DOI:
10.1039/D3DT00849E
(Perspective)
Dalton Trans., 2023,
52, 7772-7786
Oligozwitterions in coordination polymers and frameworks – a structural view
Received
20th March 2023
, Accepted 26th May 2023
First published on 30th May 2023
Abstract
Zwitterions may take many forms and have found many applications, some of which are based on their capacity to act as ligands for a wide variety of metal ions. This brief review describes recent developments in this coordination chemistry involving oligozwitterion species, as reflected in solid state X-ray structural studies of the coordination polymers and frameworks formed and with a particular focus on uranyl ion systems.
1. Introduction
Zwitterions are neutral molecules in which the electronic structure is such that they can be considered to contain equal numbers of sites with, at least formally, opposite charges of unity. One of the simplest examples of such a species is that of trimethylammonioacetate, [(CH3)3NCH2CO2], originally termed “betaine” due to its ready isolation from sugar beet,1 with one positive centre, N(+), and one negative, CO2(−). A large family of “betaines”, some, like trimethylammonioacetate, of natural origin2 and others purely synthetic, is now recognised.1 The naturally occurring betaines have important biological roles in the regulation of osmotic pressure across cell membranes and as methylation agents but their capacity to act as metal ion binding ligands has rendered them and many analogues of much broader interest. Although the pKa of [(CH3)3NCH2CO2H]+, (1.81), indicates that the carboxylate centre of trimethylammonioacetate is an approximately 1000-fold weaker base than acetate, early structural studies3–10 involving a wide range of transition and main-group metal ions showed that trimethylammonioacetate and various similar mono-zwitterions (Fig. 1)11,12 readily formed isolable complexes and could be considered comparable in their donor capacity to anionic carboxylates, an issue discussed in more detail ahead. There is of course no necessity to limit the Lewis base site of a zwitterion to a carboxylate group, nor to limit the positive site to an ammonium centre and the applications of zwitterion complexes in catalysis have been considerably developed through synthetic variations in these regards.13 Further, multiple zwitterion units can be incorporated in a single ligand, offering particular advantages in the synthesis of coordination polymers and frameworks.14–17 It is this last, recent development of zwitterion coordination chemistry that is the principal focus of this Perspective.
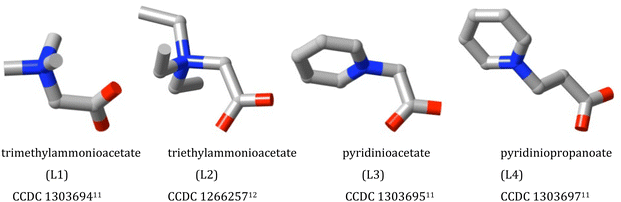 |
| Fig. 1 Trimethylammonioacetate (betaine) and three related species used in early studies of zwitterion coordination chemistry with transition and main group metals. The ligands are shown in the conformations observed in crystal structures with the indicated CCDC deposition numbers. In most cases, H-atom coordinates are not available, so for consistency all are shown as stick representations without hydrogen atoms (C = grey, N = blue, O = red). | |
2. Simple zwitterions where a single carboxylate group is the metal–ion binding site
An obvious omission in the introductory remarks above is that of the zwitterion chemistry of amino acids, formally a matter for inclusion in this section. This, however, is a vast18–21 and exceptionally important topic demanding separate treatment and, as well, is excluded here by limiting considerations to where the N-atom of the positive site of the zwitterion cannot become involved, through deprotonation, in metal ion coordination.
Although very little information is available concerning comparison of stability constants for complexes of betaine zwitterions and non-zwitterion analogues,22 the broad early structural studies3–10 of transition and main group metal ion complexes of various carboxylato-betaines established that M–O bond lengths for analogous species generally overlapped, indicating that in the absence of solvent effects (as in solid crystals), no real distinction of the donor strengths could be drawn. These studies did reveal some subtle differences (Fig. 2),23–25 for example, in that the OCO bond angles in betaines are usually 1–2° larger than in anionic carboxylates, possibly favouring their action as bridging ligands, though the versatility of both types is expressed in the same range of unidentate (κ1O), chelating (κ2O,O′), bridging (κ1O;κ1O′) and chelating–bridging (κ2O,O′;κ1O′) bonding modes being shown. It is possible5,6 to qualify these designations of bonding modes with the terms syn and anti based upon the assumption that the O-donor atom is trigonal and that there are two disposition of its lone pairs, leading to the description of the κ1O;κ1O′ mode, for example, as syn;anti in most cases, but it is not always clear in general that this assumption is justified.26
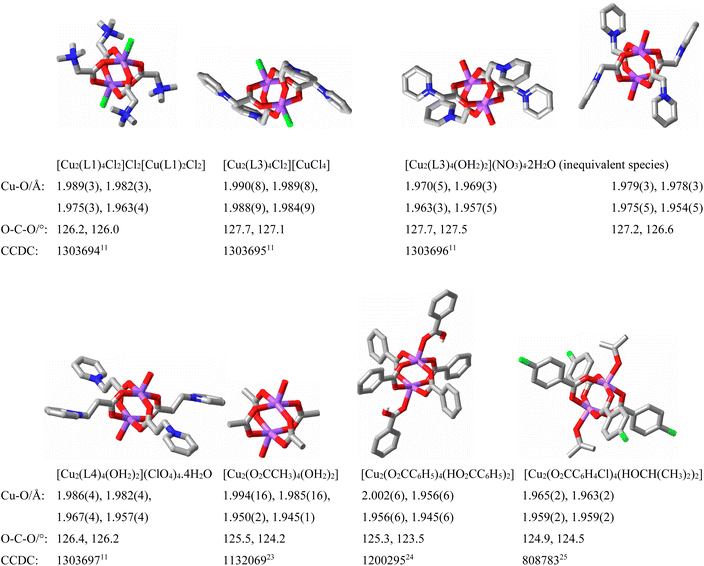 |
| Fig. 2 Structural parameters for some binuclear Cu(II) carboxylate species (involving μ2-syn,syn-κ1O;κ1O′ bridging) derived from both anionic and zwitterionic donors, illustrating their generally very close character. Ligand numbering is as in Fig. 1. (C = grey, blue = N, red = O, violet = Cu.) | |
A notable feature of simple betaine (and monocarboxylate) metal ion complexation is the fact that coordination polymers can be obtained (e.g. ref. 5, 6a, 9 and 10) even when only one carboxylate site is available, reflecting the rather special nature of the carboxylate group. Such materials involving paramagnetic metal ions have proven to be of particular interest for their magnetic behaviour and physical properties.27 More recent interest in betaine chemistry, however, has been concerned with their application in “task specific” ionic liquids.28–32 Protonation of trimethylammonioacetate, for example, produces a cation which can be paired with an appropriate anion, e.g. bis(trifluoromethylsulfonyl)amide to give an ionic liquid highly efficient in the dissolution of metal oxides both because of its acidity and the complexing ability of the zwitterion released by the consumption of acid by the oxide. This work has led in particular to the structural characterisation of uranyl ion complexes of simple zwitterions,28b,29–31 these structures serving to illustrate all four coordination modes of the carboxylate units referred to above (Fig. 3). As found for transition and main group metal ions, examination of U–O bond lengths provides no clear evidence that zwitterion carboxylate-O should be considered to differ in donor capacity towards U(VI) from an anionic carboxylate. Such comparisons are complicated by the fact that the nature and symmetry of the environment of any U–O unit must differ in the two types of complex but in the complexes [UO2(imbet)3]((CF3SO2)N)2,28b (imbet = 3-methylimidazoliumacetate = L5) (MeImH)[UO2(O2CCH3)3],33 (MeImH = protonated 2-methylimidazole) and Na[UO2(O2CCH3)3],34 for example, the mean equatorial U–O bond lengths of 2.471(3), 2.47(1) and 2.469(9) Å, respectively, are identical within experimental uncertainty.
