DOI:
10.1039/D5DT00163C
(Perspective)
Dalton Trans., 2025,
54, 5614-5639
Targeted bismuth-based materials for cancer
Received
21st January 2025
, Accepted 23rd February 2025
First published on 24th February 2025
Abstract
The use of bismuth and its compounds in biomedicine has developed rapidly in recent years. Due to their unique properties, there are great opportunities for the development of new non-invasive strategies for the early diagnosis and effective treatment of cancers. This perspective highlights key fabrication methods to generate well-defined and clinically relevant bismuth materials of varying characteristics. On the one hand, this opens up a wide range of possibilities for unimodal and multimodal imaging. On the other hand, effective treatment strategies, which are increasingly based on combinatorial therapies, are given a great deal of attention. One of the biggest challenges remains the selective tumour targeting, whether active or passive. Here we present an overview on new developments of bismuth based materials moving forward from a simple enrichment at the tumour site via uptake by the mononuclear phagocytic system (MPS) to a more active tumour specific targeting via covalent modification with tumour-seeking molecules based on either small or antibody-derived molecules.
 Amna Batool | Amna Batool is a doctoral researcher at the School of Science, Monash University specialising in nanobiotechnology and cancer theranostics. Her work focuses on the development and application of functionalised nanomaterials for biomedical use. Through the MHELTHERA collaboration at HZDR (Germany), she has engineered nanoparticles to investigate their potential in tumour-targeted diagnostics and therapy. Passionate about bridging nanotechnology and immunotherapy, Amna's research seeks to advance novel nanotechnology-based strategies for clinically relevant cancer research. |
 Ina Kopp | Ina Kopp studied chemistry at the University of Paderborn, wrote her thesis at the University of Innsbruck in 2021and is now a doctoral researcher at the Institute of Radiopharmaceutical Cancer Research at Helmholtz-Zentrum Dresden-Rossendorf (HZDR). Her work focuses on the development of novel bispidine chelators for radiopharmaceutical applications and multimodal imaging. As part of the MHELTHERA project, she was working on the detection of activated platelets at the Alfred Hospital and Monash Biomedical Imaging (MBI) in Melbourne, Australia. |
 Manja Kubeil | Manja Kubeil studied chemistry and received her Ph.D. degree from the Technische Universitat Dresden in 2014. In 2015, she was awarded with a Marie Curie International Outgoing Fellowship to undertake her postdoctoral research at the Monash University in Australia. In 2019, she became topic group leader at the Institute of Radiopharmaceutical Cancer Research at Helmholtz-Zentrum Dresden-Rossendorf (HZDR) in Germany. Since 2025, she is the head of Radiation Research on Biological Systems department at the Institute of Resource Ecology at HZDR. Her research focuses on radiopharmaceuticals for theranostic applications and radiation research to study the effects of radionuclides in living systems. |
 Michael Bachmann | Michael Bachmann Ph.D. in pharmacy (summa cum laude, Johannes-Gutenberg-University, Mainz, Germany), Professor of Physiological Chemistry, member of the Arthritis and Rheumatism Program (OMRF, OKC, U.S.A.), University Professor Medical Faculty (Technische Universität, Dresden, Germany), (i) tumourimmunology, (ii) Translational Radiopharmacology, and (Managing) Director, of the Institute of Radiopharmaceutical Cancer Research, at the Helmholtz Zentrum Dresden Rossendorf. Selected prizes: Boehringer-Ingelheim, Cell Biology, Bruno-Schuler (Rheumatology), Robert Feulgen (Int. Society of Cyto- and Histochemistry). Research focus: Radio-immunotheranostics for modular targeting systems (UniCAR, RevCAR, UniMAb). Current Research projects: Monash-Helmholtz Laboratory for Radio-Immuno-Theranostics (MHELTHERA), Ph.D. Networks: Oncoprotools, and Tolerate (European Union's Horizon Research and Innovation program). |
 Philip C. Andrews | Phil Andrews is the Head of the School of Chemistry at Monash University, Australia. After completing his PhD in 1992 at the University of Strathclyde, Glasgow, he held prestigious fellowships, including a Royal Society Fellowship at Griffith University and an Alexander von Humboldt Fellowship at Philipps Universität, Marburg. Joining Monash in 1995 he received an ARC QEII Fellowship, and is a Fellow of the Royal Society of Chemistry and the Royal Australian Chemical Institute. A founding member of the Monash Centre to Impact AMR, his research focuses on the discovery of metal compounds and materials as antimicrobials and anti-cancer agents. |
 Holger Stephan | Holger Stephan studied chemistry and received his PhD degree from the Technical University Bergakademie Freiberg in 1989. He held positions in industry and worked as Senior Scientist at the Technical University Dresden. In 1997, he moved to the Research Centre Rossendorf and was head of the working group “Nanoscalic Systems” at the Institute of Radiopharmaceutical Cancer Research of the Helmholtz-Zentrum Dresden-Rossendorf. He is currently working as the coordinator of the Monash-Helmholtz Laboratory for Radio-Immuno-Theranostics (MHELTHERA). His research focuses on the development of radiometal complexes, polynuclear metal compounds with photosensitizing and radiation-sensitizing properties, nanomaterials for multifunctional imaging and treatment of cancer. |
1. Introduction
Bismuth and many of its compounds are of great interest for medical applications due to their low toxicity.1 This behaviour is particularly influenced by the stabilising ligands, the dosage form and the administered concentration.2 The solubility of most bismuth compounds is low even in the acidic environment of the stomach and thus an almost complete elimination via the gastrointestinal tract is considered a crucial factor for low toxicity in vivo.3 This has driven forward, in particular, the development of antimicrobial and antiparasitic metallodrugs, such as bismuth subsalicylate, colloidal bismuth subcitrate or ranitidine bismuth citrate.4–6 Interactions with iron-containing proteins such as transferrin and lactoferrin as well as with various enzymes such as ureases, hydrogenases and ATPase are discussed as the underlying mechanisms of the biological activity of bismuth compounds.7 The specific interactions of bismuth compounds with biologically active molecules has also initiated studies of the anticancer activity of both small and nanoscale materials.8–12 With regard to small bismuth-based compounds, complexes with halide, nitrate, carboxylate and dithiocarbamate ligands as well as various heterocyclic compounds dominate. The majority of the investigations are limited to in vitro studies on cell toxicity.13–27 In contrast, in vivo studies in connection with bismuth complexes that show anticancer activity are only just beginning.28,29
This also applies to the structural elucidation of the bismuth complexes used in solution. For example, bismuth complexes with oxido ligands tend to form larger clusters, linking them to nanoscale bismuth-based systems, which are being actively investigated for cancer imaging and therapy.2,30–34 These new materials reveal great prospects in terms of improved imaging based on X-ray computed tomography (CT),35 photoacoustic imaging (PA),36 and infrared thermography (IRT),37,38 as well as efficient treatment options using in particular photothermal therapy (PTT),36 photodynamic therapy (PDT)39 and radiotherapy (RT).40,41 With regard to the latter, i.e. the application of external ionising radiation, there are promising developments to improve the treatment effectiveness of tumours with the help of bismuth-containing radiosensitisers.42 In contrast, radionuclide therapy (internal radiotherapy) using radioactive bismuth nuclides is still in its infancy.43,44 The increasing availability of the alpha-emitting nuclides 212Bi and 213Bi opens up a wide range of possibilities. This includes the use of radioactively labelled bifunctional chelating agents equipped with biological vector molecules and antibodies (BCFAs). There are new BFCAs with improved properties as well as selective and efficient coupling strategies, which opens up new opportunities for active targeting and the use of new radio-immunotherapeutic approaches. In addition, there is a rapid development in the field of bismuth-containing nanomaterials that are suitable for use in the combination therapy of cancer. This requires very defined particles in terms of size and morphology as well as the use of highly stable dispersions.
In this perspective article, suitable synthetic strategies for bismuth-containing materials will be discussed, which may allow a transfer to clinical use (Fig. 1). Furthermore, the state-of-the-art in the development of bismuth chelators for targeted alpha therapy is summarised and selected examples of radiolabelled target-seeking bioconjugates and nanomaterials are presented. New immunotherapeutic strategies are discussed that allow, on the one hand, effective recognition of tumours at an early stage and, on the other hand, various types of combination therapy for tumour treatment.
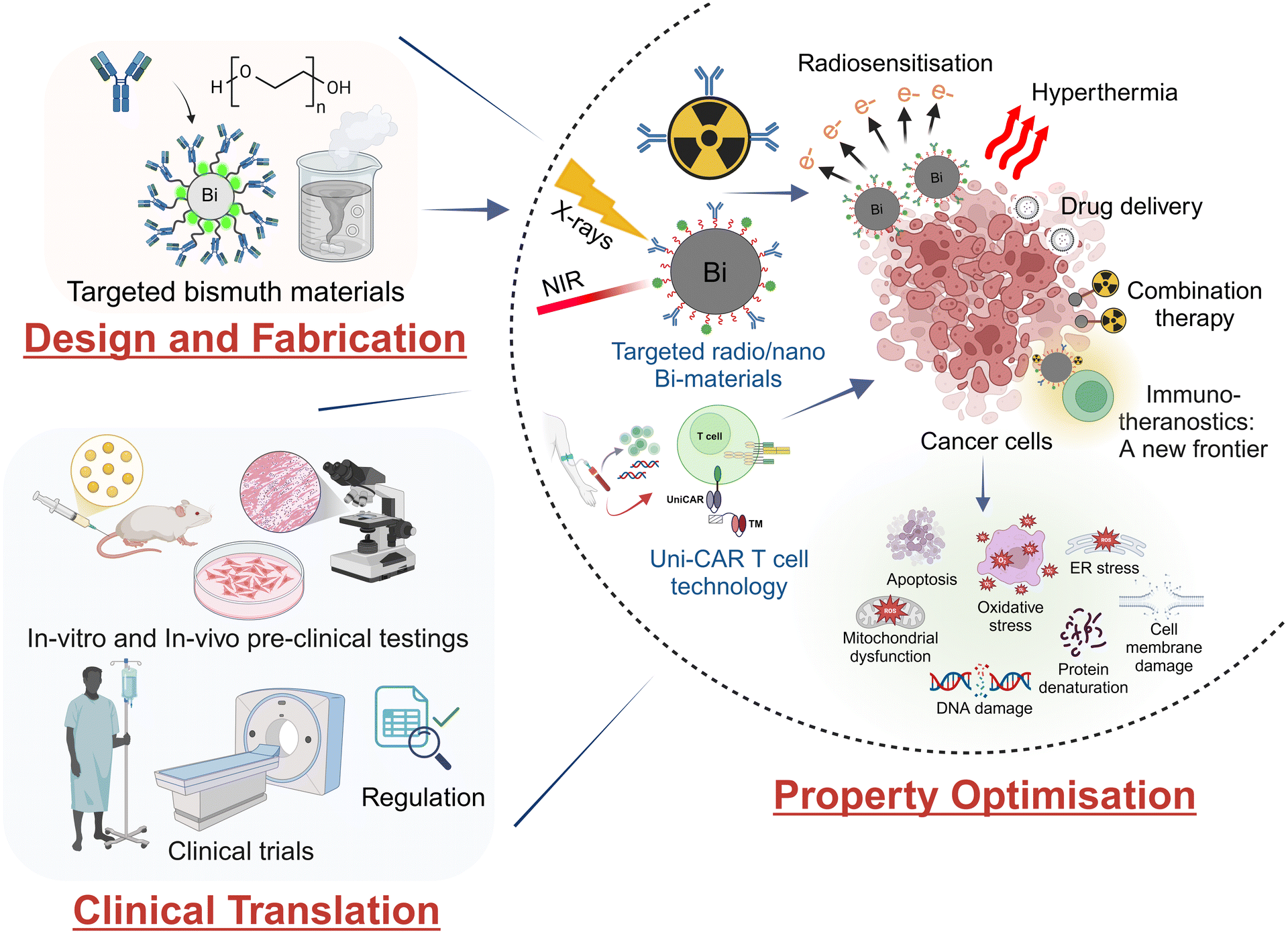 |
| Fig. 1 Development and characterisation of bismuth materials for imaging and treating cancers with a view to clinical use. | |
2. Bismuth materials: synthesis strategies and cancer applications
The synthesis of defined bismuth materials with high stability is difficult and challenging. In general, three classes of bismuth-containing materials can be distinguished, namely metallic nanoparticles (NPs), bismuth chalcogenides and bismuth-containing hybrid materials. With regard to clinical use, materials with high biocompatibility and suitable surface functionalisation are required for biological targeting. In this perspective, the following chapter summarises various synthesis strategies for clinically relevant bismuth compounds and discusses selected examples of their use in cancer imaging and therapy.
