. Ramezani, Novel nanosized chitosan-betulinic acid against resistant Leishmania major and first clinical observation of such parasite in kidney, Sci. Rep., 2018, 8(1), 1–19 CrossRef CAS PubMed
.
- T. Zadeh Mehrizi, M. Shafiee Ardestani, M. Haji Molla Hoseini, A. Khamesipour, N. Mosaffa and A. Ramezani, Novel nano-sized chitosan amphotericin B formulation with considerable improvement against Leishmania major, Nanomedicine, 2018, 13(24), 3129–3147 CrossRef PubMed
.
-
M. A. Laffan and R. A. Manning, Investigation of Haemostasis, in Dacie and Lewis Practical Haematology, ed. J. Barbara, I. B. Bain and M. A. Laffan, Elsevier, 2017, pp. 366–409 Search PubMed
.
- G. Bhopale and R. Nanda, Blood coagulation factor VIII: an overview, J. Biosci., 2003, 28(6), 783–789 CrossRef CAS PubMed
.
- K. W. Eldin and J. Teruya, Blood components for hemostasis, Lab. Med., 2012, 43(6), 237–244 CrossRef
.
- B. S. Bannow, M. Recht, C. Négrier, C. Hermans, E. Berntorp and H. Eichler,
et al., Factor VIII: long-established role in haemophilia A and emerging evidence beyond haemostasis, Blood Rev., 2019, 35, 43–50 CrossRef PubMed
.
- N. Orlova, S. Kovnir, I. Vorobiev, A. Gabibov and A. Vorobiev, Blood clotting factor VIII: from evolution to therapy, Acta Naturae, 2013, 5(2), 17 CrossRef
.
- J. S. Powell, Recombinant factor VIII in the management of hemophilia A: current use and future promise, Ther. Clin. Risk Manage., 2009, 5, 391 CrossRef CAS PubMed
.
-
B. Gangadharan, Structural and functional aspects of factor viii in the initiation of the anti-factor viii immune response, Paris, 2014, vol. 6 Search PubMed
.
-
P. M. Manuel Carcao and L. David, Hemophilia A and B, in Hematology, ed. R. Hoffman, E. J. Benz, L. E. Silberstein, H. E. Heslop, J. I. Weitz, J. Anastasi, M. E. Salama and S. A. Abutalib, Elsevier, seven edn, 2018, pp. 2001–22 Search PubMed
.
- L. A. Cafuir and C. L. Kempton, Current and emerging factor VIII replacement products for hemophilia A, Ther. Adv. Hematol., 2017, 8(10), 303–313 CrossRef CAS PubMed
.
- L. Aledort, R. Ljung, K. Mann and S. Pipe, Factor VIII therapy for hemophilia A: current and future issues, Expert Rev. Hematol., 2014, 7(3), 373–385 CrossRef CAS PubMed
.
-
C. M. K. Mariasanta Napolitano, Hemophilia A and Hemophilia B, in Consultative Hemostasis and Thrombosis, ed. C. S. Kitchens, C. M. Kessler, B. A. Konkle, M. B. Streiff and D. A. Garcia, fourth edn, Elsevier, 2019, pp. 39–58 Search PubMed
.
- Available from: https://professionaleducation.blood.ca/en/immune-globulin-products.
- L. Graf, Extended half-life factor VIII and factor IX preparations, Transfus. Med. Hemotherapy, 2018, 45(2), 86–91 CrossRef PubMed
.
- R. Cardigan, P. Van der Meer, C. Pergande, P. Cookson, B. Baumann-Baretti and J. Cancelas,
et al., Coagulation factor content of plasma produced from whole blood stored for 24 hours at ambient temperature: results from an international multicenter BEST collaborative study, Transfusion, 2011, 51, 50S–57S CrossRef CAS PubMed
.
- A. Bertling, M. F. Brodde, M. Visser, J. Treffon, M. Fennen and A. C. Fender,
et al., Components in Plasma-Derived Factor VIII, But Not in Recombinant Factor VIII Downregulate Anti-Inflammatory Surface Marker CD163 in Human Macrophages through Release of CXCL4 (Platelet Factor 4), Transfus. Med. Hemotherapy, 2017, 44(5), 351–357 CrossRef PubMed
.
