DOI:
10.1039/D2NA00177B
(Paper)
Nanoscale Adv., 2022,
4, 2922-2928
Superprotonic conduction of intrinsically zwitterionic microporous polymers based on easy-to-make squaraine, croconaine and rhodizaine dyes†
Received
23rd March 2022
, Accepted 7th June 2022
First published on 8th June 2022
Abstract
Porous organic polymers (POPs) have been prepared via a novel metal free polycondensation between a tritopic indole-based monomer and squaric, croconic and rhodizonic acids. Each of the three POPs exhibited high BET surface areas (331–667 m2 g−1) and zwitterionic structures. Impedance measurements revealed that the intrinsic POPs were relatively weak proton conductors, with a positive correlation between the density of oxo-groups and the proton conduction. Doping the materials with LiCl vastly improved the proton conductivity up to a value of 0.54 S cm−1 at 90 °C and 90% relative humidity.
Introduction
Ionic conduction, vital for energy technologies such as fuel cells and batteries, remains a challenging property to optimize in the solid state, as the mobility of protons and other ions depends on many structural and environmental factors.1–4 Of general importance is the number of charge carriers, e.g., in the form of built-in –SO3H or other ionic/acidic groups, and of ionic guests and water molecules introduced into the channels of the porous host. These charge carriers should have freedom to undergo dynamic motion and should exhibit relatively weak interactions with the host framework. Furthermore, they should be optimally spaced and orientated for passing along the H+, Li+ or other mobile ions.5 It is therefore a delicate balancing act to modify the structure and functions for achieving higher ionic conductivity.
In this context, metal–organic frameworks (MOF),6 and porous organic polymers (POP),7–9 offer structural and functional modifiability (largely from the highly variable organic building blocks),10,11 and therefore great opportunity to explore and improve solid state ionic conduction properties.1,3,4 Incidentally, POP frameworks offer the advantage of stability, especially to the aqueous acidic/basic conditions common in fuel cell setups as compared with many hydrolytically labile MOFs. Equipping the framework backbones with sulfonic and other acidic groups is a tried-and-true method, as dense arrays of appended –SO3H groups can help reach record level of proton conductivity.12–14 Many other functions have been also tried to improve ionic conductivity, including phosphonic acid,15 Troger's base (for anion exchange membranes),16 and heterocyclic guests such as 1H-imidazole or 1H-1,2,3-triazole (e.g. for the non-diffusive relay-like proton transport pathway).17
On the other hand, doping ionic guests such as LiCl into the host matrix offers a potentially versatile approach to improving ionic conductivity, because of the diverse array of ionic guests that can be post-synthetically inserted for optimization studies.18–20 For this, a host material exhibiting strong ionic character in the form of a zwitterionic POP will facilitate uptake of the ionic dopants of inorganic salts. Such salts are hydrophilic and well-hydrated species will be reluctant to penetrate the typically less polar organic matrix of POPs. Zwitterionic POPs remain, however, surprisingly rare. For example, the commonly used amine-carbonyl condensations using 1,3,5-triformylphloroglucinol do not generate polymer frameworks with sufficient ionic character, and extra base/acid groups have to be appended to impart distinct zwitterionic properties.21–26 Although zwitterionic POPs have recently started to be explored as ionic conduction materials, studies on inserting ionic salt (e.g., LiCl) dopants for boosting conductivity remains unknown for zwitterionic POP systems.
Reported here is an efficient synthesis of a series of zwitterionic POPs that can be LiCl-doped (by soaking in a saturated THF solution) to achieve proton conductivity as high as 0.54 S cm−1 at 90 °C and 90% relative humidity. The distinct zwitterionic character of the polymer framework stems from the condensation between indole and the oxocarbon anions of squaric (SQ), croconic (CR) and rhodozonic (RH) acids. SQ and CR in particular have shown importance as precursors to near-infrared (NIR) absorbing dyes and their ability to undergo condensation reactions with indole, pyrrole or aniline derivatives can generate a plethora of possible structures.27 This includes examples of POPs featuring squaraine groups spanning applications such as photocatalysis and energy storage.28–30 However, to our knowledge CR and RH have not yet been successfully incorporated into POPs. Our key hypothesis here is that the larger CR and RH, with higher density of the polar oxo-groups, may facilitate the uptake of the ionic guests and enhance proton transport throughout the polymer matrix.