2.1. Metallic bismuth nanoparticles
Owing to their unique properties, metallic bismuth particles have become very important.45–48 The core characteristics of these particles are highly dependent on their crystal structure, size, shape and morphology, which can be tailored via various synthesis techniques.49–51 These are essentially reduction methods, laser ablation, and thermal decomposition techniques. These methods can lead to particles with different size distributions and must be combined with suitable coating strategies for stabilisation in the complex biological environment.52–54
Synthesis strategies that use chemical reducing agents are the most commonly used methods for obtaining metallic bismuth materials. Reductive synthesis methods in the presence of stabilising molecules usually result in well-defined particles. For example, the reduction of Bi(NO3)3 using sodium borohydride in the presence of the biomacromolecules gelatin (GEL), bovine serum albumin (BSA) or human serum albumin (HSA) at 37 °C, was reported to form stable spherical particles with a narrow particle distribution, i.e., the size range was 15.6 ± 3.1 nm for Bi@GEL, 17.4 ± 3.3 nm for Bi@BSA and 18.9 ± 3.8 nm for Bi@HSA, respectively.55 Further, the cytotoxic anticancer drug doxorubicin (DOX) was incorporated, which allows for chemotherapeutic application. These biomacromolecule-stabilised metallic Bi-NPs have appropriate properties for theranostic applications, i.e., they are suitable for CT and infrared thermal imaging (IRI) as well as for combination therapy based on chemo- and photothermal therapy.55 Metallic Bi-NPs can also be stabilised with cellulose derivatives.56,57 Chemical reduction using NaBH4 yields very small particles (2–10 nm), while reduction using UV light results in larger particles 25–55 nm. The use of reducing bacterial cultures such as Delftia also enables the production of metallic Bi-NPs, albeit with relatively large particle sizes (40–120 nm).58 Synthesis processes based on green chemistry are becoming increasingly important.59,60 In this context, a promising way is to use biocompatible compounds as reducing agents. Ouyang et al. fabricated Bi-NPs from BiCl3 using ethylene glycol as the reducing agent.61 The metallic Bi-NPs obtained were coated with gold and doxorubicin was incorporated. These Bi@Au particles exhibit excellent blood compatibility, a high photoconversion efficiency (η = 46.6%) and are very effective in synergistic chemo-photothermal therapy proven in an A549 tumour-mice model. However, these particles are quite large at >100 nm. The use of viscous solvents like ethylene glycol slows the diffusion of reactants, which can lead to uneven nucleation and growth of particles.62,63 There are a number of other Bi-NPs with sizes above 100 nm that exhibit good biocompatibility due to suitable surface functionalisation such as PEGylation, phospholipids and silica shells.64,65 Smaller particles are better suited for biomedical applications, especially for clinical use.66 One very interesting approach is the use of 45 nm-sized Bi-NPs, which have a SiO2 shell for the incorporation of an H2O2-responsive prodrug.67 These particles have very good photothermal properties with a high photoconversion efficiency (η = 46.3%) using near IR light at 808 nm. After intra-tumoural injection of these particles, photothermal ablation in combination with sequential X-ray irradiation allows for an improved therapeutic efficacy of >97%. Most of the Bi-containing particles currently used take advantage of passive targeting based on the enhanced permeability retention (EPR) effect.68–70 The utilisation of active targeting with the help of biological vector molecules is only just beginning. In particular, specific peptides such as AR (androgen receptor) and LyP-1 as well as folate ligands are used for tumour targeting.65,71–73 An interesting approach is to use the cancer cell membranes for targeting.74
To achieve ultrasmall particles, i.e. with sizes <6 nm, chemical reduction methods are also suitable if the appropriate reaction conditions are applied.75,76 Lei et al. report a very fast (1 min) preparation method using Bi(NO3)3 as starting material, NaBH4 and polyvinylpyrrolidone (PVP) as stabiliser, resulting in rapid generation of ultrasmall uniformly sized spherical Bi-NPs with a narrow size distribution (2.7 ± 1.1 nm).75 It is worth mentioning that these Bi nanodots produce a higher CT contrast than the iodine-based contrast agent iobitridol. In vivo dual-modal CT/photothermal-imaging-guided therapy was demonstrated in a U14 tumour-mice model.75 Similarly, very small Bi-NPs (3.6 nm) were obtained by reducing bismuth acetate in a mixture of oleic acid and oleylamine.72 These primary particles were stabilised with a PEG and phospholipid shell and the nonapeptide LyP-1 is attached to the surface. The particles had a hydrodynamic diameter of 12 nm in aqueous solution and enabled dual imaging based on CT and PA. Moreover, a combination of NIR-laser and X-ray irradiation caused very effective tumour treatment (Fig. 2).
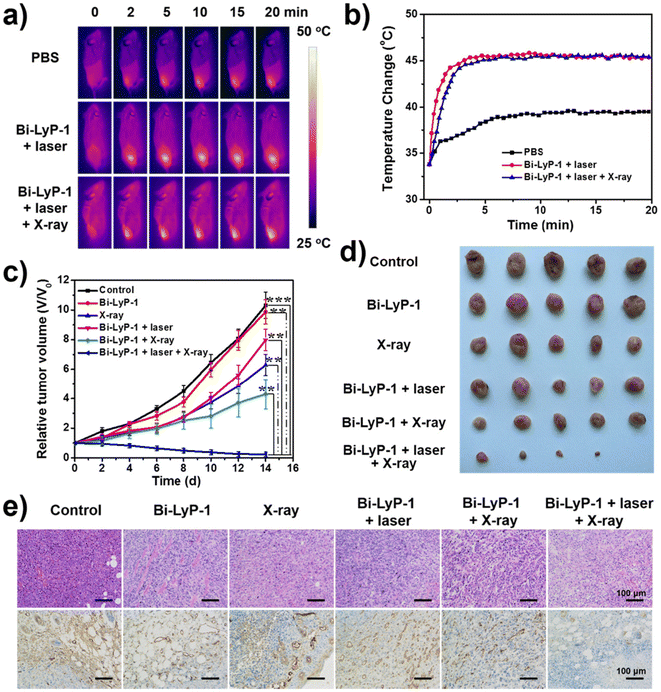 |
| Fig. 2 Tumour treatment with Bi-NPs attached with the LyP-1 peptide (Bi-LyP-1 NPs). (a) IR images of 4T1 tumour bearing mice injected with Bi-LyP-1-NPs using 1064 nm laser irradiation (0.6 W cm−2). (b) Temperature variation curves of tumour surface after NP's injection. (c) Tumour growth in each group under different treatments (d) Recorded images of tumour growth in different groups post 14 days. (e) H&E (upper row) and CD31 staining (bottom row) of tumour sections using different conditions (reprinted with permission from ref. 72, Copyright 2017 American Chemical Society). | |
An elegant method of obtaining metallic particles is laser ablation, in which a high-intensity laser is used to generate NPs from a solid target.77 Bulmahn et al. synthesized colloidal stable and pure Bi-NPs via femtosecond laser ablation of a metallic bismuth target. The results showed that metallic particles were only obtained in organic solvents like acetone, leading to spherical, primary Bi-NPs with narrow size distribution (28 ± 4 nm). In contrast, synthesis in water provides rather large nanosheets (455 ± 50 nm), consisting of bismuth subcarbonate. Colloidal stable aqueous solutions of metallic bismuth particles can be obtained in the presence of Pluronic® F68 as stabilising agent. However, DLS experiments show a broad particle distribution in the range of 50 to 150 nm. The metallic Bi-NPs showed efficient production of heat after irradiation with 808 nm NIR light, demonstrating their potential as promising photothermal agents for biomedical applications. Overall, laser ablation is a promising method for producing metallic bismuth particles, with the optimisation of various parameters such as laser energy, choice of solvent and stabilising compounds, allowing a wide range of particle size adjustment.78
The thermal decomposition of suitable bismuth compounds is another preparation route to produce metallic Bi-NPs. It should be noted that reduction processes also occur simultaneously with the solvents used. The decomposition methods at high temperatures have several benefits, including precise control over NP size and shape by suitable temperature regimes, which allows for the adjustment of a well-defined crystal structure and fabrication of particles with narrow size distribution.79 Thus, mono-dispersed spherical Bi-NPs (size ∼ 4 nm) were obtained with high yield in a one-pot synthesis approach by using bismuth neodecanoate as precursor in octadecene/oleic acid solution at 260 °C.79 These Bi-NPs can be stabilised with PEG-NH2 in phosphate buffered solution, giving narrow-sized particles smaller than 10 nm. The Bi@PEG-NPs were biocompatible as demonstrated by their haemolytic tolerance and cell-viability assessment on L929 cells. Furthermore, these metallic particles show promising contrast (CT) and fluorescence properties that predestine them for in vivo imaging studies. Biodistribution experiments using ICP-MS analysis in BALB/c mice revealed accumulation in large intestine, liver, spleen and lungs accompanied by a rather slow clearance over 7 days. This behaviour is rather atypical for such ultrasmall particles and indicates a lack of stability in vivo.80
The table below summarises the composition of metallic bismuth nanoparticles, the corresponding manufacturing methods and conditions, as well as sizes, and provides an overview of possible applications in the imaging and therapy of cancers (Table 1).
Table 1 Summary of metallic Bi-NPs with varying surface functionalization, their synthesis methods and corresponding characterisation and applications
NPs composition |
Synthesis method |
System |
Size [nm] |
Application |
Ref. |
BSA, bovine serum albumin; CT, computed tomography imaging; DLPC, 1,2-dilauroyl-sn-glycero-3-phosphocholine; DOX, doxorubicin; DSPE, 1,2-distearoyl-sn-glycero-3-phosphoethanolamine; HSA, human serum albumin; IRT, infrared thermography; PA, photoacoustic imaging; PEG, polyethylene glycol; Pluronic®F68, polyoxyethylene/polyoxypropylene block copolymer; PTT, photothermal therapy; RBC, red blood cell; TGA, thioglycolic acid; UV, ultraviolet. |
Bi@gelatin |
Reduction |
Bi nitrate |
15.6 ± 3.1 |
CT/IRT imaging |
55
|
Bi@BSA Bi@HSA |
NaBH4 |
17.4 ± 3.3 |
Chemo/PTT (tumour-bearing nude mice) |
Doxorubicin |
18.9 ± 3.8 (TEM) |
|
Bi@nanocellulose |
Reduction |
Bi nitrate |
2–10 (TEM) |
RT (4T1 tumour-bearing mice) |
56
|
NaBH4 |
|
|
Nanocellulose-COOH |
|
|
Bi@cellulose |
Reduction |
Bi/K citrate |
25–55 (TEM/DLS) |
CT/PA imaging |
57
|
UV irrediation |
|
Chemo/PTT (4T1 tumour-bearing mice) |
Carboxymethyl-cellulose |
|
|
Doxorubicin |
|
|
Bi@delftia |
Reduction |
Bi nitrate |
40–120 (TEM) |
In vitro cytotoxicity (HT29 cell line) |
58
|
Delftia bacteria |
|
|
Bi@Au |
Reduction |
BiCl3 |
>100 (DLS) |
Chemo/PTT (A549 tumour-bearing mice) |
61
|
Ethylene glycol |
|
|
Doxorubicin |
|
|
Bi@PPy-PEG |
Reduction |
Bi nitrate |
100–200 (TEM) |
CT/PA imaging |
64
|
Morpholine borane |
140–160 (DLS) |
PTT (tumour-bearing BALB/c mice) |
Polypyrrole (PPy)/PEG-dopamine coating |
|
|
Bi@SiO2 |
Reduction |
Bi nitrate |
65 (TEM) |
CT imaging |
65
|
Dodecanethiol |
160–250 (DLS) |
Targeted chemo/PTT therapy (MCF-7 tumour-bearing mice) |
Silica coating |
|
|
Doxorubicin |
|
|
AR peptide |
|
|
Bi@SiO2 |
Reduction |
Bi dodecanethiolate |
∼25 (TEM) |
IRT imaging |
67
|
Tri-octylphosphine |
45 (DLS) |
PTT/RT (4T1 tumour-bearing mice) |
Silica coating |
|
|
Redox-active prodrug |
|
|
Bi@Ag-PEG |
Reduction |
Bi nitrate |
>100 (TEM) |
IRT imaging |
71
|
Ag@PVP |
|
Chemo/PTT (A549 tumour-bearing mice) |
HS-PEG-folate |
|
|
Doxorubicine |
|
|
Bi@DSPE-PEG |
Reduction |
Bi acetate |
3.6 (TEM) |
CT/PA imaging |
72
|
Oleic acid |
12 (DLS) |
Targeted PTT/RT (4T1 tumour-bearing mice) |
Oleyl amine |
|
|
DSPE-PEG coating |
|
|