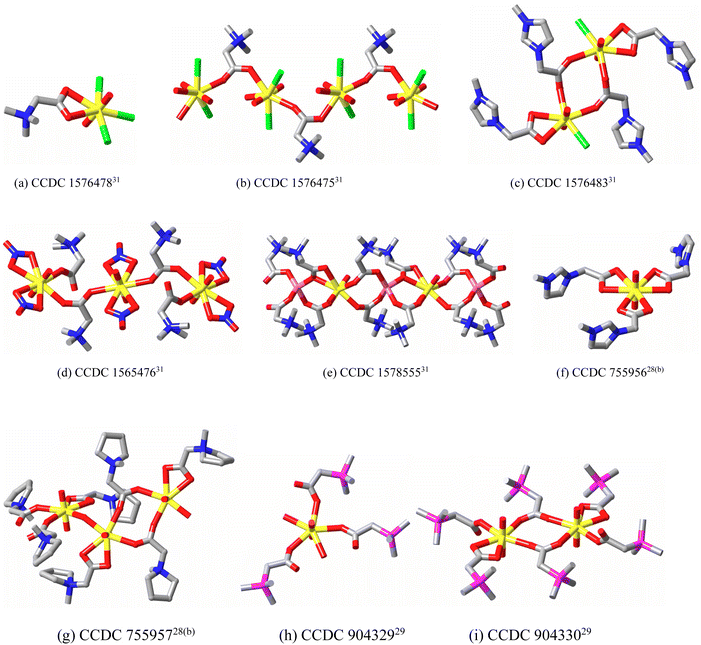 |
| Fig. 3 Coordination modes observed for simple betaines in uranyl ion complexes: (a) κ2O,O′ chelation in mononuclear K[UO2(L1)Cl3]; (b) part of the polymer chain present in [UO2Cl2(L1)(OH2)], showing κ1O;κ1O′ bridging (syn;anti); (c) the dimeric species present in [(UO2)2Cl2(L5)4][N(SO2CF3)2]2 (L5 = imbet = 3-methylimidazoliumacetate) where both κ2O,O′ chelation and κ1O;κ1O′ bridging occur; (d) the trinuclear unit present in [(UO2)3(L1)2(NO3)6(OH2)2]·H2O where κ1O coordination accompanies κ1O;κ1O′ bridging; (e) part of the polymer chain of heterometallic [UO2Li(L1)4][N(SO2CF3)2]3 where bridging of the two metal ions involves both κ1O;κ1O′ and κ2O,O′;κ1O′ coordination; (f) tris κ2O,O′ chelation in [UO2(L5)3][N(SO2CF3)2]2, showing U(VI) is not limited to pentagonal-bipyramidal coordination in betaine complexes; (g) part of the polymer chain in [(UO2)2(L6)4(OH)][N(SO2CF3)2]3, (L6 = pyrrbet = N-methylpyrrolidiniumacetate), where both κ2O,O′ and κ1O;κ1O′ binding occur together; (h) exclusive κ1O coordination in [UO2(L7)3(OH2)2][N(SO2CF3)2]2 (L7 = phosbet = trimethylphosphonioacetate); (i) the binuclear unit found in [(UO2)2(L7)6][N(SO2CF3)2]4, where three different coordination modes, κ1O, κ2O,O′ and κ1O;κ1O′ occur together. (C = grey, blue = N, red = O, green = Cl, violet = P, yellow = U.) | |
In the complexes shown in Fig. 3, further possible variations in the betaine structure are exemplified and others are certainly known,13,35 including those where aromatic thiolate replaces the carboxlate group.36 In nearly all such cases the positive and negative charge centres are insulated from one another by saturated organic links but are sufficiently close for these zwitterions to be considered, for example, as models for ion pairs.37 A much closer approach of the charge centres is possible in non-betaine species such as pyridine N-oxide, where a major canonical form is considered to be that where N(+) and O(−) are directly linked by a single bond.38 The high electron density on the O centre is considered as a crucial factor giving rise to the extended studies that have been made of the coordination chemistry of pyridine N-oxide.39 To consider pyridine N-oxide as zwitterion is to foreshadow similar consideration of a substantial number of other ligands of much greater importance due to their widespread utility in contemporary chemistry, taken thus as a major advance in zwitterion chemistry.21
3. Zwitterions with negatively charged donor atoms located in close proximity to the positive centres – “vicinal zwitterion ylides”
The valence-bond canonical forms for a broad range of neutral ligands such as borylenes,40 carbenes,41 nitrenes,42 sydnones43 and phosphorus ylides44 include species involving charge separation.21 Even a ligand as simple as carbon monoxide can be considered to involve the canonical form (−)C
O(+), a form which, with its opposite charges being located on adjacent atoms, can be termed a “vicinal zwitterion”, a description applicable also to more complicated ligands.21 Whether or not the charge-separated forms should be considered to be dominant and the molecules therefore considered as true zwitterions is a matter to be resolved through structural, spectroscopic and theoretical studies45 but with the probable exception of borylenes,21 this appears to be true in many cases of the four other categories just mentioned. Carbenes and in particular N-heterocyclic carbenes (NHCs) have an extensive and well-developed coordination chemistry involving numerous applications in catalysis,41,45,46 whereas sydnones, although much longer known43a,b than NHCs, have only relatively recently come under deliberate study as metal ion complexing agents43c and so serve as a subject of greater novelty for the present discussion. Interestingly, metal-catalysed reactions of some sydnones have been used to provide new NHCs.47
While structural studies of singlet carbenes as free ligands are much less common than those of carbene complexes (Fig. 4),41,45,48–51 the opposite is true for sydnones. This is due to the ready synthesis and stability of functionalised sydnones52 as well as to their versatility as synthetic intermediates, their biological activity and their numerous applications.43,47 The family of sydnones consists of derivatives of 5-oxo-1,2,3-oxadiazole (Fig. 5(a and b)), the parent usually being considered to be the 3-phenyl derivative (obtained by cyclisation of N-nitroso-N-phenyl glycine), as the unfunctionalised molecule is unknown. The term “mesoionic” was proposed as a descriptor for sydnones,43b since the valence bond canonical representations for the 5-oxo-1,2,3-oxadiazole ring involve charge separations of various forms (Fig. 5(c)) where both directly bonded N(+)–N(−), N(+)–C(−) and more remote N(+)–O(−) pairs appear. The crystal structure of a simple sydnone, 3-tolylsydnone,53 shows (Fig. 5(d)) bond lengths of this ring which all lie between single and double bond values, indicating that the charges must be significantly delocalised, although calculations of charge distributions in 3-phenylsydnone54 have shown that most of the negative charge resides on the exocyclic O-atom, with some on N2 and very little on C4. Consistent with this, examination of the weak interactions within the crystal of 3-tolylsydnone using CrystalExplorer55 shows that the only interactions exceeding dispersion are O⋯HC type, the shorter involving the exocyclic-O and both phenyl and sydnone H atoms (Fig. 5(e)).
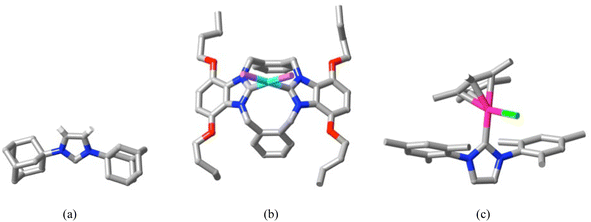 |
| Fig. 4 (a) The first structurally characterised free N-heterocyclic carbene (CCDC 1283830;49 only H-atoms bound to the five-membered ring are shown); (b) the Pd(II) dibromide complex of a macrocyclic N-heterocyclic dicarbene (CCDC 72662550); (c) an example of a Grubbs catalyst with [Ru(II)(Cp*)Cl] bound to a monocarbene (CCDC 117046751). (C = grey, N = blue, O = red, violet = Br, light blue = Pd, pink = Ru.) | |
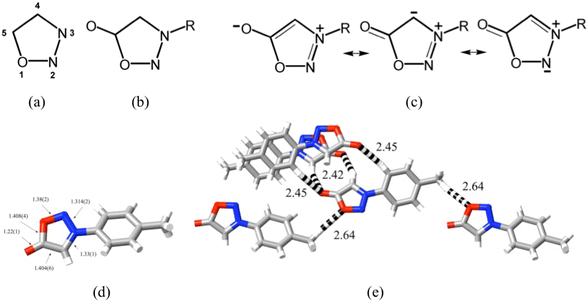 |
| Fig. 5 (a) Atom numbering in the oxadiazole ring; (b) substitution pattern of a sydnone core; (c) zwitterionic canonical forms of a sydnone; (d) bond lengths/Å within a simple sydnone species, 3-tolylsydnone (CCDC 112521553); (e) local CH⋯O contacts/Å (dashed lines) of a single 3-tolylsydnone molecule (central in the figure) within its crystal. (All molecules are equivalent, so in fact these interactions extend throughout the structure.) (H = white, C = grey, N = blue, O = red.) | |
While it appears that in principle a sydnone might act as a C-, N- or O-donor ligand, early attempts to form transition metal complexes through solid state reactions were of limited success and any complexes formed were found to dissociate completely in polar solvents,56 indicating that neutral sydnones, unlike NHCs, must be rather weak ligands (though dissociation in water may have been assisted by the low aqueous solubility of simple sydnones43a). Later work57 employing more sophisticated ball-milling techniques for solid state reactions did provide structurally characterised stable complexes involving sydnone exocyclic-O coordination in chelate rings resulting from N-coordination of a pyridyl substituent. Similar coordination was observed in a sydnone with a larger thiasemicarbazone substituent providing the chelation assistance.58 A sydnone doubly substituted with diphenylphosphino groups, however, provided only a Pd(II) complex involving phosphorus coordination,59 while an alkynylaryl sydnone gave a Pt(II) complex where again the oxadiazole unit was not bound to the metal, although it did have an influence on the optical properties of the complex.60 This small number of X-ray structures known for complexes involving neutral sydnone groups is shown in Fig. 6. In all cases where the sydnone unit is coordinated, it binds in a κ1O manner and the M–O bond lengths (Fig. 6), are all significantly longer (by ∼0.06 Å on average) than those for comparable betaine complexes.5–7 Considering also that the sydnone species involve chelation which may enhance the M–O interactions, it appears that indeed sydnone-O is a relatively weak donor.
