DOI:
10.1039/D1NR04964J
(Review Article)
Nanoscale, 2021,
13, 16405-16426
Manganese-based advanced nanoparticles for biomedical applications: future opportunity and challenges
Received
30th July 2021
, Accepted 6th September 2021
First published on 6th September 2021
Abstract
Nanotechnology is the most promising technology to evolve in the last decade. Recent research has shown that transition metal nanoparticles especially manganese (Mn)-based nanoparticles have great potential for various biomedical applications due to their unique fundamental properties. Therefore, globally, scientists are concentrating on the development of various new manganese-based nanoparticles (size and shape dependent) due to their indispensable utilities. Although numerous reports are available regarding the use of manganese nanoparticles, there is no comprehensive review highlighting the recent development of manganese-based nanomaterials and their potential applications in the area of biomedical sciences. The present review article provides an overall survey on the recent advancement of manganese nanomaterials in biomedical nanotechnology and other fields. Further, the future perspectives and challenges are also discussed to explore the wider application of manganese nanoparticles in the near future. Overall, this review presents a fundamental understanding and the role of manganese in various fields, which will attract a wider spectrum of the scientific community.
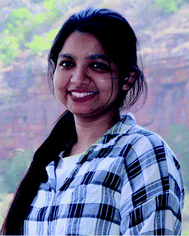 Shagufta Haque | Shagufta Haque received her B.Sc. (2015) and M.Sc. (2017) in Zoology from Lady Brabourne College and University of Calcutta, Ballygunge Campus. She secured 59th rank in Ph.D. entrance exam (CSIR-UGC-NET) held at the national level in December 2017 and joined Dr Patra's group at the CSIR-Indian Institute of Chemical Technology (CSIR-IICT), Hyderabad as a CSIR-JRF in 2018. Currently, she works at the Department of Applied Biology and her Ph.D. research involves the design and development of advanced novel nanomaterials for drug delivery in angiogenesis, wound healing and ischemic diseases. |
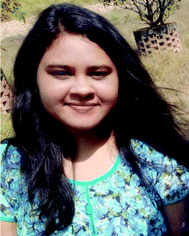 Sanchita Tripathy | Sanchita Tripathy received her B.Sc. (2016) and M.Sc. (2018) in Zoology from Utkal University. She received her MPhil (2020) in Life Sciences from Sambalpur University. She secured 80th rank in CSIR UGC JRF (NET) exam organized by Council of Scientific and Industrial Research, New Delhi, Govt. of India, held in December 2019. She joined Dr Patra's group at the CSIR-Indian Institute of Chemical Technology (CSIR-IICT), Hyderabad as a UGC-JRF in 2021. Currently, she works at the Department of Applied Biology and her Ph.D. research involves the design and development of advanced nanomedicines for cancer therapeutics. |
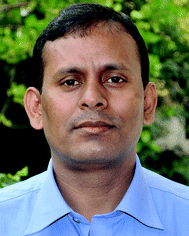 Chitta Ranjan Patra | Dr Chitta Ranjan Patra received his Ph.D. in Chemistry from the CSIR-National Chemical Laboratory (NCL), Pune. Dr Patra is currently the Principal Scientist at the Department of Applied Biology, CSIR-Indian Institute of Chemical Technology (IICT), Hyderabad. He is also an Associate Professor of Biological Sciences in the Academy of Scientific & Innovative Research (AcSIR), Ghaziabad. Dr Patra's research group is pursuing various nanomedicine research projects aimed at developing advanced biomaterial- and nanoparticle-based drug delivery systems (DDS) for the treatment of cancer, cardiovascular and ischemic disease, wounds and bacterial infection. |
1. Background of manganese
Currently, the exploration of transition metal nanoparticles has attracted increasing attention because of their revolutionary and promising properties in certain areas such as drug delivery, anticancer therapeutics, antimicrobial activities, and bioimaging.1–5 Among the transition metal nanoparticles, manganese (Mn) nanoparticles have attracted the most attention for applications such as solar cells, magnetic storage devices, biological applications such as molecular sieves and bioimaging due to their chemical as well as physical features.6–9 Manganese is the third most abundant transition element after iron and titanium, and the twelfth most common element on Earth.10,11 Manganese (Mn) with different oxidation states is present in the Earth's crust and the particulate matter in the atmosphere and water. Manganese is present as different 3d transition metal oxides such as MnO, MnO2, Mn2O3, Mn3O4 and Mn5O8.12 In living systems, manganese is considered a necessary micro-nutrient for growth, metabolism, reproduction, etc., which is acquired through food and water.13–15 Inside the human body, Mn is absorbed mainly through the gastrointestinal tract together with lungs. Mn is utilized in cellular energy regulation, blood clotting, connective tissue and bone growth, brain development, etc.16–22
The reference daily intake (RDI) of Mn suggested by the U.S. Food and Drug Administration (U.S. FDA) is 2–5 mg day−1 for adults. This dosage has been suggested to be safe by the U.S. National Research Council (NRC).23 The requirement for Mn varies depending on gender and life stage. The difference in Mn requirements is attributed to the fact that women have lower concentrations of serum ferritin than men. Furthermore, lactating women require more Mn.24 However, manganese in the form of composites has certain disadvantages such as short blood circulation time and accumulation in the brain, resulting in changes in the central nervous system followed by cognitive and movement abnormalities. Therefore, the necessity of Mn and the limitations of its complexes have encouraged scientists to explore Mn in the form of nanoparticles.25–27
Manganese-based nanomaterials are ecofriendly, economical, biocompatible, and possess strong adsorption property, and thus are suitable for different biological applications such as drug delivery, anticancer, antimicrobial, antioxidants, nanozymes, photothermal therapy, and biosensing.27–39 Although several articles have been reported regarding the utilization of manganese nanoparticles, there is no comprehensive review article on the most recent development of manganese-based nanoparticles for biomedical applications together with their challenges and future perspective. Considering their implications in healthcare and other issues, the present review article focuses on the background and different aspects of manganese and its nanoforms for various biological applications. Finally, considering their commercial opportunity in the near future, the mechanism of the actions and toxicological update of manganese nanoparticles are also discussed.
2. Historical and industrial development
Manganese has been used for decades in industrial and biological development. The use of manganese can be traced back to the Stone Age, where it was used as a pigment for cave paintings.40–42 The use of manganese as the black ore pyrolusite (manganese dioxide) in cave paintings has been well documented in the Upper Paleolithic period (17
000 years ago) or Stone Age. Manganese (found in iron ore) was utilized by Spartans in ancient Greece to develop steel weapons. Up to the 14th century, Mn was used by Roman and Egyptian glass makers to either remove or add color to glass.40 In 1774, Johan Gottlieb Gahn, a Swedish mineralogist, isolated manganese as a metal component from pyrolusite.41 The word manganese originated either from the Latin word ‘magnes’ for magnet or ‘magnesia nigra’, meaning black magnesium oxide.43 The Greek word for manganese is ‘magic’.44 In 17th century, Glauber, a German chemist, developed permanganate, the first functional manganese salt. Subsequently, Carl Wilhelm, a Swedish chemist, produced chlorine using MnO2.41
At the commencement of the 19th century, manganese was used in steel making.45 In 1816, a German scientist discovered the properties of manganese in steel making, where it increases the hardness of iron without affecting its toughness and malleability. Ferromanganese was produced with a high content of manganese first by Prieger (1826), and later by Pourcel (1875). The major breakthrough occurred in 1860 when Sir Henry Bessemer used spiegeleisen to remove sulphur and excess oxygen from steel, paving the way towards modern industrialization. The discovery of the Leclanche cell, which contains MnO2, increased the demand for manganese on a large scale.46 Large-scale commercial production utilizing Mn started in the 20th century for chemical and metallurgical applications. Since then, the demand for manganese production has increased daily. Accordingly, to fulfill the rising demand for manganese in the 21st century, deep sea mining has been used for the extraction of manganese nodules.47
3. Presence of manganese in living systems
Manganese is a crucial nutrient essential for different metabolic functions required for normal human development.15,16,48 Mn has also been reported to be involved in the synthesis and activation of enzymes, metabolism of lipids and glucose, protein synthesis, hematopoiesis, endocrine regulation and immunological functions.15,16,49–52 The metalloenzymes of Mn such as phosphoenolpyruvate decarboxylase, Mn superoxide dismutase (MnSOD), glutamine synthetase and arginase help in the metabolic processes together with scavenging free radicals, leading to a reduction in oxidative stress.13,15,18,53–55 The absorbed Mn becomes further available to other mitochondrial-rich organs especially the pituitary, liver and pancreas.56 The following sections discuss the role of Mn at different cellular and subcellular levels.
3.1. Enzymes
Enzymes are defined as biological catalysts, which at a certain range of optimum environmental conditions of pH, temperature and pressure within cells, carry out biochemical reactions with increased specificity and efficiency.54 For the proper functioning of enzymes, metal ions are required for the catalytic process, to maintain their structure, etc. Some enzymes have manganese-based catalytic activity.13,54,55 There are two categories of Mn-related enzymes, where one is Mn-activated/inactivated enzymes and the other Mn-containing enzymes.13,55,57 Mn-activated enzymes are rapidly reversible, tight, and have specific sites that form complexes with manganese for either regulatory or catalytic function.13,58 Conversely, Mn-containing enzymes include manganese superoxide dismutase and arginase. Moreover, Mn has a specific role in the different enzyme classes such as oxidoreductases, transferases, hydroxylases, lyases and ligases.49
Oxidoreductases are a class of enzymes responsible for redox reactions. The oxidoreductases that contain Mn include peroxidase, catalase, hydroxylase, and manganese superoxide dismutase (MnSOD).59 MnSOD, catalase and peroxidase protect cells from potentially damaging radical species.18,53,60,61 Transferases are a group of enzymes responsible for the transfer of functional groups between two molecules. The enzymes that require Mn for their proper functioning are glycosyl-transferases, protein kinases, DNA and RNA polymerases and a few sulpho-transferases.17,18 Among the hydroxylases, the enzymes that carry out specific functions such as growth, metabolic and cellular functions are reportedly Mn dependent such as are peptidases, amidases, proteinases, hydrolases of ATP and organo-phosphates. The other Mn-specific hydroxylases include cyclic nucleotide phosphodiesterase, phosphoinositol phosphatases, phospholipase C and several exo- and endo-nucleases.17,62 Some enzymes of the lyase group are activated by Mn, for example, phosphoenolpyruvate carboxykinase, guanylate and adenylate cyclases, which are required in cell regulation and metabolism.63 The ligases help to catalyze biosynthesis reactions via the lysis of ATP. The enzymes in the class ligase that contain Mn are alanyl–tRNA synthetase, glutamine synthetase and pyruvate carboxylase.64 Therefore, all these enzymes need Mn for their proper functioning and regulation of cellular function.
3.2. Neurotransmitters
Neurotransmitters are chemicals present endogenously for signal transmission from one neuron to another neuron, gland or muscle.65 Metal ions have various roles in the proper working of neurotransmitters such as zinc and manganese.48,65,66 Mn is required at micromolar concentrations for normal and proper functioning of the nervous system.48,66 Mn is needed during the uptake and release of neurotransmitters. Mn is also responsible for altered ATPase activity for the uptake of noradrenaline and dopamine in rat synaptosomes and in striatal tissue.67 Manganese also changes the Ca(II) uptake and catecholamine secretion in cultured adrenal medulla cells. The ionic channels acting in response to the excitatory amino acids located in the hippocampal neurons are also Mn specific.21 Guanylate cyclase, one of the enzymes released by gonadotropin-releasing hormone, requires Mn for its activation.68 There is a clear link between neurotransmitter regulation of neuronal adenylate cyclase and calmodulin activation by Mn.19 Mn affects the neurotransmitter receptors by changing the receptor binding site specificity and interaction with guanylate, adenylate cyclases, phosphatases and protein kinases for the regulation of intracellular enzymes or receptor together with stabilization of the interactions between the receptor subunits.18,20,63
3.3. DNA–RNA interactions
The nucleic acids, DNA and RNA, are comprised of nucleoside building blocks attached through phosphodiester linkages. However, the nucleic acids require metal ions for the hydrolytic cleavage of the phosphodiester bonds and other biological activities.48,69,70 Manganese has been reported to interact with DNA and RNA. The relation between DNA and Mn was studied with the help of different biophysical techniques for the detection of ligand groups and conformational changes.71 The Mn has the ability to catalyze pre-biotic DNA production without the presence of any enzymes. As mentioned earlier, Mn plays a role in DNA and RNA polymerases.17,18 During the replication process, Mn takes part in mutagenesis through its interaction with DNA and DNA polymerase I.72 Mn even has effects on translational repressor together with double-stranded RNA-activated inhibitor.73
3.4. Metabolism
Manganese plays a major role in the metabolic processes of the body together with the metabolism of prominent biomolecules.15,48,56 Mn helps in the metabolism of carbohydrates.15 PEP carboxykinase and pyruvate carboxylase are Mn specific, which are required for carbohydrate homeostasis, and thus excess or a deficiency in Mn affects the carbohydrate equilibrium.15,74,75 Mn has been reported to have an insulin-mimicking effect.76 Regarding lipid metabolism, there is a strong correlation between Mn and cholestasis given that Mn excretion takes place through the manganese–bilirubin complex.77,78 There are reports of altered high-density lipo-protein (HDL) compositions due to Mn deficiency.79 The synthesis of phosphatidyl–inositol in different tissues is Mn dependent. Certain reports in the literature highlight the relationship between lipid peroxidation and developmental problems associated with Mn deficiency.15,48 The other effects of Mn include inhibition of deoxyribose degradation by manganese–superoxide dismutase. Mn is actively involved in the metabolism of drugs in the brain.48,70 Therefore, all these cases clearly indicate the indispensable role of Mn in metabolism in the human body.
