DOI:
10.1039/D4RA04658G
(Review Article)
RSC Adv., 2024,
14, 32073-32100
Constructed wetlands combined with microbial fuel cells (CW-MFCs) as a sustainable technology for leachate treatment and power generation
Received
26th June 2024
, Accepted 12th August 2024
First published on 11th October 2024
Abstract
The physical and chemical treatment processes of leachate are not only costly but can also possibly produce harmful by products. Constructed wetlands (CW) has been considered a promising alternative technology for leachate treatment due to less demand for energy, economic, ecological benefits, and simplicity of operations. Various trends and approaches for the application of CW for leachate treatment have been discussed in this review along with offering an informatics peek of the recent innovative developments in CW technology and its perspectives. In addition, coupling CW with microbial fuel cells (MFCs) has proven to produce renewable energy (electricity) while treating contaminants in leachate wastewaters (CW-MFC). The combination of CW-MFC is a promising bio electrochemical that plays symbiotic among plant microorganisms in the rhizosphere of an aquatic plant that convert sun electricity is transformed into bioelectricity with the aid of using the formation of radical secretions, as endogenous substrates, and microbial activity. Several researchers study and try to find out the application of CW-MFC for leachate treatment, along with this system and performance. Several key elements for the advancement of CW-MFC technology such as bioelectricity, reactor configurations, plant species, and electrode materials, has been comprehensively discussed and future research directions were suggested for further improving the performance. Overall, CW-MFC may offer an eco-friendly approach to protecting the aquatic environment and come with built-in advantages for visual appeal and animal habitats using natural materials such as gravel, soil, electroactive bacteria, and plants under controlled condition.
1 Introduction
To dispose solid waste, most developing nations adopt an early stage of landfilling in the form of open dumping.1 Concerns in landfill design and operation include landfill leachate (LL), emission gas, slope stability, and odor management.2 Open dumps are places where there is no environmental protection or oversight. Designed landfills are distinguished by correct site selection and design, trash compaction, applying daily cover and leachate, and gaseous and odour systems. Designed landfills include onsite leachate treatment and a post-closure management plan.3 Pollutants in leachate are classified into four types: dissolved organic matter (organic carbon and fatty acids), inorganic materials (chlorides, ammonium, phosphorous, and nitrates), toxic metals (copper, zinc, lead, and mercury), and xenobiotic organic compounds (XOCs) (benzene, phenols, and phthalates).4,5 Raw LL has a high concentration of pollutants.6,7 If not collected and treated, it has a great potential to pollute surrounding soil and groundwater.8,9
Leachate from various sources, such as municipal waste, industrial waste, industrial landfills, and solid inert residue landfills, presents unique challenges due to their differing compositions and pollutant concentrations. Recent advancements in landfill leachate treatment technologies have provided several methods, each with distinct advantages and disadvantages. Traditional methods, while effective, often fall short in terms of environmental sustainability and economic feasibility. Among the various processes to treat LL, constructed wetlands (CWs) have proven effective and economical. Constructed wetland (CW) systems utilize vegetation that supports water purification processes for treatment.10 This technology is low-cost and uses a combination of plants, microorganisms, and soil/media to treat pollutants.11,12 CWs provide symbiotic physical processes, including filtration; physicochemical adsorption; ion exchange; chemical decomposition; precipitation; and microbial reactions such as biodegradation, ammonification, nitrification, and denitrification.13 Previous studies have shown that various inorganic and organic contaminants are removed through these mechanisms in CWs.14 CWs are advantageous over other methods based on environmental attributes, such as their ability to integrate into natural landscapes and provide additional ecosystem services.
However, the direct treatment of leachate using CW is applicable but challenging.15 Single-process treatments often struggle due to high pollutant concentrations that inhibit the process.16 CWs can produce a favorable chemical composition to enhance pollutant degradation.17–19 The performance of CWs depends on factors such as design, plants, media, hydraulic retention time, weather, and more.20–22 Combining microbial fuel cells (MFC) with CWs (CW-MFC) is a relatively new approach for treating pollutants and generating electricity.23,24
Integrated CW technologies, such as CW-MFC systems, offer a sustainable solution for leachate treatment by leveraging the synergistic effects of CWs and MFCs. CW-MFC systems combine the biological processes of CWs with the electrochemical processes of MFCs, enhancing pollutant degradation and electricity generation. This integration allows for the treatment of complex leachates while producing renewable energy. The design parameters, such as the choice of plants, electrode materials, and hydraulic configurations, significantly influence the performance of CW-MFC systems.
Electrochemical technology, which uses reduction and oxidation processes, has also shown promising results for leachate treatment.25 W-MFC treatment has been researched as a promising technology for treating diverse wastewater and producing electricity. Pollutants in multiple wastewaters can be reduced through the combination of plants, bacteria, fillers, and electrochemical redox processes in CW-MFC systems. Microorganisms in CW-MFC systems degrade organic pollutants to generate electrons and protons, occasionally converting the chemical energy of organic contaminants into electrical energy.26 A CW-MFC includes electrodes (anode and cathode) separated by gravel, sand, and soil (media CW), proton exchange membrane (PEM), and fibrous materials.27 CW-MFCs operate under both anaerobic and aerobic conditions, facilitating redox transformations.28,29 Aerobic conditions form near the CW-MFC surface (cathode location), while anaerobic conditions occur at the reactor bottom (anode location).30–32 Previous research on treating municipal sewage using CW-MFCs showed significant reductions in nitrogen, organic matter, and phosphorus.33,34 Studies using synthetic wastewater reported organic and nitrogen removal percentages of 73–98% and 50–85%, respectively, similar to leachate contents.35,36 CW-MFCs can eliminate biological oxygen demand, chemical oxygen demand, ammonium nitrogen, total nitrogen, and phosphorus, with removal percentages ranging from 35 to 76%, 22 to 76%, 10 to 90%, 0.2 to 71%, and 38 to 92%, respectively. Voltage production varied between 4 and 152 mV, with the highest power density between 50 and 527 mW m−3.37
Recent years have seen increased attention to leachate treatment, a complex and environmentally significant wastewater stream. Various treatment methods have been explored, focusing on innovative and sustainable approaches. This review differentiates and highlights the unique perspective provided by the literature on leachate treatment in CW-MFC systems. While numerous studies have examined leachate treatment methods and CW-MFC for wastewater treatment, the distinctiveness of the CW-MFC approach lies in its integration of biological processes within a CW framework, coupled with the harnessing of MFC technology. This research sets the stage for a comprehensive exploration of how CW-MFC compares to other studies, offering a nuanced understanding of its design, electricity production, advantages, and future potential as a promising solution for leachate treatment.
New pollution characteristics of leachate due to technological advancements and household applications can include hazardous compounds, insecticides, heavy metals, and persistent organic pollutants.38,39 Review papers have discussed leachate treatment and electrode materials,40,41 bioenergy generation mechanisms,42,43 and comparisons between CW and CW-MFC.44 To fill the gap, this review focuses on leachate pollutants, design, electrode materials, removal efficiencies, plant types, CW-MFC mechanisms, future directions, and challenges. Compared to other reviews, this research will provide a comprehensive report on these aspects.
2 Source of landfill leachate and characteristics
Leachate is the term for the liquid that comes from the landfill and contacts the waste pile to dissolve the dissolved material.45 Depending on the type of waste in different regions of the landfill, it will produce different leachate qualities. LL is characterized as either young (<5 years), mature (5–10 years), and old (>10 years).46–48 A high BOD/COD ratio (greater than 0.5) in leachate produced in young landfills is an indicator of leachate biodegradability. Amino acids are found in young LLs because they are released when organic molecules break down. Leachate from old landfills is rich in ammoniacal nitrogen due to hydrolysis and fermentation of the nitrogen fraction of biodegradable substrates. Changes in organic matter and ammoniacal nitrogen over time can significantly impact leachate treatment. Regardless of the age of the landfill, leachate consistently contains a variety of life-threatening and ecologically damaging toxins.49 Waste sources can be divided into three categories: solid inert residue (SIR), industrial solid waste (ISW), and municipal solid waste (MSW). Over the past year, the average MSW generation rate per capita in Qatar has reached 1.5 kg per person per day.50
2.1 Municipal waste leachate
Municipal solid waste (MSW) is defined as debris, solid refuse, and rubbish that is produced within the boundaries of a district and a municipality, regardless of where it is generated.45 The population has the greatest impact on the volume of MSW.51 Since each resident in each area will be unique, non-resident areas like institutional or business districts and resident regions can be distinguished from one another.52 The research on MSW landfill leachate has shown that it is a major source of worry for new and developing compounds. Perfluorosurfactants (PF), such as perfluoro octane sulfonate (PFOS, C8F17SO3H) and perfluorooctanoic acid, are another new class of developing surfactants (PFOA, C7F15COOH).53 They have attracted interest because of their distinct chemistry (possible water and oil repellent and surface tension lowering), persistence, bioaccumulation, and hazardous consequences. These are also the most common anionic PF surfactants and organophosphates found in the environment.54 An early investigation found sulphonamide antibiotics in groundwater near an MSW in Denmark. Sulphonamide concentrations in LL reached 18 mg L−1, which is substantially higher than is typically found in raw urban and agricultural wastewater sources.55
Due to geographic location, climate conditions, food habits, and cultural and religious events, the content of the MSW may change seasonally.56 The physical classification of waste organic matter and aggregates includes paper and plastic. The processing capability of biological treatment facilities is decreased when aggregate components are mixed with recyclable resources. The granulometry of aggregate material, which ranges from the smallest to the largest, makes separation from the waste challenging and increases processing costs for material recycling plants. For the capping and filling process in landfills, sewage sludge, dust, and sand must be present and assembled separately. The MSW's moisture content ranged from 28% to 35%. Data on moisture content provide insight into pre-treatment technologies being developed for efficient waste treatment. Between 39% and 43% of solids were observed to be volatile.56 Reduce, reuse, recycle, recover, and then landfill disposal are the principles used to handle MSW.57,58 Unsafe waste management practices can lead to environmental issues, one of which is water pollution from leachate.1,59
Due to the significant amounts of organic matter (such as carboxylic acids and dissolved solids), toxic chemicals, inorganic salts, heavy metals, ammonia, minerals, and xenobiotic organic compounds in MSW leachate from city solid waste treatment facilities, landfills, anaerobic stoves, or compost piles poses serious environmental problems that require attention.60,61 The organic part of landfill filtrate is dominated by flame retardant or non-biodegradable chemicals, such as humic substances like humic acids.62 High amounts of these environmental contaminants are found in the filtrate derived from personal care products and home chemicals. Because it may leak into groundwater and produce biomagnification, LL is dangerous and characterized by both acute and chronic toxicity.63 Leachate from landfills frequently seeps into the soil, which is an issue for landfills worldwide. There are landfills and semi-controlled landfills, and if they are open, they might leak into low-lying coastal regions and contaminate the leachate flowing water. MSW seeps into groundwater or mixes with surface waters as a result of percolation, which can also be brought on by heavy rain and melting permafrost in polar regions.58 The concentration of leachate MSW is shown in Table 1.64,65
Table 1 MSW leachate concentration
Parameter |
Concentration (mg L−1) |
pH |
6.08–8.38 |
Color |
61 |
SS |
22.3 |
Chloride |
455 |
Ammonium |
28.6–2380 |
TN |
57.1 |
TP |
6.3 |
COD |
1075–23 680 |
Oil |
0.44 |
CrVI+ |
0.025 |
Hg |
0.00004 |
Cd |
0.001 |
BOD |
350–11 300 |
2.2 Industrial landfill leachate (ISW)
Another category is ISW type, which includes all facilities that generate such solid waste, regardless of size and location, and are generated by an industrial-type facility.45 Pollution brought on by companies is a serious worry across the world, and of all industrial sectors, the food business has the most influence because of excessive waste output rates per unit of production.66 Recent reviews67–76 and scientific studies77–79 have reported on phytochemical/bioactive substances produced by the processing of product wastes. The groups of organic compounds that are most prevalent in this waste are carbohydrates (pectin and oligosaccharides, starch, cellulose, dietary fibres, monosaccharides, etc.), bisphenols (lignin, phenolic acids, flavonoids, tannins, ellagitannins, etc.), proteins, lipids, essential oils (e.g., terpenoids, hydrocarbons, alcohols and aliphatics). An average of 10% more linked disposal of industrial solid waste, such as textile, dairy, and others, has greatly increased.80 The amount of these compounds in this ISW varies from a few mg to a few grams per kilogram of waste, and their commercial value varies from several euros to several thousand euros per kg of finished product, depending on the compound and the final purity after recovery.81
There is a significant number of diverse types of garbage in landfills, where complicated physical, chemical, and metabolic processes controlled by environmental circumstances result in plastic fragmentation into microplastics.82 Landfill leachate can also be a source of dangerous chemical compounds such as bisphenol A (BPA). Plasticizers are a very important group of LL aromatic pollutants, as plastics make up a large part of waste emissions. BPA is a plastic component also found in thermal paper and most epoxies.83 According to prior research, BPA might be released from various plastic items via diffusion, hydrolysis, and decomposition, depending on their physicochemical qualities84. van Praagh et al.85 collected data on the amounts of microplastics in leachate from 11 landfills in Finland, Norway, and Ireland. The primary aims of this study were to examine the effect of different treatment procedures on microplastic removal efficiency and to identify probable sources of emission. Previous studies of LL pollutants have emphasized toxic organic chemicals such as BTEX (benzene, toluene, ethylbenzene and xylene, chlorinated and aromatic hydrocarbons, etc.)86. He et al.87 investigated the presence of microplastics in 12 leachate samples collected from two closed and four active landfill sites in China. According to ref. 88, BPA leaks from goods that have been disposed of in landfills.89 investigated 10 landfill sites in Japan and discovered BPA values ranging from 1.3 to 17
200 μg L−1. In one study, BPA was commonly detected in LL at concentrations up to 6–17 mg L−1, with a mean concentration of 45.4 μg L−1.90,91
Previous research92 has shown that developing microcontaminants, such as nonylphenol, phthalate acids, and BPA, can leak from plastic materials during weathering or aging of microplastic particles. These chemicals are classified as endocrine disruptors, and as such, they have a harmful influence on water quality. These chemicals are classified as endocrine disruptors, and as such, they have a harmful influence on water quality. They can interact with other contaminants in aqueous medium due to their hydrophobic nature and large surface area.93 BPA is a plasticizer that, due to its unique physicochemical qualities, is employed as a raw ingredient in the creation of various industrial and consumer products. BPA is one of the compounds that is widely used around the world.94
Some countries produce hundreds of millions of tons of different industrial trash each year as developing nations with strong economic growth95 and dispose of it in landfills. The leachate concentration of ISW in Northern Portugal, which collects a lot of trash from industrial processes, including sludge, ash, fibre cement, textile waste, plastic waste, metal, biodegradable waste, paper, cardboard, and wood from municipal wastewater treatment plants, is shown in Table 2.90,96,97
Table 2 ISW leachate concentration
Parameter |
Concentration (mg L−1) |
pH |
8.2 |
Color |
3800 |
SS |
219 |
Chloride |
1247 |
Ammonium |
834 |
TN |
1160 |
TP |
4.3 |
COD |
2667 |
Oil |
— |
CrVI+ |
0.2 |
Hg |
— |
Cd |
<0.05 |
BOD |
245 |
Bisphenol A (BPA) |
17 |
2.3 Solid inert residue landfill leachate
The distribution of SIR in the regions varies noticeably, and these variations are directly tied to the regional industrial structures. Geographically, Eastern China is the main generator of SIR from industry. A SIR released 32
292 million tons of garbage in 2017, or 46.7% of the overall production in China. The central/southwest portion of the area came in second with 16.2% and 13.1%, respectively. Regarding treatment capacity, cities' ability to treat SIR and disassemble big and medium amounts of e-waste greatly outpaced the quantity of treatment.80 Before being dumped in a landfill, SIR, such as bottom ash, is frequently separated from ferrous particles and occasionally from aluminium. In SIR landfills in other nations, bottom and fly ashes are still disposed of together. Almost all organic materials oxidize during combustion. Fly ash has a significantly larger leachable quantity of heavy metals than bottom ash. Several inorganic components lose their chemical connections during combustion due to the high temperatures.98
General SIR is actually a potential complex mixed resource comprising non-metal oxides, metal oxides, carbonates, and other materials.99–101 SiO2, Al2O3, Fe2O3, CaO, MgO, NaO, KO, and organic matter make up the majority of the composition's chemicals. Chemical composition, crystal structure, breakdown characteristics, particle size distribution, and mineral manufacturing methods all have a significant role in the physical and chemical features of waste. Industrial solid waste with a SiO2 component, such as sediments, carbon deposits, fly ash, and red soil, is often referred to as SIR.102,103 In order to prepare various kinds of porous materials to effectively and affordably transform SIR into porous materials, it is required to specify the physical and chemical substance qualities and degree of toxicity of various forms of SIR.104
About 71% of SIR is dumped in landfills around the world.105 Hazardous substances are frequently found in SIR, including certain batteries, paints, mercury-containing garbage, medications, auto care products, and a variety of other items.106,107 The SIR is dumped in landfills that also contain lead and mercury. To prevent these freshwater contaminants from entering groundwater aquifers, a large portion of these poisons must be adequately handled in landfills.108 Groundwater is seriously threatened by the leachate generation and poor management practices associated with unmanaged landfills, particularly open dumps. A polluted liquid called SIR leachate is released from SIR waste.109 Since leachate may infiltrate and contaminate groundwater, it needs to be carefully controlled. From SIR, the samples included 101 of the 190 chemicals analysed, with the levels of chemicals in each final leachate sample ranging from 2 nanograms per litter (ng L−1) to 17
200
000 mg L−1. Toxic items in landfills can contain these chemicals.109
Leachate is collected and treated onsite in contemporary landfills using physical/chemical treatment and biological treatment.53 Nevertheless, because LL treatment was designed primarily to focus on conventional water quality such as COD, BOD, ammonium, and so on,110 treated leachate still included significant amounts of pharmaceuticals and personal care products (PPCP). In Taiwan, for example, the average removal effectiveness of PPCPs in LLs was 15% for diclofenac (DF), gemfibrozil (GF), and carbamazepine (CBZ) over various treatment methods.111,112 About 5000 μg kg−1 of thiabendazole was found in LL together with CBZ. Similarly, CBZ in LL can contaminate groundwater through surface drainage from the point of use.113 The second major source of CBZ contamination is the groundwater–surface interface. This interface acts as an indirect way of mixing surface and groundwater through runoff and down-migration mechanisms.113 A high concentration of CBZ found in LL can easily contaminate groundwater.114,115
Ketoprofen and gemfibrozil are two carboxylic PPCPs that may be removed by activated sludge, although amide PPCPs (such as CBZ and crotamiton) are less effective.116,117 Industrial sludge, sewage treatment plant (ETP) sludge, contaminated drums, contaminated bags, and other hazardous waste produced by more than 2000 industries, including the pesticides and insecticides industry, fertilizer industry, pharmaceutical industry, general sewage treatment plants, special chemicals sector, paints and pigments sector, as well as other industries, are typically disposed of in the SIR landfills.118 Numerous studies have shown that LL is a significant source of developing contaminants, including PPCP waste.119 Leachate characteristics in the SIR landfill in Gujarat are shown in Table 3.90,109,118
Table 3 SIR leachate concentration
Parameter |
Concentration (mg L−1) |
pH |
7.4–7.7 |
Color |
— |
SS |
14 000–16 000 |
Chloride |
13 000–63 000 |
Ammonium |
16 000–22 000 |
TN |
34 000–40 000 |
TP |
30.1–35.8 |
COD |
15 000–35 000 |
Oil |
4.9–6.2 |
CrVI+ |
0.628–0.902 |
Hg |
— |
Cd |
0.318–0.573 |
BOD |
11 000–30 000 |
Erythromycin |
204 ng L−1 |
Glipizide |
155 ng L−1 |
Carbamazepine (CBZ) |
14 867 ng L−1 |
3 Recent advancements in landfill leachate treatment technologies and their advantages and disadvantages
Typically, leachates from MSW, both of which are ISW or SIR, are: (i) recyclable landfill, (ii) collected in on-site and off-site lagoons delivered directly to leachate treatment plant (LTP), (iii) treated biologically (aerobic or anaerobic) or (iv) exposed to conventional physical and chemical processes, e.g. chemical precipitation, coagulation/flocculation, sedimentation/air flotation and coating.