Results and discussion
Polymer synthesis and characterization
For constructing the polymer framework, the tritopic indole-based monomer (1) was prepared via the Suzuki–Miyaura cross-coupling between 1,3,5-benzenetriboronic acid pinacol ester and 2,3,3-trimethyl-5-bromo-3H-indole (Scheme 1). Subsequent polycondensation of 1 with SQ, CR and RH acids yielded three novel POP materials (Scheme 2). SQ, along with the lesser investigated CR and RH acids, are known to undergo condensation reactions with electron-rich aromatic molecules including pyrrole, indole or aniline derivatives, with water being the condensation by-product.27 This approach has been implemented to yield a variety of conjugated poly(squaraines), showing a modulation in the bandgap as well as a bathochromic shift in absorbance values.31 Therefore, we employed the electron-rich indole 1 in three metal-free polycondensation reactions utilizing SQ, CR and RH acids to synthesize three POPs (PSQ, PCR and PRH respectively), each with an alternating acid–indole polymeric network. The reactions were performed with quinoline in a butanol/toluene (1
:
1) solvent mixture under a nitrogen atmosphere at 120 °C for 72 h (Scheme 2). After cooling, the precipitate was filtered, washed and purified via Soxhlet extraction with toluene, chloroform and methanol then subsequently dried in vacuo. The POPs were isolated with excellent recoveries in the range of 85–97%. In short, we were thus able to integrate SQ, CR and RH dyes into a POP structure, without using metal catalysts in the polymerization step.32–41
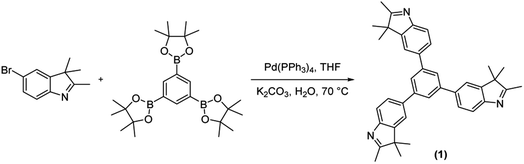 |
| Scheme 1 Synthesis of tritopic indole linker through Suzuki–Miyaura cross-coupling. | |
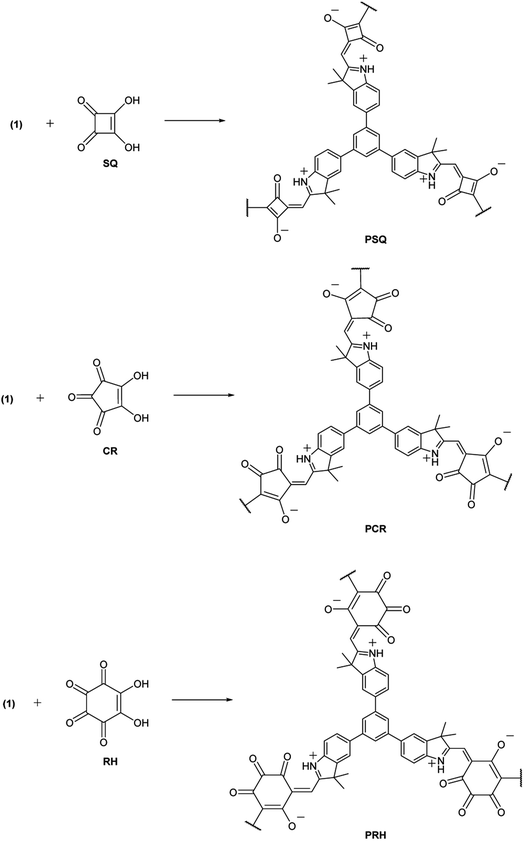 |
| Scheme 2 Synthesis of three POPs through polycondensation of 1 with SQ, CR and RH. Reaction conditions: quinoline (6 mol%), butanol/toluene (v/v 1 : 1), 72 h, Dean–Stark apparatus with 3 Å molecular sieves. | |
The resulting POPs were characterized via spectroscopic methods and other analytical methods were employed to determine their physical characteristics. Analysis of FTIR spectroscopy proved difficult to determine formation of the polymers. However, a sharp band at ∼2960 cm−1 in 1 indicates the presence of methyl groups found on the indole (Fig. S2†). This peak, while still present, reduces significantly in the spectra of the POPs, indicating the formation of a bond between the lone methyl group and the acid. Solid state 13C NMR analysis was also employed as a further spectroscopic method to determine the functional groups within the POPs. Each group in the PSQ and PCR spectra was well defined. A detailed assignment of the spectra peaks can be found in the ESI (Fig. S4–S6†). However, PRH showed some anomalies when compared to the PSQ and PCR spectra. The most notable difference was the near disappearance of the signals around 180 ppm which are indicative of a ketone functional group that can be found in RH. A new peak at 67 ppm also arises though this can be explained as a shift in the C–OH (O−) peak as it falls within the standard range of this functional group.