3.5. Cellular effects
Mn is present in the cellular components of blood and in plasma. The transport, efflux and distribution of Mn through the plasma membrane of hepatocytes occur via facilitated diffusion.56,80 There are numerous tight binding sites for Mn in the cytoplasm of cells following its active transport into the mitochondria. The Mn distribution in the cell is 40% in the cytoplasm and 60% in mitochondria. The Mn distribution in mammalian cerebral cells is similar to that in hepatocytes, but its concentration is greater in mitochondria.81 As discussed in the previous section, Mn prevents cell damage due to the generation of free radicals.18,53,60,82 After crossing the blood brain barrier, Mn binds to transferrin and maintains iron homeostasis.83 Consequently, Mn deficiency leads to convulsions and epilepsy.84
In the case of hormones, Mn directly regulates glucagon, insulin, and carbohydrate metabolism by influencing their biosynthesis and release.85 It has been reported that Mn injections are beneficial for glucagon release, which increases the blood glucose concentration.74 Besides, Mn is also involved in the regulation of prostacyclin production in aortic endothelial cells, prostaglandin synthesis in hepatocytes, discharge of noradrenaline from the pulmonary artery, etc. Mn influences oxytocin analogues on the receptors present on mammary gland and myometrial membranes. With reference to the interaction of Mn with other elements, Mn manipulates calcium uptake in the sarcoplasmic reticulum. Mn also affects copper and zinc uptake.49 In the case of heavy metal toxicity, Mn helps to increase tolerance towards cadmium hepatotoxicity and lethality.86
3.6. Role of manganese for blood sugar regulation and diabetes
Manganese, as an essential trace element, is involved in maintaining normal immune responses, cellular energy, blood sugar regulation, protection against oxidative stress, etc.87 Lower levels of Mn in the blood increases the risk of diabetes.87,88 In the case of diabetes, due to the high glucose level, various pathogenic pathways are initiated such as ROS. Further, the increased ROS affect the islet beta cells of the pancreas, leading to insulin resistance, diabetes and obesity. A relationship exists between the pancreas and Mn for maintaining the blood glucose level. Mn may accelerate insulin release from the pancreas to the bloodstream or glucagon inhibition, thereby increasing the cellular glucose uptake.88 An Mn deficiency leads to lower insulin secretion from the pancreas, followed by its increased degradation.89 Also, lower levels of Mn affect transport, glucose tolerance and enhance the risk of metabolic syndrome in adipose cells.15,90,91 Accordingly, Baly et al. demonstrated that Mn-deficient Sprague-Dawley rats showed a decrease in distal insulin receptors, leading to a decrease in glucose transport.91 This also affected the insulin-dependent glucose oxidation to CO2 and lowered the conversion of triglycerides.91 The Mn porphyrin catalytic antioxidant (MnP) upregulates glucose oxidation, followed by reduced fatty acid oxidation. Further, Mn supplementation decreases the risk of endothelial problems in diabetes.92 Therefore, Mn-related carbohydrate metabolism is due to the role of Mn in insulin release and gluconeogenesis.15
Table 1 describes the role of manganese in living systems.17,18,21,53,63,64,67,73,74,76,77,83,86
Table 1 Role of manganese in living systems
Mn |
Role of Mn |
Function |
Ref. |
MnII/MnIII |
Enzymatic |
Anti-oxidant, metabolism, and cellular regulation |
18
|
MnSOD |
Antioxidant |
Anti-oxidative/nitrosative stress |
53
|
Mn2+ |
DNA replication |
Mutagenesis |
17
|
Mn2+ |
Enzymatic |
Adenylate cyclase activity |
63
|
Mn2+ |
Enzymatic |
Stimulate ligase reaction |
64
|
Mn |
Neurotransmitter |
Neurotransmitter synthesis and metabolism |
21
|
Mn |
Brain development |
Changes in norepinephrine |
67
|
Mn2+ |
Cellular translation |
RNA-activated inhibitor |
73
|
Mn |
Lipid metabolism |
Cholestasis |
77
|
Mn |
Carbohydrate |
Insulin mimetic |
76
|
Mn |
Iron homeostasis |
Binds to transferrin |
83
|
Mn |
Glucagon release |
Blood glucose concentration |
74
|
Mn |
Heavy metal toxicity (cadmium) |
Cadmium hepatotoxicity |
86
|
4. Synthetic approaches for manganese nanoparticles
Manganese as a metal has been found to have different applications inside the human body, and hence scientists worldwide are focusing on methods for its feasible synthesis.93–97 The synthetic procedures, precursors and reaction conditions lead to the formation of manganese nanoparticles of different shapes and sizes.98,99 The following section highlights the different synthetic approaches for the preparation of Mn nanoparticles, which are briefly described below:
• Chemical synthesis93,100,101
• Physical synthesis102–106
• Biological synthesis.95–97,107,108
4.1. Chemical synthesis
The chemical methods used for the synthesis of Mn nanoparticles involve oxidation-reduction, hydrothermal methods and microemulsion techniques. The advantages underlying for chemical synthesis include less time consumption, greater yield and reduced expenditure of energy. There are several reports in the literature on chemically synthesized Mn-based nanoparticles. The chemical acts as a reducing agent and capping agent for the formation of nanoparticles.100 Several chemicals are utilized for the synthesis of manganese nanoparticles such polyvinylpyrrolidone (PVP), aqueous ammonia solution of KMnO4, and methyl acetate.93,100,101
4.2. Physical synthesis
The physical methods involve the formation of Mn nanoparticles without the use of any chemical or biological precursors. There are different types of physical methods for the synthesis of nanoparticles, namely, evaporation–condensation, arc discharge, laser ablation, and thermal decomposition.102–106
4.3. Biosynthesis
Recently, the green chemistry approach has been extensively used for the synthesis of various metal nanoparticles due to its features such as ecofriendly nature and easy synthesis. This approach overcomes the limitations of the use of harmful chemicals and excessive energy, which are generally used in industries.109–111 Similarly, manganese nanoparticles are synthesized using biological agents such as plants and microorganisms including bacteria and fungi.95–97,107,108 In this case, flower-shaped MnO2 nanoparticles doped with silver were synthesized by Jana et al. via wet chemical procedures at low temperature.112
Manganese-based nanomaterials can be characterized using several analytical tools including XRD, FTIR, DLS, XPS, SEM, TEM, and HRTEM.93,96,100,101 Readers who want to know more about the synthesis of manganese-based nanoparticles can refer to the literature.93,95,96,100–107
5. Physical and chemical properties of manganese
Manganese is the third most abundant transition element after iron and titanium, and is the twelfth most common element on Earth.10,11 Manganese (Mn), which is non-toxic and economic with different oxidation states, is present in the Earth's crust and particulate matter in the atmosphere and water.12,113 Mn exhibits efficient redox properties because its varying oxidation states, ranging from −3 to +7, and capacity to form compounds with a coordination number up to 7.5. This results in the use of high oxidation-state manganese species as strong oxidizing agents.113 Also, Mn in the form of oxides has several unique properties including redox property, surface nano-architectures and crystal structures.114 Manganese is effective in improving the catalytic activity in several oxidation reactions.115 The other determining factors for catalytic activity are the size and morphology of manganese oxide catalysts in a structure-dependent sensitive reaction.116 Manganese has the ability to transform itself for a variety of redox functions.44 There are several enzymes that require Mn for their proper functioning, as discussed in the previous sections. Mn catalases provide a platform for bioinorganic chemistry to mimic biological reactions.117 Mn-based catalysts act as substitutes for noble metals and oxides in a wide range of reactions.114
Because of the catalytic properties of manganese oxide, its nanoforms exhibit oxidase-like features, and hence are referred to as nanozymes.118 These features of manganese together with its multi oxidation states help it to act like an antioxidant and scavenge ROS.119 The activity of manganese nanozymes is dependent on their size, morphology, surface area and redox functions, which mimic the activity of redox enzymes such as superoxide dismutase, catalase and glutathione peroxidase. The role of manganese nanoparticles as nanozymes for biomedical applications is discussed in the sections below.34,118,120,121
Manganese nanoparticles are being explored for drug delivery applications due to their large surface to volume ratio, increasing their sensitivity towards the tumor microenvironment.27 Moreover, manganese nanoparticles act dually as on-demand and controlled systems for drug delivery applications. The tumor microenvironment is highly acidic and contains an increased concentration of glutathione (GSH). Hence, manganese nanoparticle-based drug delivery uses the concept of pH and GSH response for the release of drugs in a controlled manner.122 Manganese dioxide was recently studied for drug delivery applications and as a scaffold for stem cell transplantation and differentiation due to its biodegradable and non-toxic nature.123 Several reports investigated the drug delivery applications of Mn nanoparticles, which are discussed in the subsequent sections.124–126
6. Different biomedical applications
Manganese-based nanoparticles have been reported to be used for various biomedical applications such as photothermal therapy, fluorescence quenchers, biosensors, antimicrobial, and anti-angiogenic, all of which are discussed in the following subsections.27–35
6.1. Drug delivery
Conventional therapies include chemotherapy, radiation and surgery. However, the most conveniently used chemotherapy has several disadvantages such as low bioavailability, low diffusion and retention capability, nonspecific targeting, multi-drug resistance, low therapeutic indices, and high dose accompanied with side effects.124–126 Accordingly, nanotechnology can play a significant role. Therefore, to reduce the adverse effects and increase the therapeutic efficacy of different drugs, scientists are focused on the design and development of various inorganic nanomaterials (gold, silver, platinum, etc.) for efficient drug delivery or targeted drug delivery using suitable targeting agents.127–130 Recently, manganese-based nanoparticles have been extensively used for theranostics applications to overcome the above-mentioned difficulties. They have been found to be efficient systems for drug delivery due to their unique physico-chemical properties such as high surface to volume ratio in comparison to their bulk forms.27,122,131,132 This also increases their sensitivity towards the tumor microenvironment. Another exclusive point of using manganese nanoparticles for drug delivery is that they can be used both as on-demand and controlled systems. It is known that the tumor microenvironment is highly acidic and has a high concentration of glutathione (GSH). Hence, manganese nanoparticle drug delivery systems can be developed using the concept of pH and GSH response. Accordingly, Zhao et al. developed a silica-coated core of Fe3O4@SiO2 linked with NaYF4:Yb,Er shell (MSU)-modified MnO2 nanosheets on the surface of nanoparticles for the loading and release of drugs.122Fig. 1 shows an overall schematic representation for the stepwise development of the MSU/MnO2–CR drug delivery system and its biological application. MSU was synthesized for fluorescence imaging and magnetic targeting, where the MnO2 nanosheets act as quenchers for the system and a drug carrier. A multifunctional nanocomposite was further developed by loading Congo Red drug for delivery. The nanoformulation was used to check the level of intracellular GSH concentration. GSH reduced MnO2 to Mn2+, thus leading to the release of the drug followed by turning on of its upconversion luminescence.122 Manganese nanoforms have been explored in the area of gene silencing treatment. For example, Fan et al. designed DNAzyme–MnO2 nanosheets for gene slicing treatment.131 Chlorin e6-labelled DNAzymes (Ce6) were adsorbed on the MnO2 nanosheets to prevent their enzymatic digestion by endogenous nuclease. The nanosystem even inhibited Ce6 for 1O2 generation in the circulatory system. In the presence of GSH, the reduction of MnO2 to Mn2+ released Ce6, which generated 1O2 for efficient photodynamic therapy. Mn2+ was also beneficial for GSH-modulated MRI of tumor cells. The modified nanosystem helped in bimodal cancer treatment.131
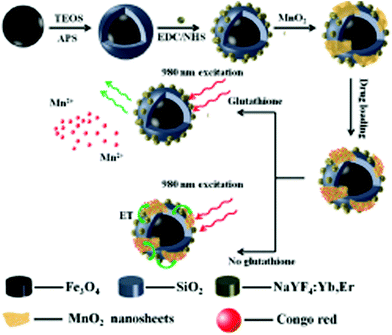 |
| Fig. 1 Schematic illustration of the synthetic procedure for the preparation of the MSU/MnO2–CR drug delivery system. Reprinted with permission from ref. 122 Zhao et al., Dalton Trans., 2014, 43, 451–457. Copyright©2014, The Royal Society of Chemistry. | |
MnO2 has a hollow space, which led scientists to explore its use for drug delivery due to its internal space, enhanced surface area, excellent permeability and low density. Exploring these characteristics, Xu et al. designed mesoporous manganese dioxide (mMnO2) grown over silica-coated mesoporous upconversion nanoparticles (UCNPs@mSiO2), which was further loaded with chlorin e6 and showed biodegradability in the tumor microenvironment.132 The loading of doxorubicin modified with polyethylene glycol in the mesoporous nanosystem was high due to its increased surface area. Inside the tumor microenvironment, GSH degraded mMnO2 to Mn2+, releasing the doxorubicin. Also, the UCNPs were coupled with Mn2+, which resulted in enhanced trimodal imaging. Further, the nanosystem helped to alleviate tumor hypoxia via the reduction of glutathione and decomposition of endogenous H2O2, resulting in effective chemo-photodynamic treatment.132 Similarly, Ren et al. developed manganese/cobalt oxide nanoparticles with doxorubicin encapsulated in their hollow cavity, which were released in the environment of GSH and exhibited efficient anticancer activity both in vitro and in vivo.133Fig. 2(a and b) show the in vivo MRI and tumor treatment by the nanoparticles, where Fig. 2a shows the T1-weighted and Fig. 2b the T2-weighted MR images at different time points. Fig. 2c exhibits the tumor volume curve, which illustrates that the tumor treated with the manganese/cobalt oxide nanoparticles with doxorubicin showed the maximum inhibition. The body weight curves shown in Fig. 2d show the minimal weight loss in the nanoparticle drug-loaded group, indicating the lowest toxicity of the drug carriers. The longest survivability was observed in the manganese/cobalt oxide nanoparticles with doxorubicin-treated mice (Fig. 2e). Fig. 2f shows the histopathological analysis of the nanoparticle-treated tumor-bearing mice. Furthermore, the histopathological stain sections of the tumor also showed no difference between the nanoparticle-treated group and the other groups, exhibiting the safety of the manganese-based nanoparticles.133 Recently, Wang and colleagues prepared superparamagnetic manganese ferrite nanoparticles (MnFe2O4) using a sonochemical method.134 The NPs showed good hydrophilicity, low in vitro cytotoxicity and high drug loading capacity. Doxorubicin hydrochloride was loaded on the surface of the MnFe2O4 nanoparticles was released in a pH-dependent manner in the tumor microenvironment.134 In another work, Zhang et al. designed hybrid nanomaterials (Au/MnO2) via the combination of gold nanorods and mesoporous manganese dioxide, resulting in a multi-responsive (GSH, pH and NIR responsive) nanoplatform for drug delivery.135 These conjugates showed a high doxorubicin drug loading capacity (through hydrogen bonding, electrostatic interaction and physical adsorption). The acidic environment and high GSH concentration of tumor cells were suitable for MnO2-based drug release. Fig. 3 exhibits an overall schematic representation of the preparation of the nanoparticles and their mechanism of drug release. The nanoparticles displayed efficient responses towards GSH, pH and NIR.135
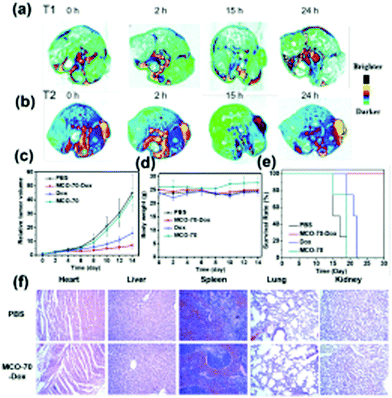 |
| Fig. 2
In vivo MRI and treatment of tumors by Dox-loaded MCO-70–Dox NPs. In vivo tumor (a) T1-weighted and (b) T2-weighted MR imaging before injection (0 h) and at different times (2 h, 15 h, and 24 h) after the injection of MCO-70–Dox NPs. (c) Tumor growth curves, (d) body weight curves, and (e) survival rate from the different treatment groups, which are PBS, MCO-70–Dox, Dox, and MCO, respectively. (f) Histological analyses of main organs from U87MG tumor-bearing nude mouse with intravenous injection of PBS and MCO–Dox NPs. Reprinted with permission from ref. 133 Ren et al., Nanoscale, 2019, 11, 23021–23026. Copyright©2019, The Royal Society of Chemistry. | |
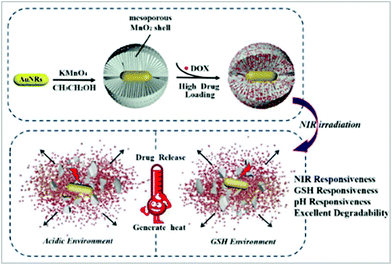 |
| Fig. 3 Scheme of the preparation of Au/MnO2 nanoparticles and drug release of Au/MnO2 nanoparticles. Reprinted with permission from ref. 135 Zhang et al., Eng. Chem. Res., 2019, 58, 2991–2999. Copyright©2019, the American Chemical Society. | |
6.2. Anti-angiogenesis for cancer
The process of angiogenesis is defined as the formation of new blood vessels from pre-existing ones.136,137 Although angiogenesis is required for several signaling pathways that are indispensable for proper functioning of living organisms, excessive angiogenesis is the root of many deadly diseases including cancer.138 Tumor angiogenesis is regarded as one of the key processes occurring during the development of cancer.139 Many chemotherapeutic agents target the inhibition of tumor angiogenesis to curb cancer. However, certain disadvantages regarding the specificity of commercial treatments have led to the use of nanoparticles. Manganese nanoparticles have been reported to exhibit anti-angiogenesis activity through the depletion of blood vessels in a chorioallantoic (CAM) membrane assay.140 In another work, Chang et al. delivered MnO2 nanoparticles in the hepatocellular carcinoma (HCC) tumor microenvironment to suppress and destroy the hypoxia-driven tumor.141 The synthesized nanoMnSor effectively delivered oxygen-producing MnO2 and sorafenib (anti-angiogenic drug) in the HCC tumor microenvironment. The hypoxic condition was alleviated by MnO2 by converting H2O2 into oxygen. In vivo treatment using these nanoparticles in a mouse orthotropic tumor model showed that sorafenib induced the suppression of primary tumor growth and decreased tumor vascularization and metastasis, helping the overall survival. NanoMnSor reprogrammed the hypoxic tumor microenvironment by reducing infiltration of tumor-associated macrophages, macrophage polarization (stimulation of M1 immunostimulatory macrophages) and activation of CD8+ cytotoxic T cells. This enhanced the efficacy of anti-PD-1 antibody and cancer vaccine immunotherapies. Therefore, manganese nanoparticles can act as anti-proliferative, anti-metastatic, anti-angiogenic, anti-immunosuppressive and tumor-based MRI agents.141
6.3. Antimicrobial activity
Antibacterial activity.