However, these methods have disadvantages, which are: (i) leachate recycling may affect landfill conditions, (ii) transfer of leachate is increasingly questioned and interferes with the proper functioning, and (iii) from a biological and physical and chemical point of view, conventional processes do not allow compliance with European standards of treated wastewater discharge standards.53 Hence, the global scientific community is focused on the development of effective LL treatment solutions.96
3.1 The technologies developed for leachate landfills
Treatment of leachate is often divided into three broad divisions: (1) the physical–chemical process, (2) the biological process, and (3) combination of the biological and physical–chemical processes.120 Coagulation, flocculation, precipitation chemistry, adsorption, filtration membranes, exchange ions, stripping air, advanced oxidation processes (AOPs), and electrochemistry are some of the physicochemical methods used to handle leachate. In order to remove non-biodegradable contaminants from LL, such as heavy metals, PCBs, and AOXs, physical–chemical processing procedures are often used.121,122
3.1.1 Advanced oxidation processes (AOPs). Due to the wide range of applications, the ability to compete using other pollutant degradation technologies, and high mineralization efficiency, AOP has recently become one of the most promising technologies for removing leachate contents. AOP converts emerging contaminants to less complex, non-toxic, and inorganic compounds ions, H2O, and CO2 to powerful oxidants known as free radicals or reactive oxygen materials. Ionizing radiation, ozonation, UV-based oxidation, Fenton and Fenton-like processes, electrochemical methods, ultrasound, photocatalysis, etc. combined techniques are some of the many AOPs strategies. Many detection studies have been conducted on PPCP from aqueous media by ozonation, Fenton, and UV-based oxidation procedures.123 Recently, much attention has been paid to oxidation—removal of PPCP from LLs. To remove PPCP, AOP was used alone or in combination with other chemical or biological methods. Fenton and ozone oxidation removal were investigated. Di(2-ethylhexyl) phthalate (DEHP) and nonylphenol (NP) from LL with autonomy variables such as reaction time 20–90 min, Fe(II) dose of 0.5–2.55 g L−1, H2O2 dose of 5.0–25.5 g L−1, and pH 3–5 for Fenton oxidation and ozonation times of 10–130 min and pH 4–10 during ozone oxidation. NP was destroyed under most operating conditions due to high volatility; but short-circuit NP ethoxylates and NP carboxyethoxylates were the primary intermediates reported in the Fenton assay. During the Fenton oxidation and ozonation processes, DEHP removal efficiency was 90% and 50%. However, by-products of phthalic anhydride, benzoic acid, and pentanoic acid have been reported in ozonation processes.124 Concerns need to be raised about increased radiation doses by increasing the ultrasound power density and increasing the current density required to break down and mineralize PPCP extension. An increase in energy consumption and more expensive additional chemicals directly affect costs associated with AOP. Some of the main issues discussed in AOP are the efficacy of these methods for large-scale treatment of industrial wastewater and the financial potential of production costs related to such scaling-up operations. In rare cases, AOP may also include unidentified products, uncontrollable by-products that may act erratically, and pose a greater risk to human health than the parental bond.125
3.1.2 Adsorption. The adsorption technique is a physicochemical treatment in which liquids are attracted to the surfaces of solid adsorbents to form bonds through physical or chemical bonds.126,127 Preparation of absorbent materials such as AC, biochar, and natural clay minerals requires temperature application. Proper preparation of adsorbents is important to improve the physicochemical properties to effectively adsorb pollutants through electrostatic attraction, complexation, hydrogen bonding, physical adsorption, and hydrophobic interactions.128 Several types of food waste in LL contents have been found to be effective adsorbents.129 The most commonly used adsorbent is activated carbon (AC), which has a relatively high surface area to adsorb pollutants such as heavy metals.130,131 AC is available in two forms: powder and granules. Granules-AC is used when the filtrate contains aromatic compounds and condensed structures. The effectivity in using powder-AC is its ability to remove 90% of COD, 40% of ammonium and 80–90% of heavy metals.132 One study reported high TSS, NH3–N, Zn, and Cu removals of 91%, 99%, 86%, and 100%, respectively, using coconut shell AC. The study showed that the rate of pollutant removal depends on the depth of the layer and the contact time of the adsorbent. Therefore, it is necessary to develop integrated and hybrid treatment processes for the efficient removal of heavy metals.131 For example, leachates that stabilized at 4500–8800 mg L−1 COD due to coagulation pre-treatment (50% COD removal) showed higher COD removal (80%) using AC adsorption.133 Similarly, other studies using banana leaves, sugarcane baguette,130 fish scales,134 and rice husks135 as adsorbents have been shown to be natural adsorbents effective in removing contaminants including COD, boron, NH3–N, phosphates and Fe. Although adsorption treatment has proven to be effective in recent years, high AC costs limit the use of this technology in developing countries.136 Many research studies recently developed mineralized adsorbents that have been shown to be effective in leachate treatment. However, AC sources need attention due to their worldwide availability as a natural mineral, their cost-effectiveness, and their ability to remove large amounts of pollutants in LL.137
3.1.3 Filtration membrane. The membrane filtration technique is based on the principle of selective permeability of ions and molecules through thin film barriers. A selective barrier allows only certain molecules to pass through a composite fluid containing several species of contaminated ions and molecules that release pollutants in bulk liquids.138 Based on the particle size, the membrane filtration methods are divided into nanofiltration (NF), microfiltration (MF), ultrafiltration (UF), and reverse osmosis (RO).139,140 The UF technique is suitable for the separation of organic molecules present in the pre-treated filtrate, which has a relatively low COD of 1560 mg L−1 and BOD of 168 mg L−1 and the removal efficiencies were 46.7% and 22.1%.141 This was followed by an integrated study using the batch system NF and RO process pre-treatment of filtrate of coagulation/flocculation. The COD, TOC and NH4–N removal efficiencies were 28%, 59% and 8%, respectively. Subsequent application of NF and the RO process further improved the removal efficiency of the same pollutants by 95%, 93% and 89%, respectively.142 In the first stage of development, UF membrane filtration was used and has reached a very high removal of 98% TOC and some specific organic compounds, including acetone, methyl ketone, methyl chloride, phenol, toluene, etc., up to 97%.143 The membrane filtration process is quite effective in removing contaminants from the pre-treated filtrate. However, the process faces several challenges in construction and operational requirements, such as the pre-treatment process, membrane fouling and fouling from organics, inorganics, purification of colloidal particles, molecules and heavy metal ions, very high energy consumption due to pressure pumping system, contaminants and the membrane after use, and improper disposal concentrated brine poses a threat to the environment and ecosystem as it contains toxic substances metals, halogenated by-products, antifouling, antifouling, etc.144Treatment of leachate is biologically frequently used for the treatment of leachate with contaminants that have a high level of organic substances; treatment with technology has a lower effective cost, is simple to operate, and can be trusted as treatment of LL by the ratio of BOD/COD were high.145 Microorganisms, such as those involved in anaerobic digestion (AD), anaerobic filter (AF), up-flow anaerobic sludge ballet (UASB), and anaerobic ammonium oxidation (AAO), decompose organic molecules into biogas under anaerobic circumstances (anammox).58
Biological processing can be either aerobic or anaerobic, depending on the availability of oxygen.146 Aerated ponds, activated sludge aerobics, sequencing batch reactors (SBR), rotating biological contactors (RBC), moving bed biofilm reactors (MBBR), fluidized-bed biofilm reactors (FBBR), membrane biological reactors (MBR), fungal treatment, and constructed wetland (CW) are examples of environments where microorganisms degrade organic compounds into carbon dioxide and sludge under aerobic conditions.48,147 So many cutting-edge methods are needed to treat leachate while minimizing energy use, sludge creation, and toxin production. These methods also need to recover organic, inorganic, and xenobiotic substances in a safe phase and optimize their positive uses.144 The creation of an aerobic granular reactor (AGR) and constructed wetland microbial fuel cells (CW-MFC) for the treatment of LL is the result of recent improvements in biological processes over physical–chemical processes. These are potential methods for treating leachate because of their small footprint, lower energy need, power energy production, high microbial activity, long-term operational stability with greater elimination of organic pollutants, and tolerance to severe shock load.148,149
3.2 Constructed wetlands are advantageous over the others based on environmental attributes
Constructed wetlands (CWs) have been produced effectively in lab-scale experiments, pilot-scale projects, and full-scale field applications to treat landfill leachate (LL), demonstrating high efficiency in pollutant reduction.150 The concept of using wetlands for wastewater treatment dates back to the earliest periods of Chinese and Egyptian civilizations, where natural wetlands were employed to manage water pollution.151 CWs were first established for digesting various types of wastewater millennia ago, and in recent decades, they have evolved into fully designed systems.
CWs are engineered systems developed and operated to cleanse different forms of leachate, utilizing and optimizing natural environmental processes while meeting operational and maintenance needs for minimal upkeep systems.152 The type of constructed wetland produced is comparable to natural wetlands but involves the use of various media, including stone, sand, and soil, to create a matrix that supports plant growth and facilitates the treatment of leachate.153 A CW is a planned or regulated wastewater treatment system created utilizing natural processes that incorporate plants, media, and microbes.154,155 As the system's plants root, they transfer oxygen into the water, which serves as an energy source and catalyst for various microbial metabolic processes. Microorganisms degrade raw organic waste in the water into simpler substances that plants can then use as nutrients.155 This setup can be a cost-effective solution for wastewater treatment due to its minimal operational and maintenance expenses, as the processes occur naturally.
One of the most noticeable characteristics of CW and what sets them apart from uncultivated land is the presence of flora. The plants in the created wetland have a number of characteristics connected to the processing process, making them a crucial part of the CW's design.156 The most commonly used plants are Typha latifolia, Phragmites australis, Juncus effusus, Iris pseudacorus, Glyceria maxima, Phragmites mauritianus, Cyperus papyrus, Typha angustifolia, Limnocharis flava, Eichhornia crassipes, Cyperus haspan, Acorus calamus, Carex rostrata, Sagittaria latifolia, and Thalia geniculate.157,158 Although local plants have been used in CW for leachate, little research has been done on their utilization.158–160 Because native plants are more adapted to the area, using plants from beyond the CW site might hasten the harm to these plants.158,161,162 According to certain studies, the system-built CW for leachate can reduce NH3–N, NO3–N, NO2–N, and COD by 91.43, 94.19, 98.11, and 88.36% using Canna indica, Phragmites australis, and Cyperus involucratus.163 In another work, the author shows that the wetland plant Scirpus validus has the capacity to cure naproxen and CBZ in hydroponic circumstances. In testing conditions, 98% of naproxen and 74% of CBZ were removed successfully. According to the data, photodegradation and biodegradation contribute to naproxen removal, whereas plant uptake and assimilation contribute to CBZ removal.115
The treatment processes in CWs involve a combination of physical, chemical, and biological mechanisms.
3.2.1 Physical mechanisms. Filtration and sedimentation: particulate matter is removed as water flows through the substrate. Larger particles settle due to gravity (sedimentation), while smaller particles are trapped by the substrate (filtration).154
3.2.2 Chemical mechanisms. Adsorption: pollutants adhere to the surface of substrate particles through physical or chemical bonds; ion exchange: ions in the wastewater exchange with ions on the surface of the substrate particles; chemical precipitation: certain chemicals in the wastewater react to form insoluble compounds, which precipitate out of the water.153
3.2.3 Biological mechanisms. Microbial degradation: microorganisms in the substrate and root zone degrade organic pollutants through aerobic and anaerobic processes; aerobic degradation: occurs in the presence of oxygen, often near the surface or root zone, where oxygen is supplied by plant roots or diffuses from the atmosphere; anaerobic degradation: occurs in the absence of oxygen, typically deeper in the substrate, where conditions are anoxic or anaerobic,154 nitrification and denitrification: nitrification is the aerobic conversion of ammonium to nitrate by nitrifying bacteria. Denitrification is the anaerobic conversion of nitrate to nitrogen gas by denitrifying bacteria, removing nitrogen from the wastewater;152 plant uptake: plants absorb nutrients such as nitrogen and phosphorus for growth, reducing the nutrient load in the water; and rhizofiltration: plants excrete root exudates that can bind and immobilize contaminants, enhancing microbial activity.155CWs leverage these mechanisms to treat wastewater efficiently while being cost-effective and environmentally sustainable. This approach minimizes operational and maintenance costs due to its reliance on natural processes, making CWs a viable solution for wastewater treatment (Fig. 1).152
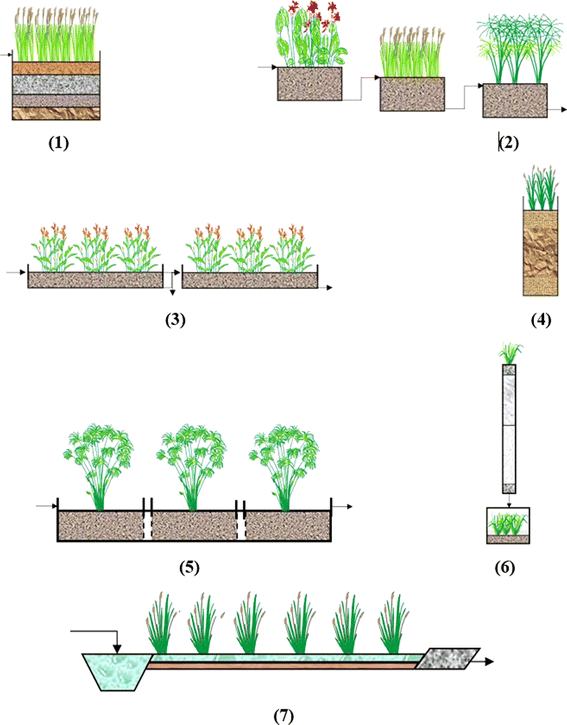 |
| Fig. 1 Design of CWs. | |
CW method is mentioned by164 using Typha angustifolia plant for leachate treatment for 9 days can decrease COD, BOD, TSS, and TKN up to 75.81%, 69.84%, 91.16%, and 25.22%. CW modification using a combination of Typha angustifolia and Cyperus papyrus and bioaugmentation can improve CW performance in leachate treatment for COD, BOD, TSS, TN, Cd, and Hg, which were 80.47%, 84.05%, 80.05%, 75.58%, 99.96%, and 90%, respectively (Table 4).170
Table 4 Comparison of CW leachate treatment data
No. |
Type of CW |
Type of leachate |
Operating condition |
Influent parameter value (mg L−1) |
Effluent parameter value (mg L−1) |
References |
1 |
Baffled-CW combined horizontal (H) and vertical (v) flow (F) Phragmites australis |
SIR |
HRT = 10 days (batch) |
COD = 5163 |
COD = 1332.57 |
165 |
TN = 439.2 |
TN = 119.81 |
TSS = 1994 |
TSS = 711.06 |
Cu2+ = 40.56 |
Cu2+ = 2.312 |
Ni2+ = 42.14 |
Ni2+ = 10.86 |
2 |
Reedbeds-CW Phragmites australis |
SIR |
Intermittent influent flow |
K = 174 |
K = 108 |
166 |
Al = 5.7 |
Al = 1.1 |
Na = 1713 |
Na = 987 |
Cr = 0.01 |
Cr = <0.001 |
Ca = 138 |
Ca = 2.5 |
Li = 0.4 |
Li = 0.2 |
3 |
HSF-CW |
SIR |
Continuous flow (1–55 L h−1) |
Al = 17.27 |
Al = 0.33 |
167 |
Phragmites australis |
V = 0.14 |
V = 0.013 |
4 |
VF-CW |
MSW |
Continuous flow (100 L per day) |
AN = 320.35 |
AN = 9.15 |
168 |
Phragmites australis |
TN = 325.3 |
TN = 55.55 |
|
TP = 17.61 |
TP = 1.91 |
5 |
TVF-CW |
MSW |
Continuous flow (10 L per day) |
TN = 394.12 |
TN = 33.76 |
169 |
Canna indica, Phragmites australis, & Cyperus involucratus |
COD = 1022 |
COD = 119 |
6 |
HSSF-CW |
MSW |
HRT = 6 days (batch) |
COD = 9216 |
COD = 1799.89 |
170 |
Typha angustifolia & Cyperus papyrus |
BOD = 1140 |
BOD = 181.83 |
|
TSS = 120 |
TSS = 23.94 |
|
TN = 70 |
TN = 17.09 |
7 |
HF-CW |
MSW |
HRT = 4 days (batch) |
NH4 = 161 |
NH4 = 74% |
171 |
Heliconia psittacorum |
COD = 691 |
COD = 84.7% |
8 |
VF-CW |
MSW |
Continuous flow (10 L per day) |
COD = 378 |
COD = 226.8 |
172 |
Typha domingensis |
NH4N = 198 |
NH4N = 51.48 |
9 |
HF-CW |
MSW |
Continuous flow (2.25 L per day) |
COD = 660 |
COD = 396 |
173 |
Cyperus papyrus |
NH4N = 142 |
NH4N = 53.96 |
10 |
Combination VF-SF |
MSW |
HRT = 23.3 days (batch) |
COD = 838.5 |
COD = 258.5 |
174 |
BOD = 274.6 |
BOD = 27.3 |
TN = 207.3 |
TN = 22 |
TP = 24.6 |
TP = 0 |
TSS = 432.6 |
TSS = 26 |
11 |
HSF-CW |
ISW |
Continuous flow (60 L h−1) |
Al = ±20 |
Al = ±0 |
175 |
Phragmites australis, Typha latifolia, & Sparganium erectum |
Cr = ±0.0015 |
Cr = n.d. |
|
Ca = ±0.08 |
Ca = ±0.02 |
|
Mg = ±0.013 |
Mg = ±0.008 |
|
Na = ±0.35 |
Na = ±0.25 |
|
V = ±0.8 |
V = ±0.05 |
|
As = ±0.075 |
As = ±0.01 |
12 |
SSF-CW |
ISW |
HRT = 7 days |
COD = 3980 |
COD = 2626.8 |
176 |
Typha angustifolia |
BOD = 3465 |
BOD = 1282.1 |
|
VFAs = 707 |
VFAs = 438.34 |
|
TSS = 32.1 |
TSS = 22.47 |
13 |
VF-CW |
ISW |
Continuous flow (0.008 m3 per m2 per day) |
COD = 9740 |
COD = 1363.6 |
177 |
Phragmites australis |
DOC = 3535 |
DOC = 300 |
|
TSS = 1900 |
TSS = 1560 |
|
TN = 35.2 |
TN = 24.8 |
|
TP = 19 |
TP = 5 |
The hydraulic loading rate is the volume of wastewater applied to the constructed wetland per unit area per unit time.178–180
where,
Q = flow rate of wastewater (m
3 per day).