- E. O'Neill, J. Rowley, M. Hansson-Wicher, S. McCarter, G. Ragno and C. Valeri, Effect of 24-hour whole-blood storageon plasma clotting factors, Transfusion, 1999, 39(5), 488–491 CrossRef PubMed
.
- L. Feng, Y. Zhao, H. Zhao and Z. Shao, Effects of storage time and temperature on coagulation tests and factors in fresh plasma, Sci. Rep., 2014, 4(1), 1–5 Search PubMed
.
- P. M. Mannucci, Hemophilia therapy: the future has begun, Haematologica, 2020, 105(3), 545 CrossRef CAS PubMed
.
- M. Schiavoni, M. Napolitano, G. Giuffrida, A. Coluccia, S. Siragusa and V. Calafiore,
et al., Status of recombinant factor VIII concentrate treatment for hemophilia a in Italy: characteristics and clinical benefits, Front. Med., 2019, 6, 261 CrossRef PubMed
.
- K. Lieuw, Many factor VIII products available in the treatment of hemophilia A: an embarrassment of riches?, J. Blood Med., 2017, 8, 67 CrossRef CAS PubMed
.
- R. J. Kaufman and J. S. Powell, Molecular approaches for improved clotting factors for hemophilia, Blood, 2013, 122(22), 3568–3574 CrossRef CAS PubMed
.
- M. Laffan, New products for the treatment of haemophilia, Br. J. Haematol., 2016, 172(1), 23–31 CrossRef PubMed
.
- S. Schulte, Innovative coagulation factors: albumin fusion technology and recombinant single-chain factor VIII, Thromb. Res., 2013, 131, S2–S6 CrossRef CAS PubMed
.
- E. Saenko and S. W. Pipe, Strategies towards a longer acting factor VIII, Haemophilia, 2006, 12, 42–51 CrossRef CAS PubMed
.
- M. Ing, N. Gupta, M. Teyssandier, B. Maillère, M. Pallardy and S. Delignat,
et al., Immunogenicity of long-lasting recombinant factor VIII products, Cell. Immunol., 2016, 301, 40–48 CrossRef CAS PubMed
.
- L. A. Valentino and V. M. Oza, Blood safety and the choice of anti-hemophilic factor concentrate, Pediatr. Blood Cancer, 2006, 47(3), 245–254 CrossRef PubMed
.
- J. Lai, C. Hough, J. Tarrant and D. Lillicrap, Biological considerations of plasma-derived and recombinant factor VIII immunogenicity, Blood, 2017, 129(24), 3147–3154 CrossRef CAS PubMed
.
- A. Gringeri, Factor VIII safety: plasma-derived versus recombinant products, Blood Transfus., 2011, 9(4), 366 Search PubMed
.
-
E. M. Agency, Report of expert meeting on factor VIII products and inhibitor development, 2007 Search PubMed
.
- H. M. van den Berg, Different impact of factor VIII products on inhibitor development?, Thromb. J., 2016, 14(1), 55–58 Search PubMed
.
- D. Pozzi, V. Colapicchioni, G. Caracciolo, S. Piovesana, A. L. Capriotti and S. Palchetti,
et al., Effect of polyethyleneglycol (PEG) chain length on the bio–nano-interactions between PEGylated lipid nanoparticles and biological fluids: from nanostructure to uptake in cancer cells, Nanoscale, 2014, 6(5), 2782–2792 RSC
.
- H. R. Stennicke, M. Kjalke, D. M. Karpf, K. W. Balling, P. B. Johansen and T. Elm,
et al., A novel B-domain O-glyco PEGylated FVIII (N8-GP) demonstrates full efficacy and prolonged effect in hemophilic mice models, Blood, 2013, 121(11), 2108–2116 CrossRef CAS PubMed
.
-
G. Bolt, B. B. S. Vandahl, L. Thim, H. R. Stennicke, T. D. Steenstrup and S. DeFrees. Novo Nordisk, Conjugated factor VIII molecules, US Pat., US9150848B2, 2015 Search PubMed
.
- A. Pastoft, M. Ezban, M. Tranholm, J. Lykkesfeldt and B. Lauritzen, Prolonged effect of a new O-glycoPEGylated FVIII (N8-GP) in a murine saphenous vein bleeding model, Haemophilia, 2013, 19(6), 913–919 CrossRef CAS PubMed
.