Microbial infections are considered to be one of the leading causes of mortality and morbidity globally.95,142–144 Different strains of bacteria, fungi, protozoans and viruses are responsible for eliciting infections in human beings. The conventional treatment strategies available such as antibiotics have limitations such as increased antibiotic resistance by microbial strains. Thus, to overcome the limitations of available therapies, metal nanoparticles have been considered. Manganese nanoparticles are reported to exhibit good antimicrobial properties (antibacterial and antifungal), and hence are being widely considered and explored.95,142,143 For example, Azhir et al. designed and synthesized Mn3O4 nanoparticles (size range: ∼10–30 nm) through precipitation methods.143 The nanoparticles exhibited efficient antibacterial activity against both Gram positive and Gram negative bacteria, which was validated through the broth microdilution method.143 In another work, Kamran et al. synthesized manganese nanoparticles using Cinnamomum verum bark extract and evaluated their antimicrobial activity on Escherichia coli and Staphylococcus aureus.142 The natural compounds present in the bark helped in the synthesis of manganese nanoforms and produced effective antimicrobial activity against the above-mentioned strains.
Dual role as antibacterial and antifungal agent.
Some manganese nanoforms exhibit both antibacterial and antifungal activity. In this context, Jayandran et al. biosynthesized Mn nanoparticles via the reduction of manganese acetate with turmeric, curcumin and lemon methanolic extract, exhibiting antibacterial and antifungal activity.95 The antibacterial activity of the nanoconjugates was studied against two Gram negative (Escherichia coli and Staphylococcus bacillus) and Gram positive (Staphylococcus aureus and Bacillus subtilis) bacteria and the results were compared with free curcumin-treated samples and a positive control experiment (chloramphenicol). Furthermore, the nanoparticles exhibited antifungal activity against four strains of fungi (Curvularia lunata, Candida albicans, Trichophyton simii and Aspergillus niger) with Fluconazole drug as the positive control. Therefore, the results clearly suggest the antibacterial and antifungal activity of the biosynthesized Mn nanoparticles.95 Among the methods for treating microbial infections, antimicrobial peptides are preferably used. However, the disadvantages associated with them include the cost of their production, stability and toxicity. Hence, to overcome all these issues and increase their efficiency, antimicrobial peptides are conjugated with various nanoparticles. For example, Lopez-Abarrategui et al. fabricated and synthesized manganese ferrite nanoparticles (modified with citric acid) with an antifungal peptide obtained from Cenchritis muricatus (sea animal).28 The nanoparticles exhibited excellent in vitro antifungal activity against Candida albicans. Thus, antifungal peptide-conjugated manganese nanoparticles can be used as an antifungal agent in the near future.28 In another report, Krishnan et al. intercalated manganese oxide on bentonite clay using thermal decomposition.145 The well diffusion and potato dextrose methods were used to study the antifungal and antibacterial activity of the manganese oxide–bentonite nanoconjugates. The antimicrobial activity of these conjugates against Staphylococcus aureus was found to be greater compared to Pseudomonas aeruginosa. Rapid antifungal behavior was also found against Candida albicans.145
Antiviral activity.
Metal nanoparticles have been recently explored as antiviral agents due to their unique properties such as large surface-to-volume ratio and small size to interact with the viral particles.146,147 Manganese has been reported to affect AIDS-related pathogens. For example, Nesterenko et al. reported the interaction between the AIDS-related pathogen, Cryptosporidium parvum, with human ileoadenocarcinoma (HCT-8) cells in vitro.148 The Mn from the MnSO4 salt inhibited the binding of the sporozoite membrane antigens to the HCT cells, thereby preventing the cells from being infected. These types of interactions are Mn sensitive. Mn2+ affects both the pathogen and cell membrane. The possible mechanism behind this type of interaction is that Mn activates the host cell surface enzymes, which affect the interactions of receptors and ligands.148 In another work, Vartanian et al. demonstrated that Mn enhanced the miscorporation of deoxynucleotide triphosphates (dNTPs) and altered the substrate specificity. Mn caused more mutation of HIV ex vivo.149 Considering the anti-viral properties of Mn-salts, we strongly believe that Mn-based nanoparticles can be useful for the treatment of viruses in the near future due to their antiviral activity.
6.4. Antioxidants
Antioxidants are regarded as substances that protect cells from the harmful effects of excessive ROS.119,150,151 However, the disadvantages of natural antioxidants such as sensitivity to temperature, short life span and bioavailability have led to the development of artificial ROS scavengers including nanoparticles. Some nanoparticles such as manganese nanoforms owing to their multi oxidation states exhibit antioxidant-like activity for scavenging ROS.119,150,151 Accordingly, Singh et al. designed Mn3O4 nanoparticles for scavenging ROS to prevent oxidative damage of cells.119 They prevented cellular biomolecules from DNA damage, lipid peroxidation and protein oxidation due to the action of ROS. The overall nanosystem can help to suppress oxidative stress-mediated pathological conditions, as shown by the schematic representation in Fig. 4.119 In another report, Yao et al. exhibited the antioxidant activity of Mn3O4 nanoparticles, which mimicked certain enzyme-like activities such as catalase, superoxide dismutase and hydroxyl radical scavenging actions.82 The nanoparticles were effective in scavenging ROS and their by-products in vitro and even in vivo by ameliorating ROS-induced ear inflammation in a mouse model. Thus, nanoparticles can pave new ways for the treatment of ROS-related diseases.82 In another study, Mahlangeni et al. biosynthesized manganese oxide nanoparticles using extracts of Cussonia zuluensis, which exhibited efficient antioxidant activity. The capping of the metal core with biomolecules of plant extracts enhanced its antioxidant activities.150
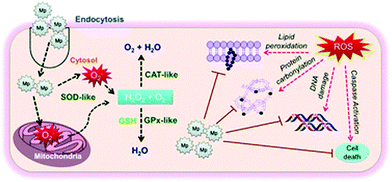 |
| Fig. 4 Schematic representation of the molecular mechanism of nanosystems in suppressing oxidative stress-mediated pathological conditions. Reprinted with permission from ref. 119 Singh et al., Nanoscale, 2019, 11, 3855–3863. Copyright©2019, The Royal Society of Chemistry. | |
Pardhiya et al. demonstrated the in vitro antioxidant activity of BSA-templated MnO2 nanoparticles in human embryonic kidney cells (HEK-293).151 These nanoparticles act in a similar manner to the antioxidant activity of catalase, superoxide dismutase, and peroxidase and were found to increase the viability of the HEK-293 cells. At a higher concentration, these nanoparticles acted like a prooxidant, becoming toxic to normal cells due to their inherent oxidase like activity.151
6.5. Nanozymes
Nanozymes are nanomaterials possessing enzyme-like features including substrate specificity, numerous active sites, enhanced catalytic activity, easy synthesis, low production cost and high efficiency.34,118,120,121 The catalytic actions of nanozymes in various microenvironments are controlled by temperature, pH, amount of glutathione, H2O2 and oxygenation levels.34 MnO2 nanoforms are referred to as nanozymes because of their oxidase-like feature. Considering these facts, Liu et al. tested the oxidase-like catalytic activity of BSA-based MnO2 nanoparticles using horseradish peroxidase (HRP) substrates [3,3′,5,5′-tetramethylbenzidine (TMB) and o-phenylenediamine (OPD)].118 The nanoparticles showed good Michaelis–Menten kinetics together with strong affinity towards HRP substrates and H2O2. The nanoforms resulted in the oxidation of the TMB from a pale yellow to blue compound (ox-TMB). The BSA–MnO2 nanoparticles were soluble, biocompatible in nature and exhibited a good dispersion. The enzymatic property of the BSA–MnO2 nanoparticles was used to detect goat anti-human IgG for colorimetric immunoassay instead of HRP. Thus, nanoparticles can be used for different bioassays and medicinal purposes in the future.118 Similarly, another colorimetric test assay was developed by Liu et al. to check the activity of MnO2 nanoforms for detecting and quantifying GSH.120 GSH inhibited the oxidation of TMB from a pale yellow to blue compound (ox-TMB) by MnO2 nanosheets in a concentration-dependent manner. This increased specificity of GSH inhibition led to its detection in human serum samples by the nanosystem.120
There are reports of manganese nanoparticles being used as DNA partzymes. Usually, the degradation of probes and biomarkers results in false positive test results. Thereafter, to protect both the target and probe, Chen et al. synthesized MnO2 nanoform-powered Janus DNA nanomachines (target- and probe-protective) for RNA imaging.121 The nanosystem was formed inside living cells via the congregation of two DNA partzymes, which are RNA responsive and a probe. The manganese nanoparticles were used both as promoters responsible for DNA cellular uptake and Mn2+ generators acting as cofactors of DNAzyme. This ensured the efficacy of catalytic cleavage. The RNA of the RNA–DNA hybrid was protected from degradation by RNase H with the help of the chemically modified DNA partzymes (having modified sugar moieties). The protection of RNA prevented the degradation of the target, resulting in the inhibition of false positive results. Fig. 5 illustrates the design and programming of the Janus nanomachine, where the cellular–RNA target, miR-21, is a green sequence. The chemical structures of DNA, PS–DNA, 2′OMe–DNA, and LNA are represented as a red circle within the gray sequences of the DNA partzymes. Within the blue sequence of the PS-modified substrate probe are the quencher (gray circles) and fluorophore (green circles). The MnO2 nanoform-powered Janus DNA nanomachine was beneficial for efficient RNA imaging.121 In another study, Singh et al. demonstrated the morphology-dependent multienzyme redox activity of manganese-based nanozymes (Mn3O4).61 The effect of the Mn3O4 nanozymes depended on the size, surface area, morphology and redox property of the manganese ions, which were found to mimic the activity of redox enzymes such as catalase, glutathione peroxidase and superoxide dismutase. The Mn3O4 nanoflowers limited the available superoxide levels inside the endothelial cells, thereby preventing the inactivation of nitric oxide (NO), which is essential in the treatment of cardiovascular diseases.61
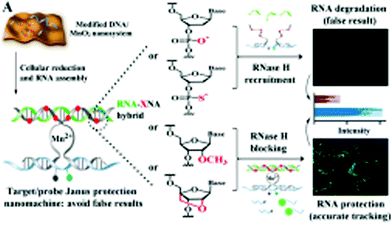 |
| Fig. 5 Design and programming of the protective Janus DNA nanomachine. The model cellular–RNA target (green sequence) is miR-21. The chemical structures of a DNA, PS–DNA, 2′OMe–DNA, and LNA monomer are presented, which are highlighted as red circles (red X) in the DNA partzymes (gray sequences). The gray and green circles in the PS-modified substrate probe (blue sequence) are the quencher and fluorophore, respectively. Reprinted with permission from ref. 121 Chen et al., Anal Chem., 2018, 90, 2271–2276. Copyright©2018, the American Chemical Society. | |
6.6. Photothermal and photodynamic therapy
Photothermal therapy is a selective, simple, non-invasive cancer treatment approach, in which near infrared light is used to produce hyperthermia in cancer cells selectively without affecting the surrounding tissue due to the specificity of the PTT agent and NIR light.152–156 Photodynamic therapy is defined as a non-invasive cancer treatment strategy in which photosensitizers are used together with light irradiation. In the presence of light and oxygen, the photosensitizers are activated to generate reactive oxygen species and singlet oxygen to kill cancer cells.27,152–156 Consequently, this initiates apoptosis and necrosis, thereby killing cancer cells. However, there are still certain limitations of photothermal therapy. Firstly, the hindrance of photothermal therapy due to the hypoxic condition of the tumor microenvironment. Secondly, the non-specificity of photosensitizers in photodynamic therapy causes their premature leakage into normal cells rather than the tumor cells, causing phototoxicity. Thus, to increase the specificity and functionality of photothermal and photodynamic therapy, they is used in combination with certain nanomaterials to increase their efficiency. Manganese dioxide nanoforms have oxidizing features and help in alleviating the hypoxic condition of the tumor microenvironment by the reaction with the H2O2 present within tumors.27,152–156 The Mn2+ ions produced after the reaction are biocompatible in nature and can be easily excreted through the kidneys. Accordingly, Zhu et al. synthesized MnO2 nanoparticles loaded with a chlorine e6 sensitizer (Ce6) conjugate followed by PEG coating for the enhancement of tumor-associated photodynamic therapy.153 The in vitro assays revealed that the Ce6@MnO2–PEG nanoparticles reacted with the H2O2 present in the cancer cells, thus generating O2. This alleviated the tumor hypoxic conditions, increasing the efficiency of photodynamic therapy killing the cancer cells. The in vivo results also corroborated the in vitro results, where the nanoparticles when injected in mice accumulated within the tumor. Here, the MnO2 nanoparticles slowly decomposed into Mn2+ ions, acting as a T1 MR contrast agent. Overall, the manganese nanoforms increased the oxygen content within the tumor environment to prevent the limitation of hypoxic condition faced by photodynamic cancer therapy.153
The untimely release of photosentisizers from their carriers and their non-specific accumulation in normal tissues instead of tumor tissues are major concerns associated with photodynamic therapy. Thus, to alleviate these issues, Ma et al. synthesized an MnO2 shell that can act as a switchable shield for the advancement of acidic H2O2 response together with the evolution of O2 for photodynamic therapy.155 The development of the nanoplatform proceeded by first loading the core with SiO2–methylene blue, which has a high photosensitizer capacity, followed by coating with the MnO2 shell. This was done to prevent the leakage of the photosensitizer after being intravenously injected into blood before reaching the tumor tissues. This led to the efficient accumulation of the photosensitizer in the tumor tissues. The MnO2 shell in the acidic tumor microenvironment reacted with H2O2, producing O2 and achieving a synergistic effect for photodynamic therapy with a decrease in tumor hypoxia. The reduced form of manganese even acts as an MRI imaging agent in vivo in the presence of high acidic H2O2.155 For photothermal therapy, Peng et al. constructed erythrocyte membrane-coated Prussian blue/manganese dioxide nanoparticles (PBMn–DOX@RBC) with a red blood cell (RBC) coating for relieving tumor hypoxia and enhancing cancer photothermal therapy and chemotherapy.156 The PBMn nanoparticles acted as an oxygen precursor or catalyzer for activating H2O2, whereas the RBC membrane increased the loading and prolonged the circulation capacity of doxorubicin (DOX) in vivo. In the presence of excess H2O2, administration of the nanoconjugates relieved the hypoxia condition in the tumor microenvironment. The O2 disrupted the RBC membrane on the nanoformulation, releasing doxorubicin and prolonging the circulation of the nanoparticles in the body. Fig. 6a depicts the photoacoustic images (PA) of the PBMn–DOX@RBC nanoparticles and Fig. 6b shows that the PA signal from the tumors was greater with the PBMn–DOX@RBC nanoparticles than only PBMn. The infrared thermal images of the tumors in Fig. 6c following irradiation with 808 nm show more heat generation from the PBMn–DOX@RBC nanoparticle-treated mice. Subsequently, the temperature of the tumors treated with the PBMn–DOX@RBC nanoparticles also increased more compared to PBMn alone (Fig. 6d). Fig. 6e illustrates the immunofluorescence staining, which shows that the hypoxic condition of the tumor was relieved with the PBMn–DOX@RBC nanoparticles. Therefore, PBMn–DOX@RBC enhanced tumor reduction via its combined effect as a chemotherapeutic agent and photothermal therapy agent.156