A = area of the constructed wetland (m
2).
The organic loading rate is the amount of organic matter applied to the constructed wetland per unit area per unit time.
where,
L = flow rate of wastewater (m
3 per day).
C = concentration of organic matter (
e.g., COD or BOD) in the wastewater (mg L
−1).
A = area of the constructed wetland (m
2).
3.2.3.1 Example calculations. (1) Hydraulic loading rate calculationExample data:
Flow rate (Q) = 100 m3 per day |
Calculation:
(2) Organic loading rate calculation
Example data:
Flow rate (L) = 100 m3 per day |
Concentration (C) = 300 mg L−1 (e.g., COD) |
Calculation:
4 Integrated CW technologies for sustainable leachate treatment
The previous research studies had several differences, including the type of processing, type of electrode, type of plant, and HRT, which are shown in Table 5. The numbering in the table provides information for Fig. 2 and 3.
Table 5 Previous research on CW-MFCs
No. |
Treatment type |
Electrodes anode/cathode |
Plant type |
HRT |
Voltage (V) |
Power density |
Current density |
References |
1 |
Synthetic WW |
Graphite/magnesium |
Typha latifolia |
4 days |
0.45–0.99 V |
5.09 mW m2 |
7.11 mA m2 |
181 |
2 |
Synthetic WW |
Graphite/magnesium |
Typha angustifolia |
4 days |
0.79–1.34 V |
181 mW m2 |
33.8 mW m2 |
10 |
3 |
Swine WW |
Stainless-steel wire mesh wrapped with AC/carbon felt |
Canna indica |
2 days |
598–713 mV |
0.456 W m3 |
22.5 mA m2 |
182 |
4 |
Settle sewage |
Granular AC/platinum coated with carbon |
Cyperus prolifer |
2 days |
510 mV |
229 mW m3 |
N/A |
183 |
5 |
Swine WW |
Stainless-steel mesh filled with charcoal/stainless-steel |
Cyperus sp |
2 days |
0.58 V |
56.9 mW m3 |
0.07 Am3 |
184 |
6 |
Urban WW |
Graphite rods/PVC hole filled with graphite sticks |
Cyperus papyrus |
3 days |
137.4 mV |
0.93 mW m2 |
N/A |
185 |
7 |
Synthetic eutrophication influent |
Stainless-steel mesh/carbon felt |
Cyperus alternifolius |
2 days |
125 mV |
6.03 mW m2 |
N/A |
186 |
8 |
Greywater |
Graphite granules/graphite granules |
Phragmites australis |
2 days |
150 mV |
719.57 mW m−3 |
N/A |
187 |
9 |
Boron in wastewater |
Graphite nodes/graphite nodes |
Typha latifolia |
7 days |
1600 mV |
78 mW m−2 |
105 mA m−2 |
188 |
10 |
Leachate |
Aluminium plate/aluminium plate |
Phragmites sp |
24 hours |
45 mV |
527 mW m−2 |
N/A |
189 |
11 |
Leachate |
Metallic aluminium/metallic aluminium |
Phragmites sp |
(N/A) minutes |
Around 25 mV |
3 mW m−2 |
Around 0.25 mA m−3 |
190 |
12 |
Leachate |
Aluminium plate/aluminium plate |
Canna indica |
0.3 days |
39–52 mV |
20 mW m−2 |
0.4–0.5 mA m−2 |
191 |
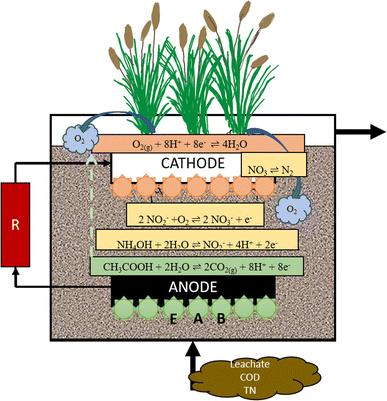 |
| Fig. 2 Mechanism of leachate treatment in CW-MFCs. | |
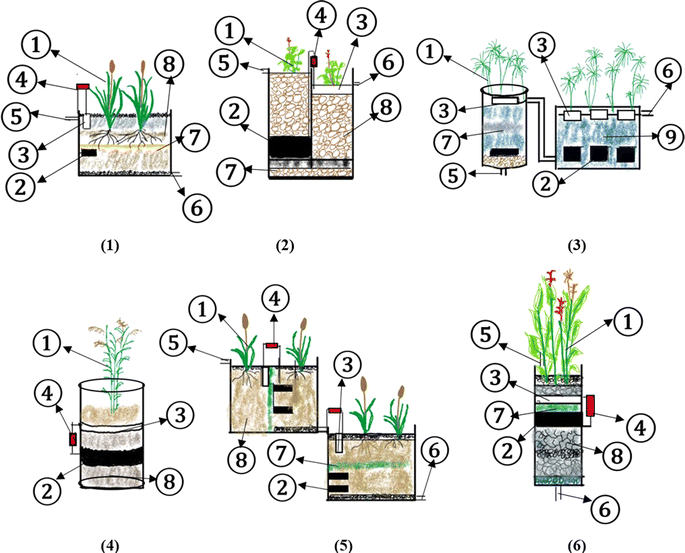 |
| Fig. 3 Reactor design from previous research ((1) plant, (2) anode, (3) cathode, (4) resistor, (5) influent, (6) effluent, (7) separator, (8) media, and (9) water). | |
4.1 CW-MFC synergistic effect and mechanism for leachate treatment
The most common substrates in leachate include ammonia, carbohydrate, and phosphate.146,192–195 The natural process that occurs in the wetland is self-purification.196 In the wetland, there are two kinds of agents that can have a big role: plants and bacteria in soil called rhizobacteria.192,197 The process includes sedimentation, filtration, reduction–oxidation (redox), and plant uptake.198 The rhizobacteria play an essential role in the elimination of pollutants through the redox process.199 Natural purification in the environment can treat water quality with slow processes; therefore, modifications have been performed by many researchers.200 In CW, the rhizobacteria will utilize some acceptors and electron donors from pollutants in the water for their growth.201 The electron acceptors in CW, as time goes by, will be limited due to the anaerobic conditions. The removal performance will reduce because of the unavailable suitable oxygen or electron acceptors. In MFC, an anode is used as an electron acceptor and a cathode for an electron donor.202 The mechanism of leachate treatment in CW-MFCs is shown in Fig. 3.
The mechanism for leachate treatment for organic compounds, nitrogen removal, and heavy metals occurs through several processes, which are physical, chemical, biological, and electrochemical and microorganism synergism processes.203 Pollutants in leachate will be decomposed and oxidized by the anode under anoxic or anaerobic conditions. In the anode, electrons will be generated and moved by an external wire to the cathode. The oxidation–reduction process during leachate treatment is shown in eqn (1) and (2).204
Oxidation that occurs in the anode
|
HOOCOOH(aq) + H2O ⇌ 2CO2(g) + 2H+ + 2e−
| (1) |
Reduction that occurs in the cathode
|
O2(g) + 4H+ + 4e− ⇌ 2H2O
| (2) |
The EAB accepts the electron from the electrode by direct electron transfer via the oxidation of hydrogen and other compounds.205 Then, protons are transferred through diffusion towards the cathode, and the protons and electrons eventually reduce with oxygen secreted from the root plant or NO3–N on electron acceptors, which is the cathode. The chemical energy will be converted into electrical energy in the cathode.206
High voltage and power density in CW-MFC can be reached through several factors; the maximum treatment and electrical efficiency in CW-MFC depends on the organic loading rate (OLR). The average and maximum voltages recorded from previous research reported that the anode region removed organics satisfactorily while the cathode region remained aerobic to carry out oxygen reduction reactions at low OL where a closed-loop HSSF CW-MFC showed satisfactory performance (98–99%) at low volume OLR (0.15 and 0.30 kg COD per m3 per day). However, at high volume OLR (0.52 kg COD per m3 per day), the performance decreased to 95.4%. Additionally, a significant performance improvement of 37.7% was observed in the closed-loop design compared to the open-loop HSSF CW MFC at a high volume OLR of 0.52 kg COD per m3 per day.207
The other factor that increases voltage and power density. Plants play an important role in the CW-MFC system because they release oxygen and release exudates into the rhizosphere through their roots, supporting the biogeochemical cycling of various elements. They provide a large surface area for microbial colonization and influence the microbiota associated with the rhizosphere. The dense and fine root network has a filtration effect that improves the quality of treated water. The difference between each plant from the previous study shows the different electricity production and the best plants need to be considered. The role of evapotranspiration in stress generation was monitored by introducing CW-MFC planted with Phragmites australis at a pilot scale. Larger voltage fluctuations were found to occur during warm days than during cold seasons due to higher evapotranspiration.208
The type of electrode material can influence the high-power density and voltage; microbial electron transfer depends on the biocompatibility of the materials. The structural and functional properties of each material determine microbial compatibility, surface area and porosity, as well as habitat quality.181 Carbon-based materials have excellent biocompatibility and electrical conductivity. Therefore, carbon-based materials such as graphite, carbon cloth or felt, and activated carbon are commonly used as electrodes in CW-MFCs. In addition, the electrode positioning affects the current output of the CW-MFC. For its efficient functioning, anaerobic and aerobic conditions are required in the anodic and cathodic regimes, respectively. Additionally, it is important to maintain a minimum distance between the electrodes to reduce internal resistance.209 reported that the optimal position is to place the cathode 1–2 cm above the water surface.
The quantity of the electrode can increase the electricity and power density production. The use of multiple electrodes in a CW-MFC system is directly related to the increase in the total surface area of the electrodes relative to the total volume of the anode or cathode. Such an arrangement ensures maximum electron transfer to and from the electrode. Placing multiple cathodes at appropriate locations changes the redox potential within the treatment bed and reduces energy loss. The maximum power densities of systems with parallel electrodes and ventilation, recirculation, and both ventilation and recirculation were 1.55 mW m−2, 3.09 mW m−2, and 7.99 mW m−2, respectively. In a previous study, the output of several anodes embedded in CW was investigated by MFC in series and parallel circuits.210
With the increased surface area, decreased charge-transfer resistance, and increased bacterial loading mentioned above. Previous research reported on an H-shaped two-chamber MFC equipped with a hybrid anode made of graphene (G) and a conducting polymer, poly(3,4-ethylenedioxythiophene) (PEDOT), (G/PEDOT) using in situ electro polymerization. Comparing the G/PEDOT hybrid anode to the CP, CP/G, or CP/PEDOT anodes, the former generated more power and had a larger loading of bacteria. The MFCs using the G/PEDOT hybrid anode ought to have a greater output power density than those made using the other three anodes. The results of this work suggest that the G/PEDOT hybrid may make a good anode material for MFCs.211 According to similar findings, GF anode modified with PEDOT shows promise as an anode material for MFC technology, both in terms of performance and scaling up.212 Furthermore, the structure and design of the electrodes have a significant impact on the anode's capabilities. Over planar electrodes made of fabric and plate construction, felt anodes were apparent as potential anodes. The felt structure's shape clearly acknowledged the ability to build biofilms and transport of electrons. Overall, the felt structural structure and PEDOT combined to secure the GF-P as a viable anode for CW-MFC applications.213
Biological processes during leachate treatment in CW-MFCs are biochemical from HRT, plant uptake (the biodegradation of fillers, microorganisms and rhizosphere), and also the conversion process by microorganisms for pollutants on the electrode surface, which was affected by electrode material types.41,204,214 Plants play an essential role in CW-MFC systems because they liberate oxygen and exudates through the root system into the rhizosphere zone and assist in the occurrence of biogeochemical cycling of various elements.196 The plants have been shown to improve bacterial activity to decompose organic compounds.24 The plant has a large specific surface area that can enhance the adsorption of electron-transfer mediators, providing advantages to the CW-MFC systems.11,27 Both electricity generation and water purification rely on the microbial oxidation of inorganic and organic matter in leachate.184 The CW-MFC can significantly improve the efficiency compared to the MFC or a CW.181,207,215,216
4.2 Effect of different design parameters
The topic of CW-MFC has recently been updated. During the past ten years, a lot of research has been done on constructed wetlands (CWs) integrated with bio electrochemical systems (BESs). These systems have been dubbed electro-wetlands, electroactive wetlands, microbial electrochemical technologies-based constructed wetlands, and constructed wetland-microbial fuel cells (CW-MFC).217
From the data collected in the table, the reactor design for each research study is shown in Fig. 3.
From the results of data collection obtained in the table, the concentration of removal for each research is shown in Fig. 4.
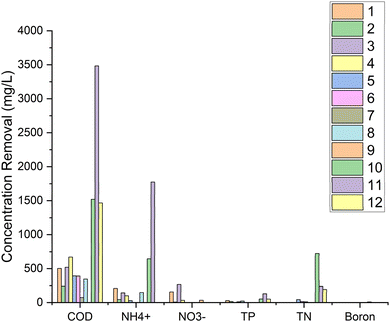 |
| Fig. 4 Removal concentration based on previous research. | |
In the numbering above, No. 1 refers to that in Table 1. Number 1, which was carried out by Yakar et al.,181 (2018), shows synthetic WW processing with anode graphite and cathode using magnesium, plants Typha latifolia for 4 days can produce a voltage of around 0.45–0.99 V, power density 5.09 mW m−2, current density 7.11 mA m−2, with removal efficiencies, COD 90.6%, NH4+ 92.6%, NO3− 81.47%, and TP 96.7%. Other studies have shown different results due to different plant species, HRT, electrode type, CW-MFCs design, and other variables.
The material or conductivity and surface energy of the electrode or conductivity is one of the important factors for the treatment process, stability, price, and bioelectricity generation in CW-MFC.181,196,218–220 The porosity and surface area, habitat quality, microbial affinity and biocompatibility of the material needed for microbial electron transfer are important.181,221 The previous studies have been conducted using granular activated carbon (GAC),183 graphite rod (GR),222 graphite gravel (GG),204,223 graphite plates (GP),224,225 graphite felt (GF),226 carbon fiber felt (CFF),227 carbon fiber brushes (CFB),224 carbon felt (CF),226 activated carbon (AC),228 carbon nanotube (CNT),229 carbon cloth (CC),183 metal nanoparticles,230,231 and graphene oxide,232 as electrode materials.
Metals have a large specific surface area that can increase electrical conductivity and harvest maximum electrons;220 in addition, carbon also has good electrical conductivity and biocompatibility.196 Graphite and carbon are low-cost materials and have strong chemical stability.233 Some researchers have developed and modified the electrode materials.220 The combination of metals modified with carbon materials234 in cathodes has the function of reducing electron acceptors, while anion materials should be resistant to abiotic or biotic degradation because the bacteria should grow on the surface and allow high electron transfer rates.205
Factors that affect pollutant degradation are based on the electrode size and type of electrode in CW-MFCs.220 Nanoporous and microporous materials will make a high surface area suitable for the growth of Electroactive Bacteria (EAB).205 Modification to activate the high surface area of the electrode can be used in pre-treatment.235 The pre-treatment for enhancing the surface area of the electrode is heat,236 adding redox mediators,237 modifications with other materials, such as zeolite clay composites with metals238 or graphene oxide (GO),232 and doping superficial nitrogen groups on the anode surface.235
4.3 Leachate treatment
4.3.1 Nitrogen. The process of nitrogen removal occurs through two major stages, known as nitrification and denitrification. In the nitrification process, aerobic bacteria are used and an electron acceptor is required to convert ammonia into nitrite and nitrate. Then, in the denitrification process, anaerobic bacteria are used and an electron donor is required for the conversion of nitrate into nitrogen gas (N2).239 The rate of the nitrogen process depends on the availability of electron donors that are COD and NH4+ and electron acceptors O2 and NO3–N in CW-MFCs. The majority of researchers reported an improvement in CW-MFCs compared to CWs for the removal of nitrogen, about >10%,28,182,240,241 although a minority of researchers did report a reduction.187,206 In MFCs, the cathode is a nitrifying bacteria zone, and anode zone can increase the community of anaerobic ammonium oxidation (anammox) and denitrifying.206,241–244 The anammox process also occurs, in which ammonia is autotrophically oxidised into N2 when NO3–N under anaerobic conditions is used as the electron acceptor.245 In addition, microbial ammonia oxidation, nitrification, denitrification, adsorption, and plant uptake N occur in CW.192,246 Overall, it can be concluded that CW-MFCs are efficient in terms of nutrient removal; however, the pollutant removal performance highly depends on the design of the system.
4.3.2 Phosphate. Polluted water usually contains 5–20 grams of phosphate and other substances.247 In the natural environment and leachate, P exists in various forms, such as ortho-phosphate (containing one phosphate unit), poly-phosphate, pyro-phosphate, meta-phosphate and their organic complexes.248 Phosphate can be removed by physicochemical and biological processes. Physicochemical (adsorption and electrochemical) processes in CW-MFCs are the most influential processes for phosphate.24,249,250 The media in CW-MFC, such as soil, gravel, and other sediments, will absorb and adsorb phosphorus.251 In previous research conducted using CW-MFC, the phosphorus parameter was reduced to 100%.252 Besides physicochemical processes, the plant plays an essential role in uptaking phosphorus for their growth. The macrophyte uptake of the phosphorus was usually highest during the growing season.251,253 Moreover, phosphate uptake is affected by pH; absorption decreases in acidic and relatively alkaline environments.254
4.3.3 Chemical oxygen demand (COD). Oxygen is used to degrade organic material for electrochemically active bacteria (EAB) activity and growth in CW-MFCs. In MFCs, organic carbon will go to the anode zone and the rest of it will move to the cathode zone with less oxygen.255–257 The COD removal can achieve greater than 95% with 44.6 mW m−2. Other factors that affect the removal of COD are C/N ratio, pH, initial nutrient concentration, chemical species, Hydraulic Retention Time (HRT) and salinity.183,241,258–267 Long HRT will make CW-MFCs to anaerobic conditions that effect on DO concentration and activities of microbial.241,262 Research have conducted that maximum level of HRT is 3 days and will decline more than the days.268
4.3.4 Heavy metals. Heavy metals in waste can also be removed with MFC through a bioelectrochemical process.269 Heavy metal removal occurs from H2O2 electrogeneration at the cathode, which is driven by heavy metal-reducing bacteria.269 Thus, the integrated CW-MFC can increase the removal of heavy metals apart from plant extraction as well as from bioelectrochemical processes. Removal of Zn and Ni in leachate using CW-MFC reached up to 80%, with Iris pseudacorus and water hyacinth plants yielding up to 534.30 mV.270 Some heavy metals in leachate-containing groups have high redox potentials that could be utilized as electron acceptors in order to get precipitated and reduced.271 In general, the anode portion is used to degrade pollutants/organic through biocatalytic oxidation, whereas the cathode is mainly an enclosure for a CW-MFC circuit or a destination for electrons and protons. Compounds with high redox potentials, such as some heavy metals, can serve as cathodic electron acceptors in CW-MFCs.272 High removal of Zn, Pb, and Cr by CW-MFC has been reported, with heavy metal concentrations 5–80 mg L−1 reaching removal efficiency up to 99%.273–276
5 Future perspective
There are many ways to use it to develop and comprehend CW technology, such as identifying root secretions, where they can in fact, serve as electron donors for plant species, enriching the rhizosphere to increase the efficiency of leachate processing, and numerous studies developing it to produce additional benefits, such as bioelectricity.277 In CW-MFCs, the anode and cathode potential still have to be thoroughly investigated for their potential influence. In addition, the fundamental difficulty with CW-MFC is that up until now, all of the research has been done on a laboratory scale. The energy generation, operating parameters, design configuration, electrode material and size, and other scaling-up issues are important, which is why CW-MFC is still being developed on a laboratory scale.217 In the development of MFC-CW, the entire Spanish application was upgraded, even if it is still in its infancy sewage clean-up. There are several issues with the system's design,208,278–280 operation and power output,281,282 electrode materials,283,284 the function of plants,208,285 enhanced biodegradation,286,287 new contaminants,288,289 and biosensing development,290,291 among others. Some review publications, such as those in ref. 292–295, presented an updated evaluation and in-depth study of CW-MFC in a timely way. Theoretically, other avenues of inquiry may focus on both aspects and practical investigations. To be more effective than other leachate treatment techniques, CW-MFC must be scaled up and deployed in practice and on a large scale. Several studies on CW-MFC have yielded a lot of information on the advantages and disadvantages of CW-MFC as a treatment technology, and these have been summarized in Table 6.