- B. Mei, C. Pan, H. Jiang, H. Tjandra, J. Strauss and Y. Chen,
et al., Rational design of a fully active, long-acting PEGylated factor VIII for hemophilia A treatment, Blood, 2010, 116(2), 270–279 CrossRef CAS PubMed
.
- A. Tiede, B. Brand, R. Fischer, K. Kavakli, S. Lentz and T. Matsushita,
et al., Enhancing the pharmacokinetic properties of recombinant factor VIII: first-in-human trial of glyco PEGylated recombinant factor VIII in patients with hemophilia A, J. Thromb. Haemostasis, 2013, 11(4), 670–678 CrossRef CAS PubMed
.
- B. A. Konkle, O. Stasyshyn, P. Chowdary, D. H. Bevan, T. Mant and M. Shima,
et al., Pegylated, full-length, recombinant factor VIII for prophylactic and on-demand treatment of severe hemophilia A, Blood, 2015, 126(9), 1078–1085 CrossRef CAS PubMed
.
- P. Giangrande, T. Andreeva, P. Chowdary, S. Ehrenforth, H. Hanabusa and F. W. Leebeek,
et al., Clinical evaluation of glyco PEGylated recombinant FVIII: efficacy and safety in severe haemophilia A, Thromb. Haemostasis, 2017, 117(2), 252–261 CrossRef PubMed
.
- T. T. Wynn and B. Gumuscu, Potential role of a new PEGylated recombinant factor VIII for hemophilia A, J. Blood Med., 2016, 7, 121 CrossRef CAS PubMed
.
- E. Mullins, O. Stasyshyn, M. Alvarez-Roman, D. Osman, R. Liesner and W. Engl,
et al., Extended half-life pegylated, full-length recombinant factor VIII for prophylaxis in children with severe haemophilia A, Haemophilia, 2017, 23(2), 238–246 CrossRef CAS PubMed
.
- S. Meunier, J. Alamelu, S. Ehrenforth, H. Hanabusa, F. A. Karim and K. Kavakli,
et al., Safety and efficacy of a glycoPEGylated rFVIII (turoctocog alpha pegol, N8-GP) in paediatric patients with severe haemophilia A, Thromb. Haemostasis, 2017, 117(9), 1705–1713 CrossRef PubMed
.
- F. Rode, K. Almholt, M. Petersen, M. Kreilgaard, M. Kjalke and D. Karpf,
et al., Preclinical pharmacokinetics and biodistribution of subcutaneously administered glyco PEGylated recombinant factor VIII (N8-GP) and development of a human pharmacokinetic prediction model, J. Thromb. Haemostasis, 2018, 16(6), 1141–1152 CrossRef CAS PubMed
.
- A. Shah, T. Coyle, S. Lalezari, K. Fischer, B. Kohlstaedde and H. Delesen,
et al., BAY 94-9027, a PEGylated recombinant factor VIII, exhibits a prolonged half-life and higher area under the curve in patients with severe haemophilia A: comprehensive pharmacokinetic assessment from clinical studies, Haemophilia, 2018, 24(5), 733–740 CrossRef CAS PubMed
.
-
J. S. V. G. Turecek and Baxalta GmbH Baxalta Inc., Pegylated factor VIII, US pat., US7683158B2, 2010 Search PubMed
.
- J. Shahabi, H. Ebrahimi Shahmabadi, S. E. Alavi, F. Movahedi, M. Koohi Moftakhari Esfahani and T. Zadeh Mehrizi,
et al., Effect of gold nanoparticles on properties of nanoliposomal hydroxyurea: an in vitro study, Indian J. Clin. Biochem., 2014, 29(3), 315–320 CrossRef CAS PubMed
.
- I. Dayan, M. Robinson and M. Baru, Enhancement of haemostatic efficacy of plasma-derived FVIII by formulation with PEGylated liposomes, Haemophilia, 2009, 15(5), 1006–1013 CrossRef CAS PubMed
.