UV-Vis spectroscopy of the POPs (Fig. 1) show a large bathochromic shift into the visible red and near IR region of the spectrum. While PSQ possesses an absorption maxima (λmax) located at 700 nm, both PCR and PRH have λmax located at approximately 800 nm. Interestingly, although PCR and PRH display similar absorbance maxima, the spectra are somewhat dissimilar. PCR exhibits a very broad absorption beginning at 1050 nm and dipping at 630 nm. However, PRH shows only a small local maximum at ∼800 nm which is relatively weak compared to the absorbance between 400-600 nm.
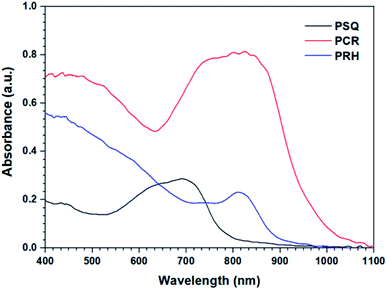 |
| Fig. 1 UV-Vis spectra of PSQ, PCR and PRH with local λmax ranging from 700–850 nm. | |
The thermal stability of the POPs was assessed using thermogravimetric analysis (TGA, Fig. S9–S11†) in the temperature region of 25–950 °C under a flow of CO2 using an empty alumina crucible as the reference sample. The POPs exhibited a slight change in mass when heating to 100 °C: this could be due to residual solvent trapped in the pores. Decomposition of the POPs began in the region of 150–200 °C and continued until 550 °C, at which point less than 10% of the initial mass was remaining. In comparison to other POPs, those reported here exhibited low thermal stability. This could suggest that the connection formed via the polycondensation is susceptible to thermal cleavage.
The surface area of the three POPs was measured via N2 and CO2 gas adsorption experiments at 77 K and 273 K respectively (Table 1, Fig. S12–S23†). The CO2 adsorption measurements revealed that the three materials exhibited high BET surface areas (385–472 m2 g−1) although utilizing N2 as the probe molecule led to much lower surface areas (2.3–106 m2 g−1). This discrepancy most likely stems from the use of N2 at cryogenic temperatures, which has been reported to kinetically restrict access to smaller micropores.42–44 By comparison, the use of CO2 as the probe molecule utilizes higher temperatures and pressures, allowing for faster diffusion and analysis of smaller micropores. For each of the POPs, type 1 CO2 adsorption isotherms were observed, which is typical for microporous polymers. The pore size distribution and pore volumes were modelled using the QSDFT (N2 isotherms) and the Monte-Carlo models (CO2 isotherms), revealing the presence of mainly micropores with additional mesopores.45 The pore volumes that were measured by N2 gas sorption were particularly low (0.005–0.09 cm3 g−1), although the range of values obtained through CO2 gas sorption experiments (0.131–0.220 cm3 g−1) were more in line with values expected for POPs. The origin of this could potentially be due to inability of the N2 probe gas to fully access the microporous structures of the three POPs, thus underestimating the pore volume significantly. The morphology of PSQ, PCR and PRH were all analyzed via scanning electron microscopy (SEM), revealing textured surfaces (Fig. S24–S26†). PCR and PRH both exhibited globular morphologies while PSQ was less well defined in its texture.
Table 1 Summary of the surface area and porous features of the synthesised POPs
Material |
S
BET/m2 g−1 |
Average pore width/nm |
Pore volume/cm3 g−1 |
Measured using N2 gas at 77 K.
Measured using CO2 gas at 273 K.