Table 6 The advantages and disadvantages of CW-MFCs
No. |
Research application |
Advantages found from the research |
Disadvantages |
Source |
1 |
CW-MFC for WW treatment |
Using activated carbon as the filler can reduce the clogging |
Clogging without filler in CW-MFC |
296 |
2 |
Research about microbial population during MFC start-up |
The addition of acetate conditions the active bacteria so they are expected to become stable |
Voltage instability |
297 |
3 |
Maximizing energy harvesting by adjusting duty cycle value |
Systems using capacitors can sustain energy losses |
High internal resistance can cause high energy loss |
298 |
4 |
CW-MFC to enhance NH4+ removal |
Microbial activity and NH4+ removal can be maximized by increasing the voltage |
Voltage must be applied to increase electron transfer and microbial activity |
299 |
5 |
Effects of different connection modes and cathode conditions in CW-MFC |
COD removal can be eliminated up to around 70% even with low power density |
The highest power density of CW-MFC depends on both the coulombic efficiency and net energy recovery |
300 |
6 |
Effect of electrode material and substrate |
Increasing the substrate concentration enhanced the power density |
Substrate limitation |
301 |
7 |
Relationship electricity performance CW-MFC in non-growing seasons |
In the growing season and suitable weather, the CW-MFC configuration will best |
In low temperatures, the plant and microbial activity will impended |
302 |
The disadvantage of CW-MFC from previous studies can be considered or improved by future studies using CW-MFC for LL treatment. From the limitations and advantages of CW-MFC, in the future, these limitations can be researched or re-examined for handling, and these advantages really make a big contribution to future researchers. Several recommendations have been made to promote the adoption and widespread use of CW-MFC for wastewater treatment and energy generation. Investigating alternative electrode materials and cost-effective fabrication techniques, investigating diverse applications beyond energy generation, conducting comprehensive economic viability and sustainability assessments, improving stability and longevity through electrode modifications, and addressing scalability challenges are among the recommendations. It is also proposed that CW-MFC technologies be integrated with membrane filtering process techniques. Implementing these ideas has the potential to make considerable progress in terms of increasing efficiency, lowering costs, and improving environmental sustainability. Thorough economic viability analyses to compare the costs of CW-MFC technology to those of conventional wastewater treatment methods have to be conducted. Life Cycle evaluation (LCA) and exergy analysis are two sustainability evaluation methodologies that may examine the environmental, economic, and social aspects of CW-MFC systems. LCA studies must be refined and expanded in the future to analyse new CW-MFC designs, materials, and operating conditions for future energy storage applications. Furthermore, the use of energy analysis to evaluate energy conversion efficiency and identify potential for improvement is advised. CW-MFCs can unlock their full potential by bridging the 4Es (Energy, Environment, Efficiency, and Economics) of bioenergy systems and comprehensively assessing their environmental effects, providing more sustainable and efficient solutions for wastewater treatment and other environmental applications.
6 Conclusions
As a result, future researchers and environmental engineers may find it challenging to achieve significant economic sustainability by optimising those factors (type of electrode material, plants, and OLR). An exhaustive effort has been made in the current study to make the CBA model as practical as possible by using raw domestic wastewater instead of synthetic wastewater and carefully analysing local factors, such as labour cost, cost of land, electricity tariff, cost of effluent quality check, and so on. However, as scaling up bioelectrochemical systems imposes a nonlinear response comparable to lab-scale reactors, the investment cost, operation and maintenance cost, and cost of power output may fluctuate throughout the installation and operation of field-scale reactors. The correlation matrix revealed a positive relationship between electricity and pollutant treatment, implying that a reactor with a greater power output will create higher-quality effluent. Future research may focus on additional advantageous uses of treated wastewater following rigorous evaluation of effluent quality criteria in order to earn more money from industrial and commercial reuse of treated wastewater. CW-MFC can be used as a sustainable treatment process for leachate along with electricity generation and environmentally friendly removal of several harmful compounds in wastewater. The power generation in CW-MFC ranges from 25–1600 mV. The removal efficiency of leachate treatment using CW-MFC from the previous study report was 90.6% for COD, 92.6% for ammonia, and 96.7% for TP. So far, mixed aspects of the CW-MFC-related design and process have been investigated on a laboratory scale. For field applications, CW-MFC needs to be further innovatively developed and optimization of technology is required by considering several major limiting parameters such as the PPCPs, plasticizer or BPA, and heavy metals.
Data availability
The necessary data used in the manuscript are already present in the manuscript.
Author contributions
Isni Arliyani: conceptualization, data curation, formal analysis, funding acquisition, investigation, methodology, project administration, resources, software, supervision, validation, visualization, writing – original draft, writing – review & editing. MD Tabish Noori: conceptualization, data curation, formal analysis, funding acquisition, investigation, methodology, project administration, resources, software, supervision, validation, visualization, writing – original draft, writing – review & editing. Muhammad Imam Ammarullah: conceptualization, data curation, formal analysis, funding acquisition, investigation, methodology, project administration, resources, software, supervision, validation, visualization, writing – original draft, writing – review & editing. Bieby Voijant Tangahu: conceptualization, data curation, formal analysis, funding acquisition, investigation, methodology, project administration, resources, software, supervision, validation, visualization, writing – original draft, writing – review & editing. Sarwoko Mangkoedihardjo: conceptualization, data curation, formal analysis, funding acquisition, investigation, methodology, project administration, resources, software, supervision, validation, visualization, writing – original draft, writing – review & editing. Booki Min: conceptualization, data curation, formal analysis, funding acquisition, investigation, methodology, project administration, resources, software, supervision, validation, visualization, writing – original draft, writing – review & editing.
Conflicts of interest
The authors declare no conflict of interest.
Acknowledgements
Authors acknowledge the financial assistance through the research grant of PKPI-PMDSU, 085.14/E4.4/KU/2022 Ministry of Education, Culture, Research, and Technology and the National Research Foundation of Korea (grant agreement numbers: 2018R1A2B6001507 and RS-2023-00208421).
References
- F. A. Osra, H. K. Ozcan, J. S. Alzahrani and M. S. Alsoufi, Municipal solid waste characterization and landfill gas generation in kakia landfill, makkah, Sustainability, 2021, 13(3), 1–13 CrossRef
. - F. Kreith and G. Tchobanoglous, Handbook of Solid Waste Management, 2nd Edition, Environmental Health, 3rd edn, 2019, [cited 2023 Mar 2];215–45, available from: https://books.google.com/books/about/Handbook_of_Solid_Waste_Management.html?hl=ko%26id=y10XiaaXeuYC Search PubMed
. - M. A. Kamaruddin, M. S. Yusoff, L. M. Rui, A. M. Isa, M. H. Zawawi and R. Alrozi, An overview of municipal solid waste management and landfill leachate treatment: Malaysia and Asian perspectives, Environ. Sci. Pollut. Res., 2017, 24(35), 26988–27020 CrossRef PubMed
. - P. Kjeldsen, M. A. Barlaz, A. P. Rooker, A. Baun, A. Ledin and T. H. Christensen, Present and long-term composition of MSW landfill leachate: a review, Crit. Rev. Environ. Sci. Technol., 2002, 32(4), 297–336, DOI:10.1080/10643380290813462
. - M. Kumari and P. Ghosh, Landfill leachate treatment using bacto-algal co-culture: an integrated approach using chemical analyses and toxicological assessment, Ecotoxicol. Environ. Saf., 2016, 128, 44–51 CrossRef PubMed
, https://linkinghub.elsevier.com/retrieve/pii/S0147651316300409. - P. Gautam, S. Kumar, S. Vishwakarma and A. Gautam, Synergistic optimization of electrocoagulation process parameters using response surface methodology for treatment of hazardous waste landfill leachate, Chemosphere, 2022, 290, 133255 CrossRef PubMed
. - Y. Hu, Z. Gu, J. He and Q. Li, Novel strategy for controlling colloidal instability during the flocculation pretreatment of landfill leachate, Chemosphere, 2022, 287, 132051 CrossRef
. - P. Gautam and S. Kumar, Characterisation of Hazardous Waste Landfill Leachate and its Reliance on Landfill Age and Seasonal Variation: A Statistical Approach, J. Environ. Chem. Eng., 2021, 9(4), 105496 CrossRef
. - H. Najafi Saleh, S. Valipoor, A. Zarei, M. Yousefi, F. Baghal Asghari and A. A. Mohammadi, et al., Assessment of groundwater quality around municipal solid waste landfill by using Water Quality Index for groundwater resources and multivariate statistical technique: a case study of the landfill site, Qaem Shahr City, Iran, Environ. Geochem. Health, 2020, 42(5), 1305–1319, DOI:10.1007/s10653-019-00417-0
. - Ç. Saz, C. Türe, O. C. Türker and A. Yakar, Effect of vegetation type on treatment performance and bioelectric production of constructed wetland modules combined with microbial fuel cell (CW-MFC) treating synthetic wastewater, Environ. Sci. Pollut. Res., 2018, 25(9), 8777–8792 CrossRef PubMed
. - S. Gupta, A. Nayak, C. Roy and A. K. Yadav, An algal assisted constructed wetland–microbial fuel cell integrated with sand filter for efficient wastewater treatment and electricity production, Chemosphere, 2021, 263, 128132 CrossRef CAS
. - C. R. Bolton and D. G. Randall, Development of an integrated wetland microbial fuel cell and sand filtration system for greywater treatment, J. Environ. Chem. Eng., 2019, 7(4), 103249 CrossRef CAS
. - H. Wu, J. Zhang, H. H. Ngo, W. Guo, Z. Hu and S. Liang, et al., A review on the sustainability of constructed wetlands for wastewater treatment: Design and operation, Bioresour. Technol., 2015, 175, 594–601 CrossRef CAS PubMed
. - Q. Liu, B. Zhou, S. Zhang, D. Xu, R. Pan and S. Xia, Embedding Microbial Fuel Cells into the Vertical Flow Constructed Wetland Enhanced Denitrogenation and Water Purification, Pol. J. Environ. Stud., 2019, 28(3), 1799–1804 CrossRef
, http://www.pjoes.com/Embedding-Microbial-Fuel-Cells-into-the-Vertical-nFlow-Constructed-Wetland-Enhanced,89982,0,2.html. - T. Saeed, M. J. Miah, N. Majed, M. Hasan and T. Khan, Pollutant removal from landfill leachate employing two-stage constructed wetland mesocosms: co-treatment with municipal sewage, Environ. Sci. Pollut. Res., 2020, 27(22), 28316–28332, DOI:10.1007/s11356-020-09208-y
. - I. F. I. Siti, P. Purwanto and B. Yulianto, The biological treatment method for landfill leachate, E3S Web Conf., 2020, 202, 06006 CrossRef
. - A. Mojiri, L. Ziyang, R. M. Tajuddin, H. Farraji and N. Alifar, Co-treatment of landfill leachate and municipal wastewater using the ZELIAC/zeolite constructed wetland system, J. Environ. Manage., 2016, 166, 124–130 CrossRef PubMed
. - T. Saeed, M. J. Miah, N. Majed, M. K. Alam and T. Khan, Effect of effluent recirculation on nutrients and organics removal performance of hybrid constructed wetlands: Landfill leachate treatment, J. Cleaner Prod., 2021, 282, 125427 CrossRef
. - E. Wojciechowska, Potential and limits of landfill leachate treatment in a multi-stage subsurface flow constructed wetland – Evaluation of organics and nitrogen removal, Bioresour. Technol., 2017, 236, 146–154 CrossRef PubMed
. - H. D. Tran, H. M. T. Vi, H. T. T. Dang and R. M. Narbaitz, Pollutant removal by Canna Generalis in tropical constructed wetlands for domestic wastewater treatment, Global J. Environ. Sci. Manage., 2019, 5(3), 331–344 CAS
, https://www.gjesm.net/article_35321.html. - Z. Tang, J. Wood, D. Smith, A. Thapa and N. Aryal, A Review on Constructed Treatment Wetlands for Removal of Pollutants in the Agricultural Runoff, Sustainability, 2021, 13, 13578 CrossRef
, https://www.mdpi.com/2071-1050/13/24/13578/htm. - Y. Wang, Z. Cai, S. Sheng, F. Pan, F. Chen and J. Fu, Comprehensive evaluation of substrate materials for contaminants removal in constructed wetlands, Sci. Total Environ., 2020, 701, 134736 CrossRef PubMed
. - G. Palanisamy, H. Y. Jung, T. Sadhasivam, M. D. Kurkuri, S. C. Kim and S. H. Roh, A comprehensive review on microbial fuel cell technologies: Processes, utilization, and advanced developments in electrodes and membranes, J. Cleaner Prod., 2019, 221, 598–621 CrossRef
. - N. E. Paucar and C. Sato, Microbial Fuel Cell for Energy Production, Nutrient Removal and Recovery from Wastewater: A Review, Processes, 2021, 9, 1318 CrossRef
, https://www.mdpi.com/2227-9717/9/8/1318/htm. - Y. Deng, X. Zhu, N. Chen, C. Feng, H. Wang and P. Kuang, et al., Review on electrochemical system for landfill leachate treatment: Performance, mechanism, application, shortcoming, and improvement scheme, Sci. Total Environ., 2020, 745, 140768 CrossRef PubMed
. - L. Doherty, Y. Zhao, X. Zhao and W. Wang, Nutrient and organics removal from swine slurry with simultaneous electricity generation in an alum sludge-based constructed wetland incorporating microbial fuel cell technology, Chem. Eng. J., 2015, 266, 74–81 CrossRef
. - W. Wang, Y. Zhang, M. Li, X. Wei, Y. Wang and L. Liu, et al., Operation mechanism of constructed wetland-microbial fuel cells for wastewater treatment and electricity generation: A review, Bioresour. Technol., 2020, 314, 123808 CrossRef CAS
. - P. Srivastava, A. K. Yadav, V. Garaniya, T. Lewis, R. Abbassi and S. J. Khan, Electrode dependent anaerobic ammonium oxidation in microbial fuel cell integrated hybrid constructed wetlands: A new process, Sci. Total Environ., 2020, 698, 134248 CrossRef CAS PubMed
. - A. Prado de Nicolás, R. Berenguer and A. Esteve-Núñez, Evaluating bioelectrochemically-assisted constructed wetland (METland®) for treating wastewater: Analysis of materials, performance and electroactive communities, Chem. Eng. J., 2022, 440, 135748 CrossRef
. - A. Ebrahimi, M. Sivakumar and C. McLauchlan, A taxonomy of design factors in constructed wetland-microbial fuel cell performance: A review, J. Environ. Manage., 2021, 291, 112723 CrossRef
. - H. M. Singh, A. K. Pathak, K. Chopra, V. v. Tyagi, S. Anand and R. Kothari, Microbial fuel cells: a sustainable solution for bioelectricity generation and wastewater treatment, Biofuels, 2018, 10(1), 11–31, DOI:10.1080/1759726920171413860
. - K. A. Dwivedi, S. J. Huang, C. T. Wang and S. Kumar, Fundamental understanding of microbial fuel cell technology: Recent
development and challenges, Chemosphere, 2022, 288, 132446 CrossRef
. - T. Saeed and M. J. Miah, Organic matter and nutrient removal in tidal flow-based microbial fuel cell constructed wetlands: Media and flood-dry period ratio, Chem. Eng. J., 2021, 411, 128507 CrossRef
. - T. Saeed, M. J. Miah and T. Khan, Intensified constructed wetlands for the treatment of municipal wastewater: experimental investigation and kinetic modelling, Environ. Sci. Pollut. Res., 2021, 28(24), 30908–30928, DOI:10.1007/s11356-021-12700-8
. - L. Wang, Q. Pang, Y. Zhou, F. Peng, F. He and W. Li, et al., Robust nitrate removal and bioenergy generation with elucidating functional microorganisms under carbon constraint in a novel multianode tidal constructed wetland coupled with microbial fuel cell, Bioresour. Technol., 2020, 314, 123744 CrossRef
. - L. Wang, Y. Zhou, F. Peng, A. Zhang, Q. Pang and J. Lian, et al., Intensified nitrogen removal in the tidal flow constructed wetland-microbial fuel cell: Insight into evaluation of denitrifying genes, J. Cleaner Prod., 2020, 264, 121580 CrossRef
. - T. Saeed, A. K. Yadav and M. J. Miah, Landfill leachate and municipal wastewater co-treatment in microbial fuel cell integrated unsaturated and partially saturated tidal flow constructed wetlands, J. Water Process Eng., 2022, 46, 102633 CrossRef
. - A. G. Ramu, L. Telmenbayar, J. Theerthagiri, D. Yang, M. Song and D. Choi, Synthesis of a hierarchically structured Fe3O4–PEI nanocomposite for the highly sensitive electrochemical determination of bisphenol A in real samples, New J. Chem., 2020, 44(43), 18633–18645 RSC
, https://rsc.66557.net/en/content/articlehtml/2020/nj/d0nj03830j. - A. Aarthi, M. R. Bindhu, M. Umadevi, R. Parimaladevi, G. v. Sathe and A. M. Al-Mohaimeed, et al., Evaluating the detection efficacy of advanced bimetallic plasmonic nanoparticles for heavy metals, hazardous materials and pesticides of leachate in contaminated groundwater, Environ. Res., 2021, 201, 111590 CrossRef