- R. Yatuv, M. Robinson, I. Dayan and M. Baru, Enhancement of the efficacy of therapeutic proteins by formulation with PEGylated liposomes; a case of FVIII, FVIIa and G-CSF, Expert Opin. Drug Delivery, 2010, 7(2), 187–201 CrossRef CAS PubMed
.
- J. Spira, O. Plyushch, N. Zozulya, R. Yatuv, I. Dayan and A. Bleicher,
et al., Safety, pharmacokinetics and efficacy of factor VIIa formulated with PEGylated liposomes in haemophilia A patients with inhibitors to factor VIII–an open label, exploratory, cross-over, phase I/II study, Haemophilia, 2010, 16(6), 910–918 CrossRef CAS PubMed
.
- J. Spira, O. Plyushch, T. Andreeva, V. Zorenko, N. Zozulya and I. Velichkoi,
et al., Safety and efficacy of a long-acting liposomal formulation of plasma-derived factor VIII in haemophilia A patients, Br. J. Haematol., 2012, 158(1), 149–152 CrossRef CAS PubMed
.
- G. Di Minno, A. Cerbone, A. Coppola, E. Cimino, M. Di Capua and F. Pamparana,
et al., Longer-acting factor VIII to overcome limitations in haemophilia management: the PEGylated liposomes formulation issue, Haemophilia, 2010, 16, 2–6 CrossRef CAS PubMed
.
- A. Peng, R. M. Straubinger and S. V. Balu-Iyer, Phosphatidylinositol containing lipidic particles reduces immunogenicity and catabolism of factor VIII in hemophilia a mice, AAPS J., 2010, 12(3), 473–481 CrossRef CAS PubMed
.
- A. Peng, M. P. Kosloski, G. Nakamura, H. Ding and S. V. Balu-Iyer, PEGylation of a factor VIII–phosphatidylinositol complex: pharmacokinetics and immunogenicity in hemophilia a mice, AAPS J., 2012, 14(1), 35–42 CrossRef CAS PubMed
.
- M. P. Kosloski, D. S. Pisal, D. E. Mager and S. V. Balu-Iyer, Nonlinear pharmacokinetics of factor VIII and its phosphatidylinositol lipidic complex in hemophilia A mice, Biopharm. Drug Dispos., 2014, 35(3), 154–163 CrossRef CAS PubMed
.
- K. A. Shetty, M. P. Kosloski, D. E. Mager and S. V. Balu-Iyer, Soy phosphatidylinositol containing nanoparticle prolongs hemostatic activity of B-domain deleted factor VIII in hemophilia A mice, J. Pharm. Sci., 2015, 104(2), 388–395 CrossRef CAS PubMed
.
- K. A. Shetty, E. P. Merricks, R. Raymer, N. Rigsbee, T. C. Nichols and S. V. Balu-Iyer, Soy Phosphatidylinositol–Containing Lipid Nanoparticle Prolongs the Plasma Survival and Hemostatic Efficacy of B-domain–Deleted Recombinant Canine Factor VIII in Hemophilia A Dogs, J. Pharm. Sci., 2016, 105(8), 2459–2464 CrossRef CAS PubMed
.
- K. A. Shetty, M. P. Kosloski, D. E. Mager and S. V. Balu-Iyer, Factor VIII associated with lipidic nanoparticles retains efficacy in the presence of anti-factor VIII antibodies in hemophilia A mice, Biopharm. Drug Dispos., 2016, 37(7), 409–420 CrossRef CAS PubMed
.
- F. Y. Glassman and S. V. Balu-Iyer, Subcutaneous administration of Lyso-phosphatidylserine nanoparticles induces immunological tolerance towards factor VIII in a hemophilia A mouse model, Int. J. Pharm., 2018, 548(1), 642–648 CrossRef CAS PubMed
.
- J. Miller, D. Dalm, A. Y. Koyfman, K. Grushin and S. Stoilova-McPhie, Helical organization of blood coagulation factor VIII on lipid nanotubes, J. Visualized Exp., 2014,(88), e51254 Search PubMed
.
- K. Csencsits-Smith, K. Grushin and S. Stoilova-McPhie, Binding of factor VIII to lipid nanodiscs increases its clotting function in a mouse model of hemophilia a, J. Blood Disord. Transfus., 2015, 6(6), 325–345 Search PubMed
.