LyP-1 peptide |
|
|
Bi@DSPE-PEG |
Reduction |
Bi nitrate |
40 (TEM) |
Targeted RT (4T1 tumour-bearing mice) |
73
|
Morpholine borane |
56 (DLS) |
|
PEG2000-DSPE-folate coating |
|
|
Bi@DSPE-PEG |
Reduction |
Bi dodecanethiolate |
42 ± 2 (TEM) |
Targeted PTT (CT26 tumour-bearing mice) |
74
|
Oleyl amine |
|
|
Octadecene |
|
|
CT26 cancer cell membrane-coating |
|
|
Bi@PVP |
Reduction |
Bi nitrate |
2.7 ± 1.1 (TEM) |
CT/IRT imaging |
75
|
NaBH4 |
10.8 (DLS) |
PTT (U14 tumour-bearing mice) |
PVP |
|
|
Bi@DLPC |
Reduction |
Bi acetate |
47 ± 3 (TEM) |
CT/PA imaging |
81
|
Dodedanethiol |
162 (DLS) |
PTT (MDA-MB 231 tumour-bearing mice) |
Oleic acid |
|
|
Trioctylphospine |
|
|
DLPC coating |
|
|
Bi@RBC |
Reduction |
Bi nitrate |
196 ± 4 (DLS) |
RT/PTT (LLC tumour bearing mice) |
82
|
HNO3 |
|
|
PVP |
|
|
NaBH4 |
|
|
RBC membrane vesicles |
|
|
DSPE-PEG2000 |
|
|
Bi@Pluronic®F68 |
Laser ablation |
Bi target in acetone |
28 ± 4 (TEM) |
Photothermal properties |
77
|
|
50–150 (DLS) |
|
Bi@PEG-NH2 |
Thermal decomposition |
Bi-neodecanate |
4 (TEM) |
CT/fluorescence imaging |
79
|
Octadecene |
<10 (DLS) |
Biodistribution in BALB/c mice |
Oleic acid |
|
|
2.2. Bismuth chalcogenides
Bismuth chalcogenides (Bi2X3) constitute unique layered structures composed of bismuth (Bi) with chalcogen elements (X) such as sulfur (S), oxygen (O), selenium (Se), or tellurium (Te).83 It represents a class of materials, which are generally non-toxic in nature, inexpensive and highly stable.84 Due to their diverse features such as unique optical, thermal and electronic properties,85,86 bismuth chalcogenides have been exploited for a variety of applications like thermo-responsive electronics, photovoltaics, (bio)catalysis, sensing as well as cancer imaging and therapy.83,87–89 With regard to the latter, bismuth chalcogenides combine their structural diversity with a high degree of biocompatibility, efficient light-to-heat conversion in the NIR region and radio-enhancement abilities, leading to exceptional image-guided dual theranostic modalities.34,90,91 Moreover, suitable surface functionalisation and the introduction of biological vector molecules can lead to improved biocompatibility and increased tumour targeting, making them interesting for a wide range of biomedical applications.91,92
Hydrothermal methods are the most frequently used processes for producing stable Bi2X3 nanomaterials. In this process, bismuth precursors are reacted with chalcogen-containing compounds in an aqueous medium at elevated temperatures and suitable pressure. Depending on the conditions used, different sizes and shapes are obtained. Bi2S3-NPs are the most frequently pre-clinical studied bismuth chalcogenides in the imaging and therapy of cancers. A few selected examples are discussed below. The reaction of Bi2O3 precursors with thioacetamide (TAA) under pressure at elevated temperature and subsequent PEGylation leads to rather large, colloidal stable particles (>200 nm), which can be loaded with 3-bromopyruvate for enhanced radiosensitising. The resulting particles were shown to serve as efficient bimodal contrast agents for CT/PA imaging and demonstrate excellent X-ray sensitisation and NIR optical absorption properties, leading to high therapeutic efficacy against 4T1 breast tumours.93 An interesting approach is described by Poudel et al., which results in larger particles (173.5 ± 2.8 nm). Starting from Bi2O3 nanoshells, a hydrothermal synthesis under pressure in the presence of TAA is used to convert them into Bi2S3-based ‘nano-urchins’, which are loaded with indocyanine green (ICG). PEGylation is used for colloidal stabilisation and to introduce the anticancer drug methotrexate and folate units for targeting. In this way redox and photo-responsive targeted Bi2S3-NPs can be produced for simultaneous use in chemotherapy, PDT and PTT.94 Bi2S3 nanorods (60 × 130 nm) were produced by hydrothermal synthesis and stabilised in an aqueous environment using PVP. These particles were then coated with mesoporous silica, loaded with doxorubicin and equipped with the antibody trastuzumab for targeting. The nanorods have excellent contrast and photothermal properties and enable the application of targeted, effective cancer therapy based on chemo- and PTT.95 Overall, colloidally stabilised Bi2S3-NPs of this size are subject to the problem of a long residence time in the body.
Bi2O3, Bi2Se3 and Bi2Te3-NPs are also accessible via hydrothermal routes and exhibit interesting photothermal and radiosensitising properties.96,97 80 nm-sized Bi2O3-NPs are obtained by hydrothermal synthesis in the presence of Triton X and chitosan as a coating agent. These particles are loaded with the photosensitiser 5-aminolevulinic acid (5-ALA) and the anticancer agent curcumin. These particles can be used for CT imaging. Initial investigations into the therapeutic efficacy of chemotherapy and radiotherapy show effects, but these still need to be optimised.91 Very small 6 nm-sized bismuth ferrite NPs were synthesised via a facile hydrothermal method. After coating with poly(acrylic acid), these particles can be applied for PTT/PDT combination therapy.98 An elegant method that, in the presence of selenocysteine, leads to well-defined, 15 nm-sized Bi2Se3-NPs, is described by Du et al.99 These selenocysteine-modified NPs coated with PVP are highly biocompatible and show high therapeutic efficacy in vivo when radiotherapy and PTT are used simultaneously. It is worth mentioning that selenium can enter the bloodstream in parts and stimulate the immune system, as well as achieve a radioprotective effect throughout the body (Fig. 3).
 |
| Fig. 3 Schematic illustration of the therapeutic principle based on Bi2Se3@PVP nanoparticles, containing selenocystein (Sec) for simultaneously synergistic photothermal/radiotherapy of tumour and enhancement of the immunity to reduce the side effects of radiation. (Reprinted with permission from ref. 99, Copyright 2017, WILEY-VCH Verlag GmbH & Co.) | |
The production of bismuth chalcogenides in organic solvents (solvothermal synthesis) instead of water usually results in smaller particles. Various shapes are available in this way. Bi2S3 nanorods,90,100–104 Bi2S3 nanosheets,105 Bi2O3 spherical particles,106,107 Bi2Te3 nanosheets108 and Bi2Te3 spherical particles109 have been generated using the solvothermal technique. The so-called polyol process yields relatively small homogeneous particles, for example Bi2O3 (40–50 nm), which can be stabilised with hyaluronic acid.110 These particles exhibit excellent contrast and radiosensitising properties and are being discussed for use in CT imaging-guided radiotherapy. Small Bi2O3-NPs (19.2 ± 6.5 nm) were produced in propylene glycol and then coated with a silica shell, which has terminal NH2 groups for conjugation of folic acid and the photosensitiser 5-ALA. These particles show high in vitro cell toxicity after UV irradiation and are being discussed for use in PDT.106 Silica-coated 100 nm large Bi2S3-NPs were produced via a surfactant-assisted solvothermal synthesis.111 The NPs were loaded with doxorubicin and RGD peptides were incorporated as biological vector molecules using a PEG linker. These particles can be used for dual imaging (CT/IRT) and show a high therapeutic efficacy based on combined chemotherapy and PTT. Bi2S3 nanorods were prepared in organic solution, then coated with a thermally retracted poly(acrylamide) based polymer, where the photosensitiser zinc protoporphyrin X has been linked. This system shows promising contrast (CT), photodynamic and photothermal properties, enabling the combination of PDT and PTT.104 Oleyl amine-capped 36 nm-sized Bi2Te3-NPs have been fabricated, stabilised in biological environment by phospholipid-PEG coating and then loaded with a Pt(IV) prodrug. These particles show a very high photoconversion efficiency (η = 48.7%), are suitable for dual imaging (PA/IRI) and enable effective combination therapy based on chemotherapy and PTT.109 Chen et al. report on an immunotherapeutic approach using sulphur-doped Bi2O3-NPs, which were produced in ethylene glycol and then stabilised with PVP.112 These NPs are loaded with the drug Ivermectin and induce a highly effective immunogenic cell death of tumours by combining PTT and a sonocatalytic therapy. Bi2Se3-NPs are also obtained by solvothermal synthesis and stabilised using PVP.113 These particles are then coated with polydopamine, loaded with doxorubicin and stabilised with HSA. These particles can be used for dual imaging (CT/IRT) and show a synergistic therapeutic effect when PTT and chemotherapy are applied in a tumour mouse model.
Over the last 10 years, biomineralisation methods have been used to produce bismuth chalcogenides. This method has been reported to generate Bi2S3 spherical particles with sizes generally below 50 nm, possessing good stability in complex biological environment and high biocompatibility.114–122,123 The treatment of bismuth precursors in the presence of BSA leads to defined Bi2S3-NPs and was first described by Wang et al. in 2016.120 The primary particles are smaller than 10 nm (TEM). However, DLS measurements show a size of around 40 nm. Accordingly, the accumulation of Bi2S3-NPs occurs not only in the tumour but also in the liver, spleen, kidneys and lungs, as shown by biodistribution studies with 99Tc-labelled particles. These particles are suitable for imaging based on CT and IRT. In vivo studies in a tumour mouse model show that a combination of radiotherapy and PTT can achieve complete tumour remission. In terms of a therapeutic application, BSA-stabilised Bi2S3-NPs are mainly used in radio-, chemo- and immunotherapy, or a combination of these.114,122,124,125 Curcumin, specific peptides such as triptorelin, folic acid and the immunoactive ganoderma lucidum polysaccharide (GLP) are used to increase the effectiveness of the therapy and for targeting. While the primary particles Bi2S3@BSA are rather small, with sizes around 10 nm, larger associates of around 100 nm are often found in the biological environment. An interesting approach for in vivo applications is the use of 3D-printed alginate scaffolds with Bi2S3@BSA-NPs.123 These implanted scaffolds show a high therapeutic efficacy in a tumour mouse model after X-ray irradiation and are discussed for post-surgical use to remove residual tumour cells in breast cancer patients. Ultrasmall Bi2Se3-NPs (3 nm) were obtained by biomineralisation and encapsulated in tumour cell-derived microparticles together with doxorubicin.126 These particles are ideal for image-guided tumour targeting with subsequent combination of chemotherapy and PTT.
The table below summarises the composition of bismuth chalcogenide-based nanoparticles, the corresponding manufacturing methods and conditions, as well as sizes, and provides an overview of possible applications in the imaging and therapy of cancers (Table 2).