. - B. Saba, M. Khan, A. D. Christy and B. V. Kjellerup, Microbial phyto-power systems - A sustainable integration of phytoremediation and microbial fuel cells, Bioelectrochemistry, 2019, 127, 1–11 CrossRef
, https://pubmed.ncbi.nlm.nih.gov/30614442/. - O. Guadarrama-Pérez, T. Gutiérrez-Macías, L. García-Sánchez, V. H. Guadarrama-Pérez and E. B. Estrada-Arriaga, Recent advances in constructed wetland-microbial fuel cells for simultaneous bioelectricity production and wastewater treatment: A review, Int. J. Energy Res., 2019, 43(10), 5106–5127, DOI:10.1002/er.4496
. - W. Wang, Y. Zhang, M. Li, X. Wei, Y. Wang and L. Liu, et al., Operation mechanism of constructed wetland-microbial fuel cells for wastewater treatment and electricity generation: A review, Bioresour. Technol., 2020, 314, 123808 CrossRef PubMed
. - P. Srivastava, S. Gupta, V. Garaniya, R. Abbassi and A. K. Yadav, Up to 399 mV bioelectricity generated by a rice paddy-planted microbial fuel cell assisted with a blue-green algal cathode, Environ. Chem. Lett., 2018, 17(2), 1045–1051, DOI:10.1007/s10311-018-00824-2
. - A. Ebrahimi, M. Sivakumar, C. McLauchlan, A. Ansari and A. S. Vishwanathan, A critical review of the symbiotic relationship between constructed wetlandand microbial fuel cell for enhancing pollutant removal and energy generation, J. Environ. Chem. Eng., 2021, 9(1), 105011, DOI:10.1016/j.jece.2020.105011
. - K. S. Randhawa, R. Kumar, H. Alkaabi, M. Q. Alkahtani, S. Islam, C. Prakash, R. Kumar and M. I. Ammarullah, Bioengineering solutions for expansive soil stabilization using waste materials: An experimental evaluation, AIP Adv., 2024, 14, 1–16, DOI:10.1063/5.0210939
. - J. Qiu, F. Lü, H. Zhang, W. Liu, J. Chen and Y. Deng, et al., UPLC Orbitrap MS/MS-based fingerprints of dissolved organic matter in waste leachate driven by waste age, J. Hazard. Mater., 2020, 383, 121205 CrossRef
. - P. Wen, Y. Huang, Z. Qiu and Q. Li, Microbial response during treatment of different types of landfill leachate in a semi-aerobic aged refuse biofilter, Chemosphere, 2021, 262, 127822 CrossRef PubMed
. - L. Lindamulla, N. Nanayakkara, M. Othman, S. Jinadasa, G. Herath and V. Jegatheesan, Municipal Solid Waste Landfill Leachate Characteristics and Their Treatment Options in Tropical Countries, Curr. Pollut. Rep., 2022, 8, 273–287 CrossRef
. - J. Antony, S. v. Niveditha, R. Gandhimathi, S. T. Ramesh and P. v Nidheesh, Stabilized landfill leachate treatment by zero valent aluminium-acid system combined with hydrogen peroxide and persulfate based advanced oxidation process, Waste Manage., 2020, 106, 1–11 CrossRef PubMed
. - J. N. Hahladakis and H. M. S. J. Aljabri, Delineating the plastic waste status in the State of Qatar: Potential opportunities, recovery and recycling routes, Sci. Total Environ., 2019, 653, 294–299 CrossRef
. - O. Buenrostro, G. Bocco and S. Cram, Classification of sources of municipal solid wastes in developing countries, Resour., Conserv. Recycl., 2001, 32(1), 29–41 CrossRef
. - O. O. Osinowo, M. O. Falufosi and E. O. Omiyale, Integrated electromagnetic (EM) and Electrical Resistivity Tomography (ERT) geophysical studies of environmental impact of Awotan dumpsite in Ibadan, southwestern Nigeria, J. Afr. Earth Sci., 2018, 140, 42–51 CrossRef
. - J. Gao, V. Oloibiri, M. Chys, W. Audenaert, B. Decostere and Y. He, et al., The present status of landfill leachate treatment and its development trend from a technological point of view, Rev. Environ. Sci. Bio/Technol., 2015, 14(1), 93–122, DOI:10.1007/s11157-014-9349-z
. - M. Deng, D. T. F. Kuo, Q. Wu, Y. Zhang, X. Liu and S. Liu, et al., Organophosphorus flame retardants and heavy metals in municipal landfill leachate treatment system in Guangzhou, China, Environ. Pollut., 2018, 236, 137–145 CrossRef PubMed
. - A. Ramakrishnan, L. Blaney, J. Kao, R. D. Tyagi, T. C. Zhang and R. Y. Surampalli, Emerging contaminants in landfill leachate and their sustainable management, Environ. Earth Sci., 2015, 73(3), 1357–1368, DOI:10.1007/s12665-014-3489-x
. - V. R. S. Cheela, S. Goel, M. John and B. Dubey, Characterization of municipal solid waste based on seasonal variations, source and socio-economic aspects, Waste Disposal Sustainable Energy, 2021, 3(4), 275–288 CrossRef
. - H. Breukelman, H. Krikke and A. Löhr, Failing Services on Urban Waste Management in Developing Countries: A Review on Symptoms, Diagnoses, and Interventions, Sustainability, 2019, 11, 6977 CrossRef
, https://www.mdpi.com/2071-1050/11/24/6977/htm. - S. Nanda and F. Berruti, Municipal solid waste management and landfilling technologies: a review, Environ. Chem. Lett., 2021, 19, 1433–1456 CrossRef
. - S. Mepaiyeda, K. Madi, O. Gwavava and C. Baiyegunhi, Geological and geophysical assessment of groundwater contamination at the Roundhill landfill site, Berlin, Eastern Cape, South Africa, Heliyon, 2020, 6(7), e04249 CrossRef PubMed
, http://www.cell.com/article/S2405844020310938/fulltext. - H. Pasalari, M. Farzadkia, M. Gholami and M. M. Emamjomeh, Management of landfill leachate in Iran: valorization, characteristics, and environmental approaches, Environ. Chem. Lett., 2019, 17(1), 335–348, DOI:10.1007/s10311-018-0804-x
. - I. A. Tałałaj, P. Biedka and I. Bartkowska, Treatment of landfill leachates with biological pretreatments and reverse osmosis, Environ. Chem. Lett., 2019, 17(3), 1177–1193, DOI:10.1007/s10311-019-00860-6
. - M. Gong, S. Nanda, M. J. Romero, W. Zhu and J. A. Kozinski, Subcritical and supercritical water gasification of humic acid as a model compound of humic substances in sewage sludge, J. Supercrit. Fluids, 2017, 119, 130–138 CrossRef
. - S. Mishra, D. Tiwary, A. Ohri and A. K. Agnihotri, Impact of Municipal Solid Waste
Landfill leachate on groundwater quality in Varanasi, India, Groundwater Sustainable Dev., 2019, 9, 100230 CrossRef
. - X. Song, H. Min, L. Zhao, Q. Fu, W. Zheng and X. Wang, et al., The Experience and Development of the Treatment Technology of Municipal Solid Waste Leachate in China, Water, 2022, 14(16), 2458 CrossRef
, https://www.mdpi.com/2073-4441/14/16/2458. - D. Zeng, G. Chen, P. Zhou, H. Xu, A. Qiong and B. Duo, et al., Factors influencing groundwater contamination near municipal solid waste landfill sites in the Qinghai-Tibetan plateau, Ecotoxicol. Environ. Saf., 2021, 211, 111913 CrossRef PubMed
, https://linkinghub.elsevier.com/retrieve/pii/S0147651321000245. - T. Ahmad, R. M. Aadil, H. Ahmed, U. U. Rahman, B. C. V. Soares and S. L. Q. Souza, et al., Treatment and utilization of dairy industrial waste: A review, Trends Food Sci. Technol., 2019, 88, 361–372 CrossRef CAS
. - H. Kumar, K. Bhardwaj, R. Sharma, E. Nepovimova, K. Kuča and D. S. Dhanjal, et al., Fruit and Vegetable Peels: Utilization of High Value Horticultural Waste in Novel Industrial Applications, Molecules, 2020, 25, 2812 CrossRef PubMed
, https://www.mdpi.com/1420-3049/25/12/2812/htm. - A. Kovalcik, S. Obruca and I. Marova, Valorization of spent coffee grounds: A review, Food Bioprod. Process., 2018, 110, 104–119 CrossRef
. - T. Ilyas, P. Chowdhary, D. Chaurasia, E. Gnansounou, A. Pandey and P. Chaturvedi, Sustainable green processing of grape pomace for the production of value-added products: An overview, Environ. Technol. Innovation, 2021, 23, 101592 CrossRef
. - A. Hejna, Potential applications of by-products from the coffee industry in polymer technology – Current state and perspectives, Waste Manage., 2021, 121, 296–330 CrossRef
. - P. Gullón, B. Gullón, G. Astray, M. Carpena, M. Fraga-Corral and M. A. Prieto, et al., Valorization of by-products from olive oil industry and added-value applications for innovative functional foods, Food Res. Int., 2020, 137, 109683 CrossRef
. - M. Fraga-Corral, P. Otero, J. Echave, P. Garcia-Oliveira, M. Carpena and A. Jarboui, et al., By-Products of Agri-Food Industry as Tannin-Rich Sources: A Review of Tannins' Biological Activities and Their Potential for Valorization, Foods, 2021, 10, 137 CrossRef
, https://www.mdpi.com/2304-8158/10/1/137/htm. - S. Ben-Othman, I. Jõudu, R. Bhat, M. Beatriz, P. Oliveira and R. C. Alves, Bioactives from Agri-Food Wastes: Present Insights and Future Challenges, Molecules, 2020, 25(3), 510 CrossRef CAS
, https://www.mdpi.com/1420-3049/25/3/510/htm. - S. L. Rodríguez García and V. Raghavan, Green extraction techniques from fruit and vegetable waste to obtain bioactive compounds—A review, Crit. Rev. Food Sci. Nutr., 2021, 62(23), 6446–6466, DOI:10.1080/1040839820211901651
. - L. C. Freitas, J. R. Barbosa, A. L. C. da Costa, F. W. F. Bezerra, R. H. H. Pinto and J. R. N. de Carvalho, From waste to sustainable industry: How can agro-industrial wastes help in the development of new products?, Resour., Conserv. Recycl., 2021, 169, 105466 CrossRef
. - M. Carmona-Cabello, I. L. Garcia, D. Leiva-Candia and M. P. Dorado, Valorization of food waste based on its composition through the concept of biorefinery, Curr. Opin. Green Sustainable Chem., 2018, 14, 67–79 CrossRef
. - I. Mármol, J. Quero, R. Ibarz, P. Ferreira-Santos, J. A. Teixeira and C. M. R. Rocha, et al., Valorization of agro-food by-products and their potential therapeutic applications, Food Bioprod. Process., 2021, 128, 247–258 CrossRef
. - P. Abbasi-Parizad, P. de Nisi, B. Scaglia, A. Scarafoni, S. Pilu and F. Adani, Recovery of phenolic compounds from agro-industrial by-products: Evaluating antiradical activities and immunomodulatory properties, Food Bioprod. Process., 2021, 127, 338–348 CrossRef
. - M. Castrica, R. Rebucci, C. Giromini, M. Tretola, D. Cattaneo and A. Baldi, Total phenolic content and antioxidant capacity of agri-food waste and by-products, Ital. J. Anim. Sci., 2018, 18(1), 336–341, DOI:10.1080/1828051X20181529544
. - W. Guo, B. Xi, C. Huang, J. Li, Z. Tang and W. Li, et al., Solid waste management in China: Policy and driving factors in 2004–2019, Resour., Conserv. Recycl., 2021, 173, 105727 CrossRef
. - E. H. Papaioannou, R. Mazzei, F. Bazzarelli, E. Piacentini, V. Giannakopoulos and M. R. Roberts, et al., Agri-Food Industry Waste as Resource of Chemicals: The Role of Membrane Technology in Their Sustainable Recycling, Sustainability, 2022, 14, 1483 CrossRef CAS
, https://www.mdpi.com/2071-1050/14/3/1483. - R. Pramila, Biodegradation of low density polyethylene (LDPE) by fungi isolated from marine water a SEM analysis, Afr. J. Microbiol. Res., 2011, 5(28), 5013–5018 Search PubMed
, https://www.academia.edu/download/86904424/296918417039.pdf. - J. R. Rochester and A. L. Bolden, Bisphenol S and F: A systematic review and comparison of the hormonal activity of bisphenol a substitutes, Environ. Health Perspect., 2015, 123(7), 643–650, DOI:10.1289/ehp.1408989
. - G. Ficociello, V. Gerardi, D. Uccelletti and A. Setini, Molecular and cellular responses to short exposure to bisphenols A, F, and S and eluates of microplastics in C. elegans, Environ. Sci. Pollut. Res., 2021, 28(1), 805–818, DOI:10.1007/s11356-020-10498-5
. - M. van Praagh, C. Hartman, and E. Brandmyr, Microplastics in Landfill Leachates in the Nordic Countries, in 14th International Conference on Environmental Effects of Nanoparticles and Nanomaterials, Nordic Council of Ministers, Copenhagen, 2019, pp. 1–4, http://urn.kb.se/resolve?urn=urn:nbn:se:norden:org:diva-5429 Search PubMed
. - J. W. Metzger, Drugs in Municipal Landfills and Landfill Leachates, Pharm. Environ., 2004, 133–137, DOI:10.1007/978-3-662-09259-0_10
. - P. He, L. Chen, L. Shao, H. Zhang and F. Lü, Municipal solid waste (MSW) landfill: A source of microplastics? -Evidence of microplastics in landfill leachate, Water Res., 2019, 159, 38–45 CrossRef
. - C. E. Talsness, A. J. M. Andrade, S. N. Kuriyama, J. A. Taylor and F. S. V. Saal, Components of plastic: experimental studies in animals and relevance for human health, Philos. Trans. R. Soc., B, 2009, 364(1526), 2079–2096, DOI:10.1098/rstb.2008.0281
. - T. Yamamoto, A. Yasuhara, H. Shiraishi and O. Nakasugi, Bisphenol A in hazardous waste landfill leachates, Chemosphere, 2001, 42(4), 415–418 CrossRef CAS
. - R. A. Adaryani and O. Keen, Occurrence of pharmaceuticals and plasticizers in leachate from municipal landfills of different age, Waste Manage., 2022, 141, 1–7 CrossRef
. - J. R. Masoner, D. W. Kolpin, E. T. Furlong, I. M. Cozzarelli and J. L. Gray, Landfill leachate as a mirror of today's disposable society: Pharmaceuticals and other contaminants of emerging concern in final leachate from landfills in the conterminous United States, Environ. Toxicol. Chem., 2016, 35(4), 906–918 CrossRef PubMed
, https://pubmed.ncbi.nlm.nih.gov/26562222/. - C. Chen, L. Chen, Y. Yao, F. Artigas, Q. Huang and W. Zhang, Organotin Release from Polyvinyl Chloride Microplastics and Concurrent Photodegradation in Water: Impacts from Salinity, Dissolved Organic Matter, and Light Exposure, Environ. Sci. Technol., 2019, 53(18), 10741–10752, DOI:10.1021/acs.est.9b03428
. - C. Chen, L. Chen, Y. Li, W. Fu, X. Shi and J. Duan, et al., Impacts of microplastics on organotins' photodegradation in aquatic environments, Environ. Pollut., 2020, 267, 115686 CrossRef PubMed
. - A. C. Narevski, M. I. Novaković, M. Z. Petrović, I. J. Mihajlović, N. B. Maoduš and V. V. Goran, et al., Occurrence of bisphenol A and microplastics in landfill leachate: lessons from South East Europe, Environ. Sci. Pollut. Res., 28(31), 42196–42203, DOI:10.1007/s11356-021-13705-z
. - H. X. Zhao, F. S. Zhou, L. M. A. Evelina, J. L. Liu and Y. Zhou, A review on the industrial solid waste application in pelletizing additives: Composition, mechanism and process characteristics, J. Hazard. Mater., 2022, 423, 127056 CrossRef
, https://linkinghub.elsevier.com/retrieve/pii/S0304389421020240. - I. D. B. Segundo, R. J. E. Martins, R. A. R. Boaventura, T. F. C. V. Silva, F. C. Moreira and V. J. P. Vilar, Finding a suitable treatment solution for a leachate from a non-hazardous industrial solid waste landfill, J. Environ. Chem. Eng., 2021, 9(2), 105168 CrossRef
. - C. Viegas, C. Nobre, A. Mota, C. Vilarinho, L. Gouveia and M. Gonçalves, A circular approach for landfill leachate treatment: Chemical precipitation with biomass ash followed by bioremediation through microalgae, J. Environ. Chem. Eng., 2021, 9(3), 105187 CrossRef
, https://linkinghub.elsevier.com/retrieve/pii/S2213343721001640. - H. J. Ehrig and R. Stegmann, Leachate Quality. Solid Waste Landfilling: Concepts, Processes, Technologies, 2018, 1, 511–539 Search PubMed
. - Y. L. Tan, B. H. Hameed and A. Z. Abdullah, Deoxygenation of pyrolysis vapour derived from durian shell using catalysts prepared from industrial wastes rich in Ca, Fe, Si and Al, Sci. Total Environ., 2020, 703, 134902 CrossRef PubMed
. - Y. J. Li, Z. Liu, Q. Y. Qin, L. L. Jiang and S. Mao, et al., Microwave digestion and alkali fusion assisted hydrothermal synthesis of zeolite from coal fly ash for enhanced adsorption of Cd(II) in aqueous solution, J. Cent. South Univ., 2018, 25(1), 9–20, DOI:10.1007/s11771-018-3712-0
. - M. Vidaurre-Arbizu, S. Pérez-Bou, A. Zuazua-Ros and C. Martín-Gómez, From the leather industry to building sector: Exploration of potential applications of discarded solid wastes, J. Cleaner Prod., 2021, 291, 125960 CrossRef
. - A. Khajeh, R. Jamshidi Chenari and M. Payan, A Simple Review of Cemented Non-conventional Materials: Soil Composites, Geotech. Geol. Eng., 2020, 38(2), 1019–1040, DOI:10.1007/s10706-019-01090-x
. - Lu H. Liu, M. Liu, L. Cai, N. Wei and Y. Liu, Microanalytical characterizations, mechanical strength and water resistance performance of solidified dredged sludge with industrial solid waste and architecture residue soil, Case Stud. Constr. Mater., 2022, 1, 17 Search PubMed
. - C. Miao, L. Liang, F. Zhang, S. Chen, K. Shang and J. Jiang, et al., Review of the fabrication and application of porous materials from silicon-rich industrial solid waste, Int. J. Miner., Metall. Mater., 2022, 29, 424–438 CrossRef
. - A. Zacarias-Farah and E. Geyer-Allély, Household consumption patterns in OECD countries: trends and figures, J. Cleaner Prod., 2003, 11(8), 819–827 CrossRef
. - R. J. Slack, J. R. Gronow and N. Voulvoulis, Household hazardous waste in municipal landfills: contaminants in leachate, Sci. Total Environ., 2005, 337(1–3), 119–137 CrossRef PubMed
, https://pubmed.ncbi.nlm.nih.gov/15626384/. - H. I. Abdel-Shafy and M. S. M. Mansour, Solid waste issue: Sources, composition, disposal, recycling, and valorization, Egypt. J. Pet., 2018, 27, 1275–1290 CrossRef
. - US EPA OO of RC and R. Municipal Solid Waste, 2016. available from: https://archive.epa.gov/epawaste/nonhaz/municipal/web/html/.
- G. Ozbay, M. Jones, M. Gadde, S. Isah and T. Attarwala, Design and Operation of Effective Landfills with Minimal Effects on the Environment and Human Health, J. Environ. Public Health, 2021, 2021, 1–13 CrossRef PubMed
. - J. Kapelewska, U. Kotowska and K. Wiśniewska, Determination of personal care products and hormones in leachate and groundwater from Polish MSW landfills by ultrasound-assisted emulsification microextraction and GC-MS, Environ. Sci.