- K. Grushin, J. Miller, D. Dalm and S. Stoilova-McPhie, Factor VIII organisation on nanodiscs with different lipid composition, Thromb. Haemostasis, 2015, 113(4), 741–749 CrossRef PubMed
.
- A.-H. Zhang, R. J. Rossi, J. Yoon, H. Wang and D. W. Scott, Tolerogenic nanoparticles to induce immunologic tolerance: prevention and reversal of FVIII inhibitor formation, Cell. Immunol., 2016, 301, 74–81 CrossRef CAS PubMed
.
-
S. D. Hortelano, Immunomodulatory nucleic acid composition comprising chitosan nanoparticles, 2012 Search PubMed
.
- C.-Y. Chen, D. M. Tran, A. Cavedon, X. Cai, R. Rajendran and M. J. Lyle,
et al., Treatment of hemophilia A using factor VIII messenger RNA lipid nanoparticles, Mol. Ther.--Nucleic Acids, 2020, 20, 534–544 CrossRef CAS PubMed
.
- J. A. Hooper, The history and evolution of immunoglobulin products and their clinical indications, LymphoSign J., 2015, 2(4), 181–194 CrossRef
.
- A. Farrugia and I. Quinti, Manufacture of immunoglobulin products for patients with primary antibody deficiencies–the effect of processing conditions on product safety and efficacy, Front. Immunol., 2014, 5, 665 Search PubMed
.
- H.-P. Hartung, Advances in the understanding of the mechanism of action of IVIg, J. Neurol., 2008, 255(3), 3–6 CrossRef CAS PubMed
.
-
P. F. Strengers, Clinical
Application of Plasma Derived Medicines: Current Situation And Future Trend, 2011 Search PubMed
.
- Y. Guo, X. Tian, X. Wang and Z. Xiao, Adverse effects of immunoglobulin therapy, Front. Immunol., 2018, 9, 1299 CrossRef PubMed
.
- A. F. Barahona Afonso and C. M. P. João, The production processes and biological effects of intravenous immunoglobulin, Biomolecules, 2016, 6(1), 15 CrossRef PubMed
.
- M. Arruebo, M. Valladares and Á. González-Fernández, Antibody-conjugated nanoparticles for biomedical applications, J. Nanomater., 2009, 2009, 1–24 CrossRef
.
- F. A. Bonilla, Intravenous and subcutaneous immunoglobulin G replacement therapy, Allergy Asthma Proc., 2016, 426–431 CrossRef PubMed
.
- J. Lamoureux, E. Aubin and R. Lemieux, Autoantibodies purified from therapeutic preparations of intravenous immunoglobulins (IVIg) induce the formation of autoimmune complexes in normal human serum: a role in the in vivo mechanisms of action of IVIg?, Int. Immunol., 2004, 16(7), 929–936 CrossRef CAS PubMed
.
- A. Hawe, J. C. Kasper, W. Friess and W. Jiskoot, Structural properties of monoclonal antibody aggregates induced by freeze–thawing and thermal stress, Eur. J. Pharm. Sci., 2009, 38(2), 79–87 CrossRef CAS PubMed
.
- E. E. Perez, J. S. Orange, F. Bonilla, J. Chinen, I. K. Chinn and M. Dorsey,
et al., Update on the use of immunoglobulin in human disease: a review of evidence, J. Allergy Clin. Immunol., 2017, 139(3), S1–S46 CrossRef CAS PubMed
.
- L. J. Chan, D. B. Ascher, R. Yadav, J. r. B. Bulitta, C. C. Williams and C. J. Porter,
et al., Conjugation of 10 kDa linear PEG onto trastuzumab Fab' is sufficient to significantly enhance lymphatic exposure while preserving in vitro biological activity, Mol. Pharmaceutics, 2016, 13(4), 1229–1241 CrossRef CAS PubMed
.
- A. Gdowski, A. Ranjan, A. Mukerjee and J. Vishwanatha, Development of biodegradable nanocarriers loaded with a monoclonal antibody, Int. J. Mol. Sci., 2015, 16(2), 3990–3995 CrossRef CAS PubMed
.