|
PSQ
|
106a |
2.382 |
0.09 |
385b |
0.822 |
0.220 |
PCR
|
2.3a |
3.096 |
0.005 |
405b |
0.458 |
0.131 |
PRH
|
42a |
2.6 |
0.056 |
472b |
0.785 |
0.172 |
Proton conductivity measurements
The proton conductivities of pristine POPs were first measured by impedance spectrometry from 30 to 90 °C under 90% relative humidity (Fig. 2, S27 and S28†). It turned out that PSQ, PCR and PRH exhibits relatively low conductivities in the range of 0.98 to 41 × 10−7 S cm−1 at 90 °C and 90% relative humidity. All of the POPs displayed an increase in conductivity with increasing temperature, which can be attributed to the enhanced diffusivity and ionic mobility at elevated temperature.46 Moreover, the proton conductivities at temperatures above 50 °C follow the order PSQ < PCR < PRH, indicating that POPs with a higher density of the polar oxo-groups may facilitate binding of hydrated proton by hydrogen-bonding and thus promote proton transport throughout the polymer matrix.47–49
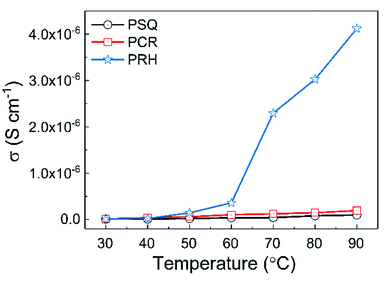 |
| Fig. 2 Proton conductivity of PSQ, PCR, PRH at different temperatures (from 30 °C to 90 °C) and 90% relative humidity. | |
Considering that SQ and CR have shown importance as precursors to NIR dyes, the effect of illumination on proton conductivities of POPs was explored. The proton conductivities of the three POPs were then measured after being illuminated by a red LED for different times at 90 °C and 90% relative humidity (Fig. S29 and S30†). However, our results show that illumination has a negligible and negative association with the proton conductivity. As the proton conductivities of the intrinsic POPs were all relatively low, we sought to increase the proton conductivities by doping the material with charge carriers. This is a method that has been previously explored with other proton conductive materials using ionic salts (e.g., LiCl, Cs2CO3),18,20 or electrolytes (e.g. imidazole, triazole).19,50 With this in mind, we doped PSQ, PCR and PRH with LiCl by soaking the POPs in LiCl/THF for 2 days at room temperature (Fig. S31–S36, see ESI for synthetic details†). This led to six orders of magnitude improvement in the proton conductivity, with values in the range of 0.88 to 5.4 × 10−1 S cm−1 at 90 °C and 90% relative humidity, which are comparable to the conventional Nafion (0.08 S cm−1, Fig. 3, Table S1†).18 According to previous studies, this huge increase in the conductivity may have be achieved by facilitating Li+ ion movements in the POPs. It is worth noting that after introducing LiCl, the proton conductivities kept the order PSQ < PCR < PRH, further confirming POPs rich in oxo-groups can boost proton transport. As a result, the greatest proton conductivity of 5.4 × 10−1 S cm−1 for LiCl@PRH was obtained at 90 °C and 90% relative humidity.51 In addition, the activation energy (Ea) of the proton conduction in three POPs were estimated from Arrhenius plots to be 0.26–0.40 eV (Fig. S30c–S35c†). Such Ea values are typical for the Grotthuss mechanism (Ea < 0.4 eV), which involves proton hopping between adjacent media accompanied by rotational reorientation motion.52–55
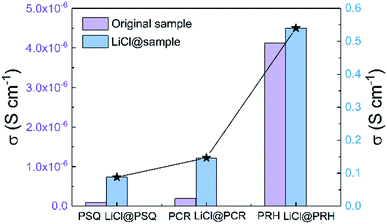 |
| Fig. 3 Proton conductivity of PSQ, PCR, PRH and LiCl@PSQ, LiCl@PCR, LiCl@PRH at 90 °C and 90% relative humidity. | |
Conclusions
In conclusion, a novel method to prepare POPs via the metal free polycondensation between a tritopic indole-based linker and squaric, croconic and rhodizonic acids has been developed. Impedance measurements carried out on the pristine polymer materials revealed that they were relatively weak proton conductors. A positive correlation between the proton conductivities at high temperature and the density of oxo-groups in the POPs was observed, suggesting that these groups facilitate proton binding through hydrogen bonding. Doping these materials with LiCl led to vastly improved proton conduction up to 5.4 × 10−1 S cm−1 at 90 °C and 90% relative humidity.
Funding sources
This work was partially funded by the National Natural Science Foundation of China (21871061) and Science and Technology Planning Project of Guangdong Province (2021A0505030066). DT and FV acknowledge the EPSRC funded (EP/L016419/1) CRITICAT Centre for Doctoral Training for Dominic Taylor's PhD. ZX acknowledges a GRF grant from the Research Grants Council of HKSAR (CityU 11303519).
Author contributions
The manuscript was written through contributions of all authors. All authors have given approval to the final version of the manuscript.
Conflicts of interest
The authors declare no competing financial interest.
Acknowledgements
We thank Hua-Qun Zhou and Dr Yonghe He for their help with this work.