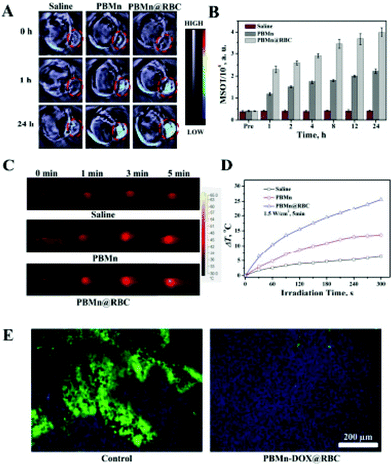 |
| Fig. 6 Photoacoustic imaging (PAI) (A) and PA intensity (MSOT) (B) mediated by PBMn and PBMn–DOX@RBC. The images represent the transverse sections of the mice during photoacoustic imaging. The tumors are marked in red circles. (C) and (D) Photo-thermal conversion of PBMn and PBMn–DOX@RBC in vivo, respectively: (C) infrared thermal images of the mice during 808 nm laser irradiation and (D) surface temperatures of the tumor during irradiation. (E) Representative immunofluorescence images of tumor sections stained with DAPI and a hypoxia marker (Hypoxyprobe-1 Kit). Reprinted with permission from ref. 156 Peng et al., ACS Appl. Mater. Interfaces, 2017, 9, 44410–44422. Copyright©2017, the American Chemical Society. | |
Some of the current reports show the use of biomolecules such as proteins for the production of bio-organic nanoparticles. Accordingly, Wang et al. synthesized MnO2 nanoparticles with the help of bovine serum albumin (BSA), which acted as a reducing agent and template for the formation of the nanoparticles.152 The biocompatible MnO2 nanoparticles exhibited absorbance in the NIR region and possessed high photothermal efficiency and stability. Thus, the nanoparticles could be used as an effective NIR photothermal antitumor agent.152 Liu et al. synthesized cobalt/manganese oxide nanocrystals through a hydrothermal process, which exhibited strong NIR absorption and were utilized for photothermal therapy.157 Also, they were employed for MR imaging in vivo. Being biocompatible in nature, the nanocrystals can be used as a potential agent for photothermal theragnosis.157 Similarly, Cheng et al., studied the magnetic resonance (MR) imaging-guided photothermal therapy of polydopamine-coated manganese carbonate nanoparticles (MnCO3@PDA NPs).158 The PDA-decorated nanoparticles had excellent MR contrast compared to the free nanoparticles due to the entrapment of more water surrounding the nanoparticles, resulting in water exchange and efficient MR contrast signals. Intratumoral injection of nanoparticles into 4T1 tumor-bearing mice produced efficient MRI-guided photothermal reduction of the tumors, making the nanoparticles excellent theranostic agents.158 Further, Sun et al. designed and synthesized folate receptor nanopalladium-decorated manganese dioxide nanosheets (Fp–Pd@MnO2) with a high doxorubicin loading capacity.159 Several factors such as near infrared stimulus, increasing concentration of glutathione and pH reduction are responsible for efficient drug release at the tumor location. Treatment of these nanoparticles with NIR irradiation (808 nm laser) resulted in a combined photothermal and chemotherapy effect in MDA-MB-231 cells along with in vivo reduction of the tumor.159 Considering bimodal application, Gupta et al. demonstrated the photothermal and magnetic hyperthermia therapeutic effect of citrate-coated manganese-doped magnetic nanoclusters on a glioblastoma cancer model using rat C6 glioblastoma cells.29Fig. 7 depicts an overall schematic representation of the combinatorial approach of photothermal and magnetic hyperthermia in killing the glioma cells. When the tumor cells were irradiated with 750 nm laser irradiation, structural changes, cytoskeletal damage, and oxidative stress corresponding to mitochondrial membrane potential reduction occurred, producing ROS-mediated apoptotic cell death.29
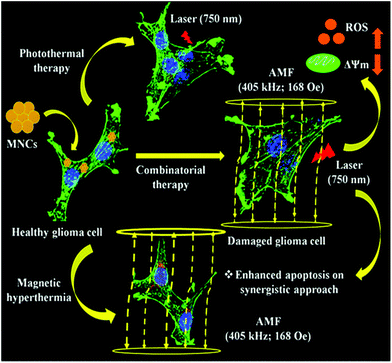 |
| Fig. 7 Schematic representation of the combinatorial approach of photothermal and magnetic hyperthermia in killing glioma cells. Reprinted with permission from ref. 29 Gupta et al., ACS Appl. Nano Mater., 2020, 3, 2026–2037. Copyright©2020, the American Chemical Society. | |
6.7. Fluorescence quenchers and biosensors
Fluorescence resonance energy transfer (FRET) is a process describing nonradiative energy transfer from a donor (luminescent) to another molecule (acceptor) in proximity to it through dipole–dipole coupling. FRET technology is used for various applications such as microscopy, immunoassays, and nucleic acid hybridization.32,160–163 Recently, 2D nanoforms of manganese with light harvesting features and ability to conduct electrons have been shown to have potential applications in photo-induced sensing (chemical and biological) transfer mechanisms, fluorescence resonance energy transfer (FRET), etc. MnO2 nanosheets exhibit an intense broad absorption peak in the NIR region and manganese, being an ultrathin semiconductor, facilitates their use as an efficient broad-spectrum quencher.32,160 There are reports illustrating that MnO2 reduction to Mn2+ reverses the MnO2-associated quenching influence and restores the fluorescence of the associated compound.32,160 It has been seen that on decreasing the distance between manganese nanoforms and carbon dots, FRET occurs, resulting in the temporary disappearance of the fluorescence of the carbon dots. Based on this, Qu et al. synthesized fluorescent carbon dots from the seed extracts of Sterculia lychnophora, which were quenched by MnO2 nanosheets and used as probes for the detection of alkaline phosphatase (ALP).32 The detection occurs by hydrolysis of acidic phosphoric acid (AAP) to ascorbic acid (AA) by ALP, and then AA reduces MnO2 to Mn2+. Following the destruction of the MnO2 nanosheets, the fluorescence of the carbon dots was restored. The detection could be performed even at lowest concentrations of ALP, and hence the nanocomplex could be efficiently used for diagnostic purposes.32 In this context, Ji et al. designed a CaO2/MnO2@polydopamine–methylene blue (MB) nanoform encapsulating CaO2 nanoparticles with the MnO2 nanosheets.161 MnO2 suppressed the fluorescence activity of the MB photosensitizer by π–π stacking or hydrophobic interaction. The nanosystem even had the ability to generate oxygen and alleviate tumor hypoxia. Once in the tumor microenvironment, MnO2 decomposed to Mn2+, releasing MB and initiating the fluorescence property. In this way, the nanoform was beneficial for both switch-controlled cell imaging and photothermal therapy.161 Because of the catalytic and oxidation activity of manganese nanoparticles, they are extensively used as biosensors. In another work, Yuan et al. fabricated an MnO2 nanosheet-modified upconversion (UC) nanostructure for the fluorescence detection of glucose in the serum and blood of humans.164 The manganese nanoparticles act as quenchers of the upconversion nanoparticles, which is relieved once H2O2 reduces MnO2 to Mn2+. The amount of H2O2 generated by enzymatic cleavage of glucose using glucose oxidase can be used to detect glucose. This nanosystem can be even exploited to identify other H2O2-based analytes.164
MnO2 nanoparticles have been further used for the detection of RNAs even at a minute concentration, given that the detection of microRNAs is very challenging owing to their low concentration, similar sequences and small size. Thus, Li et al. synthesized an MnO2 nanosheet-dependent hybridization chain reaction (HCR) system to detect the expression of miRNA inside cells at a low concentration.162 This is a cell signal amplification-based approach for the detection of miRNAs (which are down regulated), where the MnO2 nanoparticles quench the dye-labeled hairpin probes. They designed a dual labeled hairpin with FAM (a FRET donor) together with Tamra (TMR, a FRET acceptor) and loaded it on the MnO2 nanosheets. Once inside the cell, the dye-labeled hairpins were released due to the degradation of the MnO2 nanosheets by the GSH following reactions with proteins and nucleic acids. Subsequently, miRNA-21 produced dsDNA polymers via the cascade assembly of the two hairpins. Consequently, the FRET pair (FAM and TMR) comes into close contact, resulting in enhanced fluorescence signals for detecting miRNA-21 found in trace amounts. Therefore, the nanosystem can be used for the detection of trace miRNAs that are found in low concentration in living cells.162
6.8. Bioimaging and MRI contrast agents
Magnetic resonance imaging (MRI) works on the principle that many atomic nuclei in the presence of an external magnetic field absorb and emit radiofrequency energy. To increase the radiofrequency signals, hydrogen atoms are used, which are then received by the antenna from the tissues for examination. The advantages of the use of MRI include high spatial resolution, but a disadvantage associated with it is its low sensitivity. Therefore, manganese nanoforms are being used as alternative contrast agents for T1-weighted MRI, given that they are biocompatible, help in achieving brighter images, have short circulation time of Mn2+ ion chelate, and size-directed circulation time of colloidal nanoforms.26,27,165–167 The underlying mechanism is that the manganese atoms located within the manganese nanosheets make no contact with the external aqueous environment, thus preventing the transverse and longitudinal relaxation of protons. The various forms manganese nanoparticles (MnO, Mn3O4, and MnO2) are used as contrast agents in MRI, bimodal and multimodal imaging and imaging-guided tumor therapy. For T1-weighted MRI, Wang et al. designed and synthesized L-cysteine and PEG-modified manganese oxide NPs via a solvothermal process.8 The as-synthesized nanoparticles exhibited good T1-relaxivity, increased solution dispersibility, biocompatibility and colloidal stability. The modification with L-cysteine increased the blood circulation time with the reduction of cellular uptake by macrophages, thereby making the nanoparticles good contrast agents for enhancing T1-weighted MRI.8
Some manganese-coated nanoparticles are conjugated with aptamers to enhance their tumor-specific targeting ability. For example, Li et al. synthesized biocompatible PEG-coated MnO nanoparticles conjugated with a covalently cross-linked aptamer as contrast agents for T1-weighted MRI.168 These nanoparticles targeted renal cancer tumor cells together with prolonged storage of the probe within the tumor cells.168 To achieve enhanced imaging for clinical demands, PET and MRI dual-mode imaging is used through the development of PET/T1-MRI bimodal mode probes. In this context, Zhu et al. developed PET/MRI bimodal probes via the surface modification of PEI-coated Mn3O4 nanoparticles with Cu64 and folic acid (FA).169 The as-synthesized nanoprobes were injected in HeLa tumors with both blocked and unblocked folate receptors, and it was observed that the Cu64-labeled nanoparticles acted as better tracers in the non-blocked HeLa tumors even after 18 h of injection. Fig. 8 shows the micro PET images of the xenografted HeLa tumor-bearing nude mice treated with the nanoparticles, where the PEI-coated Mn3O4 nanoparticles with Cu64 and folic acid showed the maximum uptake.169 In another work, Zhan et al. synthesized and developed Mn3O4 nanoparticles that were conjugated with radionuclide copper-64 (64Cu) and anti-CD105 antibody, which helped in the targeted imaging of the tumor vasculature in mice.170 The, nanocomplex exhibited stability in both in vivo and in vitro. The biodistribution study showed the targeted accumulation of the manganese nanocomplex within the breast tumor of the mice and its specificity was further confirmed. Fig. 9 shows the serial PET scan images of the Mn3O4–radionuclide copper-64 (64Cu)–TRC105 (targeted), Mn3O4–radionuclide copper-64 (64Cu) (non-targeted) and Mn3O4–radionuclide copper-64 (64Cu)–TRC105 with pre-incubated blocking using TRC105. Therefore, this elucidates that the manganese nanocomplex can be efficiently used as potent T1 contrast agents for cancer diagnosis.170
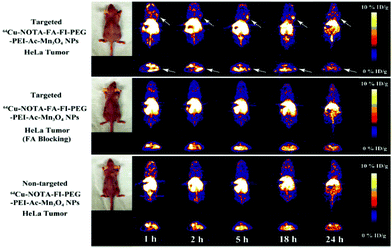 |
| Fig. 8 MicroPET images of nude mice bearing HeLa xenografted tumors at different time points post-intravenous injection of the 64Cu–NOTA–FA–FI–PEG–PEI–Ac–Mn3O4 NPs (targeted NPs), 64Cu–NOTA–FA–FI–PEG–PEI–Ac–Mn3O4 NPs with FA blocking, and 64Cu–NOTA–FI–PEG–PEI–Ac–Mn3O4 NPs (nontargeted NPs). The whole-body coronal (top) and transverse (bottom) microPET images of nude mice bearing HeLa xenografted tumors are shown. Tumors are indicated by arrows. Reprinted with permission from ref. 169 Zhu et al., ACS Appl. Mater. Interfaces, 2018, 10, 34954–34964. Copyright©2018, the American Chemical Society. | |
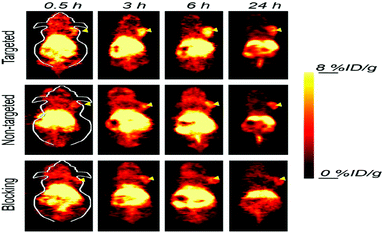 |
| Fig. 9 Serial coronal PET images of 4T1 tumor-bearing mice after injection of 64Cu–NOTA–Mn3O4@PEG–TRC105, 64Cu–NOTA–Mn3O4@PEG, or 64Cu–NOTA–Mn3O4@PEG–TRC105 after a pre-injected blocking dose of TRC105. Arrowheads indicate tumor locations. Reprinted with permission from ref. 170 Zhan et al., ACS Appl. Mater. Interfaces, 2017, 9, 38304–38312. Copyright©2017, the American Chemical Society. | |
6.9. Mechanism of action of manganese-based nanoparticles
Manganese-based nanoparticles are emerging as new-age agents for biomedical applications in the area of transition metal nanoparticles. All their applications such as drug delivery, photothermal therapy, antimicrobial, and anti-angiogenic have different mechanisms of action.27,118,119,141 Manganese nanoparticles are readily used for drug delivery applications due to their large surface to volume ratio, biocompatibility, non-toxicity and efficient targeted delivery, increasing their sensitivity towards the tumor microenvironment.27 The nanoforms of manganese oxide even have oxidase-like feature, and hence are referred to as nanozymes.118 This feature of manganese nanoparticles together with their multi oxidation states helps them to act like antioxidants and scavenge ROS.119 Manganese has a tendency to alleviate hypoxia, leading to an anti-tumor and anti-angiogenic effect for assisting cancer therapeutics.141 Further, manganese nanoparticles exhibit good antimicrobial properties (antibacterial and antifungal).142,143
For photothermal therapy, manganese nanoforms exhibit oxidizing properties for alleviating the hypoxic condition by interaction with H2O2, producing O2 in the tumor microenvironment.27 This also prevents the limitation faced by photothermal cancer therapy due to the hypoxic condition of the tumor microenvironment.153 Manganese nanoforms act as a shell to prevent leakage of photosensitizers, where the photosensitizers accumulate within the tumors during photodynamic therapy.155 Also, manganese-based nanoparticles are slowly decomposed into Mn2+ ions, helping them to be T1 MR contrast agents.153 Besides photothermal and photodynamic therapy, manganese nanoforms are efficient as fluorescence quenchers and biosensors. The 2D manganese nanoforms have light harvesting properties and conduct electrons, which are used for sensing (chemical and biological) in photo-induced transfer mechanisms, fluorescence resonance energy transfer (FRET), etc. Manganese nanosheets exhibit a broad absorption peak in the NIR region. Considering that Mn is an ultrathin semiconductor, it facilitates its use as a good broad-spectrum quencher. The reduction of MnO2 to Mn2+ reverses the MnO2-associated quenching influence and restores the fluorescence of the associated compound.32 For example, manganese nanoparticles can act as quenchers of upconversion nanoparticles, which is relieved once H2O2 reduces MnO2 to Mn2+.164 Therefore, all these mechanisms elucidate the role of manganese nanoforms for future biomedical applications.