- A. Ortiz-Dosal, E. Loredo-García, A. G. Álvarez-Contreras, J. M. Núñez-Leyva, L. C. Ortiz-Dosal and E. S. Kolosovas-Machuca, Determination of the Immunoglobulin G Spectrum by Surface-Enhanced Raman Spectroscopy Using Quasispherical Gold Nanoparticles, J. Nanomater., 2021, 2021, 1–6 CrossRef
.
- V. Lenders, X. Koutsoumpou, A. Sargsian and B. B. Manshian, Biomedical nanomaterials for immunological applications: ongoing research and clinical trials, Nanoscale Adv., 2020, 2(11), 5046–5089 RSC
.
- L. A. Dourmishev, D. V. Guleva and L. G. Miteva, Intravenous immunoglobulins: mode of action and indications in autoimmune and inflammatory dermatoses, Int. J. Inflammation, 2016, 2016, 1–6 CrossRef PubMed
.
- H. Shabaninejad, A. Asgharzadeh, N. Rezaei and A. Rezapoor, A comparative study of intravenous immunoglobulin and subcutaneous immunoglobulin in adult patients with primary immunodeficiency diseases: a systematic review and meta-analysis, Expert Rev. Clin. Immunol., 2016, 12(5), 595–602 CrossRef CAS PubMed
.
- P. J. Späth, G. Granata, F. La Marra, T. W. Kuijpers and I. Quinti, On the dark side of therapies with immunoglobulin concentrates: the adverse events, Front. Immunol., 2015, 6, 11 CrossRef PubMed
.
- U. E. Nydegger and S. P. Hauser, Use of intravenous immunoglobulins in haematological disorders, Clinical Immunotherapeutics, 1996, 5(6), 465–485 CrossRef
.
- M. Radosevich and T. Burnouf, Intravenous immunoglobulin G: trends in production methods, quality control and quality assurance, Vox Sang., 2010, 98(1), 12–28 CrossRef CAS PubMed
.
- M. Azimi, A. Aghamohammadi, H. D. Ochs and N. Rezaei, Soluble molecules in intravenous immunoglobulin: benefits and limitations, Expert Rev. Clin. Immunol., 2016, 12(2), 99–101 CrossRef CAS PubMed
.
- V. L. Dhadge, A. Hussain, A. M. Azevedo, R. Aires-Barros and A. C. Roque, Boronic acid-modified magnetic materials for antibody purification, J. R. Soc., Interface, 2014, 11(91), 20130875 CrossRef PubMed
.
-
F. Sousa, P. Fonte, A. Cruz, P. J. Kennedy, I. M. Pinto and B. Sarmento, Polyester-based nanoparticles for the encapsulation of monoclonal antibodies, Recombinant glycoprotein production, Springer, 2018, pp. 239–253 Search PubMed
.
- A. Arrighi, S. Marquette, C. Peerboom, L. Denis, J. Goole and K. Amighi, Development of PLGA microparticles with high immunoglobulin G-loaded levels and sustained-release properties obtained by spray-drying a water-in-oil emulsion, Int. J. Pharm., 2019, 566, 291–298 CrossRef CAS PubMed
.
- E. E. Reichard, N. Nanaware-Kharade, G. A. Gonzalez, S. Thakkar, S. M. Owens and E. C. Peterson, PEGylation of a high-affinity anti-(+) methamphetamine single chain antibody fragment extends functional half-life by reducing clearance, Pharm. Res., 2016, 33(12), 2954–2966 CrossRef CAS PubMed
.
- H. Pan, J. Liu, W. Deng, J. Xing, Q. Li and Z. Wang, Site-specific PEGylation of an anti-CEA/CD3 bispecific antibody improves its antitumor efficacy, Int. J. Nanomed., 2018, 13, 3189 CrossRef CAS
.
- C. Roque, A. Sheung, N. Rahman and S. F. Ausar, Effect of polyethylene glycol conjugation on conformational and colloidal stability of a monoclonal antibody antigen-binding fragment (Fab'), Mol. Pharmaceutics, 2015, 12(2), 562–575 CrossRef CAS
.
- D. Freches, H. P. Patil, M. M. Franco, C. Uyttenhove, S. Heywood and R. Vanbever, PEGylation prolongs the pulmonary retention of an anti-IL-17A Fab' antibody fragment after pulmonary delivery in three different species, Int. J. Pharm., 2017, 521(1–2), 120–129 CrossRef CAS
.