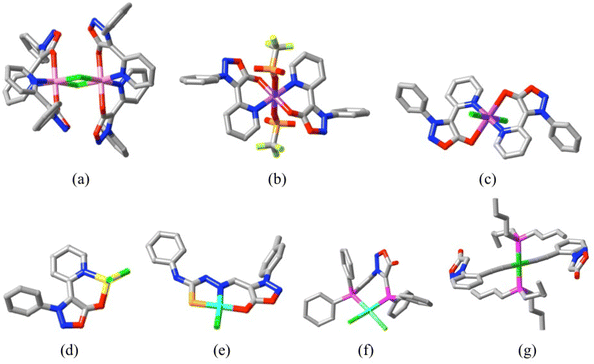 |
| Fig. 6 Structurally characterised complexes formed from sydnone derivatives: (a) [Co2(L8)4Cl2][CoCl4] (L8 = ppysyd = 3-phenyl-4-(2-pyridyl)sydnone),57 CCDC 1913663, Co–O 2.080(2) Å; (b) [Cu(L8)2(O3SCF3)2],57 CCDC 1913662, Cu–O 1.967(4) Å; (c) [Cu(L8)2Cl2],57 CCDC 1913667, Cu–O 2.583(6) Å (here, it is assumed that the sydnone-O atoms occupy the axial sites of a Jahn–Teller distorted Cu(II) coordination sphere, whereas in complex (b) these sites are occupied by triflate-O); (d) [Zn(L8)Cl2],57 CCDC 1913665, Zn–O 2.060(2) Å; (e) [Pd(L9)Cl],58 (L9 = ttssyd = 3-tolyl-4-(thiosemicarbazidylmethyl)sydnone) CCDC 713516, Pd–O 2.096(3) Å; (f) [Pd(L10)Cl2]59 (L10 = ppsyd = 3-(2-diphenylphosphinophenyl)-4-diphenylphosphinosydnone), CCDC 142604; (g) [Pt((L11)2(P(nBu)3)2]60 (L11 = pesyd = 3-(2-ethynylphenyl)-sydnone, CCDC 264664. (C = grey, N = blue, O = red, F = yellow-green, P = deep pink, S = orange, Cl = green, Co = light pink, Cu = violet, Zn = yellow, Pd = sky blue, Pt = dark green.) | |
C4-bound sydnone complexes of Hg(II)61 have been well characterised by spectroscopic and analytical methods but although described as nicely crystalline have not been studied by X-ray crystallography. They are of course complexes of the sydnone anion and not the neutral species and have been used to prepare Ni, Pd and Pt complexes of the same anion.62 Metallation at C4 has also been achieved for Li, Cu and Zn,43c though only in reaction intermediates used for the synthesis of sydnone derivatives. It has been suggested the mechanism of the Cu-catalysed reaction between sydnones and acetylides may involve sydnone N2 coordination to copper(I), this reaction being a useful variant of click chemistry.63 Overall, while it is apparent that many reactions of sydnones involve metal ion catalysis and pass through complex formation, details of the coordination chemistry involved remain open to structural study.
4. Oligo-zwitterions and the formation of coordination polymers and frameworks
Polymeric zwitterion species, mainly based on the full variety of betaine subunits (ammonio, pyridinio and phosphonio acetates, sulfates and phosphates), are long known64 and have a large number of significant applications,21,64–66 including metal ion binding. X-ray structure determinations of metal ion complexes, however, are limited to those based on small oligomers in coordination polymers or frameworks. Over the past three decades, research on inorganic coordination polymers and frameworks has become intense and an extraordinary variety of systems has been characterised. For some recent reviews illustrating their diversity, see ref. 67–71, where more comprehensive listing of background material is given. For oligo-zwitterion ligands, at least three points of interest related to their use in the synthesis of coordination polymers and frameworks arise:
(i) Unlike mono-zwitterion ligands, where bridging must bring metal ions into close proximity, oligo-zwitterions should have the capacity to link metal ions at larger separations, thereby having the potential to create more open networks with enhanced porosity.
(ii) Being uncharged, oligo-zwitterions should have the capacity to form cationic coordination polymers associated with small anions and thus the potential to act as anion exchange materials.
(iii) Being uncharged, oligo-zwitterions should also have the capacity to form coordination polymers in association with anionic bridging ligands capable of forming coordination polymers themselves alone, and thus modifying or reinforcing the structures of those simpler polymers.
X-ray structural data sufficiently extensive to consider all three points is available in the furthering, beginning with dizwitterion studies,72 of the work described in section 1 on carboxylate-donor zwitterions and as well in the coordination chemistry of the aza-aromatic N-oxide, 4,4′-bipyridine-N,N′-dioxide alone.73–76 Thus, complexes of the double betaine 1,4-diazoniabicyclo[2.2.2]octane-1,4-diacetate (L12) with Zn(ClO4)2 and ZnBr2
72a are diperiodic and monoperiodic polymers, respectively, though only the former can be regarded as a cationic species, as bromide coordination occurs in the latter. In both cases, the ligand acts as a bis(κ1O) bridge. Complexes of the double betaines 3,7-diazonia-3,3,7,7-tetramethylnonane-1,9-dioate (L13) and 3,8-diazonia-3,3,8,8-tetramethyldecane-1,10-dioate (L14) with one early (Nd) and one late (Er) lanthanide(III) ions72b consist of cationic, diperiodic coordination polymers involving in total (κ1O), (κ2O,O′), (κ1O;κ1O′) and (κ2O,O′;κ1O′) bonding modes of the carboxylate groups, with the double betaines alone providing the bridges between the cations, the perchlorate counter anions not being directly bound to them (Fig. 7).
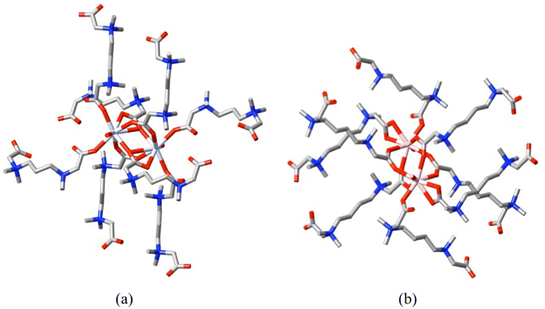 |
| Fig. 7 Perspective views of the dimer units and their dizwitterion ligand environments in (a) the Nd(III) perchlorate complex of 3,7-diazonia-3,3,7,7-tetramethylnonane-1,9-dioate (L13) (CCDC 125139872b), where the carboxylate bonding modes are κ1O,κ1O;κ1O′ and κ2O,O′;κ1O′ and (b) the Er(III) perchlorate complex of 3,8-diazonia-3,3,8,8-tetramethyldecane-1,10-dioate (L14) (CCDC 1251401,72b where the carboxylate bonding modes are κ1O,κ1O;κ1O′ and κ2O,O′. (Nd = pale blue-violet, Er = pink.) | |
Similar features have been observed in other lanthanide(III) complexes of the dizwitterion 1,4-di(4-carboxylatopyridiniomethyl)benzene (L15), where the κ1O bonding mode seems to predominate, though accompanied by κ1O;κ1O′.77 An isomer of this ligand, 1,4-di(3-carboxylatopyridiniomethyl)benzene (L16) has been shown16 to form transition metal (Co and Zn) polymer complexes where in the Co(II) species it binds as a bis(κ1O;κ1O′) bridge while in the Zn(II) complex it bridges in a bis(κ1O) fashion. A dizwitterion where the positive centre is a metal ion is [Ni(tpyc)2], L17, (tpyc = 2,2′:6′,2′′-terpyridine-4′-carboxylate), an “expanded ligand”78 and a particular member of the broad class of metalloligands,79 where any charge carried by external substituents on a bound ligand is not necessarily balanced by that of the metal, a factor which certainly does not prevent their acting as bridging species in coordination polymers.67,68,80 Both this ligand81 and its further expanded analogues, [MII(cptpy)2] (L18) (cptpy = 4′-(4-carboxylatophenyl)-2,2′:6′,2′′-terpyridine; M = Fe, Co, Ni)82 have been shown to form coordination polymers with uranyl ion where both κ1O and κ2O,O′ bonding modes are seen (Fig. 8). (Another large family of organometallic metallazwitterions exists where the metal ion may be regarded in some cases as the positive site and in others as the negative but interest in such species has been largely limited to their applications as solution catalysts.13)
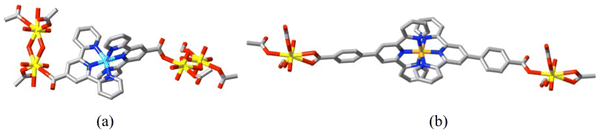 |
| Fig. 8 Portions of (a) the cationic diperiodic polymer present in [UO2Ni(tpyc)2(OH)(OH2)]·NO3·1.5H2O (CCDC 210558081a), showing the bis(κ1O) mode of bonding of the zwitterion and (b) the neutral monoperiodic polymer present in [(UO2)2Fe(cptpy)2(O2CH)4] (CCDC 2027199;82 isostructural with its Co and Ni analogues), showing the mixed κ1O plus κ2O,O′ mode of bridging by the larger expanded ligand. (Fe = orange, Ni = sky blue.) | |
The relative wealth of dizwitterions available is not matched by that of trizwitterions and studies of their coordination chemistry are therefore more limited. The ligand 1,1′,1′′-(2,4,6-trimethylbenzene-1,3,5-triyl)-trimethylenetris(4-carboxylatopyridinium) (L19) forms coordination polymers with Cu(II), Zn(II) and Cd(II)14 in which both κ1O and κ2O,O′ bonding modes are again seen, though in two of the four structures reported only two carboxylate groups of the ligand are bound. In linking tetranuclear U(VI) clusters into a diperiodic (layered) polymer structure,83 however, it functions as a tris(κ1O) ligand (giving a complex noted as the first cationic uranyl-organic framework, though it may also be noted that in a related neutral complex involving diuranate units the ligand adopts an otherwise unknown bis(κ1O);κ2O bonding mode; Fig. 9(a)). While the ligand there has a chair-like conformation as seen in the Eu(III) complex polymer,15 the Eu species is triperiodic and involves tris(κ1O;κ1O′) Eu(III)-carboxylate interactions (Fig. 9(b)). An interesting aspect of the crystal structure of the protonated ligand isolated as the tribromide14 is the inclusion of one Br− within a capsule formed by two facing ligands in a tripodal form (Fig. 9(c)) and even after deprotonation for the formation of the trizwitterion complexes, the interaction involved appears to have a structural influence (discussed ahead in relation to structures of mixed-ligand complexes involving trizwitterions).