7. Other applications of manganese nanoparticles
Besides the biomedical applications of manganese nanoparticles, they have various other uses, as reported in the literature.171–175 As mentioned in the previous sections regarding the role of Mn in living systems, Mn nanoparticles are used as nutritional supplements. They have been used for reproduction, skeletal abnormalities, growth, etc. for all living animals. Mn nanoparticles are readily used as aquatic food supplements. For example, Asaikkutti et al. developed biosynthesized Mn3O4 nanoparticles as supplements in the diet of freshwater prawn Macrobrachium rosenbergii.171 The Mn supplements increased the metabolism and antioxidant systems of the prawns, which in turn enhanced their growth and survival.171
Manganese-based nanoforms have been reported to show good electrochemical efficiency and used in lithium-ion batteries together with supercapacitors.172–174 For example, Chen et al. developed a composite containing graphene oxide–manganese oxide nanocrystals, exhibiting electrochemical behavior, and therefore can be used as electrodes for different devices, catalysis reactions and as adsorbents.172 Some reports in the literature regarding manganese nanoparticles exhibited their optical and magnetic properties.176 For example, Djerdj et al. demonstrated the ferromagnetic property of manganese nanoparticles using squid analysis.176 Biswas et al. synthesized manganese-incorporated ZnS nanorods having orange luminescence and broad Lorentzian-shaped EPR spectra (with emissions in the blue, orange and green regions) for emissive device magnetic dipole interaction.177 It is known that the paper and textile industries release a huge amount of non-degradable dye as natural carcinogens and environmental pollutants. In some reports, manganese-based nanoparticles have been shown to have degradation ability for dyes (safranin O and Congo red).175 Manganese has even been used for water purification. The toxic forms of arsenic are considered major water pollutants, which are responsible for hyperpigmentation, circulatory disorders, and skin and liver cancer. For example, Bajpai et al. developed manganese dioxide-coated sand, which helped in the removal of arsenic contamination from water bodies.178 Similarly, Camacho et al. illustrated that manganese dioxide modified with clinoptilolite–Ca zeolite removed arsenic from groundwater.179 In the presence of cerium ammonium nitrate and hydrogen peroxide, manganese oxide nanoparticles possess good catalytic property for water oxidation and epoxidation of olefins. These compounds also perform epoxidation of aromatic and non-aromatic olefins in the presence of bicarbonate ions and H2O2.180
Besides, manganese nanoparticles have a huge impact in agricultural applications including crop growth and ease from abiotic stress, rendering the lowest toxicity compared to their ionic and bulk counterparts.181 Nano metal oxide manganese (NMO–Mn) plays a scavenging role and has been utilized as sorbents and fertilizers to increase the yield of crops. In agricultural systems, Mn immobilized soil contaminants to increase the crop health in staple foods such as wheat, rice, soybean, and maize.182 Accordingly, Ye et al. investigated the ability of manganese oxide nanoparticles to reduce salinity stress in Capsicum annuum to improve stress management in agricultural yield.183 Pradhan et al. studied the detailed biophysical, biochemical and molecular mechanism of manganese nanoparticles on Vigna radiata (mung bean, a leguminous plant).184 The manganese nanoparticles did not impart any toxicity towards the mung bean plant compared to manganese sulphate, which is a commercially available manganese salt. Based on the results, it was concluded that these nanoparticles can be used as a biosafe micronutrient and nanomodulator in the agricultural sector.184 Further, Pradhan et al. investigated the role of nano Mn in non-nodulated plants. They found that nano Mn increased nitrogen assimilation, uptake and metabolism compared to the commercially available Mn sulphate salt.185
Table 2 presents the different biological applications of manganese-based nanoparticles.8,29,32,61,82,95,118–122,131–135,140–143,145,150–153,155–159,161,164,168–171
Table 2 Biological applications of manganese nanoparticles
Manganese (Mn) conjugates |
Biomedical applications |
Ref. |
MnO2–Fe3O4@SiO2, NaYF4:Yb |
Drug carrier, quenchers |
122
|
MnO2 nanosheet–DNAzyme |
Gene slicing, bimodal cancer treatment |
131
|
mMnO2–UCNPs@mSiO2–chlorin e6 |
Trimodal imaging, chemo-photodynamic |
132
|
Mn–CoO–doxorubicin |
Anticancer activity |
133
|
MnFe2O4 Np |
Drug delivery |
134
|
MnO2–Au nanorods |
Efficient delivery of doxorubicin |
135
|
Mn nanoparticle |
Anti-angiogenesis |
140
|
MnO2–sorafenib |
Treatment of cancer, MRI |
141
|
Mn3O4 nanoparticle |
Antibacterial |
143
|
Mn-Cinnamomum verum bark extract |
Antimicrobial activity |
142
|
Mn–curcumin–lemon methanolic extract |
Antibacterial and antifungal activity |
95
|
Manganese ferrite–citric acid |
Antifungal activity |
134
|
MnO–bentonite clay |
Antibacterial and antifungal activity |
145
|
Mn3O4 nanoparticle |
Scavenges ROS |
82
|
MnO extracts of Cussonia zuluensis |
Antioxidant activity |
150
|
MnO2–BSA |
Invitro antioxidant activity |
151
|
MnO2–BSA |
Detect goat anti-human IgG |
118
|
MnO2 nanoparticle |
Detect and quantify GSH |
120
|
MnO2–Janus DNA |
RNA imaging |
121
|
MnO2 nanozyme |
Antioxidant |
119
|
Mn3O4 |
Mimics activity of redox enzymes |
61
|
MnO2 |
Photothermal therapy |
156
|
MnO2–chlorine e6 sensitizer (Ce6)–PEG |
T1 MR contrast agent |
153
|
MnO2–SiO2–methylene blue |
MRI imaging, photothermal therapy |
155
|
MnO2–BSA |
NIR photothermal antitumor therapy |
152
|
Co/MnO2 nanocrystals |
MR imaging, antitumor activity |
157
|
MnCO3@PDA NPs |
MR contrast agent, anticancer |
158
|
Fp–Pd@MnO2 |
Chemo-photothermal therapy |
159
|
Citrate–Mn-doped magnetic nanoclusters |
ROS mediated photothermal therapy |
29
|
MnO2–Sterculia lychnophora |
Alkaline phosphatase detection |
32
|
CaO2/MnO2@PDA–MB |
Cell imaging, photothermal therapy |
161
|
UC–MnO2 |
Fluorescence detection |
164
|
MnO2 nanosheet–HCR |
miRNA detection |
133 and 134 |
MnO–L-cysteine–PEG |
Contrast agents for T1 weighted MRI |
8
|
PEG–MnO–aptamer |
Dual mode imaging (PET and MRI) |
168
|
Mn3O4–PEI–Cu64–folic acid |
Bioimaging |
169
|
Mn3O4–64Cu–TRC105 |
T1 contrast agent |
170
|
Mn3O4 NP |
Food technology |
171
|
8. An update of the toxicological evaluation of manganese nanoparticles
Nanomaterials have emerged as the leading candidates for several biomedical applications. Thus, for their future clinical implications, the toxicity of different nanomaterials should be evaluated.186 The extensive utilization of manganese nanoparticles for various biomedical applications calls for their toxicity assessment both in vitro and in vivo.160,187 There are reports regarding the toxicity studies of the manganese nanoparticles, both in vitro and in vivo.121,188–190 For example, He et al. synthesized manganese dioxide nanosheets-carbon dots with a quenched fluorescent system for sensing glutathione in aqueous solutions.191 The nanocomplex exhibited low cytotoxicity to HeLa cells at a concentration below 30 μg mL−1 within 24 h.191 Similarly, Yan et al. designed and fabricated GQD with manganese nanoforms to exhibit least toxicity towards MCF-7 cell lines at a concentration of 40 μg mL−1.192 Manganese nanoparticles have been found to affect neurobehavior and the functions of dopaminergic neurons. For example, Li et al. intracerebrally injected manganese nanoforms in the substantia nigra and ventral tegmental area of the brain in Sprague-Dawley rats.190 The neurological behavior of the rats was evaluated using a Morris water maze test and immunohistochemistry. The results revealed that the escape latencies of the nanoparticle-treated rat increased with respect to the control. Also, the manganese-treated groups exhibited tyrosine hydroxylase (TH)-positive cell reduction with an increase in inducible nitric oxide synthase (iNOS) and glial fibrillary acidic protein (GFAP) positive cells within the lesion side of the rat brains with respect to the contralateral area. Fig. 10 illustrates that the amount of GFAP-positive cells in the damaged side (Fig. 10A and C) is more than that in the contralateral (Fig. 10B and D) and saline control damaged side (Fig. 10E and F). Therefore, the alteration in the spatial learning techniques of rats in the manganese-treated groups resulted in neuronal dopaminergic dysfunction and activation of astrocytes.190
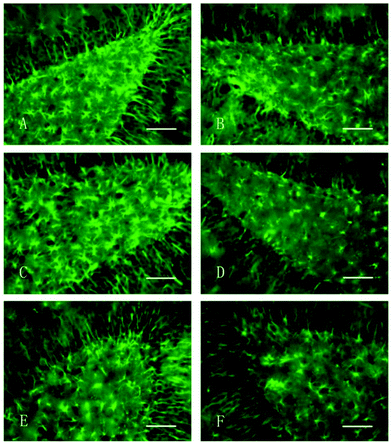 |
| Fig. 10 GFAP immunohistochemical staining of the hippocampus of rats (400×, bar = 50 μm). (A) Damaged side, nano-MnO2 group; (B) uninjured side, nano-MnO2 group; (C) damaged side, 6-OHDA group; (D) uninjured side, 6-OHDA group; (E) damaged side, saline group; and (F) uninjured side, saline group. Reprinted with permission from ref. 190 Li et al., Int. J. Environ. Res. Public Health, 2014, 11, 7918–7930. Copyright©2014 Li et al. | |
There are reports of the clearance of manganese nanoparticles through the renal route. For example, Zhou et al. designed and synthesized very small manganese and gallium-doped copper sulphide nanodots with bovine serum albumin (BSA) as a template for triple modal imaging and photothermal therapy.193 These imaging systems confirmed that the nanoparticles are efficiently cleared from the body via the renal urinary route, thus minimizing their toxicity towards non-tumor organs. Fig. 11 exhibits the renal clearance of the nanoparticles through MR imaging (Fig. 11A) and photoacoustic imaging (Fig. 11B), which shows that the nanoparticles were cleared through the renal route.193 In another work, Xu et al. synthesized manganese–melanin nanoparticles as MRI contrast agents for in vivo tumor targeting.194 Further, the authors claimed that these nanoparticles were found to be less toxic than the clinically approved MRI agent Gadodiamide. The manganese–melanin nanoparticles were also cleared from the body via the hepatobiliary and renal system, proving to be less toxic to the body organs with specific tumor targeting capability.194 Considering that the application of manganese-based nanoparticles has been increasing recently, their effect on the reproductive health of living organisms is a major concern. Accordingly, Yousefalizadegan et al. studied the reproductive toxicity of micro- and nanoforms of manganese dioxide on the spermatogenesis of male Wistar rats.195 Subcutaneous injection of nano/microparticles of manganese dioxide (100 mg kg−1) reduced the number of spermatocytes, sperms, spermatogonia, motility of sperms and diameter of seminiferous tubule. However, there was no significant effect on the production of testosterone, estradiol hormone and the weight of the epididymis, prostate and left testicle.195
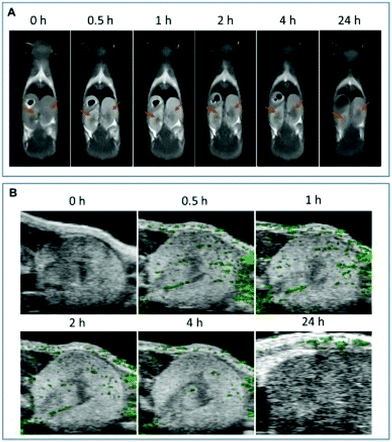 |
| Fig. 11 Renal clearance. (A) In vivo MR imaging of mice kidneys before and after intravenous injection of NDs of varying time. Kidneys are indicated by orange arrows. (B) In vivo PA imaging of mice kidneys before and after intravenous injection of NDs of varying time. Reprinted with permission from ref. 193 Zhou et al., Appl. Mater. Today, 2018, 13, 285–297. Copyright©2018, Elsevier Ltd. All rights reserved. | |
In a different study, Feng et al. investigated the magnetic targeting and tumor microenvironment response of magnetic nanoconjugates with an iron carbide–glucose oxidase–manganese nanoshell.196 The clearance and toxicity profile of the nanoparticles were studied, which showed that intravenous injection of these nanoparticles into mice resulted in time-dependent clearance (especially through the kidney and liver). The histocompatibility profile had no pathological abnormalities in the liver, spleen, heart, kidney, lung. Also, a low impact was observed on hematological parameters such as mean corpuscular hemoglobin, hemoglobin, red blood cells, and hematocrit value. Also, the biochemical assays showed that the liver enzymes alanine aminotransferase (ALT), alkaline phosphatase (ALP) and aspartate aminotransferase (AST) remained normal, indicating healthy liver function.196 In another type of work, Zhu et al. constructed multifunctional nano-sonosensitizers using mesoporous organosilica integrated with MnOx nanoparticles conjugated with cyclic arginine–glycine–aspartic pentapeptide (targeting peptide) and protoporphyrin (sonosensitizer).197 The in vivo excretion and biocompatibility of the nanoforms were evaluated. The results revealed no significant difference in the blood indexes (ALT, AST, ALP, red blood cell count, creatinine, white blood cell count, mean corpuscular hemoglobin concentration, etc.) and body weight upon intravenous injection with the nanoconjugates in a dose-dependent manner. The hepatochemistry, physiochemistry and serum parameters also indicated that the nanoparticles exhibited no toxicity.197
Manganese plays a role in the proper functioning of the nervous system through neurotransmitter uptake and release. However, excess manganese can cause neurotoxicity such as Alzheimer's and Parkinson's disease, a common form of dementia (caused by the formation of neurofibrillary tangles) together with rigidity, tremors, and bradykinesia.198 Imbalanced oxidative stress due to the decreased activity of antioxidant enzymes, induction of nitric oxide synthase in astrocytes, accumulation of inflammatory cytokines and lipid peroxidation mediate Alzheimer's and Parkinson's disease. Accordingly, Tong et al. illustrated that a higher concentration of Mn caused increased plasma amyloid beta peptides, leading to Alzheimer's.199 Excess Mn accumulation leads to a condition called manganism, the phenotypic features of which are analogous to idiopathic Parkinson's disease.200 The Mn-related parkinsonism affects dopaminergic neurons together with monoaminergic neurotransmitters within the basal ganglia and other limbic features.201 Mn also plays a secondary role in mitochondrial dysfunction, which is mainly involved in these neurological diseases.202,203 Mn induces misfolding and accumulation of the alpha-synuclein protein, which plays the primary role in the pathology of Parkinson's disease.204 Therefore, an imbalance of oxidative stress and mitochondrial metabolism is involved in Mn-induced neurotoxicity responsible for neurological disorders. Manganese neurotoxicity was first reported in 1937 in a manganese-based bleach manufacturing company, which was the largest hypochlorite bleaching power producer at that time. Also, during the First World War, 9 patients developed neurological impairment in different continental regions of Europe.40,205,206
In an agricultural area, Pradhan et al. investigated the in vitro and in vivo toxicity of manganese nanoparticles for their utilization as micronutrient in nitrate assimilation compared to the commercially utilized manganese sulfate.185 The manganese nanoparticles were non-toxic to the mice brain mitochondria except for the partial impairment of complex II and III in the electron transport chain, and thus can be used as an alternative nanofertilizer.185 For the first time, Ghosh et al. reported the genotoxic effect of manganese nanoparticles at a high concentration (20 μg mL−1), causing DNA hypomethylation in Physcomitrella patens (moss).207 The DNA strand breaks were analyzed via the comet assay, showing the generation of reactive oxygen species due to the uptake, internalization and dissolution of manganese.207
9. Future perspective and challenges
The advancement of transition metal nanotechnology involving manganese in the field of biology has paved the way towards advanced therapeutic applications in biomedical science.208 Manganese nanoforms having unique structural and functional features are widely explored for various biomedical and other applications.209 Manganese nanoforms have been readily explored for targeted delivery at specific locations, antimicrobial activities, as nanozymes to prevent damage from oxidative species, photothermal therapy and bioimaging activities.27–35
However, despite their various biomedical applications, there are certain drawbacks or challenges regarding manganese-based nanoparticles, which hamper their further progress in the commercial field.186 Firstly, the large-scale production of manganese nanoparticles at the industrial level requires the use of a large amount of organic solvents, high temperature reactors, centrifugation, milling, sonication, filtration, etc.210 A minor change in these reaction-generating conditions can lead to unstable, inactive and undesirable compounds.186 Secondly, nanoparticles due to their small size have a tendency to target non-specific healthy cells together with tissues.186 The targeting by nanoparticles should be specific to decrease their non-specific toxic effects. Thirdly, the permeability and diffusion of nanoparticles through the cell barrier are a crucial challenge related to their uptake and efficacy.186,211,212 The main blockades related to the intravascular delivery of nanoforms are immune rejection, clearance issues from the liver, spleen and kidney, permeability in the target organs, receptor-mediated entry into cells, diffusion through the cytoplasm, and nucleus entry.213 Fourthly, the toxicity of nanoparticles needs to be evaluated given that human health risks associated with the increased emergence of nanoforms are a major challenge for their application. Detailed toxicological evaluations are a major part for the use of nanoparticles.214,215 Fifthly, the immunogenicity (immune response generated inside the animal by nanoparticles) study should be properly assayed prior to their use as diagnostic and therapeutic agents in the near future.124 The biodegradability and clearance of nanoparticles from living systems are a major concern. Liposomal formulations are easily cleared from the body within a short time. However, metal nanoparticles can sometimes exhibit slow metabolic degradation.124,186 Besides, manganese nanoparticles also play a significant role (as macro/micro nutrient) in plant growth and metabolism, where their slower release properties reduce the abiotic stress of plants.216 However, certain challenges such as their complete mechanisms (plant–nanoparticle interaction, transformation, translocation and transportation studies) are poorly understood. Thus, detailed studies are required for crop stress management to improve the agricultural yield.