Table 2 Summary of Bi2X3-NPs with varying surface functionalization, their synthesis methods and corresponding characterisation and applications
NPs composition |
Synthesis method |
System |
Size [nm] |
Application |
Ref. |
5-ALA, 5-aminolevulinic acid; APTES, 3-aminopropyltriethoxysilane; BFO, bismuth ferrite; BSA, bovine serum albumin; CT, computed tomography imaging; CTAB, cetyltrimethylammonium bromide; Cur, curcumin; DOX, doxorubicin; FA, folic acid; GLP, ganoderma lucidum polysaccharide; HSA, human serum albumin; ICG, indocyanine green; IRT, infrared thermography; mPS, mesoporous silica; MTX, methotrexate; PA, photoacoustic imaging; PDA, polydopamine; PDT, photodynamic therapy; PEG, polyethylene glycol; PM, platelet membrane; Pt, platinum; PTT, photothermal therapy; TEOS, tetraethyl orthosilicate; US, ultrasound. |
Bi2O3@Chitosan |
Hydrothermal |
Bi nitrate |
80 (TEM) |
CT imaging Chemo/RT (4T1 tumour bearing mice) |
91
|
Triton-X |
204 (DLS) |
|
Chitosan |
|
|
5-ALA and curcumin loading |
|
|
Bi2S3@PEG-SH |
Hydrothermal |
Bi2O3 |
260 (DLS) |
CT/PA imaging |
93
|
Thioacetamide |
|
RT (4T1 tumour-bearing mice) |
PEG-SH coating |
|
|
3-Bromopyruvate loading |
|
|
Bi2S3@(ICG)-PEG-S-S-MTX |
Hydrothermal |
Bi2O3 nanoshells |
173.5 ± 2.8 (DLS) |
Targeted Chemo/PTT/PDT (HCT116 tumour bearing mice) |
94
|
Thioacetamide |
|
|
ICG loading |
|
|
SH-PEG-S-S-MTX |
|
|
Bi2S3@PVP(SiO2) |
Hydrothermal |
Bi nitrate |
Diameter 26 |
CT imaging |
95
|
Ethylene glycol |
Length 100 (TEM) |
Targeted Chemo/PTT (SKBR-3 tumour bearing mice) |
Sodium sulfide |
|
|
PVP (SiO2) |
|
|
Trastuzumab |
|
|
DOX loading |
|
|
Bi2O3@Fe-PAA |
Hydrothermal |
Bi nitrate |
6 (TEM) |
PTT/PDT (HepG2 tumour-bearing mice) |
98
|
Fe nitrate |
69.6 ± 1.6 (DLS) |
|
Poly(acrylic acid) |
|
|
Bi2Se3@PVP |
Hydrothermal |
Bi nitrate |
15 ± 3 (TEM) |
PTT |
99
|
PVP |
29.2 (DLS) |
RT (Bel-7402 tumour-bearing mice) |
L-Selenocystine |
|
|
Bi2S3@PAA |
Solvothermal |
Bi neodecanoate |
∅ 13 ± 2 |
CT/IRT imaging |
104
|
Thioacetamide |
l = 40 ± 5 (TEM) |
PTT/PDT therapy (4T1 tumour bearing mice) |
Oleic acid |
|
|
Oleyl amine |
|
|
PAA coating |
|
|
Protoporphyrin IX |
|
|
Bi2O3@SiO2-NH2 |
Solvothermal |
Bi nitrate |
19.2 ± 6.5 (TEM) |
PDT (KB cell line) |
106
|
Propylene glycol |
|
|
APTMS functionalization |
|
|
5-ALA and folic acid conjugation |
|
|
Bi2Te3@DSPE-PEG |
Solvothermal |
Bi neodecanoate |
36 (TEM) |
PA/IRT imaging |
109
|
TrioctylP-Te |
|
Chemo/PTT (4T1 tumour bearing mice) |
DSPE-PEG-CH3 coating |
|
|
Pt(IV) prodrug loading |
|
|
Bi2O3@HA |
Solvothermal |
Bi chloride |
45 ± 0.6 (TEM) |
CT imaging |
110
|
NaOH |
47.4 (DLS) |
RT (tumour bearing mice) |
Diethylene glycol |
|
|
Hyaluronic acid |
|
|
Bi2S3@SiO2-NH2 |
Solvothermal |
Bi chloride |
100 (TEM) |
CT/IRT imaging |
111
|
Na2S |
120 (DLS) |
Targeted Chemo/PTT (UMR-106 tumour bearing mice) |
Ethylene glycol |
|
|
CTAC |
|
|
APTES |
|
|
DOX loading |
|
|
RGD peptide |
|
|
Bi2O3−xSx@PVP |
Solvothermal |
Bi nitrate |
30 (TEM) |
PTT |
112
|
Ethylene glycol |
130 (DLS) |
Sonocatalytic therapy (4T1 Balb/c female mice) |
NH4F, Na2S |
|
|
PVP |
|
|
Ivermectin |
|
|
Bi2Se3@PDA |
Solvothermal |
Bi nitrate |
104 (TEM) > 400 (DLS) |
CT/IRT imaging |
113
|
Na2SeO3 |
|
Chemo/PTT (HeLa tumour bearing mice) |
Ethylene glycol |
|
|
PVP, PDA |
|
|
DOX loading HSA |
|
|
Bi2S3@BSA |
Biomineralisation |
Bi nitrate |
<10 (TEM) |
CT imaging |
114
|
BSA |
170 (DLS) |
RT (4T1 tumour bearing mice) |
FA coupling |
|
|
Curcumin loading |
|
|
FITC labeling |
|
|
Bi2S3@BSA |
Biomineralisation |
Bi nitrate |
6.1 ± 0.9 (TEM) |
CT/IRT imaging |
120
|
BSA |
40 (DLS) |
PTT/RT (4T1 tumour bearing mice) |
HNO3 |
|
|
NaOH |
|
|
Bi2S3@BSA |
Biomineralisation |
Bi nitrate |
15 ± 4 (TEM) |
In vitro Chemo/RT (HT-29 cells) |
122
|
HNO3 |
100 (DLS) |
|
NaOH |
|
|
BSA |
|
|
Curcumin |
|
|
Bi2S3@BSA |
Biomineralisation |
Bi nitrate |
8.5 ± 1.5 (TEM) |
In vitro RT (MCF-7 cells) |
124
|
BSA |
17 ± 2 (DLS) |
|
Triptorelin |
|
|
Bi2S3@BSA |
Biomineralisation |
Bi nitrate |
10 ± 3 (TEM) |
RT/immuotherapy (4T1 tumour-bearing BALB/c mice) |
125
|
HNO3 |
>130 (DLS) |
|
NaOH |
|
|
BSA |
|
|
GLP-SH |
|
|
Bi2S3@BSA |
Biomineralisation |
Bi nitrate |
21.6 ± 5.7 (TEM) |
RT (4T1 tumour bearing mice) |
123
|
BSA |
55 (DLS) |
|
Alginate |
|
|
Bi2Se3@BSA |
Biomineralisation |
Bismuth chloride |
3.0 ± 0.4 (TEM) |
CT/PA imaging |
126
|
Selenium powder |
13.5 ± 1.5 (DLS) |
Targeted Chemo/PTT (H22-tumour-bearing mice) |
Ethylenediamine |
|
|
Mercaptoethanol |
|
|
BSA |
|
|
Doxorubicin |
|
|
H22 tumour cell-derived microparticles |
|
|
2.3. Bismuth hybrid materials and nanocomposites
Bismuth-based hybrid materials and nanocomposites that consist of other inorganic or organic compounds and are equipped with target-seeking units are becoming increasingly important. Bismuth and bismuth compounds, when combined with other metals and metal compounds, organic materials such as stimuli-response polymers or carbon-based structures, provide novel functionalities and improved performances due to the synergistic interplay of the components, rendering nanohybrids/nanocomposites novel versatile functionalities.127–129 In the field of cancer research, on the one hand improved contrast properties can be achieved in imaging, while on the other, the range of treatment options for cancer can be expanded. Concerning the latter, emerging technologies for optimisation of targeted PTT, PDT, RT, hypoxia modulation, and combinatorial therapeutic regimes are in a state of rapid development.130–132
In the following, there are a few examples that show the diversity in this field and provide an insight into new fields of application. The combination of bismuth in its metallic form or various bismuth chalcogenides with metals such as Ag, Au, Pt and Pd leads in particular to improved contrast and radiosensitising properties, harnessing narrow band gaps and enables the use of highly effective sonodynamic therapy.133–137 By mixing bismuth chalcogenides with metallic Bi-NPs, the bismuth content can be increased compared to the chalcogenides alone, thus significantly boosting both the contrast properties and the effectiveness of PTT.138–141 Bismuth molybdate- and tungstate-based nanomaterials are gaining importance as sonosensitisers.142,143 Polyoxometalates (POMs) are an extremely interesting class of substances for cancer therapy.144,145 POMs have the great advantage that they can be produced in a very defined manner over a wide size range. However, the surface functionalisation is not trivial. Due to their radiosensitising properties, bismuth-containing POMs are of interest for improving the effectiveness of RT.146 New drug delivery systems based on biocompatible bismuth-containing metal–organic frameworks (MOFs) should also be mentioned.147 This field of research is only just beginning. However, it offers a wide range of biomedical applications. The blending of bismuth-containing materials with graphene oxide (GO) and reduced graphene oxide (rGO) is used to improve biocompatibility and for drug delivery. In addition, GO/rGO can be used to adjust the electrical and thermal conductivity of hybrid materials very precisely, thus enhancing imaging and radiosensitisation properties.127,148–151 In recent years, considerable work has been done on the development of hybrid materials with the addition of manganese oxide, magnetite and gadolinium oxide.116,152–158 This provides magnetic resonance tomography (MRT) as an additional imaging technique that is particularly suitable for good soft tissue contrast. With respect to its use in cancer therapy, the efficacy of hyperthermia can be increased and a hypoxia-related radioresistance can be overcome with these materials. An interesting inorganic–organic hybrid material is described by Zhang et al. in which metallic Bi-NPs are coated with silica and then stabilised with an organic polymer to which porphyrin loaded with iron(II) is covalently bound.159 This material can be used for combination therapy consisting of chemotherapy, PTT and PDT. Core–shell nanocomposites, consisting of ultra-small Bi2S3 nanoparticles, cerium oxide and the photosensitiser chlorin e6 have been developed for NIR-triggered PDT.160 Liu et al. reported a biodegradable and biocompatible core–shell bismuth-based hybrid material coated with a MnO2 layer and loaded with the anticancer drug docetaxel (DTX) to treat radio-resistant hypoxic tumours. The bismuth/manganese-based radiosensitising material has been stabilised with amphiphilic PEG equipped with folic acid as target-seeking groups (Fig. 4). For this targeted nanocomposite, an efficient CT/MR image-guided combination therapy based on chemotherapy, chemodynamic therapy and radiotherapy is described for a tumour mouse model.155
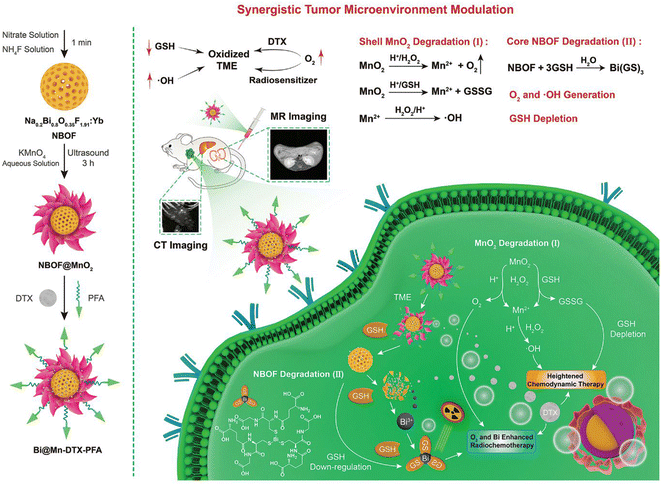 |
| Fig. 4 Schematic diagram of the synthesis process of Bi@Mn-DTX-PFA and its synergistic therapy mechanism for hypoxic tumours. (Reprinted with permission from ref. 155, Copyright 2021 John Wiley and Sons.) | |
3. Radiolabelled bismuth compounds
Radiolabelled compounds enable both imaging and the treatment of cancer. Bismuth offers two isotopes of interest for radiopharmaceutical applications. Both of them partially emit α-particles and decay via short-living daughter nuclides, which is expected to limit off-target accumulation.161 One promising candidate for targeted alpha therapy (TAT) is 212Bi, which decays via a branched pathway to 208Tl (t1/2 = 3.1 min, 36% α) and 212Po (t1/2 = 0.3 μs, 64% β−) and finally to stable 208Pb. It can be eluted from a 224Ra/212Pb/212Bi generator system as [212Bi][BiI5]2−/[212Bi][BiI4]−, either selectively or co-eluted with 212Pb. Due to the short half-life of 212Bi (t1/2 = 60.55 min), co-elution and subsequent use as a 212Pb/212Bi in vivo generator became of interest over the last few years.162,163 However, one major issue is the high energetic γ-line emitted by 208Tl (Eγ = 2.6 MeV), which can be problematic regarding dosimetry.164
The second isotope of interest is 213Bi (t1/2 = 45.6 min), one longer living daughter nuclide of 225Ac. It decays via209Tl (t1/2 = 2.2 min, 2% alpha) and 213Po (t1/2 = 3.7 μs, 98% beta) to 209Pb (t1/2 = 3.2 h) and ultimately to 209Bi (stable). It can be eluted from the respective 225Ac/213Bi generator as [213Bi][BiI5]2−/[213Bi][BiI4]−, but the separation from the other daughter nuclides 221Fr (t1/2 = 4.9 min) and 217At (t1/2 = 32.3 ms) can be challenging. A mixture of 0.1 M HCl/0.1 M HI was found to enable selective elution of 213Bi.164
The relatively short half-life of 212/213Bi can be advantageous for radiopharmaceutical applications, but some studies (e.g. biodistribution studies) can require measurements over a longer time. For that purpose, the β+-emitter 205/206Bi (t1/2 = 14.9 d and 6.2 d) can be used instead. While 206Bi decays directly to stable 206Pb, 205Bi decays via the long living daughter 205Pb (t1/2 = 1.7 × 107 years) to stable 205Tl.165–167205/206Bi can be obtained by irradiation of natPb with a proton beam for up to 7 h and selectively eluted from a Pb-SpecTM resin (18-crown-6-based resin) using HNO3.168
To ensure safe delivery of bismuth radioisotopes to the targeted tissue, a wide range of chelators (Scheme 1) have been developed with some already tested in vivo. One class of interest is acyclic chelators, which typically enable fast labelling kinetics under mild conditions. Prominent examples are DTPA (diethylenetriaminepentaacetic acid) and its derivatives, which were conjugated to a variety of proteins.40,169,170 However, some of the derivatives were found to be unstable, which led to the development of CHX-A′′-DTPA, in which a cyclohexyl moiety was installed in the backbone to provide rigidity and improve the stability.170 Other promising acyclic chelators such as H4Neunpa and H2ampa showed favourable labelling kinetics, high molar activities and provided high stabilities (pM = 26 for [Bi(ampa)]+).161,171
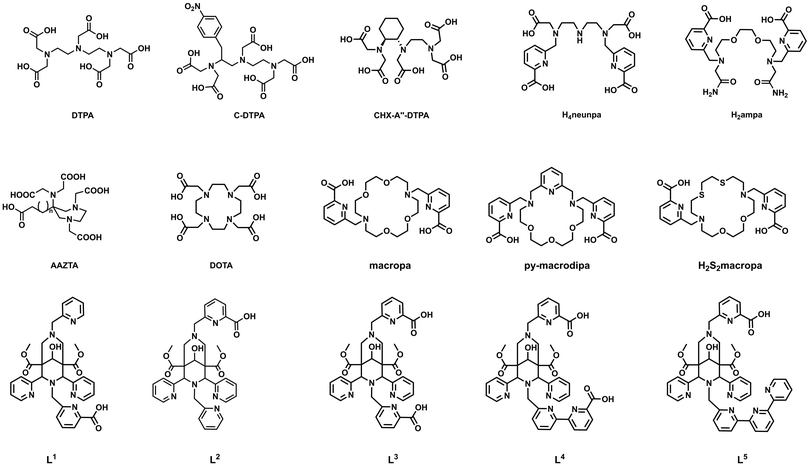 |
| Scheme 1 Chemical structures of acyclic and macrocyclic chelators for bismuth(III) discussed in this article. | |
Cyclic chelators generally form highly stable complexes, but the formation kinetics of the respective complex can be slow. The gold standard for a broad range of metals is DOTA (1,4,7,10-tetraazacyclododecane-1,4,7,10-tetraacetic acid), a cyclen-based chelator that undergoes continuous optimization to match the chemical requirements of different metals even better. The introduction of “soft” electron donors such as phosphonic acids was found to increase the thermodynamic stability of the complex and lead to higher radiolabelling efficiencies compared to the carboxylic acid bearing DOTA.172
Another subclass is based on the crown ether macropa (N,N′-bis((6-carboxy-2-pyridil)methyl)-4,13-diaza-18-crown-6), which is also known to form stable complexes and, in contrast to DOTA, forms complexes at milder reaction conditions. Especially py-macrodipa, a derivative with one oxygen donor replaced by a nitrogen and a pyridine ring attached to the backbone, showed efficient radiolabelling with 213Bi after 5 min at room temperature.173 Recently, it was shown that the introduction of sulphur donors into the macropa system reduces both the thermodynamic stability and the kinetic inertness of (radio)bismuth complexes.174
Characteristics of both chelator classes are combined in bispidines (3,7-diazabicylco[3.3.1]nonanes), which have a rigid backbone but flexible side arms to form stable complexes under mild conditions. Some octa- and nonadentate bispidines (L1–L5) were investigated and especially the nonadentate ones were found to be promising candidates for TAT.175,176
3.1 Radiolabelled small molecules, peptides and proteins
Targeted radiobismuth therapies have been applied with small molecules, peptides, antibody fragments, monoclonal antibodies, or any other kind of immunoproteins. They have shown promising results in pre-clinical and clinical studies in various tumour entities especially in small-volume or micro-metastasis such as breast cancer,177–179 bladder cancer,180 colon cancer,181,182 glioma,183,184 melanoma,165,171,185–188 multiple myeloma,189–191 non-Hodgkin lymphoma,192,193 ovarian cancer,194–196 pancreatic cancer and neuroendocrine tumours197–202 and prostate cancer.203 Of note, the first radiobismuth immunoconjugate (212Bi-CHIP) was studied in human pancreatic carcinoma cells (SHAW) in 1988.200 To this end, the results are summarized in other reviews,40,169,170 in which TAT showed success with locoregional and systemic administration. Herein, we only focus on recently published pre-clinical studies and highlight the clinical translations of radiolabelled bismuth conjugates.