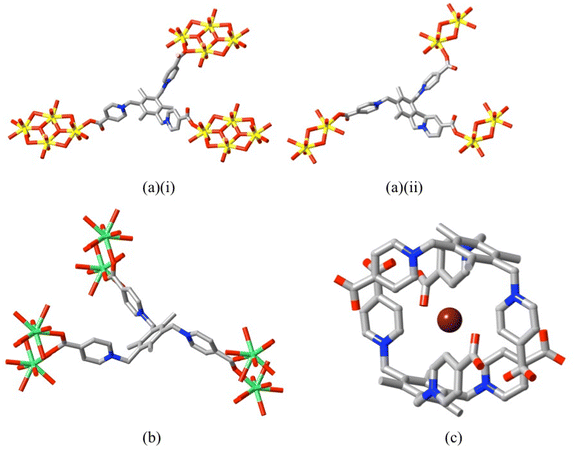 |
| Fig. 9 (a) A 1,1′,1′′-(2,4,6-trimethylbenzene-1,3,5-triyl)-trimethylenetris(4-carboxylatopyridinium) ligand (L19) and its interactions with uranate clusters in (i) the neutral complex [(UO2)2(L19)(OH)O(O2CH)](CCDC 150007683), where two arms bind in a κ1O fashion and one as κ2O, and in (ii) the cationic species [UO2(L19)(OH)]Br (CCDC 150007783), where the bonding is tris(κ1O) (U = yellow); (b) interactions of the ligand in [Eu(L19)(OH2)2](NO3)3 (CCDC 101522615) where a tris(κ1O;κ1O′) mode is adopted (Eu = green); (c) encapsulation of a bromide ion within the structure of [H3L19]Br3 (CCDC 81912614) (Br = brown). | |
The behaviour of 4,4′-bipyridine-N,N′-dioxide (4,4′-bpdo = L20) as a dizwitterionic ligand, especially as seen in coordination to the lanthanides,76 is remarkably different to that of the polybetaine-related species just discussed. It is a ligand of very limited flexibility and can be considered as a linear rod, though some degree of twisting of one pyridine ring relative to the other is usually seen and binding by the O-donors involves bent M–O–N bond angles, so that convergent (syn) and divergent (anti) bridging modes are possible.76 Like dibetaines, it is a good bridging ligand and favours solid state polymerisation but its capacity to exploit the high coordination numbers of the lanthanides results in species of exceptionally high connectivity involving La(III), for example, in 8-coordination by bpdo only (Fig. 10(a)), better described as frameworks84 rather than simply as coordination polymers, though not all are strictly triperiodic.85 In contrast, transition metal ions generally provide solid complexes with low metal-ion
:
4,4′-bpdo ratios, resulting in polymers which are monoperiodic (chains) or diperiodic (sheets) showing little or no porosity.75,86 Units such as [Cu(4,4′-bpdo)6]2+ and [Cd(4,4′-bpdo)6]2+ are known but simply as mononuclear, non-polymeric complexes.75 Even with very heavy metal ions such as Bi(III), which otherwise shows many similarities to the lanthanides in its coordination chemistry, species with a 1
:
1 metal-ion
:
4,4′-bpdo ratio where just monoperiodic polymer chains are present can be isolated (Fig. 10(b)).73
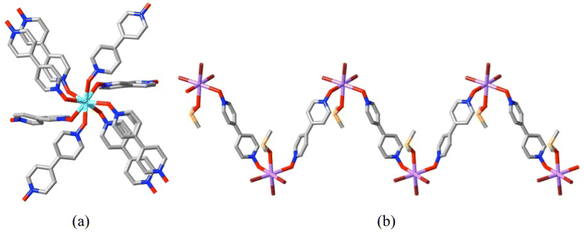 |
| Fig. 10 Differing stoichiometry in complexes of 4,4′-bpdo: (a) the eight-coordinate La(III) centre in the diperiodic polymer [La(L20)4](ClO4)3 (CCDC 23960885) where all ligands bridge in a bis(κ1O) manner to other La(III) ions (not shown). (La = sky blue); (b) similar bridging of Bi(III) centres in the monoperiodic polymer [BiBr3(dmso)(L20)] (CCDC 200867473). (Bi = violet, Br = brown, S = orange; partial disorder of the dmso ligands not shown.) | |
The essential message to be gleaned from the discussion above is that oligo-zwitterions with a variety of functional groups can give rise alone to coordination polymers and frameworks. It is misleading to conclude that this means that metal ion separations in these materials must be large, since one carboxylate group alone can still serve as a bridge, as can (but rarely76) one oxygen of 4,4′-bpdo. In the Eu(III) complex of 1,1′,1′′-(2,4,6-trimethylbenzene-1,3,5-triyl)-trimethylenetris(4-carboxylatopyridinium) (L19), for example,15 the Eu⋯Eu separation of the pair linked by the ligand arm which binds in the κ1O;κ1O′ manner is 4.47(1) Å, while the Eu⋯Eu separations between cations on separate arms range from 12.788(3) to 19.4(5) Å. In the mixed-ligand complex [La4(4,4′-bpdo)3(bztfacac)6]·3H2O,87 (bztfacac2− = benzoyltrifluoroacetonate) the La(III) centres bridged by κ2O bonding of one 4,4′-bpdo oxygen are 4.225(1) Å apart, while other close La⋯La separations range between 11.320(7) and 13.123(2) Å. A feature of all the complexes discussed above is that there is very little evidence, from either direct experimentation75 or estimates of the Kitaigorodsky porosity index (KPI) (calculated with PLATON88) cited in numerous publications (e.g. ref. 81a), of their capacity to undergo reversible absorption of small molecules or of the presence of cavities large enough to accommodate organic molecules, in part a consequence of the common observation of interpenetration.76,81 This is a situation perhaps in the process of being redressed,89 with the implication that success in creating a coordination polymer showing reversible gas absorption resulting from the use of the very rigid dizwitterion 1,4-di(4-carboxylatophenyl)-1,4-bipyridinium (L21) (Fig. 11), being that the flexibility of the systems discussed above may have been a disadvantage. Nonetheless, these are systems in which the magnetic, spectroscopic and ion exchange properties have provided numerous aspects of interest.73,77,90,91 Note that in the structure83 of the uranyl ion complex of the trizwitterion L19, 1,1′,1′′-(2,4,6-trimethylbenzene-1,3,5-triyl)-trimethylenetris(4-carboxylatopyridinium), shown to undergo anion exchange with retention of the coordination polymer structure, the counter anions are not coordinated to the metal ion, so that the polymer is truly cationic and the system can be regarded as equivalent to conventional organic ion exchangers. Given the lability of equatorial sites on U(VI),92 it might be anticipated that non-bridging counter-anions coordinated to U(VI) sites of a zwitterion coordination polymer, thus making it a neutral species, would also undergo exchange. While such exchange has not been directly demonstrated for any uranyl ion complex of the metallazwitterion [Ni(tpyc)2], it has been found possible to isolate complexes with different counter-anions, some (F−, NO3−, CH2(SO3)22−) proving to be coordinated, others (CF3SO3−, I3−) not.93 Here, however, the form of the coordination polymer varies with each anion, indicating that direct exchange reactions would probably not be reversible.
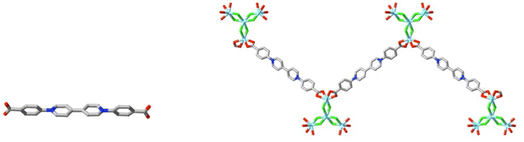 |
| Fig. 11 The rigid ligand 1,4-di(4-carboxylatophenyl)-1,4-bipyridinium (L21) and a fragment of the triperiodic polymer formed with CdCl2, [Cd4(L21)3Cl6][CdCl4]·8H2O (CCDC 147308289), showing the bis(κ2O,O′) bonding mode to Cd(II). (Cd = light blue, Cl = green.) | |
The observations that coordination polymers in which oligo-zwitterions are the principal bridging units are usually of negligible porosity and may not always be suitable for ion exchange reactions appear to pose limits on their applications. It has long been recognised, at least for uranyl ion complexes in general,94,95 however, that mixed-ligand species can have novel properties96 and this approach has been the basis of recent developments in oligo-zwitterion chemistry in this domain. Luminescence is a fundamental aspect of U(VI) chemistry97 and in mixed-ligand complexes where both 4,4′-bpdo and polycyanometallates function as bridging ligands to give both di- and triperiodic polymer networks (Fig. 12), the usual green emission97 from uranyl ions has been shown to be modifiable over a range of colours from green to orange depending upon the cyanometallate.98 This is viewed as having possible application in new light-emitting devices. The more obvious potential applications of uranyl ion complexes are of course those based on the photo-oxidation capacity of U(VI) centres97,99 and it has been shown, for example, that uranyl ion coordination polymers can be used for removal and visible-light photoactivated destruction of rhodamine B present as an environmental contaminant.100 Any use of a radioactive material for such purposes must involve rather stringent requirements concerning security, the scale of usage and recovery and recyclability of the catalyst,69 so that small-scale use of uranyl ion coordination polymers or frameworks as heterogeneous catalysts for selective synthetic transformations would seem to be a more realistic prospect.