Future perspectives towards the development of efficient manganese-based nanoparticles for various biomedical applications involve different factors. During the initial development of manganese nanoparticles on the lab scale, suitable approaches should be considered for their large-scale manufacture, considering the synthesis conditions for a larger yield, better quality and higher efficiency of the nanoparticles.124,186 Therefore, factors such as ecofriendly, low cost, biocompatible synthesis, reproducible formulation of the nanoparticle, and characterization are to be tested prior to their commercialization. Also, the production cost should be verified to enter the global market for high quality production strategies. Next the specificity of the nanoparticles should be modified for active targeting using targeting agents such as peptides, integrins, and drugs for increased therapeutic efficacy and less toxic effects.213 Further, the diffusion and entry blockades of nanoparticles should be given the utmost care for their efficient therapeutic ability. The size, shape, charge and other structural and functional parameters should be consistent for proper permeation within target organs. Also, detailed toxicity profile (long-term toxicity study such as bioaccumulation/biodistribution, dosage, renal clearable properties, metabolism, and pharmacodynamic and pharmacokinetic profiles) and immunological studies need to be evaluated properly by using different animal models prior their FDA approval and clinical application.214,217,218 Currently, there are several nanoparticles that are either US FDA approved or undergoing clinical trials. Although most of the nanoformulations that are FDA approved are inclined towards liposomal, polymeric and nanocrystal compounds, there is an increasing trend for the development of protein-based nanoparticles, micelles and inorganic metal nanomaterials for clinical trials.219,220 Therefore, Mn nanoparticles will have future opportunity to be FDA approved drugs for various biomedical applications after their proper bio-safety evaluation in large animals and humans in the future. The earlier discussed Section 8 highlighted several reports of in vitro and in vivo toxicity studies of manganese-based nanoforms. Moreover, the mechanism behind the biodegradability and clearance of metal nanoparticles is currently considered an active area of nanotechnology research. Considering all the above-mentioned facts, manganese-based nanoparticles hold a promising future in biology, medicine and other industrial sectors.
10. Conclusion
Over the last decade, the use of the manganese-based transition metal nanoparticles has revolutionized theranostics applications in the field of biology through their multifunctional features. Referring to its presence in living systems, manganese is widely applied for drug delivery, as antimicrobial agents, anti-angiogenic agents, enzymes, photothermal agents, fluorescence agents, etc. Considering the potential biomedical applications of manganese, the discovery of new, efficient, economically cheap, safe manganese nanomaterials that can be utilized as an alternative treatment strategy for various diseases is urgently required. Therefore, scientists globally are designing new size- and shape-dependent manganese-based nanomaterials by changing the reaction parameters during their synthesis thorough chemical, physical and biological synthetic approaches. Manganese-based nanoforms can be readily utilized in the commercial market in combination with other compounds depending on the advancement stages with optimization and after their proper biosafety evaluation. The other factors including efficacy, metabolic fate, immunogenicity and pharmacokinetic studies should be properly evaluated before their commercial utilization. Considering that manganese offers multiple benefits not only in healthcare but also in the electronics, food, water and textile industries, the current comprehensive review article will attract a wider spectrum of the scientific community (both specialized and non-specialized).
Abbreviations
AA | Ascorbic acid |
AAP | Acidic phosphoric acid |
ALP | Alkaline phosphatase |
ALT | Alanine aminotransferase |
AST | Aspartate aminotransferase |
ATP | Adenosine triphosphate |
BSA | Bovine serum albumin |
CAM | Chorioallantoic membrane assay |
DNA | Deoxyribonucleic acid |
DOX | Doxorubicin |
EDX | Energy dispersive X-ray analysis |
EELS | Electron energy loss spectroscopy |
FESEM | Field emission scanning electron microscopy |
FRET | Fluorescence resonance energy transfer |
GFAP | Glial fibrillary acidic protein |
GSH | Glutathione |
GQD | Graphene quantum dots |
HCC | Hepatocellular carcinoma |
HDL | High-density lipo-protein |
HRP | Horseradish peroxidase |
HRTEM | High resolution transmission electron microscopy |
iNOS | Inducible nitric oxide synthase |
MRI | Magnetic resonance imaging |
NIR | Near infrared |
NPs | Nanoparticles |
PDA | Polydopamine |
PEG | Polyethylene glycol |
PET | Positron emission tomography |
PVP | Polyvinylpyrrolidone |
PS | Photosensitizer |
Mn | Manganese |
MnSOD | Manganese superoxide dismutase |
OPD |
o-Phenylenediamine |
RBC | Red blood cell |
RDI | Reference daily intake |
ROS | Reactive oxygen species |
RNA | Ribonucleic acid |
SEM | Scanning electron microscopy |
SQUID | Superconducting quantum interface device |
TEM | Transmission electron microscopy |
TH | Tyrosine hydroxylase |
TMB | Tetramethylbenzidine |
UC | Upconversion |
U.S. FDA | U.S. Food and Drug Administration |
NRC | National Research Council |
XRD | X-ray diffraction |
Author contributions
The article was written through contributions of all authors. All authors have given approval to the final version of the manuscript.
Conflicts of interest
The authors declare no competing financial interest.
Acknowledgements
CRP is grateful to CSIR, New Delhi for generous financial support (HCP0040). We thank the Director of the CSIR - Indian Institute of Chemical Technology (Ms. No. IICT/Pubs./2021/215) for providing all the required facilities to carry out the work. SH and ST are thankful to the CSIR and UGC, New Delhi for supporting their Research Fellowship, respectively.
Notes and references
- N. Pandey, S. Dhiman, T. Srivastava and S. Majumder, Chem.-Biol. Interact., 2016, 254, 221–230 CrossRef CAS PubMed
.
- W. Zhao, A. Li, A. Zhang, Y. Zheng and J. Liu, ChemMedChem, 2018, 13, 2134–2149 CrossRef CAS PubMed
.
- L. Ricciardi and M. La Deda, SN Appl. Sci., 2021, 3, 372 CrossRef CAS
.
- A. D. Karthik and K. Geetha, Open Access J., 2016, 3, 28–34 Search PubMed
.
- D. J. Bonda, G. Liu, P. Men, G. Perry, M. A. Smith and X. Zhu, CNS Neurol. Disord.: Drug Targets, 2012, 11, 81–85 CrossRef CAS PubMed
.
- M. Wu, J. Shi and H. Deng, Arabian J. Chem., 2018, 11, 924–934 CrossRef CAS
.
- T. E. Stevens, C. J. Pearce, C. N. Whitten, R. P. Grant and T. C. Monson, Sci. Rep., 2017, 7, 44191 CrossRef CAS PubMed
.
- P. Wang, J. Yang, B. Zhou, Y. Hu, L. Xing, F. Xu, M. Shen, G. Zhang and X. Shi, ACS Appl. Mater. Interfaces, 2017, 9, 47–53 CrossRef CAS PubMed
.
- A. Ottmann, M. Scholz, M. Haft, E. Thauer, P. Schneider, M. Gellesch, C. Nowka, S. Wurmehl, S. Hampel and R. Klingeler, Sci. Rep., 2017, 7, 13625 CrossRef PubMed
.
-
Chemistry of the Elements, ed. N. N. Greenwood and A. Earnshaw, Butterworth-Heinemann, Oxford, 2nd edn, 1997, pp. 1040–1069, DOI:10.1016/B978-0-7506-3365-9.50030-4
.
- D. Wang and D. Astruc, Chem. Soc. Rev., 2017, 46, 816–854 RSC
.
- S. K. Ghosh, ACS Omega, 2020, 5, 25493–25504 CrossRef CAS PubMed
.
-
L. H. Madkour, in Reactive Oxygen Species (ROS), Nanoparticles, and Endoplasmic Reticulum (ER) Stress-Induced Cell Death Mechanisms, ed. L. H. Madkour, Academic Press, 2020, pp. 81–105, DOI:10.1016/B978-0-12-822481-6.00004-9
.
-
B. Alloway, Heavy Metals and Metalloids as Micronutrients for Plants and Animals, Environmental Pollution, Springer, Dordrecht, 2013, vol. 22, pp. 195–209 Search PubMed
.
- L. Li and X. Yang, Oxid. Med. Cell. Longevity, 2018, 2018, 7580707–7580707 Search PubMed
.
- J. L. Aschner and M. Aschner, Mol. Aspects Med., 2005, 26, 353–362 CrossRef CAS PubMed
.
- R. A. Beckman, A. S. Mildvan and L. A. Loeb, Biochemistry, 1985, 24, 5810–5817 CrossRef CAS PubMed
.
- F. C. Wedler, Prog. Med. Chem., 1993, 30, 89–133 CrossRef CAS PubMed
.
- L. F. Reichardt and R. B. Kelly, Annu. Rev. Biochem., 1983, 52, 871–926 CrossRef CAS PubMed
.
-
D. Klimis-Zacas, Manganese in health and disease, CRC Press, 1993 Search PubMed
.
-
D. S. Avila, R. L. Puntel and M. Aschner, in Interrelations between essential metal ions and human diseases, Springer, 2013, pp. 199–227 Search PubMed
.
-
Icahn School of Medicine at Mount Sinai, https://icahn.mssm.edu/about/departments/environmental-public-health/divisions/occupational-environmental/manganese, 2021.
- C. King, M. Myrthil, M. Carroll and E. Catapane, In Vivo, 2008, 29, 26–34 Search PubMed
.
- J. W. Finley, P. E. Johnson and L. K. Johnson, Am. J. Clin. Nutr., 1994, 60, 949–955 CrossRef CAS PubMed
.
- T. R. Guilarte, Front. Aging Neurosci., 2013, 5, 23 CAS
.
- X. Cai, Q. Zhu, Y. Zeng, Q. Zeng, X. Chen and Y. Zhan, Int. J. Nanomed., 2019, 14, 8321–8344 CrossRef CAS PubMed
.
- Y. Chen, H. Cong, Y. Shen and B. Yu, Nanotechnology, 2020, 31, 202001 CrossRef CAS PubMed
.
- C. Lopez-Abarrategui, V. Figueroa-Espi, M. B. Lugo-Alvarez, C. D. Pereira, H. Garay, J. A. Barbosa, R. Falcão, L. Jiménez-Hernández, O. Estévez-Hernández, E. Reguera, O. L. Franco, S. C. Dias and A. J. Otero-Gonzalez, Int. J. Nanomed., 2016, 11, 3849–3857 CrossRef CAS PubMed
.
- R. Gupta and D. Sharma, ACS Appl. Nano Mater., 2020, 3, 2026–2037 CrossRef CAS
.
- A. Chowdhury, S. Kunjiappan, T. Panneerselvam, B. Somasundaram and C. Bhattacharjee, Int. Nano Lett., 2017, 7, 91–122 CrossRef CAS
.
- S. M. Hussain, A. K. Javorina, A. M. Schrand, H. M. Duhart, S. F. Ali and J. J. Schlager, Toxicol. Sci., 2006, 92, 456–463 CrossRef CAS PubMed
.
- F. Qu, H. Pei, R. Kong, S. Zhu and L. Xia, Talanta, 2017, 165, 136–142 CrossRef CAS PubMed
.
- S. Khan, A. A. Ansari, A. A. Khan, M. Abdulla, O. Al-Obeed and R. Ahmad, MedChemComm, 2016, 7, 1647–1653 RSC
.
- D. Jiang, D. Ni, Z. T. Rosenkrans, P. Huang, X. Yan and W. Cai, Chem. Soc. Rev., 2019, 48, 3683–3704 RSC
.
- J. Jankowski, K. Ognik, A. Stępniowska, Z. Zduńczyk and K. Kozłowski, PLoS One, 2018, 13, e0201487 CrossRef PubMed
.
- N. Polley, S. Saha, A. Adhikari, S. Banerjee, S. Darbar, S. Das and S. K. Pal, Nanomedicine, 2015, 10, 2349–2363 CrossRef CAS PubMed
.
- Y. Hao, L. Wang, B. Zhang, D. Li, D. Meng, J. Shi, H. Zhang, Z. Zhang and Y. Zhang, Int. J. Nanomed., 2016, 11, 1759 CrossRef CAS PubMed
.
- S. D. Anderson, V. V. Gwenin and C. D. Gwenin, Nanoscale Res. Lett., 2019, 14, 1–16 CrossRef CAS PubMed
.
- B. Ding, S. Shao, C. Yu, B. Teng, M. Wang, Z. Cheng, K. L. Wong, P. A. Ma and J. Lin, Adv. Mater., 2018, 30, 1802479 CrossRef PubMed
.
- L. Alessio, M. Campagna and R. Lucchini, Am. J. Ind. Med., 2007, 50, 779–787 CrossRef CAS PubMed
.
- Journal. Asian metal, Metalpedia. Weblink: http://metalpedia.asianmetal.com/metal/manganese/history.shtml.
- A. Sharma and R. M. Singh, Heritage, 2021, 4, 1970–1994 CrossRef
.
- Journal. RSC, Weblink: https://www.rsc.org/periodic-table/element/25/manganese.
- A. R. Reddi, L. T. Jensen and V. C. Culotta, Chem. Rev., 2009, 109, 4722–4732 CrossRef CAS PubMed
.
- P. D. Blanc, NeuroToxicology, 2018, 64, 5–11 CrossRef CAS PubMed
.
- M. M. Thackeray, J. R. Croy, E. Lee, A. Gutierrez, M. He, J. S. Park, B. T. Yonemoto, B. R. Long, J. D. Blauwkamp, C. S. Johnson, Y. Shin and W. I. F. David, Sustainable Energy Fuels, 2018, 2, 1375–1397 RSC
.
- O. Sparenberg, Extr. Ind. Soc., 2019, 6, 842–854 Search PubMed
.
- K. J. Horning, S. W. Caito, K. G. Tipps, A. B. Bowman and M. Aschner, Annu. Rev. Nutr., 2015, 35, 71–108 CrossRef CAS PubMed
.
- J. Roth, S. Ponzoni and M. Aschner, Met. Ions Life Sci., 2013, 12, 169–201 Search PubMed
.
- T. E. Kehl-Fie and E. P. Skaar, Curr. Opin. Chem. Biol., 2010, 14, 218–224 CrossRef CAS PubMed
.
- H. Haase, Immunity, 2018, 48, 616–618 CrossRef CAS PubMed
.