References
- X. Zhao, Y. Chen, Z. Wang and Z. Zhang, Design and Application of Covalent Organic Frameworks for Ionic Conduction, Polym. Chem., 2021, 12(34), 4874–4894 RSC
.
- A. Gupta, S. Goswami, S. M. Elahi and S. Konar, Role of Framework-Carrier Interactions in Proton-Conducting Crystalline Porous Materials, Cryst. Growth Des., 2021, 21(3), 1378–1388 CrossRef CAS
.
- D. W. Lim and H. Kitagawa, Proton Transport in Metal-Organic Frameworks, Chem. Rev., 2020, 120(16), 8416–8467 CrossRef CAS PubMed
.
- D. W. Kang, M. Kang and C. S. Hong, Post-Synthetic Modification of Porous Materials: Superprotonic Conductivities and Membrane Applications in Fuel Cells, J. Mater. Chem. A, 2020, 8(16), 7474–7494 RSC
.
- S. Kim, B. Joarder, J. A. Hurd, J. Zhang, K. W. Dawson, B. S. Gelfand, N. E. Wong and G. K. H. Shimizu, Achieving Superprotonic Conduction in Metal-Organic Frameworks through Iterative Design Advances, J. Am. Chem. Soc., 2018, 140(3), 1077–1082 CrossRef CAS PubMed
.
- S. Yuan, L. Feng, K. Wang, J. Pang, M. Bosch, C. Lollar, Y. Sun, J. Qin, X. Yang and P. Zhang,
et al., Stable Metal–Organic Frameworks: Design, Synthesis, and Applications, Adv. Mater., 2018, 30(37), 1–35 Search PubMed
.
- N. B. McKeown and P. M. Budd, Polymers of Intrinsic Microporosity (PIMs): Organic Materials for Membrane Separations, Heterogeneous Catalysis and Hydrogen Storage, Chem. Soc. Rev., 2006, 35(8), 675–683 RSC
.
- T. Ben and S. Qiu, Porous Aromatic Frameworks: Synthesis, Structure and Functions, CrystEngComm, 2013, 15(1), 17–26 RSC
.
- Y. Yuan and G. Zhu, Porous Aromatic Frameworks as a Platform for Multifunctional Applications, ACS Cent. Sci., 2019, 5(3), 409–418 CrossRef CAS PubMed
.
- D. Taylor, S. J. Dalgarno, Z. Xu and F. Vilela, Conjugated Porous Polymers: Incredibly Versatile Materials with Far-Reaching Applications, Chem. Soc. Rev., 2020, 49(12), 3981–4042 RSC
.
-
D. Taylor, S. J. Dalgarno and F. Vilela, Structure–Function Relationship in Conjugated Porous Polymers, in Concepts and Design of Materials Nanoarchitectonics, 2022, pp. 226–246 Search PubMed
.
- S. Mukhopadhyay, J. Debgupta, C. Singh, R. Sarkar, O. Basu and S. K. Das, Designing UiO-66-Based Superprotonic Conductor with the Highest Metal-Organic Framework Based Proton Conductivity, ACS Appl. Mater. Interfaces, 2019, 11(14), 13423–13432 CrossRef CAS PubMed
.
- F. Yang, G. Xu, Y. Dou, B. Wang, H. Zhang, H. Wu, W. Zhou, J. R. Li and B. Chen, A Flexible Metal-Organic Framework with a High Density of Sulfonic Acid Sites for Proton Conduction, Nat. Energy, 2017, 2(11), 877–883 CrossRef CAS
.
- Y. He, J. Dong, Z. Liu, M. Q. Li, J. Hu, Y. Zhou, Z. Xu and J. He, Dense Dithiolene Units on Metal-Organic Frameworks for Mercury Removal and Superprotonic Conduction, ACS Appl. Mater. Interfaces, 2022, 14(1), 1070–1076 CrossRef CAS PubMed
.
- J. M. Taylor, K. W. Dawson and G. K. H. Shimizu, A Water-Stable Metal-Organic Framework with Highly Acidic Pores for Proton-Conducting Applications, J. Am. Chem. Soc., 2013, 135(4), 1193–1196 CrossRef CAS PubMed
.
- X. Du, Y. Yuan, T. Dong, X. Chi and Z. Wang, Polymer Electrolyte Membranes from Microporous Troger's Base Polymers for Fuel Cells, ACS Appl. Energy Mater., 2021, 4(11), 13327–13334 CrossRef CAS
.