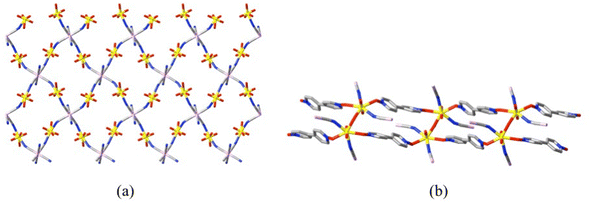 |
| Fig. 12 Partial views of the components of the triperiodic coordination framework found in [(UO2)2(OH)(L20)2][Ir(CN)6] (CCDC 185791698) showing (a) a section of the diperiodic network formed by bis(κ1N) bridging of uranyl centres by four of the cyano groups of each [Ir(CN)6]3− unit and (b) the double-chain of uranyl centres linked by bis(κ1O) coordination of 4,4′-bpdo ligands and crosslinked by hydroxido bridges that, through connection further to [Ir(CN)6]3− units, generates the full 3-dimensional structure. | |
Although as yet unexplored in regard to any possible applications, it has been demonstrated17,82,101–104 that carboxylate di- or tri-zwitterions and anionic polycarboxylates can form mixed ligand coordination polymers in which both ligands act as bridges, seemingly consistent with the different donor species having almost identical bonding interactions. Bond lengths to zwitterion carboxylate-O and anionic carboxylate-O are similar but the common adoption of a bis or tris(κ1O) mode of bonding by the zwitterions may indicate that they have a weaker structural influence and in the particular case of thiophene-2,5-dicarboxylate as the anion,103 the isolated crystals have the composition of a mixed-ligand species but the structure in fact involves independent polymer networks of the two ligands (Fig. 13), meaning that the solid is ionic, where a cationic polymer formed by the zwitterion 1,1′-[(2,3,5,6-tetramethylbenzene-1,4-diyl)bis(methylene)]bis(pyridin-1-ium-3-carboxylate), L22, is accompanied by an anionic polymer formed by the dicarboxylate. Thus, it is not clear that a true mixed ligand species must be obtained rather than separate species, each with a unique bridging component. While there is little indication of significant porosity in any of the complexes, the complex formed101 from adamantane-1,3-dicarboxylate and 1,1′,1′′-(2,4,6-trimethylbenzene-1,3,5-triyl)-trimethylenetris(4-carboxylatopyridinium) (L19) actually contains an encapsulated bromide ion bound (through Br⋯HC interactions) in a manner similar to that found in the bromide salt of the protonated trizwitterion, this cation also being a component of the structure. Hence, the appropriate choice of zwitterion might be a means of controlling guest inclusion in such complexes. Added to these considerations, it has also been shown recently104 that the moderately flexible dizwitterion 4,4′-bis(carboxylatoethyl)-4,4′-bipyridinium (L23) in combination with 1,3-phenylenediacetate (1,3-pda2−) can form a chiral, binuclear cage species [(UO2)2(L23)(1,3-pda)2]. Cage complexes are relatively rare in U(VI) coordination chemistry105 but they bring with them the posssibility of selective inclusion of substrates susceptible to reaction with photoexcited U(VI) as well as the possibility of sufficient solubility for use as homogeneous catalysts. Little is presently known in these regards.
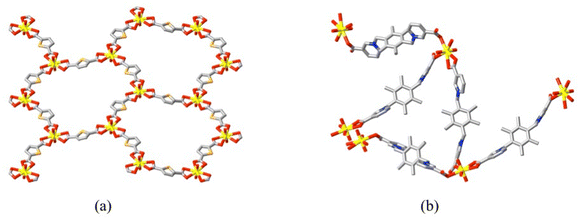 |
| Fig. 13 Partial views of the ionic components of the mixed-ligand uranyl ion complex of L′′ = 1,1′-[(2,3,5,6-tetramethylbenzene-1,4-diyl)bis(methylene)]bis(pyridin-1-ium-3-carboxylate) and thiophene-2,5-dicarboxylate (TDC), [(UO2)2(L′′)3(H2O)2][(UO2)2(TDC)3]2·10H2O (CCDC 2202990101) showing (a) a section of the diperiodic, distorted honeycomb annionic polymer involving TDC2− only and (b) portion of the undulating cationic polymer formed by κ1O plus κ1O;κ1O′ bridging by L′′ only. | |
This is far from a comprehensive review of zwitterion coordination chemistry, its focus on solid state structures being intended only to define the benefits that may derive from the use of oligozwitterions in the generation of coordination polymers and frameworks, particularly in the formation of mixed-ligand species. Although only two binding units (N-oxide and carboxylate-O) have been considered in any detail as components of oligozwitterions, their properties are such as to make such zwitterions efficacious as ligands in a variety of novel materials. The differences observed between di- and tri-zwitterions indicate that extension of the list to higher species offering something approaching the extraordinary variety of known polycarboxylates could give rise a considerable expansion of the area and a range of surprises in the structures obtained.
Conflicts of interest
There are no conflicts to declare.
References
-
V. R. Preedy, Betaine: Chemistry, Analysis, Function and Effects, Royal Society of Chemistry, 2015 Search PubMed
.
- See, for example: M. Liebeke and J. G. Bundy, Biochemical diversity of betaines in earthworms, Biochem. Biophys. Res. Commun., 2013, 430, 1306–1311 CrossRef CAS PubMed
.
- R. S. McEwen, Crystal structures of complexes with zwitterionic ligands, J. Chem. Soc., Chem. Commun., 1973, 68–69 RSC
.
- H.-G. Unruh, F. Hero and V. Dvorak, On the incomplete devil's staircase of betaine calcium chloride dihydrate, Solid State Commun., 1989, 70, 403–408 CrossRef CAS
.
-
X.-M. Chen, Synthesis and Structural Studies of Some Metal Complexes of Betaines, Doctoral thesis, The Chinese University of Hong Kong, 1992 Search PubMed
.
-
(a) X.-M. Chen and T. C. W. Mak, Metal-betaine interactions I. Crystal structure of polymeric trans-diaquabis(pyridine betaine)manganese(II) dichloride, J. Crystallogr. Spectrosc. Res., 1991, 21, 21–25 CrossRef CAS
;
(b) X.-M. Chen and T. C. W. Mak, Metal-Betaine Interactions. XXI: Crystal structures of lithium 3-carboxy-1-pyridinioacetate monohydrate and sodium 3-carboxy-1-pyridinioacetate tetrahydrate, J. Crystallogr. Spectrosc. Res., 1993, 23, 291–296 CrossRef CAS
. (Intervening contributions, including ref. 10 and 11 below, to these extensive studies are referred to therein).
- M.-Y. Chow, Z.-Y. Zhou and T. C. W. Mak, A linear polymeric copper(II) complex bridged simultaneously by azido, nitrato, and betaine ligands. Crystal structure of catena-[bis(μ-(1,1)-azido)bis(μ-nitrato-O,O′)bis-((μ-trimethylammonio)acetato-O,O′)dicopper(II)], [Cu2(N3)2(NO3)2(Me3NCH2CO2)2]n, Inorg. Chem., 1992, 31, 4900–4902 CrossRef CAS
.
- M. R. Chaves, A. Almeida, J. C. Tolédano, J. Schneck, J. M. Kiat, W. Schwarz, J. L. Ribeiro, A. Klöpperpieper, J. Albers and H. E. Müser, Effect of electric fields on modulated structure of deuterated betaine calcium chloride dihydrate, Phys. Rev. B: Condens. Matter Mater. Phys., 1993, 48, 13318–13325 CrossRef CAS PubMed
.
-
(a) J. Schreuer and S. Haussühl, Crystal structure of catena-tri-μ-trimethylammonium acetatomanganese tetrachloromanganate (((CH3)3NCH2COO)3Mn) MnCl4, Z. Kristallogr., 1993, 205, 309–310 CrossRef CAS
;
(b) J. Schreuer and S. Haussühl, Crystal structure of catena-(trimethylammoniumacetato) tetrachloro-aquadimanganese, ((CH3)3NCH2COO)Cl4(H2O)Mn2, Z. Kristallogr., 1993, 205, 313–315 CAS
.
- Y.-X. Tong, X.-M. Chen and S. W. Ng, Synthesis and crystal structures of two polymeric cadmium(II) complexes containing skew-skew carboxylato-bridges, Polyhedron, 1997, 16, 3363–3369 CrossRef CAS
.
- X.-M. Chen and T. C. W. Mak, Metal-betaine interactions. Part 17. A study of intradimer Cu⋯Cu distance in copper(II) betaine complexes containing [Cu2(carboxylato-O,O′)4L2]n+ species, Struct. Chem., 1993, 4, 247–259 CrossRef CAS
.
- X.-M. Chen, T. C. W. Mak, W.-Y. Huang and L. Lu, Metal-betaine interactions. XI. Structure of catena-[μ-dichloro(triethylammonioacetato)cadmium(II)], Acta Crystallogr., Sect. C: Cryst. Struct. Commun., 1992, 48, 57–59 CrossRef
.
- R. Puerta-Oteo, A. I. Ojeda-Amador, M. V. Jiménez and J. J. Pérez-Torrente, Catalytic applications of zwitterionic transition metal compounds, Dalton Trans., 2022, 51, 817–830 RSC
.
- G. Q. Kong and C.-D. Wu, Four Novel Coordination Polymers Based on a Flexible Zwitterionic Ligand and Their Framework Dependent Luminescent Properties, Cryst. Growth Des., 2010, 10, 4590–4595 CrossRef CAS
.
- R. M. Wen, S.-D. Han, G.-J. Ren, Z. Chang, Y.-W. Li and X.-H. Bu, A flexible zwitterion ligand based lanthanide metal–organic framework for luminescence sensing of metal ions and small molecules, Dalton Trans., 2015, 44, 10914–10917 RSC
.
- J.-J. Liu, S.-B. Xia, X. Shen, D. Liu and F.-X. Cheng, Two metal-carboxylate-azide coordination networks derived from 1,4-bis(3-carboxylatopyridinium-1-methylene)benzene: Synthesis, structure and properties, J. Solid State Chem., 2019, 275, 88–94 CrossRef CAS
.
- P. Thuéry and J. Harrowfield, Ni(2,2′:6′,2′′-terpyridine-4′-carboxylate)2 Zwitterions and Carboxylate Polyanions in Mixed-Ligand Uranyl Ion Complexes with a Wide Range of Topologies, Inorg. Chem., 2022, 61, 9725–9745 CrossRef PubMed
.
- H. C. Freeman, Crystal Structures of Metal-Peptide Complexes, Adv. Protein Chem., 1967, 22, 257–424 CrossRef CAS PubMed
.
- T. Wyttenbach, M. Witt and M. T. Bowers, On the Stability of Amino Acid Zwitterions in the Gas Phase: The Influence of Derivatization, Proton Affinity, and Alkali Ion Addition, J. Am. Chem. Soc., 2000, 122, 3458–3464 CrossRef CAS
.