- D. V. Sotnikov, I. V. Safenkova, A. V. Zherdev, V. G. Avdienko, I. V. Kozlova and S. S. Babayan,
et al., A Mechanism of Gold Nanoparticle Aggregation by Immunoglobulin G Preparation, Appl. Sci., 2020, 10(2), 475 CrossRef CAS
.
- M. A. Hamaly, S. R. Abulateefeh, K. M. Al-Qaoud and A. M. Alkilany, Freeze-drying of monoclonal antibody-conjugated gold nanorods: colloidal stability and biological activity, Int. J. Pharm., 2018, 550(1–2), 269–277 CrossRef CAS PubMed
.
- C. T. Matea, T. Mocan, F. Zaharie, C. Iancu and L. Mocan, A novel immunoglobulin G monolayer silver bio-nanocomposite, Chem. Cent. J., 2015, 9(1), 1–7 CrossRef PubMed
.
- S. B. Gunnarsson, K. Bernfur, A. Mikkelsen and T. Cedervall, Analysis of nanoparticle biomolecule complexes, Nanoscale, 2018, 10(9), 4246–4257 RSC
.
- N. Pimpha, S. Chaleawlert-umpon, N. Chruewkamlow and W. Kasinrerk, Preparation of anti-CD4 monoclonal antibody-conjugated magnetic poly(glycidyl methacrylate) particles and their application on CD4+ lymphocyte separation, Talanta, 2011, 84(1), 89–97 CrossRef CAS PubMed
.
- P. Gagnon, P. Toh and J. Lee, High productivity purification of immunoglobulin G monoclonal antibodies on starch-coated magnetic nanoparticles by steric exclusion of polyethylene glycol, J. Chromatogr. A, 2014, 1324, 171–180 CrossRef CAS PubMed
.
- L. Borlido, L. Moura, A. M. Azevedo, A. C. Roque, M. R. Aires-Barros and J. P. S. Farinha, Stimuli-Responsive magnetic nanoparticles for monoclonal antibody purification, Biotechnol. J., 2013, 8(6), 709–717 CrossRef CAS PubMed
.
- S. Odabaş, F. Sayar, G. Güven, G. Yanıkkaya-Demirel and E. Pişkin, Separation of mesenchymal stem cells with magnetic nanosorbents carrying CD105 and CD73 antibodies in flow-through and batch systems, J. Chromatogr. B: Anal. Technol. Biomed. Life Sci., 2008, 861(1), 74–80 CrossRef PubMed
.
- H. Xu, Z. P. Aguilar, L. Yang, M. Kuang, H. Duan and Y. Xiong,
et al., Antibody conjugated magnetic iron oxide nanoparticles for cancer cell separation in fresh whole blood, Biomaterials, 2011, 32(36), 9758–9765 CrossRef CAS PubMed
.
- F. F. Magalhães, M.
R. Almeida, S. F. Soares, T. Trindade, M. G. Freire and A. L. Daniel-da-Silva,
et al., Recovery of immunoglobulin G from rabbit serum using κ-carrageenan-modified hybrid magnetic nanoparticles, Int. J. Biol. Macromol., 2020, 150, 914–921 CrossRef PubMed
.
- D. Čakara and M. Borkovec, Microscopic protonation mechanism of branched polyamines: poly (amidoamine) versus poly (propyleneimine) dendrimers, Croat. Chem. Acta, 2007, 80(3–4), 421–428 Search PubMed
.
- M. Ciolkowski, M. Rozanek, M. Bryszewska and B. Klajnert, The influence of PAMAM dendrimers surface groups on their interaction with porcine pepsin, Biochim. Biophys. Acta, Proteins Proteomics, 2013, 1834(10), 1982–1987 CrossRef CAS PubMed
.
- M. Camarada, V. Márquez-Miranda, I. Araya-Durán, A. Yévenes and F. González-Nilo, PAMAM G4 dendrimers as inhibitors of the iron storage properties of human L-chain ferritin, Phys. Chem. Chem. Phys., 2015, 17(29), 19001–19011 RSC
.