3.1.1 Bladder cancer.
In a recent pilot bacillus Calmette Guèrin (BCG) therapy study, 12 patients with carcinoma in situ (CIS) were treated with [213Bi]Bi-CHX-A′′-DTPA-anti-EGFR-mAb by injecting 366–821 MBq via transurethral catheter. The pilot study proved that no severe side effects were observed in all patients. However, the authors addressed several strategies to improve therapeutic efficacy (3 out of 12 patients showed complete eradication after treatment).169
3.1.2 Glioma.
In an initial study, five patients with critically located gliomas were treated with the neurokinin type-1-receptor (NK-1) targeting conjugate [213Bi]Bi-DOTA-SP (substance P). Substance P, a small peptide with a weight of 1.8 kDa, showed high NK-1 affinity in the nanomolar range, which in turn was found to be selectively overexpressed in all malignant glioblastomas. Biodistribution studies were performed by co-injection of the 68Ga-labelled conjugate via an intratumoural catheter. One patient received 7.36 GBq of the conjugate over 4 therapeutic cycles, the rest was treated in one cycle with 1.07–2.00 GBq of [213Bi]Bi-DOTA-SP. All patients showed radionecrotic effects on the tumour, however the patient with the lowest dose administered showed only 50% reduction in tumour volume. No severe local or systemic toxicity was observed in any of the patients.204
A subsequent study with 50 patients was performed with [213Bi]Bi-DOTA-SP, where nine patients were diagnosed with secondary glioblastomas and the rest with different types of malignant gliomas. The median total dose administered over 1–6 cycles was 5.8 GBq and ranged between 1.4–9.7 GBq. The overall survival of patients who received [213Bi]Bi-DOTA-SP was extended to ten months in comparison to patients who underwent surgical resection only (overall survival of two months).205
In the latest glioma study, 20 patients with recurrent glioblastoma were treated with [213Bi]Bi-DOTA-SP (substance P) with a median injected activity of 3.3 GBq in total. The median overall survival of treated patients increased to 10.9 months after diagnosis of recurrence.183
3.1.3 Melanoma.
For metastatic uveal melanoma, [213Bi](BiNeunpa-Ph-Pip-Nle-CycMSHhex) targeting melanocortin 1 receptor (MC1R) was studied in vivo. Labelling of the conjugate was performed at room temperature at pH 5, achieving molar activities up to 21 MBq nmol−1 after 5 min of incubation. The novel bismuth complex showed high stability (pM = 27.0) as well as high chemical inertness in human serum over 2 h. The conjugate showed high tumour uptake in B16-F10 tumour xenografted mice (5.91 %ID g−1 1 h p.i.).171
The biodistribution of [205/206Bi]Bi-DOTA-IPB-NAPamide in B16-F10 tumour bearing mice was investigated with and without the albumin binder 4-(p-iodo-phenyl)butyryl (IPB) to prolong the circulation time and therefore increase the tumour uptake. Higher tumour uptake was observed for the IPB containing conjugate (4.50 %ID g−1vs. 3.14 %ID g−1), but high off-target accumulation in kidneys (8.78 %ID g−1) as well. Therefore, application of albumin binders for short-living radionuclides was found to be disadvantageous.165
In a first initial patient trial the efficacy of a radiobismuth labelled monoclonal antibody targeting melanoma receptors (melanoma-associated chondroitin sulfate proteoglycan) was conducted in 2005.186 Since the [213Bi]Bi-cDTPA-9.2.27 was injected intralesional, high doses of up to 49.95 MBq (1350 μCi) were tolerable with no accumulation found in the kidneys. The author highlighted that intralesional TAT can be used for inoperable secondary melanoma or primary ocular melanoma.
In 2007, 22 patients with stage IV melanoma/in-transit metastasis were treated intravenously with the same radiolabelled bismuth immunoconjugate (55–947 MBq) to establish an effective dose.187 A tolerable dose of 592 MBq with no renal damage was found. 20% of the patients showed partial or complete response (6% complete and 14% partially).
Building on the results gained from the phase I trial, another phase I study with 38 patients with end-stage metastatic melanoma was conducted by the same group in 2011.188 The study extended the factors which may influence overall survival by investigating the melanoma-inhibitory activity protein, age, gender, disease stage and lactate dehydrogenase. The maximum injected does of 925 MBq did not cause any severe effects and thus, the maximum tolerance could not be determined. The phase I trial showed an overall 50% response rate and has the potential to be used as a treatment option for end-stage metastatic melanoma cancer.
The group of E. Dadachova injected intraperitoneally [213Bi]Bi-CHX-A′′-DTPA labelled (3.7 MBq μL−1) mAb (8C3) targeting melanin alone and in combination with a standard drug for melanoma treatment (anti-CTLA4 mAb 9D9; 100 μg μL−1), into tumour-bearing melanoma C57BL6 mice.185 As an outcome, there was no synergistic effect observed when RIT was used in combination with immunotherapy. The authors justified it by the lack of anti-CTLA4 mAb efficacy in the murine model.
3.1.4 Myeloid leukemia.
A systemic α immunotherapy study with 18 patients suffering from myeloid leukemia were treated with 10.36–37.0 MBq kg−1 [213Bi]Bi-CHX-A′′-DTPA-lintuzumab (anti-CD33).206 Although an anti-leukemic effect could be measured in most of the patients, complete remission was not observed.
Eight years later the same group performed a phase I/II trial in 31 patients in combination with cytoreductive chemotherapy to test the maximum tolerated dose and antileukemia effect of [213Bi]Bi-CHX-A′′-DTPA-anti-CD33 (18.5–46.25 MBq kg−1).207 The authors claimed in both trials to administer alpha emitters with longer half-life to significantly enhance the therapeutic outcome.
3.1.5 Ovarian cancer.
For the treatment of metastatic ovarian cancer, a radiolabelled HER2-targeting single domain antibody fragment (a2Rs15d) was investigated. The overall survival rate was studied in SKOV-3 xenografted mice by intravenously injection of fractionated administration of [213Bi]Bi-DTPA-a2Rs15d (0.5–2 MBq) alone or in combination with trastuzumab.208 Synergistic effects led to an increased median survival by applying three times 0.5 MBq in combination with trastuzumab compared to the control group. Biodistribution data was gained from Cherenkov radiation (from β decay and β decay of its daughters) and SPECT/CT imaging. High accumulation was not only found in the tumour (4.9 ± 0.05 %ID g−1 after 15 min p.i.), but also in the kidneys (59.9 ± 5.1 %ID g−1 after 60 min p.i.). By co-injection of gelofusine the uptake was reduced by 50%. However, the severe kidney uptake currently hinders translation into the clinic.
3.1.6 Neuroendocrine tumours (NETs) and pancreatic cancer.
The conjugate [205/206Bi][Bi(AAZTA-C4-TATE)]− was obtained quantitatively with a chelator concentration of 10−5 M at pH 3 at room temperature after 5 min of incubation. The conjugate was found to be stable in PBS, 10 mM DTPA and human plasma for 21 h. In vivo experiments in AR42 tumour bearing mice showed higher tumour uptake compared to [213Bi][Bi(DOTA-TATE)]− (9.32 vs. 6.5 %ID g−1) and reduced kidney uptake (10.1 vs. 17.4 %ID g−1).197 One initial clinical trial was performed using [213Bi]Bi-DOTATOC in seven patients with NET pre-treated with 177Lu and 90Y and one with bone marrow carcinosis (Fig. 5). Successful treatment of all patients was achieved with even progression free survival up to 30 months after treatment.199
 |
| Fig. 5 (a) [68Ga]Ga-DOTATOC-PET image of a patient with a tumour in the liver and metastases in the bone marrow as shown in coronal and sagittal maximum intensity projections. (B) Reduced tumour burden in the liver after treatment with 10.5 GBq [213Bi]Bi-DOTATOC, as well as reduction of the bone metastases 6 month after treatment. (Reprinted with permission from ref. 199 Copyright 2014 Springer.) | |
Novel proteins belonging to the family of Cancer/Testis Antigen (CTA) were developed to address the highly aggressive and to this stage incurable pancreatic ductal adenocarcinoma.201 [213Bi]Bi-CHX-A′′-DTPA-labelled antibodies targeting CETN1 (centrin1) showed a tumour-specific uptake and effective treatment of intraperitoneally injected [213Bi]Bi-CHX-A′′-DTPA-labelled antibodies (single dose of 3.7 and 7.4 MBq) in MiaPaCa tumour-bearing mice. In comparison to the 177Lu-labelled injected counterpart (same dose), only the alpha labelled mAb showed a reduced tumour growth rate pointing out that RIT is more effective than radionuclide therapy (RNT).