- B. Sarac and S. Hadzi, Analysis of Protonation Equilibria of Amino Acids in Aqueous Solutions Using Microsoft Excel, J. Chem. Educ., 2021, 98, 1001–1007 CrossRef CAS
, and references therein.
- D. Munz and K. Meyer, Charge frustration in ligand design and functional group transfer, Nat. Rev. Chem., 2021, 5, 422–439 CrossRef CAS PubMed
.
- M. Birus, N. Kujundzic, M. Pribanic and Z. Tabor, Equilibrium Studies on Complexation of Iron(III) by Acet-, Glycinium and Betaine Hydroxamic Acids, Croat. Chim. Acta, 1984, 57, 313–324 CAS
.
- P. De Meester, S. R. Fletcher and A. C. Skapski, Refined crystal structure of tetra-m-acetato-bisaquodicopper(II), J. Chem. Soc., Dalton Trans., 1973, 2575–2578 RSC
.
- T. Kawata, H. Uekusa, S. Ohba, T. Furukawa, T. Tokii, Y. Muto and M. Kato, Magneto-structural correlation in dimeric copper(II) benzoates, Acta Crystallogr., Sect. B: Struct. Sci., 1992, 48, 253–261 CrossRef
.
- S. J. Jenniefer and P. T. Muthiah, Synthesis, characterisation and X-ray structural studies of four copper(II) complexes containing dinuclear paddle-wheel structures, Chem. Cent. J., 2013, 7, 35 CrossRef PubMed
.
- J. Harrowfield and P. Thuéry, Charge localisation in heavy alkali metal ion complexes of 4,4′-biphenyldicarboxylate, Aust. J. Chem., 2016, 69, 505–511 CrossRef CAS
.
-
(a) L. Wiehl, J. Schreuer, E. Haussühl, B. Winkler, K. Removic-Langer, B. Wolf, M. Lang and V. Milman, Structural and magnetic properties of betaine adducts with transition metals: I. ((CH3)3NCH2COO)3Mn MCl4 with M = Mn2+, Co2+, Zn2+, J. Phys.: Condens. Matter, 2006, 18, 11067–11079 CrossRef CAS
;
(b) E. Haussühl, J. Schreuer, L. Wiehl and N. Paulsen, Structure-property relations of orthorhombic [((CH3)3NCH2COO)2(C uCl2)3·2H2O, J. Solid State Chem., 2014, 212, 205–212 CrossRef
.
-
(a) P. Nockemann, B. Thijs, S. Pittois, J. Thoen, C. Gloriex, K. Van Hecke, L. Van Meervelt, B. Kirchner and K. Binnemans, Task-specific ionic liquid for solubilizing metal oxides, J. Phys. Chem. B, 2006, 110, 20978–20992 CrossRef CAS PubMed
;
(b) P. Nockemann, R. Van Deun, B. Thijs, D. Huys, E. Vanecht, K. Van Hecke, L. Van Meervelt and K. Binnemans, Uranyl complexes of carboxyl-functionalized ionic liquids, Inorg. Chem., 2010, 49, 3351–3360 CrossRef CAS PubMed
.
- X.-Y. Chen, G. S. Goff, M. Quiroz-Guzman, D. P. Fagnant Jr., J. F. Brennecke, B. L. Scott and W. Runde, Directed nucleation of monomeric and dimeric uranium(VI) complexes with a room temperature carboxyl-functionalized phosphonium ionic liquid, Chem. Commun., 2013, 49, 1903–1905 RSC
.
- P. A. Smith and P. C. Burns, Ionothermal effects on low-dimensionality uranyl compounds using task-specific ionic liquids, CrystEngComm, 2014, 16, 7244–7250 RSC
.
- P. A. Smith, T. L. Spano and P. C. Burns, Synthesis and structural characterization of a series of uranyl-betaine coordination complexes, Z. Kristallogr. - Cryst. Mater., 2018, 233, 507–513 CrossRef CAS
.
- M. Llaver, E. F. Fiorentini, P. Y. Quintas, M. N. Oviedo, M. B. Botella Arenas and R. G. Wuilloud, Task-specific ionic liquids: Applications in sample preparation and the chemistry behind their selectivity, Adv. Sample Prep., 2022, 1, 100004 CrossRef
.
- A. S. Lermontov, E. K. Lermontova and Y.-Y. Wang, Synthesis, structure and optic properties of 2-methylimidazolium and 2-phenylimidazolium uranyl acetates, Inorg. Chim. Acta, 2009, 362, 3751–3755 CrossRef CAS
.
- W. H. Zachariasen and H. A. Plettinger, Crystal Chemical Studies of the 5f Series of Elements. XXV. The Crystal Structure of Sodium Uranyl Acetate, Acta Crystallogr., 1959, 12, 526–530 CrossRef CAS
.
- Q. Shao and S. Jiang, Molecular Understanding and Design of Zwitterionic Materials, Adv. Mater., 2014, 27, 15–26 CrossRef PubMed
.
- S.-J. Bao, C. Y. Liu, M. Zhang, X.-R. Chen, H. Yu, H.-X. Li, P. Braunstein and J.-P. Lang, Metal complexes with the zwitterion 4-(trimethylammonio)benzene thiolate: Synthesis, structures and applications, Coord. Chem. Rev., 2019, 397, 28–53 CrossRef CAS
.
- Y. Marcus and G. Hefter, Ion Pairing, Chem. Rev., 2006, 106, 4585–4621 CrossRef CAS PubMed
.
- Y. Wang and L. Zhang, Recent Developments in the Chemistry of Heteroaromatic N-oxides, Synthesis, 2015, 289–305 Search PubMed
.
-
(a) M. Orchin and P. J. Schmidt, Pyridine 1-oxide complexes of platinum(II), Coord. Chem. Rev., 1968, 3, 345–373 CrossRef CAS
;
(b) R. G. Garvey, J. H. Nelson and R. O. Ragsdale, The coordination chemistry of aromatic amine N-oxides, Coord. Chem. Rev., 1968, 3, 375–407 CrossRef CAS
;
(c) N. M. Karayannis, L. L. Pydewski and C. M. Mikulski, Metal complexes of aromatic amine N-oxides, Coord. Chem. Rev., 1973, 11, 93–159 CrossRef CAS
;
(d) L. Ramakrishnan and S. Soundararajan, Pyridine 1-oxide complexes of lanthanide iodides, Monatsh. Chem., 1975, 106, 625–632 CrossRef CAS
.
- M. Soleilhavoup and G. Bertrand, Borylenes: An Emerging Class of Compounds, Angew. Chem., Int. Ed., 2017, 56, 10282–10292 CrossRef CAS
.
- F. E. Hahn, Introduction: carbene chemistry, Chem. Rev., 2018, 118, 9455–9456 CrossRef CAS PubMed
, and following articles.
- D. Wannipurage, S. S. Kurup and S. Groysman, Heterocoupling of Different Aryl Nitrenes to Produce Asymmetric Azoarenes using Iron-Alkoxide Catalysis and Investigation of the Cis-Trans Isomerism of Selected Bulky Asymmetric Azoarenes, Organometallics, 2021, 40, 3637–3644 CrossRef CAS
.
-
(a) F. H. C. Stewart, The chemistry of the sydnones, Chem. Rev., 1964, 64, 129–147 CrossRef CAS
;
(b) W. Baker and W. D. Ollis, Meso-ionic compounds, Q. Rev., Chem. Soc., 1957, 11, 15–29 RSC
;
(c) X. Bantreil, N. Pétry and F. Lamaty, Coordination complexes involving sydnones as ligands, Dalton Trans., 2019, 48, 15753–15761 RSC
.
- A. Sarbajna, V. S. V. S. N. Swamy and V. H. Gessner, Phosphorus ylides: powerful substituents for the stabilisation of reactive main group compounds, Chem. Sci., 2021, 12, 2016–2024 RSC
.
- C. Wentrup, Carbenes and Nitrenes: Recent Developments in Fundamental Chemistry, Angew. Chem., Int. Ed., 2018, 57, 11508–11521 CrossRef CAS PubMed
.
- A. Jayaraj, A. V. Raveedran, A. T. Latha, D. Priyadarshini and P. C. A. Swamy, Coordination Versatility of NHC-metal Topologies in Asymmetric Catalysis: Synthetic Insights and Recent Trends, Coord. Chem. Rev., 2023, 478, 214922 CrossRef CAS
.
-
(a) A.-L. Lücke, S. Wiechmann, T. Freese, Z. Guan and A. Schmidt, Palladium complexes of anionic N-heterocyclic carbenes derived from sydnones in catalysis, Z. Naturforsch., 2016, 71, 643–650 CrossRef
;
(b) S. Mummel, F. Lederle, E. G. Hübner, J. C. Namyslo, M. Nieger and A. Schmidt, Sydnone Methides – A Forgotten Class of Mesoionic Compounds for the Generation of Anionic N-Heterocyclic Carbenes, Angew. Chem., Int. Ed., 2021, 60, 18882–18887 CrossRef CAS PubMed
.
- D. Bourissou, O. Guerret, F. P. Gabbai and G. Bertrand, Stable Carbenes, Chem. Rev., 2000, 100, 39–91 CrossRef CAS PubMed
.
- A. J. Arduengo, R. L. Harlow and M. Kline, A stable crystalline carbene, J. Am. Chem. Soc., 1991, 113, 361–363 CrossRef CAS
.
- M. V. Baker, D. H. Brown, P. Simpson, B. W. Skelton and A. H. White, Palladium complexes of o-xylyl-linked alkoxybenzimidazolin-2-ylidenes: interesting structural conformations and applications in catalysis, Dalton Trans., 2009, 7294–7307 RSC
.
- J. Huang, E. D. Stevens, S. P. Nolan and J. L. Petersen, Olefin Metathesis-Active Ruthenium Complexes Bearing a Nucleophilic Carbene Ligand, J. Am. Chem. Soc., 1999, 121, 2674–2678 CrossRef CAS
.