Pollut. Res. Int., 2016, 23(2), 1642–1652 CrossRef
, https://pubmed.ncbi.nlm.nih.gov/26381788/. - M. C. Lu, Y. Y. Chen, M. R. Chiou, M. Y. Chen and H. J. Fan, Occurrence and treatment efficiency of pharmaceuticals in landfill leachates, Waste Manage., 2016, 55, 257–264 CrossRef
. - X. Yu, Q. Sui, S. Lyu, W. Zhao, X. Cao and J. Wang, et al., Do high levels of PPCPs in landfill leachates influence the water environment in the vicinity of landfills? A case study of the largest landfill in China, Environ. Int., 2020, 135, 105404 CrossRef
, https://linkinghub.elsevier.com/retrieve/pii/S0160412019330880. - D. J. Lapworth, N. Baran, M. E. Stuart and R. S. Ward, Emerging organic contaminants in groundwater: A review of sources, fate and occurrence, Environ. Pollut., 2012, 163, 287–303 CrossRef PubMed
. - A. Pal, K. Y. H. Gin, A. Y. C. Lin and M. Reinhard, Impacts of emerging organic contaminants on freshwater resources: Review of recent occurrences, sources, fate and effects, Sci. Total Environ., 2010, 408(24), 6062–6069 CrossRef PubMed
. - Y. Ravikumar, J. Yun, G. Zhang, H. M. Zabed and X. Qi, A review on constructed wetlands-based removal of pharmaceutical contaminants derived from non-point source pollution, Environ. Technol. Innovation, 2022, 26, 102504 CrossRef
, https://linkinghub.elsevier.com/retrieve/pii/S2352186422001201. - C. Teng and W. Chen, Technologies for the treatment of emerging contaminants in landfill leachate, Curr. Opin. Environ. Sci. Health, 2023, 31, 100409 CrossRef
, https://linkinghub.elsevier.com/retrieve/pii/S2468584422000848. - K. He, Y. Asada, S. Echigo and S. Itoh, Biodegradation of pharmaceuticals and personal care products in the sequential combination of activated sludge treatment and soil aquifer treatment, Environ. Technol., 2018, 41(3), 378–388, DOI:10.1080/0959333020181499810
. - P. Gautam and S. Kumar, Characterisation of hazardous waste landfill leachate and its reliance on landfill age and seasonal variation: A statistical approach, J. Environ. Chem. Eng., 2021, 9(4), 105496 CrossRef
, https://linkinghub.elsevier.com/retrieve/pii/S2213343721004735. - F. Parvin and S. M. Tareq, Impact of landfill leachate contamination on surface and groundwater of Bangladesh: a systematic review and possible public health risks assessment, Appl. Water Sci., 2021, 11(6), 100, DOI:10.1007/s13201-021-01431-3
. - S. M. Iskander, R. Zhao, A. Pathak, A. Gupta, A. Pruden and J. T. Novak, et al., A review of landfill leachate induced ultraviolet quenching substances: Sources, characteristics, and treatment, Water Res., 2018, 145, 297–311 CrossRef
. - S. M. Raghab, A. M. Abd El Meguid and H. A. Hegazi, Treatment of leachate from municipal solid waste landfill, HBRC J., 2019, 9(2), 187–192, DOI:10.1016/j.hbrcj201305007
. - T. A. Kurniawan, W. H. Lo and G. Y. S. Chan, Physico-chemical treatments for removal of recalcitrant contaminants from landfill leachate, J. Hazard. Mater., 2006, 129(1–3), 80–100 CrossRef CAS PubMed
. - H. Ateş and M. E. Argun, Advanced oxidation of landfill leachate: removal of micropollutants and identification of by-products, J. Hazard. Mater., 2021, 413, 125326 CrossRef PubMed
, https://linkinghub.elsevier.com/retrieve/pii/S0304389421002892. - H. Ateş and M. E. Argun, Fate of phthalate esters in landfill leachate under subcritical and supercritical conditions and determination of transformation products, Waste Manage., 2023, 155, 292–301 CrossRef PubMed
. - A. Laiju and R. Gandhimathi, Removal of pharmaceutical and personal care products in landfill leachate treatment process, Curr. Opin. Environ. Sci. Health, 2023, 31, 100434 CrossRef
, https://linkinghub.elsevier.com/retrieve/pii/S246858442200109X. - M. A. M. Reshadi, A. Bazargan and G. McKay, A review of the application of adsorbents for landfill leachate treatment: Focus on magnetic adsorption, Sci. Total Environ., 2020, 731, 138863 CrossRef
, https://linkinghub.elsevier.com/retrieve/pii/S0048969720323809. - E. P. Kuncoro, I. Arliyani and H. Darmoekoesoemo, Removal of Pb (II) Ions from Aqueous Solution using Mahogany (Swietenia macrophylla King) Sawdust as Lowcost Adsorbent, Jurnal Kimia dan Pendidikan Kimia, 2022, 7(1), 38 CrossRef
, https://www.researchgate.net/publication/361352765_Removal_of_Pb_II_Ions_from_Aqueous_Solution_using_Mahogany_Swietenia_macrophylla_King_Sawdust_as_Lowcost_Adsorbent. - M. Arif, G. Liu, B. Yousaf, R. Ahmed, S. Irshad and A. Ashraf, et al., Synthesis, characteristics and mechanistic insight into the clays and clay minerals-biochar surface interactions for contaminants removal-A review, J. Cleaner Prod., 2021, 310, 127548 CrossRef
. - Z. H. Zhang, H. J. Zhu, C. H. Zhou and H. Wang, Geopolymer from kaolin in China: An overview, Appl. Clay Sci., 2016, 119, 31–41 CrossRef CAS
. - K. Y. Foo, L. K. Lee and B. H. Hameed, Preparation of activated carbon from sugarcane bagasse by microwave assisted activation for the remediation of semi-aerobic landfill leachate, Bioresour. Technol., 2013, 134, 166–172 CrossRef CAS PubMed
. - I. K. Erabee, A. Ahsan, B. Jose, M. M. A. Aziz, A. W. M. Ng and S. Idrus, et al., Adsorptive Treatment of Landfill Leachate using Activated Carbon Modified with Three Different Methods, KSCE J. Civ. Eng., 2018, 22(4), 1083–1095 CrossRef
, https://researchers.cdu.edu.au/en/publications/adsorptive-treatment-of-landfill-leachate-using-activated-carbon-. - A. Alshameri, H. He, J. Zhu, Y. Xi, R. Zhu and L. Ma, et al., Adsorption of ammonium by different natural clay minerals: Characterization, kinetics and adsorption isotherms, Appl. Clay Sci., 2018, 159, 83–93 CrossRef
. - C. Papastavrou, D. Mantzavinos and E. Diamadopoulos, A comparative treatment of stabilized landfill leachate: coagulation and activated carbon adsorption vs. electrochemical oxidation, Environ. Technol., 2009, 30(14), 1547–1553 CrossRef
, https://pubmed.ncbi.nlm.nih.gov/20183999/. - R. Poblete, E. Cortes, J. Bakit and Y. Luna-Galiano, Use of fish scales as an adsorbent of organic matter present in the treatment of landfill leachate, J. Chem. Technol. Biotechnol., 2020, 95(5), 1550–1558 CrossRef
. - A. A. Halim, N. N. Z. Abidin, N. Awang, A. Ithnin, M. S. Othman and M. I. Wahab, Ammonia And Cod Removal From Synthetic Leachate Using Rice Husk Composite Adsorbent, J. Urban Environ. Eng., 2011, 5(1), 24–31 CrossRef
. - S. Sri Shalini and K. Joseph, Nitrogen management in landfill leachate: application of SHARON, ANAMMOX and combined SHARON-ANAMMOX process, Waste Manage., 2012, 32(12), 2385–2400 CrossRef PubMed
, https://pubmed.ncbi.nlm.nih.gov/22766438/. - Y. Cheng, T. Huang, X. Shi, G. Wen and Y. Sun, Removal of ammonium ion from water by Na-rich birnessite: Performance and mechanisms, J. Environ. Sci., 2017, 57, 402–410 CrossRef CAS
, https://pubmed.ncbi.nlm.nih.gov/28647261/. - H. Luo, Y. Zeng, Y. Cheng, D. He and X. Pan, Recent advances in municipal landfill leachate: A review focusing on its characteristics, treatment, and toxicity assessment, Sci. Total Environ., 2020, 703, 135468 CrossRef PubMed
. - B. Cancino-Madariaga, C. F. Hurtado and R. Ruby, Effect of pressure and pH in ammonium retention for nanofiltration and reverse osmosis membranes to be used in recirculation aquaculture systems (RAS), Aquacult. Eng., 2011, 45(3), 103–108 CrossRef
. - K. Häyrynen, E. Pongrácz, V. Väisänen, N. Pap, M. Mänttäri and J. Langwaldt, et al., Concentration of ammonium and nitrate from mine water by reverse osmosis and nanofiltration, Desalination, 2009, 240(1–3), 280–289 CrossRef
. - D. Kulikowska, M. Zielińska and K. Konopka, Treatment of stabilized landfill leachate in an integrated adsorption–fine-ultrafiltration system, Int. J. Environ. Sci. Technol., 2019, 16(1), 423–430 CrossRef
. - K. Košutić, D. Dolar and T. Strmecky, Treatment of landfill leachate by membrane processes of nanofiltration and reverse osmosis, Desalin. Water Treat., 2014, 55(10), 2680–2689, DOI:10.1080/19443994.2014.939863
. - M. Pirbazari, V. Ravindran, B. N. Badriyha and S. H. Kim, Hybrid membrane filtration process for leachate treatment, Water Res., 1996, 30(11), 2691–2706 CrossRef
. - S. A. Siddiqi, A. Al-Mamun, M. S. Baawain and A. Sana, A critical review of the recently developed laboratory-scale municipal solid waste landfill leachate treatment technologies, Sustainable Energy Technol. Assess., 2022, 52, 102011 CrossRef
. - Y. G. Miao, T. Tao and Y. Peng, Recent advances in nitrogen removal from landfill leachate using biological treatments – A review, J. Environ. Manage., 2019, 235, 178–185 CrossRef PubMed
. - H. Luo, Y. Zeng, Y. Cheng, D. He and X. Pan, Recent advances in municipal landfill leachate: A review focusing on its characteristics, treatment, and toxicity assessment, Sci. Total Environ., 2020, 703, 135468 CrossRef PubMed
. - V. Torretta, N. Ferronato, I. A. Katsoyiannis, A. K. Tolkou and M. Airoldi, Novel and conventional technologies for landfill leachates treatment: A review, Sustainability, 2017, 9(1), 9 CrossRef
, http://www.mdpi.com/2071-1050/9/1/9. - T. Saeed, M. J. Miah and A. K. Yadav, Development of electrodes integrated hybrid constructed wetlands using organic, construction, and rejected materials as filter media: Landfill leachate treatment, Chemosphere, 2022, 303, 135273 CrossRef PubMed
. - V. Saxena, S. Kumar Padhi, P. Kumar Dikshit and L. Pattanaik, Recent developments in landfill leachate treatment: Aerobic granular reactor and its future prospects, Environ. Nanotechnol., Monit. Manage., 2022, 18, 100689 Search PubMed
. - J. Nivala, M. B. Hoos, C. Cross, S. Wallace and G. Parkin, Treatment of landfill leachate using an aerated, horizontal subsurface-flow constructed wetland, Sci. Total Environ., 2007, 380(1–3), 19–27 CrossRef
. - H. Brix, Wastewater treatment in constructed wetlands: system design, removal processes, and treatment performance, Constructed wetlands for water quality improvement, 1993 pp. pp. 9–22, available from: https://books.google.co.in/books?hl=en%26lr=%26id=qKb8DwAAQBAJ%26oi=fnd%26pg=PP23%26dq=Wastewater+treatment+in+constructed+wetlands:+system+design,+removal+processes,+and+treatment+performance.+In:+Constructed+Wetlands+for+Water+Quality+Improvement%26ots=evbl98VyBq%26sig=ah Search PubMed.
- G. Dotro, G. Langergraber, P. Molle, J. Nivala, J. Puigagut, O. Stein, et al., Treatment Wetlands, 2017, vol. 15, p. 172 Search PubMed
. - Z. Q. Liang and T. Sun, A research review and technical improvement analysis of constructed wetland systems for wastewater treatment, Chin. J. Ecol., 2003, 2, 49–55 Search PubMed
. - J. Vymazal and L. Kröpfelová, Removal of organics in constructed wetlands with horizontal sub-surface flow: A review of the field experience, Sci. Total Environ., 2009, 407(13), 3911–3922 CrossRef CAS PubMed
. - J. Vymazal, Constructed wetlands for wastewater treatment: Five decades of experience, Environ. Sci. Technol., 2011, 45(1), 61–69 CrossRef CAS PubMed
. - H. Brix, Do macrophytes play a role in constructed treatment wetlands?, in Water Science and Technology, 1997 Search PubMed
. - Z. C. F. Liang, C. L. Peng, Z. L. Lai, D. F. Chen and Z. H. Chen, Plant growth, community structure, and nutrient removal in monoculture and mixed constructed wetlands, Ecol. Eng., 2011, 37(2), 309–316 CrossRef
. - S. Mangkoedihardjo and I. Arliyani, Performance of Selected Plants Based Growth on Landfill Leachate Treatment Using Wetland Application, Israa Univ. J. Appl. Sci., 2023, 6(2), 71–84 CrossRef
, https://iujas.israa.edu.ps/Fileproject/Files/Journal/voulm/pdffile/2023326114540.pdf. - M. A. Belmont and C. D. Metcalfe, Feasibility of using ornamental plants
(Zantedeschia aethiopica) in subsurface flow treatment wetlands to remove nitrogen, chemical oxygen demand and nonylphenol ethoxylate surfactants - A laboratory-scale study, Ecol. Eng., 2003, 21(4–5), 233–247 CrossRef
, https://linkinghub.elsevier.com/retrieve/pii/S0925857403000934. - F. Zurita, J. de Anda and M. A. Belmont, Performance of laboratory-scale wetlands planted with tropical ornamental plants to treat domestic wastewater, Water Qual. Res. J. Can., 2006, 41(4), 410–417 CrossRef CAS
, https://iwaponline.com/wqrj/article/41/4/410/39754/Performance-of-LaboratoryScale-Wetlands-Planted. - E. J. J. Sieben, N. B. Collins, H. Mtshali and C. E. Venter, The vegetation of inland wetlands with salt-tolerant vegetation in South Africa: Description, classification and explanatory environmental factors, S. Afr. J. Bot., 2016, 104, 199–207 CrossRef
, https://linkinghub.elsevier.com/retrieve/pii/S0254629916000065. - E. J. J. Sieben, T. Nyambeni, H. Mtshali, F. T. J. Corry, C. E. Venter and D. R. MacKenzie, et al., The herbaceous vegetation of subtropical freshwater wetlands in South Africa: Classification, description and explanatory environmental factors, S. Afr. J. Bot., 2016, 104, 158–166 CrossRef
, https://linkinghub.elsevier.com/retrieve/pii/S0254629916000120. - H. Wang, C. Yang, B. Wang, Z. He and T. Fu, Nitrogen removal performance and microbiological characteristics for the landfill leachate treatment in a three-stage vertical flow constructed wetlands system, Environ. Technol. Innovation, 2022, 28, 102728 CrossRef
, https://linkinghub.elsevier.com/retrieve/pii/S2352186422002383. - A. Ikhlaq, F. Javed, A. Akram, U. Y. Qazi, Z. Masood and T. Ahmed, et al., Treatment of leachate through constructed wetlands using Typha angustifolia in combination with catalytic ozonation on Fe-zeolite A, Int. J. Phytorem., 2021, 23(8), 809–817 CrossRef
. - N. Meky, M. Fujii and A. Tawfik, Treatment of hypersaline hazardous landfill leachate using a baffled constructed wetland system: effect of granular packing media and vegetation, Environ. Technol., 2019, 40(4), 518–528 CrossRef
. - H. I. Gomes, W. M. Mayes, P. Whitby and M. Rogerson, Constructed wetlands for steel slag leachate management: Partitioning of arsenic, chromium, and vanadium in waters, sediments, and plants, J. Environ. Manage., 2019, 243, 30–38 CrossRef PubMed
. - G. O’Connor and R. Courtney, Constructed wetlands for the treatment of bauxite residue leachate: Long term field evidence and implications for management, Ecol. Eng., 2020, 158, 106076 CrossRef
. - A. Wdowczyk, A. Szymańska-Pulikowska and B. Gałka, Removal of selected pollutants from landfill leachate in constructed wetlands with different filling, Bioresour. Technol., 2022, 353, 127136 CrossRef CAS PubMed
, https://linkinghub.elsevier.com/retrieve/pii/S0960852422004655. - H. Wang, C. Yang, B. Wang, Z. He and T. Fu, Nitrogen removal performance and microbiological characteristics for the landfill leachate treatment in a three-stage vertical flow constructed wetlands system, Environ. Technol. Innovation, 2022, 28, 102728 CrossRef
, https://linkinghub.elsevier.com/retrieve/pii/S2352186422002383. - I. Arliyani, B. V. Tangahu, S. Mangkoedihardjo, E. Zulaika and S. B. Kurniawan, Enhanced leachate phytodetoxification test combined with plants and rhizobacteria bioaugmentation, Heliyon, 2023, 9(1), e12921 CrossRef PubMed
, https://linkinghub.elsevier.com/retrieve/pii/S2405844023001287. - V. Cano, D. v. Vich, H. H. B. Andrade, D. T. P. Salinas and M. A. Nolasco, Nitrification in multistage horizontal flow treatment wetlands for landfill leachate treatment, Sci. Total Environ., 2020, 20, 704 Search PubMed
. - N. E. Camaño Silvestrini, M. A. Maine, H. R. Hadad, E. Nocetti and M. A. Campagnoli, Effect of feeding strategy on the performance of a pilot scale vertical flow wetland for the treatment of landfill leachate, Sci. Total Environ., 2019, 648, 542–549 CrossRef PubMed
. - V. Cano, D. v. Vich, D. P. L. Rousseau, P. N. L. Lens and M. A. Nolasco, Influence of recirculation over COD and N-NH4
removals from landfill leachate by horizontal flow constructed treatment wetland, Int. J. Phytorem., 2019, 21(10), 998–1004 CrossRef
. - T. Saeed, J. Miah, N. Majed, M. Hasan and T. Khan, Pollutant removal from landfill leachate employing two-stage constructed wetland mesocosms: co-treatment with municipal sewage, Environ. Sci. Pollut. Res, 2020, 27(22), 28316–28332, DOI:10.1007/s11356-020-09208-y
. - D. Higgins, T. Curtin and R. Courtney, Effectiveness of a constructed wetland for treating alkaline bauxite residue leachate: a 1-year field study, Environ. Sci. Pollut. Res., 2017, 24(9), 8516–8524 CrossRef
. - A. Masbough, K. Frankowski, K. J. Hall and S. J. B. Duff, The effectiveness of constructed wetland for treatment of woodwaste leachate, in Ecological Engineering, 2005, pp. 552–566 Search PubMed