- D. Basak, C. Versek, J. A. Harvey, S. Christensen, J. Hillen, S. M. Auerbach, M. T. Tuominen and D. Venkataraman, Enhanced Anhydrous Proton Conduction in Binary Mixtures of 1H-Imidazole-1H-1,2,3-Triazole Based Compounds, J. Mater. Chem., 2012, 22(38), 20410–20417 RSC
.
- S. Horike, Y. Kamitsubo, M. Inukai, T. Fukushima, D. Umeyama, T. Itakura and S. Kitagawa, Postsynthesis Modification of a Porous Coordination Polymer by LiCl to Enhance H+ Transport, J. Am. Chem. Soc., 2013, 135(12), 4612–4615 CrossRef CAS PubMed
.
- S. Nandi, V. M. Dhavale, S. Shalini, U. Werner-Zwanziger, H. Singh, S. Kurungot and R. Vaidhyanathan, Lithium-Assisted Proton Conduction at 150 °C in a Microporous Triazine-Phenol Polymer, Adv. Mater. Interfaces, 2015, 2(16), 1–7 Search PubMed
.
- S. Shalini, S. Aggarwal, S. K. Singh, M. Dutt, T. G. Ajithkumar and R. Vaidhyanathan, 10000-Fold Enhancement in Proton Conduction by Doping of Cesium Ions in a Proton-Conducting Zwitterionic Metal–Organic Framework, Eur. J. Inorg. Chem., 2016, 2016(27), 4382–4386 CrossRef CAS
.
- Y. Yang, W. Zhao, H. Niu and Y. Cai, Mechanochemical Construction 2D/2D Covalent Organic Nanosheets Heterojunctions Based on Substoichiometric Covalent Organic Frameworks, ACS Appl. Mater. Interfaces, 2021, 13(35), 42035–42043 CrossRef CAS PubMed
.
- S. Chandra, T. Kundu, K. Dey, M. Addicoat, T. Heine and R. Banerjee, Interplaying Intrinsic and Extrinsic Proton Conductivities in Covalent Organic Frameworks, Chem. Mater., 2016, 28(5), 1489–1494 CrossRef CAS
.
- S. Kandambeth, A. Mallick, B. Lukose, M. V. Mane, T. Heine and R. Banerjee, Construction of Crystalline 2D Covalent Organic Frameworks with Remarkable Chemical (Acid/Base) Stability via a Combined Reversible and Irreversible Route, J. Am. Chem. Soc., 2012, 134(48), 19524–19527 CrossRef CAS PubMed
.
- Z. J. Mu, X. Ding, Z. Y. Chen and B. H. Han, Zwitterionic Covalent Organic Frameworks as Catalysts for Hierarchical Reduction of CO2 with Amine and Hydrosilane, ACS Appl. Mater. Interfaces, 2018, 10(48), 41350–41358 CrossRef CAS PubMed
.
- Y. Li, H. Wu, Y. Yin, L. Cao, X. He, B. Shi, J. Li, M. Xu and Z. Jiang, Fabrication of Nafion/Zwitterion-Functionalized Covalent Organic Framework Composite Membranes with Improved Proton Conductivity, J. Membr. Sci., 2018, 568, 1–9 CrossRef CAS
.
- Y. Fu, Y. Wu, S. Chen, W. Zhang, Y. Zhang, T. Yan, B. Yang and H. Ma, Zwitterionic Covalent Organic Frameworks: Attractive Porous Host for Gas Separation and Anhydrous Proton Conduction, ACS Nano, 2021, 15(12), 19743–19755 CrossRef CAS PubMed
.
- L. Beverina and P. Salice, Squaraine Compounds: Tailored Design and Synthesis towards a Variety of Material Science Applications, Eur. J. Org. Chem., 2010, 7, 1207–1225 CrossRef
.
- A. Nagai, X. Chen, X. Feng, X. Ding, Z. Guo and D. Jiang, A Squaraine-Linked Mesoporous Covalent Organic Framework, Angew. Chem., Int. Ed., 2013, 52(13), 3770–3774 CrossRef CAS PubMed
.
- R. Xue, H. Gou, L. Zhang, Y. Liu, H. Rao and G. Zhao, A New Squaraine-Triazine Based Covalent Organic Polymer as an Electrode Material with Long Life and High Performance for Supercapacitors, New J. Chem., 2021, 45(2), 679–684 RSC
.