3.1.7 Prostate cancer.
Initial dose calculations for [213Bi]Bi-DOTA-PSMA617 were performed based on [68Ga]Ga-DOTA-TATE PET images from three patients. The dosimetry was found to be reasonable, but off-target accumulation, especially in the kidneys, was found to be even higher than for the respective 225Ac or 177Lu conjugates.209
However, a first patient with metastatic castration resistant prostate cancer (mCRPC) was treated with 592 MBq [213Bi]Bi-DOTA-PSMA-617 given in two cycles. PSA levels were found to decrease from 237 μg L−1 to 43 μg L−1 eleven months post treatment (Table 3).210
Table 3 Overview over preclinically and clinically investigated radiolabelled small molecules and antibodies
Preclinical |
Substance |
Dose [MBq] |
Tumour uptake [%ID g−1] |
Cytotoxic effects [%ID g−1] |
Disease |
Ref. |
[205/206Bi]Bi-DOTA-IPB-NAPamide with and without 4-(p-iodo-phenyl)butyryl |
2.39 |
4.50 ± 0.98/3.14 ± 0.32 |
8.78% ± 3.61 in kidneys |
Melanoma |
165
|
[213Bi](Bi-Neunpa-Ph-Pip-Nle-CycMSHhex) |
0.1 |
5.91 ± 1.33 |
31.5 ± 4.99 in liver |
Melanoma |
171
|
[213Bi]Bi-CHX-A′′-DTPA-8C3 |
3.7 + 7.4 |
N/A |
N/A |
Melanoma |
185
|
[205/206Bi][Bi(AAZTA-C4-TATE)] |
1.18 |
9.32 ± 3.96 |
10.1 in kidneys |
NET |
197
|
[213Bi][Bi(DOTA-TATE)] |
16.8 + 33.1 |
6.5 ± 2.3 |
17.4 ± 2.2 in kidneys |
NET |
197
|
[213Bi]Bi-CHX-A′′-DTPA-labelled antibodies targeting CETN1 |
3.7 + 7.4 |
N/A |
N/A |
NET |
201
|
[213Bi]Bi-DTPA-a2Rs15d |
8.8 + 10.7 |
4.9 ± 0.05 |
59.9 ± 5.1 in kidneys |
Ovarian cancer |
208
|
Clinical |
Substance |
Total administered dose [MBq] |
Cytotoxic effects |
Disease |
Ref. |
[213Bi]Bi-CHX-A′′-DTPA-anti-EGFR-mAb |
366–821 |
— |
Bladder cancer |
169
|
[213Bi]Bi-DOTA-SP |
1400–9700 |
— |
Glioma |
183, 204 and 205 |
[213Bi]Bi-DOTATOC |
3300–20 600 |
Moderate hematological effects |
NET |
195
|
[213Bi]Bi-cDTPA-9.2.27 |
592–925 |
— |
Melanoma |
186–188
|
[213Bi]Bi-CHX-A′′-DTPA-lintuzumab |
1195–4755 |
10% treatment-related death |
Myeloid leukemia |
206 and 207 |
16% liver abnormalities |
[213Bi]Bi-DOTA-PSMA617 |
592 |
N/A |
Prostate cancer |
209 and 210 |
3.2 Radiolabelled nanostructures
There are only limited reports on radiolabelled bismuth nanomaterials. The short half-life of 212/213Bi hinders the feasibility of implementation. An indirect strategy is the use of 212Pb/212Bi as an in vivo generator.162
The first study was reported by Diener et al.211 They synthesised 212Pb-labelled endohedral fullerenes by recoil synthesis following α decay and were further functionalized with malonic acid. The biodistribution behaviour was investigated in non-tumour bearing mice. A high uptake in the liver (53.7 ± 7.1 %ID g−1) and spleen (27.2 ± 3.2 %ID g−1) could be observed 8 h post injection and pointed to slow clearence from the body. The authors claimed that 212Pb was encapsulated otherwise bone marrow uptake would have been observed.
Recently, ultrasmall silver telluride nanoparticles (∼2 nm) were coated with gluthathion and radiolablled with 212Pb without any chelator.212 The [212Pb]Pb-GSHAg2Te showed high stability (∼96% monitored by radio-TLC) in the presence of 1 mM EDTA after 24 h. However, these particles have not been investigated further neither in vitro nor in vivo.
One example of a 212Bi-labelled nanostructure is reported by Kauffman et al.213 They sucessfully labelled FDA-approved macroaggregated albumin (MAA) and investigated the conjugate in vitro and in vivo in breast cancer models based on 4T1 and EO771 cells. Reduction of tumour cell growth could be observed in both cell lines in vitro using clonogenic and survival assays. Biodistribution studies showed that after intratumoural administration, about 90% of the injected dose remains in 4T1 or EO771 orthotopic breast tumours in mice, resulting in a significant reduction in tumour size over a 18 day monitoring period.
4. Novel targeting/therapeutic strategies
Approaches to widen the spectrum of therapeutic conjugates by implementing a variety of molecules and nanomaterials have been provided over the years and attempts to create synergistic effects in combination with other treatment options are still ongoing. A combination of radiation therapies with immunotherapeutic approaches, in particular, appears to be highly promising.214 For example, beam irradiation or endogenous radiotherapies may result in the release of tumour associated antigens (TAA) from dying tumour cells.215 Uptaken by dendritic cells, TAAs can be processed and presented by the immune system, thereby, leading to the activation of immune effector cells. This may explain the observed vaccination effects of radiotherapies against tumour cells, including in patients. For example, even non-irradiated tumour tissues become recognized by the immune system as a consequence of radiotherapy, also known as abscopal effect.216 Besides vaccination, there is experimental evidence that irradiation of tumour tissues including by TAT217 can enhance the entry of immune effector cells into tumour tissues, which can modulate the immunosuppressive tumour microenvironment (TME) to more inflammatory conditions, thereby facilitating the recognition of tumour cells by the immune system.218–220 For these reasons it is expected that the combination of radiotherapy can improve immunotherapies including with bispecific antibodies (BsAb) or adoptive transfer of T cells genetically modified to express chimeric antigen receptors (CAR).189,221
Bearing in mind, (i) the above summarised potential applications of bismuth-based radiotheranostics on the one hand, and (ii) the promising data related to combined applications of radiotherapeutic strategies with immunotherapies on the other hand, novel bismuth-based immunotheranostics appear highly attractive and should therefore come into the focus of future pre-clinical and clinical evaluation. For these reasons, we include here an overview of such potential future combinatorial applications in the next chapter.
4.1 Immunotheranostics: history and future
Since the description of the magic bullets by Paul Ehrlich at the beginning of the 20th century,222 for decades experimental immunotherapeutic approaches were developed for the treatment of tumours. Since the underlying mechanisms were not well understood such treatments were not reliable and difficult to reproduce. Therefore, tumour immunology was even considered as “alternative medicine”. Even the development of monoclonal antibodies (mAbs), and later the detection of cellular immunity mediated by T-, NK and dendritic cells, did not lead to the anticipated major break-through of immunotherapies. The obvious drawback of murine mAbs is their weak interaction with both the human humoral and cellular immune effector mechanisms known as complement derived cytotoxicity (CDC), and antibody derived cellular cytotoxicity (ADCC). ADCC is mediated mainly by NK cells via the interaction of the Fc portion of the antibody with the Fc receptor expressed on immune cells. To overcome the limited cytotoxic capability of murine mAbs a variety of technologies were put forward, including for example the construction of immunodrug conjugates or radiolabelling of antibodies, as mentioned above. Limitations still remain today: the most obvious problem of full-size antibodies is their pharmacology. Antibodies having an Ig format stay within the blood stream for several weeks. Due to the high osmotic pressure, their entry into the tumour tissues is difficult and takes around 4 to 48 h. In addition, ADCC is inhibited by the immunosuppressive environment inside of tumours. One of the next steps to overcome this limitation of full-size natural antibodies was the development of smaller antibody derived fragments such as the single fragment variables (scFvs) using upcoming recombinant antibody technologies. Alternatively, natural occurring small antibodies, so called nanobodies (nbs), from camelides or sharks came in the focus as potential immunotheranostics. However, their foreign immunogenic character leads to neutralization due to an immune response in humans. Besides, the pharmacokinetic of nbs and scFvs is also not favourable for usage as immune drug conjugate, either for imaging or therapy, as they are rapidly eliminated via the kidney, usually within 15 to 30 min after application. For this reason, only a low tumour enrichment can be achieved. So even now, and in spite of the dramatic progress made, there is still significant room for improvement in antibody based humoral or cellular immune therapies.
The first real major breakthrough of antibody-based immunotherapies was the detection of Abs that can release the brakes of the immune system,223 which were termed as checkpoint inhibitors. The treatment of tumour patients with checkpoint inhibitors provided for the first-time convincing evidence for the tremendous potential of the humoral and cellular immune components to recognize and destroy tumour cells. In addition, it became obvious that tumour cells must have tricked the immune system and successfully circumvented all the obviously existing highly efficient natural immunological defence barriers when cancer is established in a patient.
Unfortunately, checkpoint inhibitors are only working against tumour cells that have collected manifold mutations during their evolution to become a tumour cell.224 Therefore, scientists try nowadays to reinstall the capability of the immune system via the development of more efficient immunotherapeutic treatment modalities based on vaccination, immunotheranostic compounds including BsAb, modified advanced nanomaterials, and most recently another major breakthrough using immune cells genetically modified to express chimeric artificial receptors (CARs).225
4.1.1 Overview on immunotherapeutic approaches.
Bispecific antibodies.
Bispecific antibodies (BsAbs) for tumour therapies are usually directed to a tumour associated antigen (TAA, e.g. CD19), which is overexpressed on the surface of a tumour cell, and also to an activating receptor (e.g. CD3) on the cell surface of an immune effector cell. BsAbs can be constructed from the variable domains of the heavy and light chains of two monoclonal antibodies (mAbs). The domains of both mAbs are recombinantly fused to form single chain fragment variables (scFv), which are then fused to form the BsAb. BsAb can cross-link T cells with target cells, which finally leads to the destruction of the tumour cell. A schematic overview is shown in Fig. 6 (upper panel).
 |
| Fig. 6 Construction and retargeting of immune cells with BsAbs (upper panel) and conventional CAR T cells (lower panel). ScFvs can be constructed from the variable domains of two mAbs and fused to a BsAb. Cross-linkage via the BsAb leads to the killing of the tumour cell by the redirected T cell. A conventional CAR consists of three portions, the extracellular domain targeting a TAA usually a scFv directed to a TAA, a transmembrane domain and intracellular signalling domains taken from activatory receptors of the T cell receptor complex. The gene encoding such an artificial receptor can be transduced into T cells. The resulting CAR T cells can recognize tumour cells via their extracellular antibody domain. The interaction with the target cell leads to the formation of an artificial immune synapse, which finally triggers the cell death of the tumour cell. | |
Conventional CARs.
Conventional CARs consist of three domains: (i) an extracellular antibody-based domain, usually in the scFv format (ii) a transmembrane domain, and (iii) intracellular signalling domains. Via the extracellular antibody domain CAR T cells can bind to the TAA on the surface of the tumour cell and destroy it (Fig. 6 lower panel).
For CAR T immunotherapy, T cells are isolated from the patient and transduced with the gene encoding the respective CAR construct. The resulting genetically modified CAR T cells are then adoptively transferred back into the patient.
The idea to equip T cells with an antibody based artificial receptor was already described at the end of the 1980s.226 However, it took decades of development until their more recent successful clinical application and first approvals by the respective legal authorities.
Although the CAR T cell technology remains extremely expensive, an impressive number of patients have been successfully treated with such individualised living drugs. While the positive outcome of CAR T cell treatments have underlined their high potential, their limitations have also become evident: activated CAR T cells strongly proliferate and produce high amounts of cytokines. As a consequence, CAR T cell treatments are associated with a high risk of severe life-threatening side effects, including cytokine release syndrome (CRS). In addition, OFF tumour On target effects can occur as the expression of the targeted TAA is usually not limited to the tumour cell but may also occur on the surface of healthy tissues.
Despite their side effects, the specificity of conventional CAR T cells is limited to just one target, which facilitates the development of tumour cell escape mechanisms.
Adaptor CARs.
To overcome these problems, we and others have developed modular CAR T cell treatment platforms, nowadays known as adaptor CAR T cells. Starting from a modular BsAb approach, which we termed the UniMAB system (Fig. 7, left panel),227 we have established two versions of adaptor CAR platforms which we term the UniCAR and RevCAR system (patents228–230) (Fig. 7, right panel).221,225 For both adaptor CAR platforms proof of functionality has not only been shown in pre-clinical in vitro and in vivo studies231,232 but also in currently running clinical phase 1 trials (UniCAR: NCT04230265; NCT04633148; RevCAR: NCT05949125).
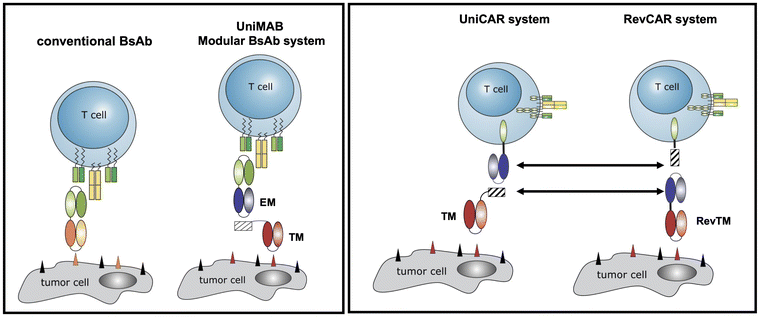 |
| Fig. 7 Originally, to accelerate the development of conventional BsAbs, we established the modular bispecific antibody platform UniMAB. For this purpose, we decided to split the BsAB into two components, an effector module (EM) and a targeting module (TM). The EM is a bispecific antibody, which recognizes on the one hand an activating domain of the immune cell (e.g. CD3) and on the other hand a peptide epitope. This epitope sequence is part of the TM. Thus, EM and TM can form an immune complex with properties similar to conventional BsAbs. This is the same strategy as we later used for our adaptor CAR platform technologies UniCAR and RevCAR. In contrast to conventional CARs, UniCARs do not recognize a TAA directly on the surface of a tumour cell but the same peptide epitope as the EM of the UniMAB system. Consequently, UniCAR T cells can establish an interaction with tumour cells via the same TMs used in the UniMAB system.221 The RevCAR system differs from the UniCAR system as follows: the scFv domain of the UniCAR is replaced by the peptide epitope and vice versa the epitope tag in the TM is replaced by the anti-peptide epitope scFv. | |
In contrast to conventional CAR T cells, which are permanently active after adoptive transfer of the genetically modified immune cells, the function of adaptor CAR T cells depends on the presence of a bridging molecule (target module, TM), which is required for the cross-linkage of the adaptor CAR T cell with the tumour cell (Fig. 7). This strategy allows a repeated stop and go treatment via regulation of the infusion of the respective TM (Fig. 8).