. - P. Grafias, N. P. Xekoukoulotakis, D. Mantzavinos and E. Diamadopoulos, Pilot treatment of olive pomace leachate by vertical-flow constructed wetland and electrochemical oxidation: An efficient hybrid process, Water Res., 2010, 44(9), 2773–2780 CrossRef
. - H. I. Abdel-Shafy, M. A. El-Khateeb, M. Regelsberger, R. El-Sheikh and M. Shehata, Integrated system for the treatment of blackwater and greywater via UASB and constructed wetland in Egypt, Desalin. Water Treat., 2009, 8(1–3), 272–278, DOI:10.5004/dwt.2009.788
. - M. A. El-Khateeb and F. A. El-Gohary, Combining UASB technology and constructed wetland for domestic wastewater reclamation and reuse, Water Sci. Technol.: Water Supply, 2003, 3(4), 201–208 Search PubMed
, https://www.researchgate.net/publication/237774090_Combining_UASB_technology_and_constructed_wetland_for_domestic_wastewater_reclamation_and_reuse. - M. A. El-Khateeb, A. Z. Al-Herrawy, M. M. Kamel and F. A. El-Gohary, Use of wetlands as post-treatment of anaerobically treated effluent, Desalination, 2009, 245(1–3), 50–59 CrossRef
. - A. Yakar, C. Türe, O. C. Türker, J. Vymazal and Ç. Saz, Impacts of various filtration media on wastewater treatment and bioelectric production in up-flow constructed wetland combined with microbial fuel cell (UCW-MFC), Ecol. Eng., 2018, 117, 120–132 CrossRef
. - F. Liu, L. Sun, J. Wan, A. Tang, M. Deng and R. Wu, Organic matter and ammonia removal by a novel integrated process of constructed wetland and microbial fuel cells, RSC Adv., 2019, 9(10), 5384–5393 RSC
, https://rsc.66557.net/en/content/articlehtml/2019/ra/c8ra10625h. - A. Oodally, M. Gulamhussein and D. G. Randall, Investigating the performance of constructed wetland microbial fuel cells using three indigenous South African wetland plants, J. Water Process Eng., 2019, 32, 100930 CrossRef
. - B. Ren, T. Wang and Y. Zhao, Two-stage hybrid constructed wetland-microbial fuel cells for swine wastewater treatment and bioenergy generation, Chemosphere, 2021, 268, 128803 CrossRef PubMed
. - G. S. Colares, N. Dell'Osbel, C. V. Barbosa, C. Lutterbeck, G. A. Oliveira and L. R. Rodrigues, et al., Floating treatment wetlands integrated with microbial fuel cell for the treatment of urban wastewaters and bioenergy generation, Sci. Total Environ., 2021, 766, 142474 CrossRef PubMed
. - X. L. Yang, T. Li, Y. G. Xia, R. P. Singh, H. L. Song and H. Zhang, et al., Microbial fuel cell coupled ecological floating bed for enhancing bioelectricity generation and nitrogen removal, Int. J. Hydrogen Energy, 2021, 46(20), 11433–11444 CrossRef
. - I. Araneda, N. F. Tapia, K. L. Allende and I. T. Vargas, Constructed Wetland-Microbial Fuel Cells for Sustainable Greywater Treatment, Water, 2018, 10(7), 940 CrossRef
, https://www.mdpi.com/2073-4441/10/7/940/htm. - O. C. Türker and A. Yakar, A hybrid constructed wetland combined with microbial fuel cell for boron (B) removal and bioelectric production, Ecol. Eng., 2017, 102, 411–421 CrossRef
. - T. Saeed, A. K. Yadav and M. J. Miah, Landfill leachate
and municipal wastewater co-treatment in microbial fuel cell integrated unsaturated and partially saturated tidal flow constructed wetlands, J. Water Process Eng., 2022, 46, 102633 CrossRef
, https://linkinghub.elsevier.com/retrieve/pii/S2214714422000769. - T. Saeed, M. J. Miah and A. K. Yadav, Development of electrodes integrated hybrid constructed wetlands using organic, construction, and rejected materials as filter media: Landfill leachate treatment, Chemosphere, 2022, 303, 135273 CrossRef PubMed
, https://linkinghub.elsevier.com/retrieve/pii/S0045653522017660. - T. Saeed, N. Majed, M. J. Miah and A. K. Yadav, A comparative landfill leachate treatment performance in normal and electrodes integrated hybrid constructed wetlands under unstable pollutant loadings, Sci. Total Environ., 2022, 838, 155942 CrossRef PubMed
, https://linkinghub.elsevier.com/retrieve/pii/S004896972203039X. - I. Arliyani, B. Tangahu and S. Mangkoedihardjo, Performance of Reactive Nitrogen in Leachate Treatment in Constructed Wetlands, J. Ecol. Eng., 2021, 22(5), 205–213 CrossRef
. - I. Arliyani, B. V. Tangahu and S. Mangkoedihardjo, Plant Diversity in a Constructed Wetland for Pollutant Parameter Processing on Leachate: A Review, J. Ecol. Eng., 2021, 22(4), 240–255 CrossRef
. Available from: https://yadda.icm.edu.pl/baztech/element/bwmeta1.element.baztech-6e98ecdd-00b4-4c16-b312-a291d84aff2d. - I. Arliyani, B. v. Tangahu and S. Mangkoedihardjo, Selection of Plants for Constructed Wetlands Based on Climate and Area in the Interest of Processing Pollutant Parameters on Leachate: A Review, IOP Conf. Ser.: Earth Environ. Sci., 2021, 835(1), 012003, DOI:10.1088/1755-1315/835/1/012003
. - B. Tangahu, A. A. Kartika, K. Sambodho, S. M. Marendra and I. Arliyani, Shallow Groundwater Pollution Index Around the Location of Griyo Mulyo Landfill (Jabon Landfill) in Jabon District, Sidoarjo Regency, East Java, Indonesia, J. Ecol. Eng., 2021, 22(3), 199–210 CrossRef
, http://www.jeeng.net/Shallow-Groundwater-Pollution-Index-Around-the-Location-of-Griyo-Mulyo-Landfill-Jabon,132658,0,2.html. - S. Gupta, P. Srivastava, S. A. Patil and A. K. Yadav, A comprehensive review on emerging constructed wetland coupled microbial fuel cell technology: Potential applications and challenges, Bioresour. Technol., 2021, 320, 124376 CrossRef PubMed
, https://linkinghub.elsevier.com/retrieve/pii/S0960852420316503. - P. Bhanse, M. Kumar, L. Singh, M. K. Awasthi and A. Qureshi, Role of plant growth-promoting rhizobacteria in boosting the phytoremediation of stressed soils: Opportunities, challenges, and prospects, Chemosphere, 2022, 303, 134954 CrossRef CAS
. - S. Wu, J. Vymazal and H. Brix, Critical review: biogeochemical networking of iron in constructed wetlands for wastewater treatment, Environ. Sci. Technol., 2019, 53(14), 7930–7944, DOI:10.1021/acs.est.9b00958
. - N. Khan and A. Bano, Role of PGPR in the phytoremediation of heavy metals and crop growth under municipal wastewater irrigation, Phytoremediation: Management of Environmental Contaminants, 2019, vol. 6, pp. 135–149, DOI:10.1007/978-3-319-99651-6_5
. - K. H. Vardhan, P. S. Kumar and R. C. Panda, A review on heavy metal pollution, toxicity and remedial measures: Current trends and future perspectives, J. Mol. Liq., 2019, 290, 111197, DOI:10.1016/J.MOLLIQ.2019.111197
. - E. Xiao, Y. Zhou, D. Xu, R. Lu, Y. Chen and Q. Zhou, et al., The physiological response of Arundo donax and characteristics of anodic bacterial community in BE-CW systems: Effects of the applied voltage, Chem. Eng. J., 2020, 380, 122604, DOI:10.1016/J.CEJ.2019.122604
. - J. Verma, D. Kumar, N. Singh, S. S. Katti and Y. T. Shah, Electricigens and microbial fuel cells for bioremediation and bioenergy production: a review, Environ. Chem. Lett., 2021, 19(3), 2091–2126, DOI:10.1007/s10311-021-01199-7
. - K. Elmaadawy, B. Liu, J. Hu, H. Hou and J. Yang, Performance evaluation of microbial fuel cell for landfill leachate treatment: Research updates and synergistic effects of hybrid systems, J. Environ. Sci., 2020, 96, 1–20, DOI:10.1016/J.JES.2020.05.005
. - L. Xu, Y. Zhao and X. Wang, Applying multiple bio-cathodes in constructed wetland-microbial fuel cell for promoting energy production and bioelectrical derived nitrification-denitrification, Chem. Eng. J., 2018, 344, 105–113, DOI:10.1016/J.CEJ.2018.03.065
. - A. A. Mier, H. Olvera-Vargas, M. Mejía-López, A. Longoria, L. Verea and P. J. Sebastian, et al., A review of recent advances in electrode materials for emerging bioelectrochemical systems: From biofilm-bearing anodes to specialized cathodes, Chemosphere, 2021, 283, 131138, DOI:10.1016/j.chemosphere.2021.131138
. - F. Xu, F. Q. Cao, Q. Kong, L. L. Zhou, Q. Yuan and Y. J. Zhu, et al., Electricity production and evolution of microbial community in the constructed wetland-microbial fuel cell, Chem. Eng. J., 2018, 339, 479–486, DOI:10.1016/J.CEJ.2018.02.003
. - P. Srivastava, R. Abbassi, V. Garaniya, T. Lewis and A. K. Yadav, Performance of pilot-scale horizontal subsurface flow constructed wetland coupled with a microbial fuel cell for treating wastewater, J. Water Process Eng., 2020, 33, 100994, DOI:10.1016/J.JWPE.2019.100994
. - Y. L. Oon, S. A. Ong, L. N. Ho, Y. S. Wong, F. A. Dahalan and Y. S. Oon, et al., Role of macrophyte and effect of supplementary aeration in up-flow constructed wetland-microbial fuel cell for simultaneous wastewater treatment and energy recovery, Bioresour. Technol., 2017, 224, 265–275, DOI:10.1016/J.BIORTECH.2016.10.079
. - C. Corbella and M. Garfí, Long-term assessment of best cathode position to maximise microbial fuel cell performance in horizontal subsurface flow constructed wetlands, Sci. Total Environ., 2016, 563–564, 448–455, DOI:10.1016/j.scitotenv.2016.03.170
. - C. Tang, Y. Zhao, C. Kang, Y. Yang, D. Morgan and L. Xu, Towards concurrent pollutants removal and high energy harvesting in a pilot-scale CW-MFC: Insight into the cathode conditions and electrodes connection, Chem. Eng. J., 2019, 373, 150–160, DOI:10.1016/j.cej.2019.05.035
. - Y. Wang, C. Zhao, D. Sun, J. Zhang and J. J. Zhu, A graphene/poly (3, 4-ethylenedioxythiophene) hybrid as an anode for high-performance microbial fuel cells, ChemPlusChem, 2013, 78(8), 823–829, DOI:10.1002/cplu.201300102
. - P. Mishra, M. A. Malla, A. Malla, S. Kumar Gupta, P. Mishra and M. A. Malla, et al., Poly (3, 4-ethylenedioxythiophene)-Modified Graphite Felt and Carbon Cloth Anodes for Use in Microbial Fuel Cells, ChemistrySelect, 2022, 7(5), e202103920, DOI:10.1002/slct.202103920
. - Y. Kang and S. Ibrahim, Synergetic effect of conductive polymer poly (3, 4-ethylenedioxythiophene) with different structural configuration of anode for microbial fuel cell application, Bioresour. Technol., 2015, 189, 364–369, DOI:10.1016/j.biortech.2015.04.044
. - Y. L. Oon, S. A. Ong, L. N. Ho, Y. S. Wong, F. A. Dahalan and Y. S. Oon, et al., Constructed wetland–microbial fuel cell for azo dyes degradation and energy recovery: Influence of molecular structure, kinetics, mechanisms and degradation pathways, Sci. Total Environ., 2020, 720, 137370, DOI:10.1016/J.SCITOTENV.2020.137370
. - D. Yan, X. Song, B. Weng, Z. Yu, W. Bi and J. Wang, Bioelectricity generation from air-cathode microbial fuel cell connected to constructed wetland, Water Sci. Technol., 2018, 78(9), 1990–1996, DOI:10.2166/WST.2018.471
. - C. N. Khuman, G. D. Bhowmick, M. M. Ghangrekar and A. Mitra, Effect of Using a Ceramic Separator on the Performance of Hydroponic Constructed Wetland-Microbial Fuel Cell, J. Hazard., Toxic Radioact. Waste, 2020, 24(3), 04020005, DOI:10.1061/(ASCE)HZ.2153-5515.0000499
. - S. Gupta, P. Srivastava and A. K. Yadav, Integration of microbial fuel cell into constructed wetlands: effects, applications, and future outlook, in Integrated Microbial Fuel Cells for Wastewater Treatment, Elsevier, 2020. pp. 273–293. DOI: DOI:10.1016/B978-0-12-817493-7.00013-8
. - P. Srivastava, A. K. Yadav, V. Garaniya and R. Abbassi, Constructed wetland coupled microbial fuel cell technology: development and potential applications, in Microbial Electrochemical Technology, Elsevier, 2019, pp. 1021–1036, DOI:10.1016/B978-0-444-64052-9.00042-X.
- P. Srivastava, R. Abbassi, A. Yadav, V. Garaniya, N. Kumar and S. J. Khan, et al., Enhanced chromium (VI) treatment in electroactive constructed wetlands: influence of conductive material, J. Hazard. Mater., 2020, 387, 121722, DOI:10.1016/j.jhazmat.2019.121722
. - X. Huang, C. Duan, W. Duan, F. Sun, H. Cui and S. Zhang, et al., Role of electrode materials on performance and microbial characteristics in the constructed wetland coupled microbial fuel cell (CW-MFC): A review, J. Cleaner Prod., 2021, 301, 126951, DOI:10.1016/j.jclepro.2021.126951
. - J. Wang, X. Song, Y. Wang, B. Abayneh, Y. Ding and D. Yan, et al., Microbial community structure of different electrode materials in constructed wetland incorporating microbial fuel cell, Bioresour. Technol., 2016, 221, 697–702, DOI:10.1016/j.biortech.2016.09.116
. - C. Corbella and M. Garfí, Long-term assessment of best cathode position to maximise microbial fuel cell performance in horizontal subsurface flow constructed wetlands, Sci. Total Environ., 2016, 563–564, 448–455, DOI:10.1016/j.scitotenv.2016.03.170
. - Y. Yang, Y. Zhao, C. Tang, L. Xu, D. Morgan and R. Liu, Role of macrophyte species in constructed wetland-microbial fuel cell for simultaneous wastewater treatment and bioenergy generation, Chem. Eng. J., 2020, 392, 123708, DOI:10.1016/J.CEJ.2019.123708
. - X. Wang, Y. Tian, H. Liu, X. Zhao and S. Peng, The influence of incorporating microbial fuel cells on greenhouse gas emissions from constructed wetlands, Sci. Total Environ., 2019, 656, 270–279, DOI:10.1016/J.SCITOTENV.2018.11.328
. - B. Das, S. Thakur, M. Chaithanya and P. Biswas, Batch investigation of constructed wetland microbial fuel cell with reverse osmosis (RO) concentrate and wastewater mix as substrate, Biomass Bioenergy, 2019, 122, 231–237, DOI:10.1016/j.biombioe.2019.01.017
. - L. Di, Y. Li, L. Nie, S. Wang and F. Kong, Influence of plant radial oxygen loss in constructed wetland combined with microbial fuel cell on nitrobenzene removal from aqueous solution, J. Hazard. Mater., 2020, 394, 122542, DOI:10.1016/J.JHAZMAT.2020.122542
. - J. Wang, X. Song, Y. Wang, J. Bai, M. Li and G. Dong, et al., Bioenergy generation and rhizodegradation as affected by microbial community distribution in a coupled constructed wetland-microbial fuel cell system associated with three macrophytes, Sci. Total Environ., 2017, 607–608, 53–62, DOI:10.1016/J.SCITOTENV.2017.06.243
. - Y. L. Oon, S. A. Ong, L. N. Ho, Y. S. Wong, F. A. Dahalan and Y. S. Oon, et al., Role of macrophyte and effect of supplementary aeration in up-flow constructed wetland-microbial fuel cell for simultaneous wastewater treatment and energy recovery, Bioresour. Technol., 2017, 224, 265–275, DOI:10.1016/J.BIORTECH.2016.10.079
. - J. Lin, X. Zeng, Y. Xiao, L. Tang, J. Nong and Y. Liu, et al., Novel near-infrared II aggregation-induced emission dots for in vivo bioimaging, Chem. Sci., 2019, 10(4), 1219–1226, 10.1039/C8SC04363A
. - H. O. Mohamed, E. T. Sayed, M. Obaid, Y. J. Choi, S. G. Park and S. Al-Qaradawi, et al., Transition metal nanoparticles doped carbon paper as a cost-effective anode in a microbial fuel cell powered by pure and mixed biocatalyst cultures, Int. J. Hydrogen Energy, 2018, 43(46), 21560–21571, DOI:10.1016/J.IJHYDENE.2018.09.199
. - S. Narayanasamy and J. Jayaprakash, Application of carbon-polymer based composite electrodes for Microbial fuel cells, Rev. Environ. Sci. Biotechnol., 2020, 19, 595–620, DOI:10.1007/s11157-020-09545-x
. - D. Paul, M. T. Noori, P. P. Rajesh, M. M. Ghangrekar and A. Mitra, Modification of carbon felt anode with graphene oxide-zeolite composite for enhancing the performance of microbial fuel cell, Sustainable Energy Technol. Assess., 2018, 26, 77–82, DOI:10.1016/J.SETA.2017.10.001
. - E. Taskan and H. Hasar, Comprehensive Comparison of a New Tin-Coated Copper Mesh and a Graphite Plate Electrode as an Anode Material in Microbial Fuel Cell, Appl. Biochem. Biotechnol., 2015, 175(4), 2300–2308, DOI:10.1007/s12010-014-1439-4
. - C. Kim, J. R. Kim and J. Heo, Enhancement of bioelectricity generation by a microbial fuel cell using Ti nanoparticle-modified carbon electrode, J. Chem. Technol. Biotechnol., 2019, 94(5), 1622–1627, DOI:10.1002/jctb.5931
. - H. O. Mohamed, E. T. Sayed, H. Cho, M. Park, M. Obaid and H. Y. Kim, et al., Effective strategies for anode surface modification for power harvesting and industrial wastewater treatment using microbial fuel cells, J. Environ. Manage., 2018, 206, 228–235, DOI:10.1002/JCTB.5931
. - A. Fiorani, V. Eßmann, C. S. Santos and W. Schuhmann, Enhancing Electrogenerated Chemiluminescence on Platinum Electrodes through Surface Modification, ChemElectroChem, 2020, 7(5), 1256–1260, DOI:10.1002/celc.202000103
. - S. Sevda, S. Sharma, C. Joshi, L. Pandey, N. Tyagi and I. Abu-Reesh, et al., Biofilm formation and electron transfer in bioelectrochemical systems, Environ. Technol. Rev., 2018, 7(1), 220–234, DOI:10.1080/2162251520181486889
. - H. Xu, X. Quan, Z. Xiao and L. Chen, Effect of anodes decoration with metal and metal oxides nanoparticles on pharmaceutically active compounds removal and power generation in microbial fuel cells, Chem. Eng. J., 2018, 335, 539–547, DOI:10.1080/21622515.2018.1486889