- T. Zadeh Mehrizi, M. Shafiee Ardestani, A. Khamesipour, M. Haji Molla Hoseini, N. Mosaffa and A. Anissian,
et al., Reduction toxicity of amphotericin B through loading into a novel nanoformulation of anionic linear globular dendrimer for improve treatment of leishmania major, J. Mater. Sci.: Mater. Med., 2018, 29(8), 125 CrossRef
.
- J. M. Rae and B. Jachimska, Analysis of dendrimer-protein interactions and their implications on potential applications of dendrimers in nanomedicine, Nanoscale, 2021, 13(4), 2703–2713 RSC
.
- M. Marcinkowska, E. Sobierajska, M. Stanczyk, A. Janaszewska, A. Chworos and B. Klajnert-Maculewicz, Conjugate of PAMAM dendrimer, doxorubicin and monoclonal antibody—trastuzumab: the new approach of a well-known strategy, Polymers, 2018, 10(2), 187 CrossRef PubMed
.
- M. Marcinkowska, M. Stanczyk, A. Janaszewska, E. Sobierajska, A. Chworos and B. Klajnert-Maculewicz, Multicomponent conjugates of anticancer drugs and monoclonal antibody with PAMAM dendrimers to increase efficacy of HER-2 positive breast cancer therapy, Pharm. Res., 2019, 36(11), 1–17 CAS
.
- J. B. Otis, H. Zong, A. Kotylar, A. Yin, S. Bhattacharjee and H. Wang,
et al., Dendrimer antibody conjugate to target and image HER-2 overexpressing cancer cells, Oncotarget, 2016, 7(24), 36002 CrossRef PubMed
.
- W. Cheng and T. Allen, The use of single chain Fv as targeting agents for immunoliposomes: an update on immunoliposomal drugs for cancer treatment, Expert Opin. Drug Delivery, 2010, 7(4), 461–478 CrossRef CAS PubMed
.
- B. Tiwari, A. Agarwal, A. K. Kharya, N. Lariya, G. Saraogi and H. Agrawal,
et al., Immunoglobulin immobilized liposomal constructs for transmucosal vaccination through nasal route, J. Liposome Res., 2011, 21(3), 181–193 CrossRef CAS PubMed
.
- Z. Hatami, F. Jalali, M. A. Tabrizi and M. Shamsipur, Application of metal-organic framework as redox probe in an electrochemical aptasensor for sensitive detection of MUC1, Biosens. Bioelectron., 2019, 141, 111433 CrossRef CAS PubMed
.
- C. Wang, J. Gao and H. Tan, Integrated antibody with catalytic metal–organic framework for colorimetric immunoassay, ACS Appl. Mater. Interfaces, 2018, 10(30), 25113–25120 CrossRef CAS PubMed
.
- T. Zhang, B. Zou, M. Shao, X. Chen, S. Zhang and L. Li,
et al., Metal-Organic Framework Wears a Protective Cover for Improved Stability, Chem.–Eur. J., 2017, 23(32), 7663–7666 CrossRef CAS PubMed
.
- X.-G. Wang, Z.-Y. Dong, H. Cheng, S.-S. Wan, W.-H. Chen and M.-Z. Zou,
et al., A multifunctional metal–organic framework based tumor targeting drug delivery system for cancer therapy, Nanoscale, 2015, 7(38), 16061–16070 RSC
.
- Z. Hu, X. Wang, J. Wang and X. Chen, PEGylation of metal-organic framework for selective isolation of glycoprotein immunoglobulin G, Talanta, 2020, 208, 120433 CrossRef CAS PubMed
.
- Z. Hu, J. Meng, X. Wang, W. Li and X. Chen, Tailoring the Surface Properties of Co-based Metal–Organic Frameworks for Highly Efficient and Selective Enrichment of Immunoglobulin G, ACS Appl. Mater. Interfaces, 2020, 12(49), 55453–55459 CrossRef CAS PubMed
.
- H. Setiawan, E. Yuba, A. Harada, I. Aoki and K. Kono, Fabrication of gold nanohybrids modified with antibody and functional dendrimers for targeted photothermal theranostics, Nano Select, 2021, 779–790 CrossRef
.
|
This journal is © The Royal Society of Chemistry 2021 |
Click here to see how this site uses Cookies. View our privacy policy here.