-
(a) N. L. Ahlburg, T. Freese, S. Kolb, S. Mummel, A. Schmidt and D. B. Werz, Functionalisation of Sydnones with Donor-Acceptor Cyclopropanes, Cyclobutanes and Michael Acceptors, Eur. J. Org. Chem., 2021, 1603–1606 CrossRef CAS
;
(b) L. Plougastel, D. Lamaa, E. Yen-Pon, D. Audisio and F. Taran, Fluorogenic Probes based on polycyclic sydnone scaffolds, Tetrahedron, 2020, 76, 131250 CrossRef CAS
.
- Y. Wang, P. L. Lee and M.-Y. Yeh, Structure of 3-(p-ethoxyphenyl)sydnone (1), C10H10N2O3, and 3-(p-tolyl)sydnone (2), C9H8N2O2, Acta Crystallogr., Sect. C: Cryst. Struct. Commun., 1984, 40, 1226–1228 CrossRef
.
-
(a) W. Hill and L. E. Sutton, Les moments dipolaires de quelques composés semi-ioniques, J. Chim. Phys., 1949, 46, 244–248 CrossRef
;
(b) L. E. Orgel, T. L. Cottrell, W. Dick and L. E. Sutton, The calculation of the electric dipole moments of some conjugated heterocyclic compounds, Trans. Faraday Soc., 1951, 47, 113–119 RSC
.
-
(a) C. F. McKenzie, P. R. Spackman, D. Jayatilaka and M. A. Spackman, CrystalExplorer model energies and energy frameworks: extension to metal coordination compounds, organic salts, solvates and open-shell systems, IUCrJ, 2017, 4, 575–587 CrossRef PubMed
;
(b)
P. R. Spackman, M. J. Turner, J. J. McKinnon, S. K. Wolff, D. J. Grimwood, D. Jayatilaka and M. A. Spackman, Crystal Explorer 21.5, University of Western Australia, Perth, Australia, 2021 Search PubMed
.
- P. S. Gentile and T. L. Mao, Preparation of Complexes of Sydnones by Solid-Solid Interaction, J. Inorg. Nucl. Chem., 1965, 27, 867–869 CrossRef CAS
.
- N. Pétry, T. Vanderbeeken, A. Mather, Y. Bringer, P. Retailleau, X. Bantreil and F. Lamaty, Mechanosynthesis of sydnone-containing coordination complexes, Chem. Commun., 2019, 55, 9495–9498 RSC
.
- M.-H. Shih, J. C. Chen, G.-L. Lin, T.-T. Lin and M.-H. Sun, Novel synthesis of palladium(II) complexes derived from 3-arylsydnone-4-carbaldehyde N(4)-phenylthiosemicarbazones and biological activity, J. Pharm. Pharmacol., 2014, 66, 73–83 CrossRef CAS PubMed
.
- S.-T. Lin, H.-S. Choe, S.-H. Liu and J. C. Wang, Preparation of complexes of palladium with di-tert-butylsydnonylphosphine and diphenylsydnonylphosphine and structural analysis of the sydnone ring containing phosphine and palladium complex, J. Organomet. Chem., 2000, 610, 1–7 CrossRef CAS
.
- T. M. Cooper, B. C. Hall, D. G. McLean, J. E. Rogers, A. R. Burke, K. Turnbull, A. Weisner, A. Fratini, Y. Liu and K. S. Schanze, Structure-Optical Property Relationships in Organometallic Sydnones, J. Phys. Chem. A, 2005, 109, 999–1007 CrossRef CAS PubMed
.
- J. M. Tien and I. M. Hunsberger, Sydnones. III. Preparation and Interconversion of Mercurated Derivatives of N-(3-Pyridyl)-sydnone, J. Am. Chem. Soc., 1961, 83, 178–182 CrossRef CAS
.
-
(a) V. N. Kalinin, S.-F. Min and P. V. Petrovskii, Synthesis of sydnone complexes with C4-Pd and C4-Pt σ-bonds. Application of palladium salts for the preparation of 4-alkenylsydnones, J. Organomet. Chem., 1989, 379, 195–199 CrossRef CAS
;
(b) L. N. Morozova, L. S. Isaeva, P. V. Petrovskii, D. N. Kratsov, S.-F. Min and V. N. Kalinin, Nickel σ-sydnonyl, stabilsed by tertiary phosphines, J. Organomet. Chem., 1990, 381, 281–284 CrossRef CAS
.
- S. Kolodych, E. Rasolofonjatovo, M. Chaumontet, M.-C. Nevers, C. Crémino and F. Taran, Discovery of Chemoselective and Biocompatible Reactions Using a High-Throughput Immunoassay Screening, Angew. Chem., Int. Ed., 2013, 52, 12056–12060 CrossRef CAS PubMed
.
- A. B. Lowe and C. L. McCormick, Synthesis and solution properties of zwitterionic polymers, Chem. Rev., 2002, 102, 4177–4190 CrossRef CAS PubMed
.
- S. Racovita, M.-A. Trofin, D. F. Loghin, M.-M. Zaharia, F. Bucatariu, M. Mihal and S. Vasiliu, Polybetaines in Biomedical Applications, Int. J. Mol. Sci., 2021, 22, 9321 CrossRef CAS PubMed
.
- S. J. Nikkhah and M. Vandichel, Modeling Polyzwitterion-Based Drug Delivery Platforms: A Perspective of the Current State-of-the-Art and Beyond, ACS Eng. Au, 2022, 2, 274–294 CrossRef PubMed
.
- K. Berijani, L.-M. Chang and Z.-G. Gu, Chiral templated synthesis of homochiral metal-organic frameworks, Coord. Chem. Rev., 2023, 474, 214852 CrossRef CAS
.
- N. Yoshinari and T. Konno, Multitopic metal-organic carboxylates available as supramolecular building units, Coord. Chem. Rev., 2023, 474, 214850 CrossRef CAS
.
- K. Lv, S. Fichter, M. Gu, J. März and M. Schmidt, An updated status and trends in actinide metal-organic frameworks (An-MOFs): from synthesis to application, Coord. Chem. Rev., 2021, 446, 214011 CrossRef CAS
.
- C. Castillo-Blas, C. Montoro, A. E. Platero-Plats, P. Ares, P. Amo-Ochoa, J. Conesa and F. Zamora, The role of defects in the properties of functional coordination polymers, Adv. Inorg. Chem., 2020, 76, 73–119 CrossRef
.
- E. R. Engel and J. L. Scott, Advances in the green chemistry of coordination polymer materials, Green Chem., 2020, 22, 3693–3715 RSC
.
-
(a) P.-R. Wei, D.-D. Wu, Z. Y. Zhou, S.-L. Li and T. C. W. Mak, Crystal structures of polymeric complexes of zinc(II) bromide and perchlorate with 1,4-diazoniabicyclo[2.2.2]octane-1,4-diacetate, J. Chem. Crystallogr., 1997, 27, 609–615 CrossRef CAS
;
(b) P.-R. Wei, D.-D. Wu, Z. Y. Zhou, S.-L. Li and T. C. W. Mak, Lanthanide coordination polymers with dicarboxylate-like ligands: crystal structures of polymeric neodymium(III) and erbium(III) complexes with flexible double betaines, Polyhedron, 1997, 16, 749–763 CrossRef CAS
.
- O. Toma, N. Mercier, M. Allain, F. Meinardi, A. Forni and C. Botta, Mechanochromic Luminescence of N,N′-Dioxide-4,4′-bipyridine Bismuth Coordination Polymers, Cryst. Growth Des., 2020, 20, 7658–7666 CrossRef CAS
.
- R. Sarma, H. Deka, A. K. Boudalis and J. B. Baruah, Coordination Polymers of Lanthanide(III): Towards Encapsulation of Well-Defined Assembly of Guest Molecules, Cryst. Growth Des., 2011, 11, 547–554 CrossRef CAS
.
- J. Jia, A. J. Blake, N. R. Champness, P. Hubbertstey, C. Wilson and M. Schröder, Multi-Dimensional Transition Metal Coordination Polymers of 4,4′-bipyridine-N,N′ dioxide: 1D Chains and 2D Sheets, Inorg. Chem., 2008, 47, 8652–8664 CrossRef CAS PubMed
.
- R. J. Hill, D.-L. Long, N. R. Champness, P. Hubbertstey and M. Schröder, New Approaches to the Analysis of High Connectivity Materials: Design Frameworks Based upon 44- and 63-Subnet Tectons, Acc. Chem. Res., 2005, 38, 337–350 CrossRef PubMed
.
-
(a) Y.-L. Gai, Q. Guo, X.-Y. Zhao, Y. Chen, S. Liu, Y. Zhang, C.-X. Zhuo, C. Yao and K.-C. Xiong, Extremely stable europium-organic framework for luminescent sensing of Cr2O72− and Fe3+ in aqueous systems, Dalton Trans., 2018, 47, 12051–12055 RSC
;
(b) X. Liu, W. Tang, S. Zhou, F. Wang, Y. Liu, M. Xiong and Q. Pan, Template-directed Synthesis of a Novel Two-dimensional Dy(III) Complex Containing Helical Chains Using [PMo12O40]3−, Chem. J. Chin. Univ., 2016, 37, 1025–1029 CAS
;
(c) X. L. Hao, Y.-Y. Ma, Y.-H. Wang, W.-Z. Zhou and Y.-G. Li, New organic-inorganic hybrid assemblies based on metal-bis(betaine) coordination complexes and Keggin-type polyoxometallates, Inorg. Chem. Commun., 2014, 41, 19–24 CrossRef CAS
;
(d) X. Meng, S.-Y. Song, X.-Z. Song, M. Zhu, S.-N. Zhao, L.-L. Wu and H.-J. Zhang, A Eu/Tb-codoped coordination polymer luminescent thermometer, Inorg. Chem. Front., 2014, 1, 757–760 RSC
;
(e) X.-L. Hao, M.-F. Luo, W. Yao, Y.-G. Li, Y.-H. Wang and E.-B. Wang, Polyoxometallate-templated lanthanide-organic hybrid layers based on 63-honeycomb-like 2D nets, Dalton Trans., 2011, 40, 5971–5976 RSC
;
(f) X.-L. Hao, M.-F. Luo, X. Wang, X.-J. Feng, Y.-G. Li, Y.-H. Wang and E.-B. Wang, A new organic-inorganic hybrid compound based on lanthanide-organic chain and Keggin-type polyoxometallate, Inorg. Chem. Commun., 2011, 14, 1698–1702 CrossRef CAS
.