- X. Zhang, J. Zhao, Y. Zhao, Z. Bakenov and Y. Zhang, Engineering Zwitterionic Barrier by Squaraine-Based Porous Organic Framework Fiber for Superior Lithium-Sulfur Batteries, Electrochim. Acta, 2021, 397, 139276 CrossRef CAS
.
- A. Ajayaghosh, Donor-Acceptor Type Low Band Gap Polymers: Polysquaraines and Related Systems, Chem. Soc. Rev., 2003, 32(4), 181–191 RSC
.
- X. Ding and B. H. Han, Copper Phthalocyanine-Based CMPs with Various Internal Structures and Functionalities, Chem. Commun., 2015, 51(64), 12783–12786 RSC
.
- J. Lee, O. Buyukcakir, T. W. Kwon and A. Coskun, Energy Band-Gap Engineering of Conjugated Microporous Polymers via Acidity-Dependent In situ Cyclization, J. Am. Chem. Soc., 2018, 140(35), 10937–10940 CrossRef CAS PubMed
.
- M. Liu, C. Yao, C. Liu and Y. Xu, Ag+ Doped into Azo-Linked Conjugated Microporous Polymer for Volatile Iodine Capture and Detection of Heavy Metal Ions, Sci. Rep., 2018, 8(1), 14072 CrossRef PubMed
.
- Y. Kou, Y. Xu, Z. Guo and D. Jiang, Supercapacitive Energy Storage and Electric Power Supply Using an Aza-Fused π-Conjugated Microporous Framework, Angew. Chem., Int. Ed., 2011, 50(37), 8753–8757 CrossRef CAS PubMed
.
- Y. Wei, W. Chen, X. Zhao, S. Ding, S. Han and L. Chen, Solid-State Emissive Cyanostilbene Based Conjugated Microporous Polymers: Via Cost-Effective Knoevenagel Polycondensation, Polym. Chem., 2016, 7(24), 3983–3988 RSC
.
- Z. H. Guo, C. Wang, Q. Zhang, S. Che, H. C. Zhou and L. Fang, Cost-Effective Synthesis and Solution Processing of Porous Polymer Networks through Methanesulfonic Acid-Mediated Aldol Triple Condensation, Mater. Chem. Front., 2018, 2(2), 396–401 RSC
.
- P. Ju, S. Wu, Q. Su, X. Li, Z. Liu, G. Li and Q. Wu, Salen-Porphyrin-Based Conjugated Microporous Polymer Supported Pd Nanoparticles: Highly Efficient Heterogeneous Catalysts for Aqueous C–C Coupling Reactions, J. Mater. Chem. A, 2019, 7(6), 2660–2666 RSC
.
- Z. Li, X. Li and Y. W. Yang, Conjugated Macrocycle Polymer Nanoparticles with Alternating Pillarenes and Porphyrins as Struts and Cyclic Nodes, Small, 2019, 15(12), 1–10 Search PubMed
.
- B. P. Biswal, D. Becker, N. Chandrasekhar, J. S. Seenath, S. Paasch, S. Machill, F. Hennersdorf, E. Brunner, J. J. Weigand and R. Berger, et al. Exploration of Thiazolo[5,4-d]Thiazole Linkages in Conjugated Porous Organic Polymers for Chemoselective Molecular Sieving, Chem.–Eur. J., 2018, 24(42), 10868–10875 CrossRef CAS PubMed
.
- Q. Huang, L. Guo, N. Wang, X. Zhu, S. Jin and B. Tan, Layered Thiazolo[5,4- d] Thiazole-Linked Conjugated Microporous Polymers with Heteroatom Adoption for Efficient Photocatalysis Application, ACS Appl. Mater. Interfaces, 2019, 11(17), 15861–15868 CrossRef CAS PubMed
.
- J. Weber, J. Schmidt, A. Thomas and W. Böhlmann, Micropore Analysis of Polymer Networks by Gas Sorption and 129Xe NMR Spectroscopy: Toward a Better Understanding of Intrinsic Microporosity, Langmuir, 2010, 26(19), 15650–15656 CrossRef CAS PubMed
.
- D. Lozano-Castelló, D. Cazorla-Amorós and A. Linares-Solano, Usefulness of CO2 Adsorption at 273 K for the Characterization of Porous Carbons, Carbon, 2004, 42(7), 1233–1242 CrossRef
.