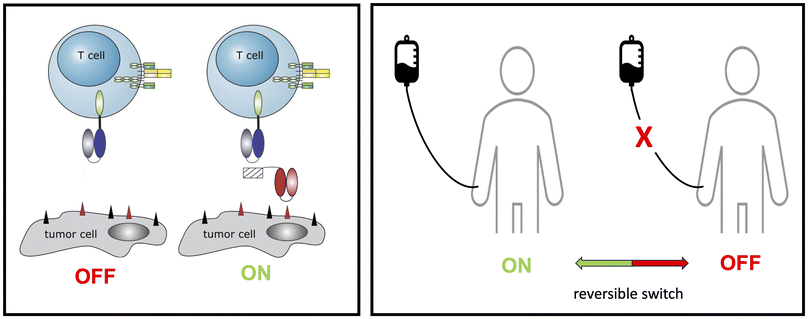 |
| Fig. 8 UniCAR (and RevCAR T cells) are inactive in the absence of a TM. They can be turned on by adding of the TM and turned OFF by elimination of the TM. In the clinical setting TMs are applied by continuous infusion. In case of side effects, the infusion can be stopped and thereby the function of the UniCAR T cells (RevCAR T cells) can be turned OFF. The adaptor CAR T cells can be restarted by further infusion of the TM. An additional advantage of adaptor CARs is the chance to apply an alternative TM with a different specificity in case tumour escape variants occur. | |
Obviously, the pharmacokinetics of the respective TM determines the efficiency and safety profile of adapter CAR T cells. TMs that can be rapidly eliminated allow a fast turning OFF of the adaptor CAR T cells. However, the downside is that their application requires a permanent infusion to achieve the TM concentration necessary for efficiency. During treatment, the patient is hospitilized at an intensive care unit, which is obviously not convenient for the patient. Vice versa, a TM having an extended half-life would facilitate the application for the patient but increases the risk of severe site-effects as adaptor CARs targeting TMs based on full size antibodies will behave more or less like non-regulatable conventional CARs.
For the UniCAR and RevCAR platforms, we have therefore developed a series of TMs having different pharmacokinetic profiles, which is achieved by the construction of molecules having different molecular weight.221 A revised treatment modality with UniCAR or RevCAR T cells would start with TMs having a short half live allowing a fast interruption of the T cell function if necessary. Once most of the tumour load has been destroyed and the risk of CRS becomes low, a TM having a long half live could be applied. Although feasible and working in in vitro and in vivo models, the need for different TMs would obviously enhance the cost for the development of the respective GMP grade TMs and would require additional clinical trials.
Conjugation of TMs with Bi-NMs may not only be useful for optimization of the pharmacokinetic properties of TMs but also for imaging of tumours and therapeutic treatments. Furthermore, radioactive versions could help to overcome the immunosuppressive tumour microenvironment.
5. Outlook and conclusion
Recent advances in cancer therapies, integrating metals to precisely target cancer cells for effective imaging and therapy, are transforming the modern therapeutics. Towards this goal, bismuth in its various forms, has garnered much attention as a non-toxic and highly stable metal with many fascinating properties. The clinical application of small bismuth complexes appears to be feasible as only minor side effects, if at all, were observed when these compounds were carefully applied.2,8 Kidney damage was only seen in the event of an overdose.233 Overall, the lower toxicity but also higher cost-effectiveness of bismuth-based materials compared to common precious metal-based anticancer compounds has led to an emerging rush towards commercialization. In the field of bismuth-containing nanomaterials, there are also promising developments that would justify clinical use. For example, the CT contrast of Bi-NMs is significantly higher than that of clinically approved iodine-based contrast agents, the photoconversion efficiency is very high and there are targeted materials available that achieve high tumour accumulation. However, to our knowledge, no clinical studies with Bi-NMs have been conducted to date. In the future, extensive clinical studies will be required here, with scientists, physicians and stakeholders of regulatory authority having to work closely together. In particular, the fabrication of nanomaterials under the conditions of good manufacturing practice (GMP) is a challenge. However, in the meantime, the art of synthesis in this field has progressed considerably.
At the nanoscale, bismuth is known for its effective radiosensitising and strong photothermal conversion properties as well as its remarkable performance as a strong contrast agent for various imaging modalities including CT, IRT, PA and MRI. In the field of imaging, ultrasmall particles (<6 nm) with appropriate surface functionalisation are becoming increasingly important because, they can reach all parts of the body and are quickly excreted by the kidneys.234 Additionally, Bi-NPs can be functionalised with various targeting agents and coating molecules to generate well-defined and site-targeted Bi-NPs for therapeutic applications on the basis of PTT, PDT and RT. Besides imaging, another highly attractive feature of Bi-based materials is based on the existence of radioactive bismuth compounds. Such radiobismuth compounds conjugated for instance with small molecules like peptides are highly promising radiopharmaceuticals including for endoradionuclide therapy.
With clinical applications in patients in mind, it is noteworthy to mention that the surface of Bi-NPs can be designed to prevent the formation of a protein corona and thus avoid off-target effects.235 Besides well-known biological vector molecules such as specific peptides and antibodies and their fragments, there is the possibility of using cancer cell membranes for targeting. A major problem with the introduction of new materials into the clinic still remains the dilemma of nanotoxicity.236 But with no doubt, nanomaterials and especially Bi-NPs may lead to promising novel treatment modalities for the imaging and treatment of otherwise fatal diseases for which global unmet medical needs exists. The currently remaining problems may hopefully be solved in the near future through interdisciplinary collaboration and partnerships across various disciplines.237
One example of a success story of interdisciplinary cooperation is currently the development of tumours therapies based on combinations of cytostatic drugs, radio-, and immunotherapies. Bearing in mind that local beam or endoradionuclide-based irradiation or the treatment with cytostatic drugs does not only lead to cell death but also alters the tumour microenvironment from immunosuppressive to inflammation, combinations of modern immunotherapeutic approaches based on BsAbs or CAR T cell technologies with well-established conventional tumour therapies appear very promising. Also, combinations with immunotheranostics and/or radiosensitisers based on advanced nanomaterials represent highly promising strategies to further improve the efficacy of future tumour immunotherapies. Due to the above summarized interesting features, bismuth-based nanoparticles may become of special interest and should therefore be tested in the near future in combination with BsAbs, modular BsAbs, CAR T cells, and the adaptor CAR T cell platforms UniCAR and RevCARs. TMs for adaptor CAR T cells could be constructed, consisting of Bi-NPs conjugated with both the antibody domain against a TAA and the peptide epitope recognized by an adaptor CAR T cell (Fig. 9).
 |
| Fig. 9 Multimodal (Bi based) NP can be constructed and conjugated with TMs to optimize their pharmacokinetic properties. Radioactively labelled TM versions could be used to modulate the tumour microenvironment from immunosuppressive to inflammation. Besides such Bi based NP could also be used for imaging of tumours and of the therapeutic effects: Bi-NPs based TMs could be established and equipped with additional features, for example via conjugation of small molecules or recombinant antibody derivatives to Bi-NPs, the pharmacokinetic property of a TM could be altered and fine-tuned. Radioactive versions of Bi-NP based TMs could directly modulate the tumour microenvironment from immunosuppressive towards inflammation as a prerequisite for attraction, invasion and activation of adaptor CAR T cells. | |
In summary, this perspective provides an overview of different types of Bi-NMs, such as metallic Bi-NPs, Bi-chalcogenides and Bi-hybrid structures that are relevant for clinical applications, and discusses key examples of colloidal stable, biocompatible and efficacious anti-cancer Bi-NPs and its related forms. Moreover, an overview of developments in radiobismuth-labelled compounds for targeted alpha therapy are presented, highlighting specific examples of radiolabelled bioconjugates and nanomaterials engineered for selective tumour treatment. A potential construction of Bi-NPs based TMs for multimodal usage in combination with adaptor CAR T cell technologies for cellular immunotherapy approaches of tumours are also presented. Using such Bi-NP based TMs may not only allow an optimisation of the pharmacokinetic properties of TMs but the modulation of the tumour microenvironment towards an improved attraction, invasion and killing efficacy of adaptor CAR T cells and thereby be helpful to overcome current limitations of cellular immunotherapies. These insights into the anti-cancer potential of Bi-materials offer a strong foundation for future research in cancer diagnostics and therapy, with the potential to drive the clinical implementation of Bi-based materials for cancer (immuno)theranostics.
Data availability
This is a perspective article that contains no original data.
Conflicts of interest
There are no conflicts to declare.
Acknowledgements
We are grateful for the excellent work carried out by co-workers and colleagues mentioned in the references, specifically the groups in the MHELTHERA consortium. For financial support, we acknowledge Helmholtz-Zentrum Dresden-Rossendorf, the Australian Research Council (DP220103632), Monash University, and in particular the Helmholtz Initiative and Networking Fund (Radio-Immuno-Theranostics (MHELTHERA) project ID: InterLabs-0031).
References
- R. Mohan, Nat. Chem., 2010, 2, 336–336 CrossRef CAS PubMed.
- Â. Gonçalves, M. Matias, J. A. R. Salvador and S. Silvestre, Int. J. Mol. Sci., 2024, 25, 1600 CrossRef PubMed.
- J. A. Salvador, S. A. Figueiredo, R. M. Pinto and S. M. Silvestre, Future Med. Chem., 2012, 4, 1495–1523 CrossRef CAS PubMed.
- G. G. Briand and N. Burford, Chem. Rev., 1999, 99, 2601–2658 CrossRef CAS PubMed.
- K. D. Mjos and C. Orvig, Chem. Rev., 2014, 114, 4540–4563 CrossRef CAS PubMed.
- D. M. Griffith, H. Li, M. V. Werrett, P. C. Andrews and H. Sun, Chem. Soc. Rev., 2021, 50, 12037–12069 RSC.
- R. Ge and H. Sun, Acc. Chem. Res., 2007, 40, 267–274 CrossRef CAS PubMed.
- J. D. S. Rosário, F. H. Moreira, L. H. F. Rosa, W. Guerra and P. P. Silva-Caldeira, Molecules, 2023, 28, 5921 CrossRef PubMed.
- K. Iuchi, S. Shirai, Y. Tasaki and H. Hisatomi, Anti-Cancer Drugs, 2020, 31, 55–59 CrossRef CAS PubMed.
- Y.-P. Liu, J. Lei, L.-W. Tang, Y. Peng, C.-T. Au, Y. Chen and S.-F. Yin, Eur. J. Med. Chem., 2017, 139, 826–835 CrossRef CAS PubMed.
- K. Iuchi and T. Yagura, Toxicol. in Vitro, 2018, 50, 172–178 CrossRef CAS PubMed.
- K. Iuchi, Y. Tasaki, S. Shirai and H. Hisatomi, Biomed. Pharmacother., 2020, 125, 109928 CrossRef CAS PubMed.
- K. Iuchi, Y. Hatano and T. Yagura, Biochem. Pharmacol., 2008, 76, 974–986 CrossRef CAS PubMed.
- Y.-K. Li, M. Yang, M.-X. Li, H. Yu, H.-C. Wu and S.-Q. Xie, Bioorg. Med. Chem. Lett., 2013, 23, 2288–2292 CrossRef CAS PubMed.
- M. Arda, I. I. Ozturk, C. N. Banti, N. Kourkoumelis, M. Manoli, A. J. Tasiopoulos and S. K. Hadjikakou, RSC Adv., 2016, 6, 29026–29044 RSC.
- P. F. Chan, K. P. Ang and R. A. Hamid, BioMetals, 2021, 34, 365–391 CrossRef CAS PubMed.
- K. Onishi, M. Douke, T. Nakamura, Y. Ochiai, N. Kakusawa, S. Yasuike, J. Kurita, C. Yamamoto, M. Kawahata, K. Yamaguchi and T. Yagura, J. Inorg. Biochem., 2012, 117, 77–84 CrossRef CAS PubMed.
- P. F. Chan, K. P. Ang and R. A. Hamid, JBIC, J. Biol. Inorg. Chem., 2024, 29, 217–241 CrossRef CAS PubMed.
- D. H. A. Ishak, K. K. Ooi, K.-P. Ang, A. M. Akim, Y.-K. Cheah, N. Nordin, S. N. B. A. Halim, H.-L. Seng and E. R. T. Tiekink, J. Inorg. Biochem., 2014, 130, 38–51 CrossRef CAS PubMed.
- A. Islam, B. L. Rodrigues, I. M. Marzano, E. C. Perreira-Maia, D. Dittz, M. T. Paz Lopes, M. Ishfaq, F. Frézard and C. Demicheli, Eur. J. Med. Chem., 2016, 109, 254–267 CrossRef CAS PubMed.