- E. C. Constable, Expanded ligands – An assembly principle for supramolecular chemistry, Coord. Chem. Rev., 2008, 252, 842–855 CrossRef CAS
.
- G. Kumar and R. Gupta, Molecularly designed architectures – the metalloligand way, Chem. Soc. Rev., 2013, 42, 9403–9453 RSC
.
- P. S. Donnelly, J. M. Harrowfield, B. W. Skelton and A. H. White, Chirality in coordination polymers: Homo- vs, hetero-chiral strand construction, J. Chem. Soc., Dalton Trans., 2001, 3078–3083 RSC
.
-
(a) P. Thuéry and J. Harrowfield, Uranyl ion complexes with 2,2′:6′,2′′-terpyridine-4′-carboxylate. Interpenetration of networks involving ‘expanded ligands’, CrystEngComm, 2021, 23, 7305–7313 RSC
;
(b) P. Thuéry and J. Harrowfield, Varying Structure-Directing Anions in Uranyl Ion Complexes with Ni(2,2′:6′,2′′-terpyridine-4′-carboxylate)2, Eur. J. Inorg. Chem., 2022, 02200011 Search PubMed
.
- X. Kong, K. Hu, L. Mei, Q. Wu, Z. Huang, K. Liu, Z. Chai, C. Nie and W. Shi, Construction of Hybrid Bimetallic Uranyl Compounds Based on a Preassembled Terpyridine Metalloligand, Chem. – Eur. J., 2021, 27, 2124–2130 CrossRef CAS PubMed
.
- Z. Bai, Y. Wang, Y. Li, W. Liu, L. Chen, D. Sheng, J. Diwu, Z. Chai, T. E. Albrecht-Schmitt and S. Wang, First Cationic Uranyl–Organic Framework with Anion-Exchange Capabilities, Inorg. Chem., 2016, 55, 6358–6360 CrossRef CAS PubMed
.
- D.-L. Long, A. J. Blake, N. R. Champness and M. Schröder, Lanthanide coordination frameworks of 4,4′-bipyridine-N,N′-dioxide, Chem. Commun., 2000, 1369–1370 RSC
.
- R. J. Hill, D.-L. Long, M. S. Turvey, A. J. Blake, N. R. Champness, P. Hubbertstey, C. Wilson and M. Schröder, Unprecedented bilayer topologies in 5- and 6-connected framework polymers, Chem. Commun., 2004, 1792–1793 RSC
.
- A. D. Jana, S. C. Manna, G. M. Rosair, M. G. B. Drew, G. Mostafa and N. R. Chauduri, 4,4′-Dipyridyl-N,N′-dioxide Complexes of Metal-Thiocyanate/Selenocyanate: π-Stacked Molecular Rods as Three-Dimensional Support
for Two-Dimensional Polymeric Sheets and Intra/Interchain S⋯S Interaction Dependent Architecture of the R22(8) Synthon Driven Assembly of One-Dimensional Polymeric Chains, Cryst. Growth Des., 2007, 7, 1365–1372 CrossRef CAS
.
- S.-L. Ma, W.-X. Zhu, G.-H. Huang, D.-Q. Yuan and X. Yan, Novel three-dimensional network of lanthanum(III) complex {[La2(BTA)6(4,4′-bpdo)1.5]·1.5H2O}n, J. Mol. Struct., 2003, 646, 89–94 CrossRef CAS
.
- A. L. Spek, Structure validation in chemical crystallography, Acta Crystallogr., Sect. D: Biol. Crystallogr., 2009, 65, 148–155 CrossRef CAS PubMed
.
- M. Leroux, N. Mercier, M. Allain, M.-C. Dul, J. Dittmer, A. H. Kassiba, P. Bellat, G. Weber and I. Bezverkhyy, Porous Coordination Polymer Based on Bipyridinium Linkers with High and Reversible Ammonia Uptake, Inorg. Chem., 2016, 55, 8587–8594 CrossRef CAS PubMed
.
- Y.-Q. Wang, Q.-X. Jia, K. Wang, A.-L. Cheng and E.-Q. Gao, Diverse Manganese(II) Coordination Polymers with Mixed Azide and Zwitterionic Carboxylate Ligands: Structure and Magnetic Properties, Inorg. Chem., 2010, 49, 1551–1560 CrossRef CAS PubMed
.
- M. Pan, B.-B. Du, Y.-X. Zhu, M.-Q. Yue, Z.-W. Wei and C.-Y. Su, Highly Efficient Visible-to-NIR Luminescence of Lanthanide(III) Complexes with Zwitterionic Ligands Bearing Charge-Transfer Character: Beyond Triplet Sensitisation, Chem. – Eur. J., 2016, 22, 2440–2451 CrossRef CAS PubMed
.
- S. Kerisit and C. Liu, Structure, Kinetics and Thermodynamics of the Aqueous Uranyl(VI) Cation, J. Phys. Chem. A, 2013, 117, 6421–6432 CrossRef CAS PubMed
.
- P. Thuéry and J. Harrowfield, Varying Structure-Directing Anions in Uranyl Ion Complexes with Ni(2,2′:6′,2′′-terpyridine-4′-carboxylate)2, Eur. J. Inorg. Chem., 2022, 02200011 Search PubMed
.
- K.-X. Wang and J.-S. Chen, Extended Structures and Physicochemical Studies of Uranyl-Organic Compounds, Acc. Chem. Res., 2011, 44, 531–540 CrossRef CAS PubMed
.
- M. B. Andrews and C. L. Cahill, Uranyl Bearing Hybrid Materials: Synthesis, Speciation and Solid-State Structures, Chem. Rev., 2013, 113, 1121–1136 CrossRef CAS PubMed
.
- M. Viciano-Chumillas, X. Liu, A. Leyva-Pérez, D. Armentano, J. Ferrando-Soria and E. Pardo, Mixed Component Metal-Organic Frameworks: Heterogeneity and Complexity at the Service of Application Performances, Coord. Chem. Rev., 2022, 451, 214273 CrossRef CAS
.
- H. D. Burrows and T. J. Kemp, The Photochemistry of the Uranyl Ion, Chem. Soc. Rev., 1974, 3, 139–165 RSC
.
- S. Chorazy, J. J. Zakrzewski, M. Reczynski and B. Sieklucka, Multi-colour uranyl emission efficiently tuned by hexacyanidometallates within hybrid coordination frameworks, Chem. Commun., 2019, 55, 3057–3060 RSC
.
- X. Zhang, P. Li, M. Krzyaniak, J. Knapp, M. R. Wasielewski and O. K. Farha, Stabilization of Photocatalytically Active Uranyl Species in a Uranyl–Organic Framework for Heterogeneous Alkane Fluorination Driven by Visible Light, Inorg. Chem., 2020, 59, 16795–16798 CrossRef CAS PubMed
.
- X. Tong, S. Wang, H.-X. Gao, Y. Ge, J. Zuo, F. Liu, J. Ding and J. Xiong, Hydrothermal synthesis of two 2D uranyl coordination polymers: structure, luminescence, and photocatalytic degradation of rhodamine B, CrystEngComm, 2020, 22, 5716–5722 RSC
, and references therein.
- S. Kusumoto, Y. Atoini, S. Masuda, J. Y. Kim, S. Hayami, Y. Kim, J. Harrowfield and P. Thuéry, Zwitterionic and Anionic Polycarboxylates as Coligands in Uranyl Ion Complexes, and their Influence on Periodicity and Topology, Inorg. Chem., 2022, 61, 15182–15203 CrossRef CAS PubMed
.
- S. Kusumoto, Y. Atoini, S. Masuda, J. Y. Kim, S. Hayami, Y. Kim, J. Harrowfield and P. Thuéry, Varied role of organic carboxylate dizwitterions and anionic donors in mixed-ligand uranyl ion coordination polymers, CrystEngComm, 2022, 24, 7833–7844 RSC
.
- S. Kusumoto, Y. Atoini, S. Masuda, J. Y. Kim, S. Hayami, Y. Kim, J. Harrowfield and P. Thuéry, Flexible Aliphatic Diammonioacetates as Zwitterionic Ligands for UO22+: Complexes with Various Topologies and Interpenetrated Structures, Inorg. Chem., 2023, 62, 3929–3946 CrossRef CAS PubMed
.
- S. Kusumoto, Y. Atoini, S. Masuda, Y. Koide, K. Chainok, Y. Kim, J. Harrowfield and P. Thuéry, Woven, Polycatenated, or Cage Structures: Effect of Modulation of Ligand Curvature in Heteroleptic Uranyl Ion Complexes, Inorg. Chem., 2023, 62, 7803–7813 CrossRef CAS PubMed
.
- J. Harrowfield and P. Thuéry, Uranyl ion complexes of polycarboxylates – steps towards isolated photoactive cavities, Chemistry, 2020, 2, 63–79 CrossRef CAS
.
|
This journal is © The Royal Society of Chemistry 2023 |
Click here to see how this site uses Cookies. View our privacy policy here.