- R. H. Hartman, G. Matrone and G. H. Wise, J. Nutr., 1955, 57, 429–439 CrossRef CAS PubMed
.
- E. B. Kurutas, Nutr. J., 2016, 15, 71–71 CrossRef PubMed
.
-
A. Blanco and G. Blanco, in Med. Biochem., ed. A. Blanco and G. Blanco, Academic Press, 2017, pp. 153–175, DOI:10.1016/B978-0-12-803550-4.00008-2
.
-
R. B. Rucker, A. J. Fascetti and C. L. Keen, in Clin. Biochem. Domestic Animals, ed. J. J. Kaneko, J. W. Harvey and M. L. Bruss, Academic Press, San Diego, 6th edn, 2008, pp. 663–693, DOI:10.1016/B978-0-12-370491-7.00022-2
.
- P. Chen, J. Bornhorst and M. Aschner, Front. Biosci.-Landmark, 2018, 23, 1655–1679 CrossRef CAS PubMed
.
-
C. Molina-Poveda, in Aquafeed Formulation, ed. S. F. Nates, Academic Press, San Diego, 2016, pp. 75–216, DOI:10.1016/B978-0-12-800873-7.00004-X
.
-
C. L. Keen and S. Zidenberg-Cherr, in Encyclopedia of Food Sciences and Nutrition, ed. B. Caballero, Academic Press, Oxford, 2nd edn, 2003, pp. 3686–3691, DOI:10.1016/B0-12-227055-X/00732-X
.
-
I. I. Ahmetov and O. N. Fedotovskaya, in Adv. Clin. Chem., ed. G. S. Makowski, Elsevier, 2015, vol. 70, pp. 247–314 Search PubMed
.
-
I. Rahman and S. K. Biswas, in Encyclopedia of Respiratory Medicine, ed. G. J. Laurent and S. D. Shapiro, Academic Press, Oxford, 2006, pp. 258–266, DOI:10.1016/B0-12-370879-6/00283-0
.
- N. Singh, M. Geethika, S. M. Eswarappa and G. Mugesh, Chem. – Eur. J., 2018, 24, 8393–8403 CrossRef CAS PubMed
.
- L.-Y. Chau and H.-H. Tai, Biochim. Biophys. Acta, Lipids Lipid Metab., 1988, 963, 436–444 CrossRef CAS
.
- A. R. Kornblihtt, M. M. Flawia and H. N. Torres, Biochemistry, 1981, 20, 1262–1267 CrossRef CAS PubMed
.
- J. Sekiguchi and S. Shuman, Biochemistry, 1997, 36, 9073–9079 CrossRef CAS PubMed
.
-
A. Balakrishnan, M. Padigaru and A. Morde, in Nutraceuticals in Brain Health and Beyond, ed. D. Ghosh, Academic Press, 2021, pp. 281–292, DOI:10.1016/B978-0-12-820593-8.00019-7
.
- D. Leu, I. Spasojevic, H. Nguyen, B. Deng, A. Tovmasyan, T. Weitner, R. S. Sampaio, I. Batinic-Haberle and T.-T. Huang, Redox Biol., 2017, 12, 864–871 CrossRef CAS PubMed
.
- J. G. Anderson, S. C. Fordahl, P. T. Cooney, T. L. Weaver, C. L. Colyer and K. M. Erikson, Brain Res., 2009, 1281, 1–14 CrossRef CAS PubMed
.
- D. L. Vesely, Mol. Cell. Biochem., 1985, 66, 145–149 CrossRef CAS PubMed
.
- J. K. Bashkin and L. A. Jenkins, Comments Inorg. Chem., 1994, 16, 77–93 CrossRef CAS
.
- D. S. Harischandra, S. Ghaisas, G. Zenitsky, H. Jin, A. Kanthasamy, V. Anantharam and A. G. Kanthasamy, Front. Neurosci., 2019, 13, 654 CrossRef PubMed
.
- A. M. Polyanichko, V. V. Andrushchenko, E. V. Chikhirzhina, V. I. Vorob'ev and H. Wieser, Nucleic Acids Res., 2004, 32, 989–996 CrossRef CAS PubMed
.
-
E. H. Chu and W. M. Generoso, Mutation, cancer, and malformation, Springer Science & Business Media, 2012 Search PubMed
.
- M. Gross and D. A. Kaplansky, Biochim. Biophys. Acta, 1983, 740, 255–263 CrossRef CAS
.
- D. L. Baly, C. L. Keen and L. S. Hurley, Biol. Trace Elem. Res., 1986, 11, 201–212 CrossRef CAS PubMed
.
- G. S. Shukla and S. V. Chandra, Acta Pharmacol. Toxicol., 1982, 51, 209–216 CrossRef CAS PubMed
.
- M. R. Bryan and A. B. Bowman, Adv. Neurobiol., 2017, 18, 113–142 Search PubMed
.
- E. de Lamirande and G. L. Plaa, Arch. Int. Pharmacodyn. Ther., 1979, 239, 24–35 CAS
.
-
T. P. Moyer, in Pharmacol. Ther., ed. S. A. Waldman, A. Terzic, L. J. Egan, J.-L. Elghozi, A. Jahangir, G. C. Kane, W. K. Kraft, L. D. Lewis, J. D. Morrow, L. V. Zingman, D. R. Abernethy, A. J. Atkinson, N. L. Benowitz, D. C. Brater, J. Gray, P. K. Honig, G. L. Kearns, B. A. Levey, S. P. Spielberg, R. Weinshilboum and R. L. Woosley, W. B. Saunders, Philadelphia, 2009, pp. 1213–1220, DOI:10.1016/B978-1-4160-3291-5.50090-1
.
- J. Kawano, D. M. Ney, C. L. Keen and B. O. Schneeman, J. Nutr., 1987, 117, 902–906 CrossRef CAS PubMed
.
- K. J. Thompson, J. Hein, A. Baez, J. C. Sosa and M. Wessling-Resnick, Am. J. Physiol.: Gastrointest. Liver Physiol., 2018, 315, G351–G363 CrossRef CAS PubMed
.
- L. Tillman, Biosci. Horiz.: Int. Dent. J. Stud. Res., 2019, 11, DOI:10.1093/biohorizons/hzy012
.
- J. Yao, Y. Cheng, M. Zhou, S. Zhao, S. Lin, X. Wang, J. Wu, S. Li and H. Wei, Chem. Sci., 2018, 9, 2927–2933 RSC
.
- Q. Ye, J. E. Park, K. Gugnani, S. Betharia, A. Pino-Figueroa and J. Kim, Metallomics: Integr. Biometal Sci., 2017, 9, 1028–1046 CrossRef CAS PubMed
.
- J. S. Crossgrove and R. A. Yokel, Neurotoxicology, 2004, 25, 451–460 CrossRef CAS PubMed
.
- L. S. Hurley, C. L. Keen and D. L. Baly, Neurotoxicology, 1984, 5, 97–104 CAS
.
- P. L. Goering and C. D. Klaassen, Biochem. Pharmacol., 1985, 34, 1371–1379 CrossRef CAS PubMed
.
- E. S. Koh, S. J. Kim, H. E. Yoon, J. H. Chung, S. Chung, C. W. Park, Y. S. Chang and S. J. Shin, BMC Endocr. Disord., 2014, 14, 24–24 CrossRef PubMed
.
- A. H. Rubenstein, N. W. Levin and G. A. Elliott, Lancet, 1962, 2, 1348–1351 CrossRef CAS
.
- D. L. Baly, D. L. Curry, C. L. Keen and L. S. Hurley, J. Nutr., 1984, 114, 1438–1446 CrossRef CAS PubMed
.
- J. H. Gong, K. Lo, Q. Liu, J. Li, S. Lai, A. H. Shadyab, C. Arcan, L. Snetselaar and S. Liu, Diabetes Care, 2020, 43, 1344 CrossRef CAS PubMed
.
- D. L. Baly, J. S. Schneiderman and A. L. Garcia-Welsh, J. Nutr., 1990, 120, 1075–1079 CrossRef CAS PubMed
.
- E. Burlet and S. K. Jain, J. Biol. Chem., 2013, 288, 6409–6416 CrossRef CAS PubMed
.
- N. Wang, X. Cao, G. Lin and Y. Shihe, Nanotechnology, 2007, 18, 475605 CrossRef
.
- Y. Chen, Y. Hong, Y. Ma and J. Li, J. Alloys Compd., 2010, 490, 331–335 CrossRef CAS
.
- M. Jayandran, M. M. Haneefa and V. Balasubramanian, J. Appl. Pharm. Sci., 2015, 5, 105–110 CrossRef CAS
.
- V. Kumar, K. Singh, S. Panwar and S. K. Mehta, Int. Nano Lett., 2017, 7, 123–131 CrossRef CAS
.
- A. Sinha, V. N. Singh, B. R. Mehta and S. K. Khare, J. Hazard. Mater., 2011, 192, 620–627 CrossRef CAS PubMed
.
- A. Sukhdev, M. Challa, L. Narayani, A. S. Manjunatha, P. R. Deepthi, J. V. Angadi, P. Mohan Kumar and M. Pasha, Heliyon, 2020, 6, e03245 CrossRef PubMed
.
- X. Liu, C. Chen, Y. Zhao and B. Jia, J. Nanomater., 2013, 2013, 736375 Search PubMed
.
- T. Soejima, K. Nishizawa and R. Isoda, J. Colloid Interface Sci., 2018, 510, 272–279 CrossRef CAS PubMed
.
- D. Ghosh, S. Bhandari and D. Khastgir, Phys. Chem. Chem. Phys., 2016, 18, 32876–32890 RSC
.
- M. B. Ward, R. Brydson and R. F. Cochrane, J. Phys.: Conf. Ser., 2006, 26, 296–299 CrossRef
.
- C. Martinez de la Torre, J. H. Grossman, A. A. Bobko and M. F. Bennewitz, PLoS One, 2020, 15, e0239034 CrossRef CAS PubMed
.
- B. Ding, P. Zheng, P. A. Ma and J. Lin, Adv. Mater., 2020, 32, 1905823 CrossRef CAS PubMed
.
- T. Simao, D. M. Chevrier, J. Jakobi, A. Korinek, G. Goupil, M. Lau, S. Garbarino, P. Zhang, S. Barcikowski, M.-A. Fortin and D. Guay, J. Phys. Chem. C, 2016, 120, 22635–22645 CrossRef CAS
.
- J.-G. Lee, P. Li, C.-J. Choi and X.-L. Dong, Thin Solid Films, 2010, 519, 81–85 CrossRef CAS
.
- V. Hoseinpour and N. Ghaemi, J. Photochem. Photobiol., B, 2018, 189, 234–243 CrossRef CAS PubMed
.
- S. Waghmare, A. Deshmukh, S. Kulkarni and L. Oswaldo, Univers. J. Environ. Res. Technol., 2011, 1, 64–69 CAS
.
- J. Singh, T. Dutta, K.-H. Kim, M. Rawat, P. Samddar and P. Kumar, J. Nanobiotechnol., 2018, 16, 84 CrossRef CAS PubMed
.
- D. Zhang, X.-L. Ma, Y. Gu, H. Huang and G.-W. Zhang, Front. Chem., 2020, 8, 799 CrossRef CAS PubMed
.
- S. Iravani, Green Chem., 2011, 13, 2638–2650 RSC
.
- S. Jana, S. Pande, A. K. Sinha, S. Sarkar, M. Pradhan, M. Basu, S. Saha and T. Pal, J. Phys. Chem. C, 2009, 113, 1386–1392 CrossRef CAS
.
- J. R. Carney, B. R. Dillon and S. P. Thomas, Eur. J. Org. Chem., 2016, 3912–3929 CrossRef CAS
.
- S. Zhu, S.-H. Ho, C. Jin, X. Duan and S. Wang, Environ. Sci. Nano, 2020, 7, 368–396 RSC
.
- R. Dardouri, A. Gannouni and M. S. Zina, Adv. Mater. Sci. Eng., 2019, 2019, 6024876 Search PubMed
.
- S. Dey and V. V. Praveen Kumar, Curr. Opin. Green Sustainable Chem., 2020, 3, 100012 CrossRef
.
- A. J. Wu, J. E. Penner-Hahn and V. L. Pecoraro, Chem. Rev., 2004, 104, 903–938 CrossRef CAS PubMed
.
- X. Liu, Q. Wang, H. Zhao, L. Zhang, Y. Su and Y. Lv, Analyst, 2012, 137, 4552–4558 RSC
.
- N. Singh, M. A. Savanur, S. Srivastava, P. D'Silva and G. Mugesh, Nanoscale, 2019, 11, 3855–3863 RSC
.
- J. Liu, L. Meng, Z. Fei, P. J. Dyson, X. Jing and X. Liu, Biosens. Bioelectron., 2017, 90, 69–74 CrossRef CAS PubMed
.
- F. Chen, M. Bai, Y. Zhao, K. Cao, X. Cao and Y. Zhao, Anal. Chem., 2018, 90, 2271–2276 CrossRef CAS PubMed
.
- P. Zhao, Y. Zhu, X. Yang, J. Shen, X. Jiang, J. Zong and C. Li, Dalton Trans., 2014, 43, 451–457 RSC
.
- A. Greene, J. Hashemi and Y. Kang, Nanotechnology, 2020, 32, 025713 CrossRef PubMed
.
- J. K. Patra, G. Das, L. F. Fraceto, E. V. R. Campos, M. D. P. Rodriguez-Torres, L. S. Acosta-Torres, L. A. Diaz-Torres, R. Grillo, M. K. Swamy, S. Sharma, S. Habtemariam and H.-S. Shin, J. Nanobiotechnol., 2018, 16, 71–71 CrossRef PubMed
.
- L. Yan, J. Shen, J. Wang, X. Yang, S. Dong and S. Lu, Dose-Response, 2020, 18, 1559325820936161–1559325820936161 CAS
.
- S. Senapati, A. K. Mahanta, S. Kumar and P. Maiti, Signal Transduction Targeted Ther., 2018, 3, 7 CrossRef PubMed
.
- M. J. Mitchell, M. M. Billingsley, R. M. Haley, M. E. Wechsler, N. A. Peppas and R. Langer, Nat. Rev. Drug Discovery, 2021, 20, 101–124 CrossRef CAS PubMed
.
- M. U. Farooq, V. Novosad, E. A. Rozhkova, H. Wali, A. Ali, A. A. Fateh, P. B. Neogi, A. Neogi and Z. Wang, Sci. Rep., 2018, 8, 2907 CrossRef PubMed
.
- I. Ghiuţă and D. Cristea, Adv. Drug Delivery Rev., 2020, 347–371, DOI:10.1016/B978-0-08-102985-5.00015-2
.
- X. Zeng, J. Sun, S. Li, J. Shi, H. Gao, W. Sun Leong, Y. Wu, M. Li, C. Liu, P. Li, J. Kong, Y.-Z. Wu, G. Nie, Y. Fu and G. Zhang, Nat. Commun., 2020, 11, 567 CrossRef CAS PubMed
.
- H. Fan, Z. Zhao, G. Yan, X. Zhang, C. Yang, H. Meng, Z. Chen, H. Liu and W. Tan, Angew. Chem., Int. Ed., 2015, 54, 4801–4805 CrossRef CAS PubMed
.
- J. Xu, W. Han, P. Yang, T. Jia, S. Dong, H. Bi, A. Gulzar, D. Yang, S. Gai, F. He, J. Lin and C. Li, Adv. Funct. Mater., 2018, 28, 1803804 CrossRef
.
- Q. Ren, K. Yang, R. Zou, Z. Wan, Z. Shen, G. Wu, Z. Zhou, Q. Ni, W. Fan, J. Hu and Y. Liu, Nanoscale, 2019, 11, 23021–23026 RSC
.
- G. Wang, D. Zhao, Y. Ma, Z. Zhang, H. Che, J. Mu, X. Zhang and Z. Zhang, Appl. Surf. Sci., 2018, 428, 258–263 CrossRef CAS
.
- Z. Zhang and Y. Ji, Ind. Eng. Chem. Res., 2019, 58, 2991–2999 CrossRef CAS
.