- K. A. Cychosz and M. Thommes, Progress in the Physisorption Characterization of Nanoporous Gas Storage Materials, Engineering, 2018, 4(4), 559–566 CrossRef CAS
.
- J. Rouquerol, D. Avnir, C. W. Fairbridge, D. H. Everett, J. M. Haynes, N. Pernicone, J. D. F. Ramsay, K. S. W. Sing and K. K. Unger, Recommendations for the Characterization of Porous Solids, Pure Appl. Chem., 1994, 66(8), 1739–1758 CrossRef CAS
.
- L. Liu, W. Chen and Y. Li, An Overview of the Proton Conductivity of Nafion Membranes through a Statistical Analysis, J. Membr. Sci., 2016, 504, 1–9 CrossRef CAS
.
- S. K. Konavarapu, A. Goswami, A. G. Kumar, S. Banerjee and K. Biradha, MOFs Containing a Linear Bis-Pyridyl-Tris-Amide and Angular Carboxylates: Exploration of Proton Conductivity, Water Vapor and Dye Sorptions, Inorg. Chem. Front., 2019, 6(1), 184–191 RSC
.
- T. Kawabata and Y. Matsuo, Role of Acetyl Group on Proton Conductivity in Chitin System, J. Mater., 2019, 5(2), 258–263 Search PubMed
.
- S. S. Xue, X. X. Li, Y. M. Lee, M. S. Seo, Y. Kim, S. Yanagisawa, M. Kubo, Y. K. Jeon, W. S. Kim and R. Sarangi,
et al., Enhanced Redox Reactivity of a Nonheme Iron(V)-Oxo Complex Binding Proton, J. Am. Chem. Soc., 2020, 142(36), 15305–15319 CrossRef CAS PubMed
.
- Y. Ye, L. Zhang, Q. Peng, G. E. Wang, Y. Shen, Z. Li, L. Wang, X. Ma, Q. H. Chen and Z. Zhang,
et al., High Anhydrous Proton Conductivity of Imidazole-Loaded Mesoporous Polyimides over a Wide Range from Subzero to Moderate Temperature, J. Am. Chem. Soc., 2015, 137(2), 913–918 CrossRef CAS PubMed
.
- S. Li, Y. Zhao, S. Knoll, R. Liu, G. Li, Q. Peng, P. Qiu, D. He, C. Streb and X. Chen, High Proton-Conductivity in Covalently Linked Polyoxometalate Organoboronic Acid-Polymers, Angew. Chem., Int. Ed., 2021, 60(31), 16953–16957 CrossRef CAS PubMed
.
- S. Pili, S. P. Argent, C. G. Morris, P. Rought, V. García-Sakai, I. P. Silverwood, T. L. Easun, M. Li, M. R. Warren and C. A. Murray,
et al., Proton Conduction in a Phosphonate-Based Metal-Organic Framework Mediated by Intrinsic “Free Diffusion inside a Sphere”, J. Am. Chem. Soc., 2016, 138(20), 6352–6355 CrossRef CAS PubMed
.
- Y. Wang, J. Yin, D. Liu, C. Gao, Z. Kang, R. Wang, D. Sun and J. Jiang, Guest-tuned proton conductivity of a porphyrinylphosphonate-based hydrogenbonded organic framework, J. Mater. Chem. A, 2021, 9(5), 2683–2688 RSC
.
- X. Jiang, K. Zhang, Y. Huang, B. Xu, X. Xu, J. Zhang, Z. Liu, Y. Wang, Y. Pan and S. Bian,
et al., Conjugated Microporous Polymer with C
C and C–F Bonds: Achieving Remarkable Stability and Super Anhydrous Proton Conductivity, ACS Appl. Mater. Interfaces, 2021, 13(13), 15536–15541 CrossRef CAS PubMed
.
- L. Xin, D. Zhang, K. Qu, Y. Lu, Y. Wang, K. Huang, Z. Wang, W. Jin and Z. Xu,
et al., Zr-MOF-Enabled Controllable Ion Sieving and Proton Conductivity in Flow Battery Membrane, Adv. Funct. Mater., 2021, 31(42), 2104629 CrossRef CAS
.
Footnotes |
† Electronic supplementary information (ESI) available: Synthesis and characterisation of compound 1 and polymers PSQ, PCR and PRH. See https://doi.org/10.1039/d2na00177b |
‡ These authors contributed equally. |
|
This journal is © The Royal Society of Chemistry 2022 |
Click here to see how this site uses Cookies. View our privacy policy here.