. - A. Ebrahimi, M. Sivakumar, C. McLauchlan, A. Ansari and A. S. Vishwanathan, A critical review of the symbiotic relationship between constructed wetland and microbial fuel cell for enhancing pollutant removal and energy generation, J. Environ. Chem. Eng., 2021, 9, 105011, DOI:10.1016/J.CEJ.2017.10.159
. - M. Hartl, D. F. Bedoya-Ríos, M. Fernández-Gatell, D. P. L. Rousseau, G. du Laing and M. Garfí, et al., Contaminants removal and bacterial activity enhancement along the flow path of constructed wetland microbial fuel cells, Sci. Total Environ., 2019, 652, 1195–1208, DOI:10.1016/J.SCITOTENV.2018.10.234
. - H. Li, S. Zhang, X. L. Yang, Y. L. Yang, H. Xu and X. N. Li, et al., Enhanced degradation of bisphenol A and ibuprofen by an up-flow microbial fuel cell-coupled constructed wetland and analysis of bacterial community structure, Chemosphere, 2019, 217, 599–608, DOI:10.1016/j.chemosphere.2018.11.022
. - M. Wei, J. Rakoczy, C. Vogt, F. Harnisch, R. Schumann and H. H. Richnow, Enhancement and monitoring of pollutant removal in a constructed wetland by microbial electrochemical technology, Bioresour. Technol., 2015, 196, 490–499, DOI:10.1016/J.BIORTECH.2015.07.111
. - L. Wang, Y. Zhou, F. Peng, A. Zhang, Q. Pang and J. Lian, et al., Intensified nitrogen removal in the tidal flow constructed wetland-microbial fuel cell: Insight into evaluation of denitrifying genes, J. Cleaner Prod., 2020, 264, 121580, DOI:10.1016/J.JCLEPRO.2020.121580
. - T. González, J. Puigagut and G. Vidal, Organic matter removal and nitrogen transformation by a constructed wetland-microbial fuel cell system with simultaneous bioelectricity generation, Sci. Total Environ., 2021, 753, 142075, DOI:10.1016/J.SCITOTENV.2020.142075
. - Y. Hu, N. Li, J. Jiang, Y. Xu and X. Luo, et al., Simultaneous Feammox and anammox process facilitated by activated carbon as an electron shuttle for autotrophic biological nitrogen removal, Front. Environ. Sci. Eng., 2021, 16(7), 90, DOI:10.1007/s11783-021-1498-z
. - J. Lu, Z. Guo, Y. Pan, M. Li, X. Chen and M. He, et al., Simultaneously enhanced removal of PAHs and nitrogen driven by Fe2+/Fe3+ cycle in constructed wetland through automatic tidal operation, Water Res., 2022, 215, 118232, DOI:10.1016/j.watres.2022.118232
. - W. W. Li, H. Q. Yu and B. E. Rittmann, Chemistry: Reuse water pollutants, Nature, 2015, 528(7580), 29–31, DOI:10.1038/528029a
. - Z. T. Khanzada, Phosphorus removal from landfill leachate by microalgae, Biotechnol. Rep., 2020, 25, e00419, DOI:10.1016/j.btre.2020.e00419
. - S. Lu, X. Zhang, J. Wang and L. Pei, Impacts of different media on constructed wetlands for rural household sewage treatment, J. Cleaner Prod., 2016, 127, 325–330, DOI:10.1016/J.JCLEPRO.2016.03.166
. - M. Molinos-Senante, F. Hernández-Sancho and R. Sala-Garrido, Cost–benefit analysis of water-reuse projects for environmental purposes: A case study for Spanish wastewater treatment plants, J. Environ. Manage., 2011, 92(12), 3091–3097, DOI:10.1016/J.JENVMAN.2011.07.023
. - J. Vymazal, Removal of nutrients in various types of constructed wetlands, Sci. Total Environ., 2007, 380(1–3), 48–65, DOI:10.1016/j.scitotenv.2006.09.014
. - W. Apollon, I. Rusyn, N. González-Gamboa, T. Kuleshova, A. I. Luna-Maldonado and J. A. Vidales-Contreras, et al., Improvement of zero waste sustainable recovery using microbial energy generation systems: A comprehensive review, Sci. Total Environ., 2022, 817, 153055, DOI:10.1016/J.SCITOTENV.2022.153055
. - J. Lu, S. E. Bunn and M. A. Burford, Nutrient release and uptake by littoral macrophytes during water level fluctuations, Sci. Total Environ., 2018, 622–623, 29–40, DOI:10.1016/J.SCITOTENV.2017.11.199
. - G. Markou and D. Georgakakis, Cultivation of filamentous cyanobacteria (blue-green algae) in agro-industrial wastes and wastewaters: A review, Appl. Energy, 2011, 88(10), 3389–3401, DOI:10.1016/J.APENERGY.2010.12.042
. - S. Liu, H. Song, S. Wei, F. Yang and X. Li, Bio-cathode materials evaluation and configuration optimization for power output of vertical subsurface flow constructed wetland — Microbial fuel cell systems, Bioresour. Technol., 2014, 166, 575–583, DOI:10.1016/J.BIORTECH.2014.05.104
. - J. Villaseñor, P. Capilla, M. A. Rodrigo, P. Cañizares and F. J. Fernández, Operation of a horizontal subsurface flow constructed wetland – Microbial fuel cell treating wastewater under different organic loading rates, Water Res., 2013, 47(17), 6731–6738, DOI:10.1016/J.WATRES.2013.09.005
. - X. Wang, Y. Tian, H. Liu, X. Zhao and Q. Wu, Effects of influent COD/TN ratio on nitrogen removal in integrated constructed wetland–microbial fuel cell systems, Bioresour. Technol., 2019, 271, 492–495, DOI:10.1016/J.BIORTECH.2018.09.039
. - H. Wen, H. Zhu, B. Yan, B. Shutes, X. Yu and R. Cheng, et al., Constructed wetlands integrated with microbial fuel cells for COD and nitrogen removal affected by plant and circuit operation mode, Environ. Sci. Pollut. Res., 2021, 28(3), 3008–3018, DOI:10.1007/S11356-020-10632-3
. - F. Xu, D. L. Ouyang, E. R. Rene, H. Y. Ng, L. L. Guo and Y. J. Zhu, et al., Electricity production enhancement in a constructed wetland-microbial fuel cell system for treating saline wastewater, Bioresour. Technol., 2019, 288, 121462, DOI:10.1016/J.BIORTECH.2019.121462
. - K. Zhang, X. Wu, H. Luo, X. Li, W. Chen and J. Chen, et al., CH4 control and associated microbial process from constructed wetland (CW) by microbial fuel cells (MFC), J. Environ. Manage., 2020, 260, 110071, DOI:10.1016/J.JENVMAN.2020.110071
. - H. Li, H. Xu, H. L. Song, Y. Lu and X. L. Yang, Antibiotic resistance genes, bacterial communities, and functions in constructed wetland-microbial fuel cells: Responses to the co-stresses of antibiotics and zinc, Environ. Pollut., 2020, 265, 115084, DOI:10.1016/J.ENVPOL.2020.115084
. - X. Wang, Y. Tian, H. Liu, X. Zhao and S. Peng, Optimizing the performance of organics and nutrient removal in constructed wetland–microbial fuel cell systems, Sci. Total Environ., 2019, 653, 860–871, DOI:10.1016/J.SCITOTENV.2018.11.005
. - H. Li, H. L. Song, X. L. Yang, S. Zhang, Y. L. Yang and L. M. Zhang, et al., A continuous flow MFC-CW coupled with a biofilm electrode reactor to simultaneously attenuate sulfamethoxazole and its corresponding resistance genes, Sci. Total Environ., 2018, 637–638, 295–305, DOI:10.1016/J.SCITOTENV.2018.04.359
. - Y. L. Oon, S. A. Ong, L. N. Ho, Y. S. Wong, F. A. Dahalan and Y. S. Oon, et al., Up-flow constructed wetland-microbial fuel cell for azo dye, saline, nitrate remediation and bioelectricity generation: From waste to energy approach, Bioresour. Technol., 2018, 266, 97–108, DOI:10.1016/J.BIORTECH.2018.06.035
. - J. Wang, X. Song, Y. Wang, B. Abayneh, Y. Li and D. Yan, et al., Nitrate removal and bioenergy production in constructed wetland coupled with microbial fuel cell: Establishment of electrochemically active bacteria community on anode, Bioresour. Technol., 2016, 221, 358–365, DOI:10.1016/J.BIORTECH.2016.09.054
. - G. Wang, Y. Guo, J. Cai, H. Wen, Z. Mao and H. Zhang, et al., Electricity production and the analysis of the anode microbial community in a constructed wetland-microbial fuel cell, RSC Adv., 2019, 9(37), 21460–21472, 10.1039/C8RA10130B
. - L. Wang, Y. Zhou, F. Peng, A. Zhang, Q. Pang and J. Lian, et al., Intensified nitrogen removal in the tidal flow constructed wetland-microbial fuel cell: Insight into evaluation of denitrifying genes, J. Cleaner Prod., 2020, 264, 121580, DOI:10.1016/J.JCLEPRO.2020.121580
. - Z. Fang, H. L. Song, N. Cang and X. N. Li, Electricity production from Azo dye wastewater using a microbial fuel cell coupled constructed wetland operating under different operating conditions, Biosens. Bioelectron., 2015, 68, 135–141, DOI:10.1016/J.BIOS.2014.12.047
. - L. Liu, Y. Yuan, F. Li and C. Feng, In-situ Cr(VI) reduction with electrogenerated hydrogen peroxide driven by iron-reducing bacteria, Bioresour. Technol., 2011, 102(3), 2468–2473, DOI:10.1016/J.BIORTECH.2010.11.013
. - L. Wang, D. Xu, Q. Zhang, T. Liu and Z. Tao, Simultaneous removal of heavy metals and bioelectricity generation in microbial fuel cell coupled with constructed wetland: an optimization study on substrate and plant types, Environ. Sci. Pollut. Res., 2022, 29(1), 768–778, DOI:10.1007/s11356-021-15688-3
. - A. S. Mathuriya and J. V. Yakhmi, Microbial fuel cells to recover heavy metals, Environ. Chem. Lett., 2014, 12(4), 483–494, DOI:10.1007/s10311-014-0474-2
. - A. S. Mathuriya, Eco-Affectionate Face of Microbial, Fuel Cells, 2013, 44(2), 97–153, DOI:10.1080/106433892012710445
. - F. fei Liu, T. Lu and Y. X. Zhang, Performance assessment of constructed wetland-microbial fuel cell for treatment of mariculture wastewater containing heavy metals, Process Saf. Environ. Prot., 2022, 168, 633–641, DOI:10.1016/J.PSEP.2022.10.026
. - Q. Wang, R. Lv, E. R. Rene, X. Qi, Q. Hao and Y. Du, et al., Characterization of microbial community and resistance gene (CzcA) shifts in up-flow constructed wetlands-microbial fuel cell treating Zn (II) contaminated wastewater, Bioresour. Technol., 2020, 302, 122867, DOI:10.1016/J.BIORTECH.2020.122867
. - C. C. Zhao, D. W. Shang, Y. L. Zou, Y. d. Du, Q. Wang and F. Xu, et al., Changes in electricity production and microbial community evolution in constructed wetland-microbial fuel cell exposed to wastewater containing Pb(II), Sci. Total Environ., 2020, 732, 139127, DOI:10.1016/J.SCITOTENV.2020.139127
. - S. Liu, F. Lu, D. Qiu and X. Feng, Wetland plants selection and electrode optimization for constructed wetland-microbial fuel cell treatment of Cr(VI)-containing wastewater, J. Water Process Eng., 2022, 49, 103040, DOI:10.1016/J.JWPE.2022.103040
. - O. Guadarrama-Pérez, T. Gutiérrez-Macías, L. García-Sánchez, V. H. Guadarrama-Pérez and E. B. Estrada-Arriaga, Recent advances in constructed wetland-microbial fuel cells for simultaneous bioelectricity production and wastewater treatment: A review, Int. J. Energy Res., 2019, 43, 5106–5127, DOI:10.1002/er.4496
. - C. Tang, Y. Zhao, C. Kang, Y. Yang, D. Morgan and L. Xu, Towards concurrent pollutants removal and high energy harvesting in a pilot-scale CW-MFC: Insight into the cathode conditions and electrodes connection, Chem. Eng. J., 2019, 373, 150–160, DOI:10.1016/J.CEJ.2019.05.035
. - L. Xu, Y. Zhao, X. Wang and W. Yu, Applying multiple bio-cathodes in constructed wetland-microbial fuel cell for promoting energy production and bioelectrical derived nitrification-denitrification process, Chem. Eng. J., 2018, 344, 105–113, DOI:10.1016/J.CEJ.2018.03.065
. - A. Aguirre-Sierra, T. Bacchetti-De Gregoris, J. J. Salas, A. de Deus and A. Esteve-Núñez, A new concept in constructed wetlands: assessment of aerobic electroconductive biofilters, Environ. Sci., 2020, 6(5), 1312–1323, 10.1039/C9EW00696F
. - F. Xu, D. L. Ouyang, E. R. Rene, H. Y. Ng, L. L. Guo and Y. J. Zhu, et al., Electricity production enhancement in a constructed wetland-microbial fuel cell system for treating saline wastewater, Bioresour. Technol., 2019, 288, 121462, DOI:10.1016/J.BIORTECH.2019.121462
. - L. Xu, B. Wang, X. Liu, W. Yu and Y. Zhao, Maximizing the energy harvest from a microbial fuel cell embedded in a constructed wetland, Appl. Energy, 2018, 214, 83–91, DOI:10.1016/J.APENERGY.2018.01.071
. - J. Wang, X. Song, Y. Wang, B. Abayneh, Y. Ding and D. Yan, et al., Microbial community structure of different electrode materials in constructed wetland incorporating microbial fuel cell, Bioresour. Technol., 2016, 221, 697–702, DOI:10.1016/J.BIORTECH.2016.09.116
. - X. Ge, X. Cao, X. Song, Y. Wang, Z. Si and Y. Zhao, et al., Bioenergy generation and simultaneous nitrate and phosphorus removal in a pyrite-based constructed wetland-microbial fuel cell, Bioresour. Technol., 2020, 296, 122350, DOI:10.1016/J.BIORTECH.2019.122350
. - Y. Yang, Y. Zhao, C. Tang, L. Xu, D. Morgan and R. Liu, Role of macrophyte species in constructed wetland-microbial fuel cell for simultaneous wastewater treatment and bioenergy generation, Chem. Eng. J., 2020, 392, 123708, DOI:10.1016/J.CEJ.2019.123708
. - Z. Fang, H. L. Song, N. Cang and X. N. Li, Performance of microbial fuel cell coupled constructed wetland system for decolorization of azo dye and bioelectricity generation, Bioresour. Technol., 2013, 144, 165–171, DOI:10.1016/J.BIORTECH.2013.06.073
. - H. Li, S. Zhang, X. L. Yang, Y. L. Yang, H. Xu and X. N. Li, et al., Enhanced degradation of bisphenol A and ibuprofen by an up-flow microbial fuel cell-coupled constructed wetland and analysis of bacterial community structure, Chemosphere, 2019, 217, 599–608, DOI:10.1016/J.CHEMOSPHERE.2018.11.022
. - B. Ji, P. Kang, T. Wei and Y. Zhao, Challenges of aqueous per- and polyfluoroalkyl substances (PFASs) and their foreseeable removal strategies, Chemosphere, 2020, 250, 126316, DOI:10.1016/J.CHEMOSPHERE.2020.126316
. - C. C. Zhao, D. W. Shang, Y. L. Zou, Y. d. Du, Q. Wang and F. Xu, et al., Changes in electricity production and microbial community evolution in constructed wetland-microbial fuel cell exposed to wastewater containing Pb(II), Sci. Total Environ., 2020, 732, 139127, DOI:10.1016/J.SCITOTENV.2020.139127
. - L. Xu, Y. Zhao, C. Fan, Z. Fan and F. Zhao, First study to explore the feasibility of applying microbial fuel cells into constructed wetlands for COD monitoring, Bioresour. Technol., 2017, 243, 846–854, DOI:10.1016/J.BIORTECH.2017.06.179
. - C. Corbella, M. Hartl, M. Fernandez-gatell and J. Puigagut, MFC-based biosensor for domestic wastewater COD assessment in constructed wetlands, Sci. Total Environ., 2019, 660, 218–226, DOI:10.1016/J.SCITOTENV.2018.12.347
. - Y. Wang, Y. Zhao, L. Xu, W. Wang, L. Doherty and C. Tang, et al., Constructed wetland integrated microbial
fuel cell system: looking back, moving forward, Water Sci. Technol., 2017, 76(2), 471–477, DOI:10.2166/WST.2017.190
. - O. Guadarrama-Pérez, T. Gutiérrez-Macías, L. García-Sánchez, V. H. Guadarrama-Pérez and E. B. Estrada-Arriaga, Recent advances in constructed wetland-microbial fuel cells for simultaneous bioelectricity production and wastewater treatment: A review, Int. J. Energy Res., 2019, 43(10), 5106–5127, DOI:10.1002/er.4496
. - P. Srivastava, R. Abbassi, A. K. Yadav, V. Garaniya and M. Asadnia, A review on the contribution of electron flow in electroactive wetlands: Electricity generation and enhanced wastewater treatment, Chemosphere, 2020, 254, 126926, DOI:10.1016/J.CHEMOSPHERE.2020.126926
. - Y. Zhao, B. Ji, R. Liu, B. Ren and T. Wei, Constructed treatment wetland: Glance of development and future perspectives, Water Cycle, 2020, 1, 104–112, DOI:10.1016/j.watcyc.2020.07.002
. - Q. Yang, C. Gao, Z. X. Wu, S. N. Liang and M. H. Liu, Activated carbon clogging analysis in an integration of constructed wetland with microbial fuel cell, E3S Web Conf., 2018, 53, 1025, DOI:10.1051/e3sconf/20185301025
. - A. Paitier, A. Godain, D. Lyon, N. Haddour, T. M. Vogel and J. M. Monier, Microbial fuel cell anodic microbial population dynamics during MFC start-up, Biosens. Bioelectron., 2017, 92, 357–363, DOI:10.1016/J.BIOS.2016.10.096
. - L. Xu, B. Wang, X. Liu, W. Yu and Y. Zhao, Maximizing the energy harvest from a microbial fuel cell embedded in a constructed wetland, Appl. Energy, 2018, 214, 83–91, DOI:10.1016/J.APENERGY.2018.01.071
. - B. Yu, C. Liu, S. Wang, W. Wang, S. Zhao and G. Zhu, Applying constructed wetland-microbial electrochemical system to enhance NH4+ removal at low temperature, Sci. Total Environ., 2020, 724, 138017, DOI:10.1016/J.SCITOTENV.2020.138017
. - C. Tang, Y. Zhao, C. Kang, Y. Yang, D. Morgan and L. Xu, Towards concurrent pollutants removal and high energy harvesting in a pilot-scale CW-MFC: Insight into the cathode conditions and electrodes connection, Chem. Eng. J., 2019, 373, 150–160, DOI:10.1016/J.CEJ.2019.05.035
. - J. Wang, X. Song, Y. Wang, Z. Zhao, B. Wang and D. Yan, Effects of electrode material and substrate concentration on the bioenergy output and wastewater treatment in air-cathode microbial fuel cell integrating with constructed wetland, Ecol. Eng., 2017, 99, 191–198, DOI:10.1016/J.ECOLENG.2016.11.015
. - Y. Zhou, D. Xu, E. Xiao, D. Xu, P. Xu and X. Zhang, et al., Relationship between electrogenic performance and physiological change of four wetland plants in constructed wetland-microbial fuel cells during non-growing seasons, J. Environ. Sci., 2018, 70, 54–62, DOI:10.1016/J.JES.2017.11.008
.
|
This journal is © The Royal Society of Chemistry 2024 |
Click here to see how this site uses Cookies. View our privacy policy here.