- T. Tonini, F. Rossi and P. P. Claudio, Oncogene, 2003, 22, 6549–6556 CrossRef CAS PubMed
.
- A. K. Barui, S. K. Nethi, S. Haque, P. Basuthakur and C. R. Patra, ACS Appl. Bio Mater., 2019, 2, 5492–5511 CrossRef CAS
.
- G. Niu and X. Chen, Curr. Drug Targets, 2010, 11, 1000–1017 CrossRef CAS PubMed
.
- R. I. Teleanu, C. Chircov, A. M. Grumezescu and D. M. Teleanu, J. Clin. Med., 2020, 9, 84 CrossRef CAS PubMed
.
- M. Majidifard and M. Hassanpour-Ezatti, Daneshvar Med., 2016, 23, 21–28 CrossRef
.
- C.-C. Chang, T. K. Dinh, Y.-A. Lee, F.-N. Wang, Y.-C. Sung, P.-L. Yu, S.-C. Chiu, Y.-C. Shih, C.-Y. Wu and Y.-D. Huang, ACS Appl. Bio Mater., 2020, 12, 44407–44419 CrossRef CAS PubMed
.
- U. Kamran, H. N. Bhatti, M. Iqbal, S. Jamil and M. Zahid, J. Mol. Struct., 2019, 1179, 532–539 CrossRef CAS
.
- E. Azhir, R. Etefagh, N. Shahtahmasebi, M. Mashreghi and P. Pordeli, Phys. Chem. Res., 2015, 3, 197–204 CAS
.
- Y. Lin, H. Betts, S. Keller, K. Cariou and G. Gasser, Chem. Soc. Rev., 2021, 50, 10346–10402 RSC
.
- B. Krishnan and S. Mahalingam, Res. Chem. Intermed., 2017, 43, 2351–2365 CrossRef CAS
.
- L. Singh, H. G. Kruger, G. E. M. Maguire, T. Govender and R. Parboosing, Ther. Adv. Infect. Dis., 2017, 4, 105–131 CAS
.
- K. Maduray and R. Parboosing, Biol. Trace Elem. Res., 2021, 199, 3159–3176 CrossRef CAS PubMed
.
- M. V. Nesterenko, K. M. Woods and S. J. Upton, Biol. Trace Elem. Res., 1997, 56, 243–253 CrossRef CAS PubMed
.
- J.-P. Vartanian, M. Sala, M. Henry, S. Wain-Hobson and A. Meyerhans, J. Gen. Virol., 1999, 80, 1983–1986 CrossRef CAS PubMed
.
- N. T. Mahlangeni, J. Magura, R. Moodley, H. Baijnath and H. Chenia, Chem. Pap., 2020, 74, 4253–4265 CrossRef CAS
.
- S. Pardhiya, E. Priyadarshini and P. Rajamani, SN Appl. Sci., 2020, 2, 1–12 Search PubMed
.
- Y. Wang, Y. Song, G. Zhu, D. Zhang and X. Liu, Chin. Chem. Lett., 2018, 29, 1685–1688 CrossRef CAS
.
- W. Zhu, Z. Dong, T. Fu, J. Liu, Q. Chen, Y. Li, R. Zhu, L. Xu and Z. Liu, Adv. Funct. Mater., 2016, 26, 5490–5498 CrossRef CAS
.
- Z. Yu, Q. Li, J. Wang, Y. Yu, Y. Wang, Q. Zhou and P. Li, Nanoscale Res. Lett., 2020, 15, 1–14 CrossRef PubMed
.
- Z. Ma, X. Jia, J. Bai, Y. Ruan, C. Wang, J. Li, M. Zhang and X. Jiang, Adv. Funct. Mater., 2017, 27, 1604258 CrossRef
.
- J. Peng, Q. Yang, W. Li, L. Tan, Y. Xiao, L. Chen, Y. Hao and Z. Qian, ACS Appl. Mater. Interfaces, 2017, 9, 44410–44422 CrossRef CAS PubMed
.
- J. Liu, G. Wu, Z. Tang, Q. Sun, J. Wu and R. Lv, Front. Mater., 2019, 6, 286 CrossRef
.
- Y. Cheng, S. Zhang, N. Kang, J. Huang, X. Lv, K. Wen, S. Ye, Z. Chen, X. Zhou and L. Ren, ACS Appl. Mater. Interfaces, 2017, 9, 19296–19306 CrossRef CAS PubMed
.
- C. Sun, Y. Liu, R. Zhou, L. Yao, R. Wang, W. Zang and W. Meng, ACS Appl. Bio Mater., 2019, 2, 4747–4755 CrossRef CAS
.
- M. Wu, P. Hou, L. Dong, L. Cai, Z. Chen, M. Zhao and J. Li, Int. J. Nanomed., 2019, 14, 4781–4800 CrossRef CAS PubMed
.
- C. Ji, Z. Lu, Y. Xu, B. Shen, S. Yu and D. Shi, J. Biomed. Mater. Res., Part B, 2018, 106, 2544–2552 CrossRef CAS PubMed
.
- J. Li, D. Li, R. Yuan and Y. Xiang, ACS Appl. Mater. Interfaces, 2017, 9, 5717–5724 CrossRef CAS PubMed
.
- J. H. Han, L. Sudheendra and M. I. Kennedy, Anal. Bioanal. Chem., 2015, 407, 5243–5247 CrossRef CAS PubMed
.
- J. Yuan, Y. Cen, X.-J. Kong, S. Wu, C.-L. Liu, R.-Q. Yu and X. Chu, ACS Appl. Mater. Interfaces, 2015, 7, 10548–10555 CrossRef CAS PubMed
.
- Z. Zhen and J. Xie, Theranostics, 2012, 2, 45 CrossRef CAS PubMed
.
- C. M. de la Torre and M. F. Bennewitz, J. Visualized Exp., 2020, e61572 CAS
.
- B. Song, W. Shi, W. Shi, X. Qin, H. Ma, M. Tan, W. Zhang, L. Guo and J. Yuan, Nanoscale, 2019, 11, 6784–6793 RSC
.
- J. Li, C. Wu, P. Hou, M. Zhang and K. Xu, Biosens. Bioelectron., 2018, 102, 1–8 CrossRef CAS PubMed
.
- J. Zhu, H. Li, Z. Xiong, M. Shen, P. S. Conti, X. Shi and K. Chen, ACS Appl. Mater. Interfaces, 2018, 10, 34954–34964 CrossRef CAS PubMed
.
- Y. Zhan, S. Shi, E. B. Ehlerding, S. A. Graves, S. Goel, J. W. Engle, J. Liang, J. Tian and W. Cai, ACS Appl. Mater. Interfaces, 2017, 9, 38304–38312 CrossRef CAS PubMed
.
- A. Asaikkutti, P. S. Bhavan, K. Vimala, M. Karthik and P. Cheruparambath, J. Trace Elem. Med. Biol., 2016, 35, 7–17 CrossRef CAS PubMed
.
- S. Chen, J. Zhu, X. Wu, Q. Han and X. Wang, ACS Nano, 2010, 4, 2822–2830 CrossRef CAS PubMed
.
- H.-Y. Wang, F.-X. Xiao, L. Yu, B. Liu and X. W. Lou, Small, 2014, 10, 3181–3186 CrossRef CAS PubMed
.
- B. Xu, C. R. Fell, M. Chi and Y. S. Meng, Energy Environ. Sci., 2011, 4, 2223–2233 RSC
.
- S. A. Moon, B. K. Salunke, B. Alkotaini, E. Sathiyamoorthi and B. S. Kim, IET Nanobiotechnol., 2015, 9, 220–225 CrossRef PubMed
.
- I. Djerdj, D. Arčon, Z. Jagličić and M. Niederberger, J. Phys. Chem. C, 2007, 111, 3614–3623 CrossRef CAS
.
- S. Biswas, S. Kar and S. Chaudhuri, J. Phys. Chem. B, 2005, 109, 17526–17530 CrossRef CAS PubMed
.
- S. Bajpai and M. Chaudhuri, Int. J. Environ. Eng., 1999, 125, 782–784 CrossRef CAS
.
- L. M. Camacho, R. R. Parra and S. Deng, J. Hazard. Mater., 2011, 189, 286–293 CrossRef CAS PubMed
.
- M. M. Najafpour, F. Rahimi, M. Amini, S. Nayeri and M. Bagherzadeh, Dalton Trans., 2012, 41, 11026–11031 RSC
.
- Y. Ye, I. A. Medina-Velo, K. Cota-Ruiz, F. Moreno-Olivas and J. L. Gardea-Torresdey, Ecotoxicol. Environ. Saf., 2019, 184, 109671 CrossRef CAS PubMed
.
- E. Gillispie, S. Taylor, N. Qafoku and M. Hochella, Environ. Chem., 2019, 16, 377–390 CrossRef CAS
.
- Y. Ye, K. Cota-Ruiz, J. A. Hernández-Viezcas, C. Valdés, I. A. Medina-Velo, R. S. Turley, J. R. Peralta-Videa and J. L. Gardea-Torresdey, ACS Sustainable Chem. Eng., 2020, 8, 1427–1436 CrossRef
.
- S. Pradhan, P. Patra, S. Das, S. Chandra, S. Mitra, K. K. Dey, S. Akbar, P. Palit and A. Goswami, Environ. Sci. Technol., 2013, 47, 13122–13131 CrossRef CAS PubMed
.
- S. Pradhan, P. Patra, S. Mitra, K. K. Dey, S. Jain, S. Sarkar, S. Roy, P. Palit and A. Goswami, J. Agric. Food Chem., 2014, 62, 8777–8785 CrossRef CAS PubMed
.
- S. Mukherjee and C. R. Patra, Nanoscale, 2016, 8, 12444–12470 RSC
.
-
D. Milatovic and R. C. Gupta, in Veterinary Toxicology, ed. R. C. Gupta, Academic Press, 3rd edn, 2018, pp. 445–454, DOI:10.1016/B978-0-12-811410-0.00030-1
.
- M. Ou, J. Huang, X. Yang, X. He, K. Quan, Y. Yang, N. Xie, J. Li and K. Wang, ChemBioChem, 2018, 19, 147–152 CrossRef CAS PubMed
.
- I. Razumov, E. Zav'yalov, S. Y. Troitskii, A. Romashchenko, D. Petrovskii, K. Kuper and M. Moshkin, Bull. Exp. Biol. Med., 2017, 163, 561–565 CrossRef CAS PubMed
.
- T. Li, T. Shi, X. Li, S. Zeng, L. Yin and Y. Pu, Int. J. Environ. Res. Public Health, 2014, 11, 7918–7930 CrossRef CAS PubMed
.
- D. He, X. Yang, X. He, K. Wang, X. Yang, X. He and Z. Zou, Chem. Commun., 2015, 51, 14764–14767 RSC
.
- X. Yan, Y. Song, C. Zhu, J. Song, D. Du, X. Su and Y. Lin, ACS Appl. Mater. Interfaces, 2016, 8, 21990–21996 CrossRef CAS PubMed
.
- B. Zhou, J. Zhao, Y. Qiao, Q. Wei, J. He, W. Li, D. Zhong, F. Ma, Y. Li and M. Zhou, Appl. Mater. Today, 2018, 13, 285–297 CrossRef
.
- W. Xu, J. Sun, L. Li, X. Peng, R. Zhang and B. Wang, Biomater. Sci., 2018, 6, 207–215 RSC
.
- N. Yousefalizadegan, Z. Mousavi, T. Rastegar, Y. Razavi and P. Najafizadeh, Int. J. Reprod. Biomed., 2019, 17, 361 CAS
.
- L. Feng, R. Xie, C. Wang, S. Gai, F. He, D. Yang, P. Yang and J. Lin, ACS Nano, 2018, 12, 11000–11012 CrossRef CAS PubMed
.
- P. Zhu, Y. Chen and J. Shi, ACS Nano, 2018, 12, 3780–3795 CrossRef CAS PubMed
.
- A. C. Martins Jr., P. Morcillo, O. M. Ijomone, V. Venkataramani, F. E. Harrison, E. Lee, A. B. Bowman and M. Aschner, Int. J. Environ. Res. Public Health, 2019, 16, 3546 CrossRef PubMed
.
- Y. Tong, H. Yang, X. Tian, H. Wang, T. Zhou, S. Zhang, J. Yu, T. Zhang, D. Fan, X. Guo, T. Tabira, F. Kong, Z. Chen, W. Xiao and D. Chui, J. Alzheimer's Dis., 2014, 42, 865–878 CAS
.
- H. Dieter, T. Bayer and G. Multhaup, Acta Hydrochim. Hydrobiol., 2005, 33, 72–78 CrossRef CAS
.
- V. A. Fitsanakis, C. Au, K. M. Erikson and M. Aschner, Neurochem. Int., 2006, 48, 426–433 CrossRef CAS PubMed
.
- N. Ammal Kaidery and B. Thomas, Neurochem. Int., 2018, 117, 91–113 CrossRef CAS PubMed
.
- D. Milatovic, Z. Yin, R. C. Gupta, M. Sidoryk, J. Albrecht, J. L. Aschner and M. Aschner, Toxicol. Sci., 2007, 98, 198–205 CrossRef CAS PubMed
.
- T. V. Peres, M. R. Schettinger, P. Chen, F. Carvalho, D. S. Avila, A. B. Bowman and M. Aschner, BMC Pharmacol. Toxicol., 2016, 17, 57 CrossRef PubMed
.
- R. G. Lucchini, C. J. Martin and B. C. Doney, NeuroMol. Med., 2009, 11, 311–321 CrossRef CAS PubMed
.
- R. G. Lucchini, S. Guazzetti, S. Zoni, F. Donna, S. Peter, A. Zacco, M. Salmistraro, E. Bontempi, N. J. Zimmerman and D. R. Smith, Neurotoxicology, 2012, 33, 687–696 CrossRef CAS PubMed
.
- I. Ghosh, A. Sadhu, Y. Moriyasu, M. Bandyopadhyay and A. Mukherjee, Mutat. Res., Genet. Toxicol. Environ. Mutagen., 2019, 842, 146–157 CrossRef CAS PubMed
.
- A. Kaushik, Front. Nanotechnol., 2019, 1, 1 CrossRef
.
- Y. Yang, Z. Qin, W. Zeng, T. Yang, Y. Cao, C. Mei and Y. Kuang, Nanotechnol. Rev., 2017, 6, 279–289 CAS
.
- N. Desai, AAPS J., 2012, 14, 282–295 CrossRef CAS PubMed
.
- D. Rosenblum, N. Joshi, W. Tao, J. M. Karp and D. Peer, Nat. Commun., 2018, 9, 1410 CrossRef PubMed
.
- S. P. Singh, M. Kumari, S. I. Kumari, M. F. Rahman, M. Mahboob and P. Grover, J. Appl. Toxicol., 2013, 33, 1165–1179 CrossRef CAS PubMed
.
- S. Barua and S. Mitragotri, Nano Today, 2014, 9, 223–243 CrossRef CAS PubMed
.
- H. Bahadar, F. Maqbool, K. Niaz and M. Abdollahi, Iran. Biomed. J., 2016, 20, 1–11 Search PubMed
.
- S. L. O'Neal and W. Zheng, Curr. Environ. Health Rep., 2015, 2, 315–328 CrossRef PubMed
.
- C. O. Dimkpa, U. Singh, I. O. Adisa, P. S. Bindraban, W. H. Elmer, J. L. Gardea-Torresdey and J. C. White, Agronomy, 2018, 8, 158 CrossRef CAS
.
- S. Alarifi, D. Ali and S. Alkahtani, BioMed Res. Int., 2017, 2017, 5478790 Search PubMed
.
- J. Fredericks, S. Senapati and M. J. Wannemuehler, F1000Research, 2020, 9, 975 CAS
.
- C. L. Ventola, P T, 2017, 42, 742–755 Search PubMed
.
- D. Bobo, K. Robinson, J. Islam, K. Thurecht and S. Corrie, Pharm. Res., 2016, 33, 2373–2387 CrossRef CAS PubMed
.
|
This journal is © The Royal Society of Chemistry 2021 |
Click here to see how this site uses Cookies. View our privacy policy here.