- M. López-Cardoso, H. Tlahuext, M. Pérez-Salgado, D. G. Vargas-Pineda, P. P. Román-Bravo, A. M. Cotero-Villegas, M. Acevedo-Quiroz, R. S. Razo-Hernández, P. Alvarez-Fitz, M. A. Mendoza-Catalán, V. Jancik and R. Cea-Olivares, J. Mol. Struct., 2020, 1217, 128456 CrossRef.
- I. M. Marzano, D. Tomco, R. J. Staples, E. H. Lizarazo-Jaimes, D. A. Gomes, M. Bucciarelli-Rodriguez, W. Guerra, Í. P. De Souza, C. N. Verani and E. C. Pereira Maia, J. Inorg. Biochem., 2021, 222, 111522 CrossRef CAS PubMed.
- S. Yarar, I. I. Ozturk, C. N. Banti, N. Panagiotou, C. Papatriantafyllopoulou, M. Manoli, M. J. Manos, A. J. Tasiopoulos and S. K. Hadjikakou, Inorg. Chim. Acta, 2018, 471, 23–33 CrossRef CAS.
- N. Zhang, Y. Tai, M. Li, P. Ma, J. Zhao and J. Niu, Dalton Trans., 2014, 43, 5182 Search PubMed.
- E. R. T. Tiekink, Crit. Rev. Oncol. Hematol., 2002, 42, 217–224 CrossRef PubMed.
- H. Li, C. S. Lai, J. Wu, P. C. Ho, D. De Vos and E. R. T. Tiekink, J. Inorg. Biochem., 2007, 101, 809–816 CrossRef CAS PubMed.
- A. Kumar Singh, A. Kumar, H. Singh, P. Sonawane, P. Pathak, M. Grishina, J. Pal Yadav, A. Verma and P. Kumar, Chem. Biodiversity, 2023, 20, e202300061 CrossRef CAS PubMed.
- R. Ouyang, Y. Yang, X. Tong, K. Feng, Y. Yang, H. Tao, X. Zhang, T. Zong, P. Cao, F. Xiong, N. Guo, Y. Li, Y. Miao and S. Zhou, J. Inorg. Biochem., 2017, 168, 18–26 CrossRef CAS PubMed.
- X. Jia, J. Pang, Y. Chu, S. Li, W. Li, M. Jiang and F. Yang, J. Mol. Struct., 2024, 1318, 139389 CrossRef CAS.
- P. C. Andrews, G. B. Deacon, C. M. Forsyth, P. C. Junk, I. Kumar and M. Maguire, Angew. Chem., Int. Ed., 2006, 45, 5638–5642 CrossRef CAS PubMed.
- D. C. Senevirathna, M. V. Werrett, M. Kubeil, H. Stephan and P. C. Andrews, Dalton Trans., 2019, 48, 15962–15969 RSC.
- Y. Cheng and H. Zhang, Chem. – Eur. J., 2018, 24, 17405–17418 CrossRef CAS PubMed.
- S. Badrigilan, J. Choupani, H. Khanbabaei, M. Hoseini-Ghahfarokhi, T. J. Webster and L. Tayebi, Adv. Healthcare Mater., 2020, 9, 1901695 CrossRef CAS PubMed.
- Q. Wang, J. Du, R. Ouyang, B. Liu, Y. Miao and Y. Li, Coord. Chem. Rev., 2023, 492, 215281 CrossRef CAS.
- O. Rabin, J. Manuel Perez, J. Grimm, G. Wojtkiewicz and R. Weissleder, Nat. Mater., 2006, 5, 118–122 CrossRef CAS PubMed.
- Y. Liu, P. Bhattarai, Z. Dai and X. Chen, Chem. Soc. Rev., 2019, 48, 2053–2108 RSC.
- B. B. Lahiri, S. Bagavathiappan, T. Jayakumar and J. Philip, Infrared Phys. Technol., 2012, 55, 221–235 CrossRef CAS PubMed.
- D. Kesztyüs, S. Brucher, C. Wilson and T. Kesztyüs, Medicina, 2023, 59, 2139 CrossRef PubMed.
- W. Li, Y. Fan, J. Lin, P. Yu, Z. Wang and C. Ning, Adv. Ther., 2022, 5, 2200027 CrossRef CAS.
- M. Kowalik, J. Masternak and B. Barszcz, Curr. Med. Chem., 2019, 26, 729–759 CrossRef CAS PubMed.
- N. Kavousi, M. Nazari, M. T. B. Toossi, H. Azimian and M. Alibolandi, J. Drug Delivery Sci. Technol., 2024, 101, 106136 CrossRef CAS.
- J. Xie, L. Gong, S. Zhu, Y. Yong, Z. Gu and Y. Zhao, Adv. Mater., 2019, 31, 1802244 CrossRef PubMed.
- T. I. Kostelnik and C. Orvig, Chem. Rev., 2019, 119, 902–956 CrossRef CAS PubMed.
- G. Sgouros, L. Bodei, M. R. McDevitt and J. R. Nedrow, Nat. Rev. Drug Discovery, 2020, 19, 589–608 CrossRef CAS PubMed.
- D. Shahbazi-Gahrouei, Y. Choghazardi, A. Kazemzadeh, P. Naseri and S. Shahbazi-Gahrouei, IET Nanobiotechnol., 2023, 17, 302–311 CrossRef PubMed.
- J.-J. Xu, W.-C. Zhang, Y.-W. Guo, X.-Y. Chen and Y.-N. Zhang, Drug Delivery, 2022, 29, 664–678 Search PubMed.
- A. Hheidari, J. Mohammadi, M. Ghodousi, M. Mahmoodi, S. Ebrahimi, E. Pishbin and A. Rahdar, Front. Bioeng. Biotechnol., 2024, 12, 1436297 Search PubMed.
- R. Khursheed, K. Dua, S. Vishwas, M. Gulati, N. K. Jha, G. M. Aldhafeeri, F. G. Alanazi, B. H. Goh, G. Gupta, K. R. Paudel, P. M. Hansbro, D. K. Chellappan and S. K. Singh, Biomed. Pharmacother., 2022, 150, 112951 CrossRef CAS PubMed.
- G. Jia, Y. Wang, M. Sun, H. Zhang, L. Li, Y. Shi, L. Zhang, X. Cui, T. W. B. Lo, B. Huang and J. C. Yu, J. Am. Chem. Soc., 2023, 145, 14133–14142 CrossRef CAS PubMed.
- D. Leng, T. Wang, Y. Li, Z. Huang, H. Wang, Y. Wan, X. Pei and J. Wang, Inorg. Chem., 2021, 60, 17258–17267 CrossRef CAS PubMed.
- I. Khan, K. Saeed and I. Khan, Arabian J. Chem., 2019, 12, 908–931 CrossRef CAS.
- Á. Y. Aguilera, G. Krepper and M. S. Di Nezio, J. Cluster Sci., 2022, 33, 1417–1426 CrossRef.
- J. Wu, F. Qin, Z. Lu, H.-J. Yang and R. Chen, Nanoscale Res. Lett., 2011, 6, 66 CrossRef PubMed.
- M. Mahiuddin and B. Ochiai, RSC Adv., 2021, 11, 26683–26686 RSC.
- C. Liu, L. Zhang, X. Chen, S. Li, Q. Han, L. Li and C. Wang, Chem. Eng. J., 2020, 382, 122720 CrossRef CAS.
- L. Jiao, Q. Li, J. Deng, N. Okosi, J. Xia and M. Su, Nanoscale, 2018, 10, 6751–6757 RSC.
- Y. Xuan, X. Q. Yang, Z. Y. Song, R. Y. Zhang, D. H. Zhao, X. L. Hou, X. L. Song, B. Liu, Y. D. Zhao and W. Chen, Adv. Funct. Mater., 2019, 29, 1900017 CrossRef.
- M. Shakibaie, H. Forootanfar, A. Ameri, M. Adeli-Sardou, M. Jafari and H. R. Rahimi, IET Nanobiotechnol., 2018, 12, 653–657 CrossRef PubMed.
- Z. Saddique, M. Imran, A. Javaid, S. Latif, T. H. Kim, M. Janczarek, M. Bilal and T. Jesionowski, Environ. Res., 2023, 229, 115861 CrossRef CAS PubMed.
- C. Gomez, G. Hallot, A. Pastor, S. Laurent, E. Brun, C. Sicard-Roselli and M. Port, Ultrason. Sonochem., 2019, 56, 167–173 Search PubMed.
- R. Ouyang, Q. Zhang, P. Cao, Y. Yang, Y. Zhao, B. Liu, Y. Miao and S. Zhou, Colloids Surf., B, 2023, 222, 113116 Search PubMed.
- M. Baričić, J. M. Nuñez, M. H. Aguirre, D. Hrabovsky, M. Seydou, C. Meneghini, D. Peddis and S. Ammar, Sci. Rep., 2024, 14, 12529 CrossRef PubMed.
- S. Ammar and F. Fiévet, Nanomaterials, 2020, 10, 1217 CrossRef CAS PubMed.
- S. Yang, Z. Li, Y. Wang, X. Fan, Z. Miao, Y. Hu, Z. Li, Y. Sun, F. Besenbacher and M. Yu, ACS Appl. Mater. Interfaces, 2018, 10, 1605–1615 CrossRef CAS PubMed.
- Q. Bao, Y. Zhang, X. Liu, T. Yang, H. Yue, M. Yang and C. Mao, Adv. Opt. Mater., 2023, 11, 2201482 CrossRef CAS.
- D. Sun, S. Zhou and W. Gao, ACS Nano, 2020, 14, 12281–12290 CrossRef CAS PubMed.
- H. Xiang, Y. Wu, X. Zhu, M. She, Q. An, R. Zhou, P. Xu, F. Zhao, L. Yan and Y. Zhao, J. Am. Chem. Soc., 2021, 143, 11449–11461 CrossRef CAS PubMed.
- J. Fang, H. Nakamura and H. Maeda, Adv. Drug Delivery Rev., 2011, 63, 136–151 CrossRef CAS PubMed.
- S. K. Golombek, J.-N. May, B. Theek, L. Appold, N. Drude, F. Kiessling and T. Lammers, Adv. Drug Delivery Rev., 2018, 130, 17–38 CrossRef CAS PubMed.
- A. Nel, E. Ruoslahti and H. Meng, ACS Nano, 2017, 11, 9567–9569 CrossRef CAS PubMed.
- Y. Huang, Z. Xue and S. Zeng, ACS Appl. Mater. Interfaces, 2020, 12, 31172–31181 CrossRef CAS PubMed.
- X. Yu, A. Li, C. Zhao, K. Yang, X. Chen and W. Li, ACS Nano, 2017, 11, 3990–4001 CrossRef CAS PubMed.
- J. Deng, S. Xu, W. Hu, X. Xun, L. Zheng and M. Su, Biomaterials, 2018, 154, 24–33 Search PubMed.
- X. Ren, S. Yang, N. Yu, A. Sharjeel, Q. Jiang, D. K. Macharia, H. Yan, C. Lu, P. Geng and Z. Chen, J. Colloid Interface Sci., 2021, 591, 229–238 CrossRef CAS PubMed.
- P. Lei, R. An, P. Zhang, S. Yao, S. Song, L. Dong, X. Xu, K. Du, J. Feng and H. Zhang, Adv. Funct. Mater., 2017, 27, 1702018 CrossRef.
- R. Vazquez-Munoz, M. J. Arellano-Jimenez and J. L. Lopez-Ribot, BMC Biomed. Eng., 2020, 2, 11 CrossRef PubMed.
- J. C. Bulmahn, G. Tikhonowski, A. A. Popov, A. Kuzmin, S. M. Klimentov, A. V. Kabashin and P. N. Prasad, Nanomaterials, 2020, 10, 1463 Search PubMed.
- M. B. Larosi, J. D. V. García and A. R. Rodríguez, Nanomaterials, 2022, 12, 2903 CrossRef CAS PubMed.
- H. Bi, F. He, Y. Dong, D. Yang, Y. Dai, L. Xu, R. Lv, S. Gai, P. Yang and J. Lin, Chem. Mater., 2018, 30, 3301–3307 CrossRef CAS.
- K. Zarschler, L. Rocks, N. Licciardello, L. Boselli, E. Polo, K. P. Garcia, L. De Cola, H. Stephan and K. A. Dawson, Nanomedicine, 2016, 12, 1663–1701 CrossRef CAS PubMed.
- C. Yang, C. Guo, W. Guo, X. Zhao, S. Liu and X. Han, ACS Appl. Nano Mater., 2018, 1, 820–830 CrossRef CAS.
- C. Liu, Y. Zhang, J. Wen, J. Liu, M. Huo, Y. Shen, H. Luo and H. Zhang, J. Drug Targeting, 2024, 1–13, DOI:10.1080/1061186x.2024.2329110.
- R. Singh, P. Kumari, M. Kumar, T. Ichikawa and A. Jain, Molecules, 2020, 25, 3733 CrossRef CAS PubMed.
- J. Deng and Z.-Y. Zhao, Comput. Mater. Sci., 2018, 142, 312–319 CrossRef CAS.
- W. W. Anku, S. O. B. Oppong and P. P. Govender,
Bismuth-Based Nanoparticles as Photocatalytic Materials
, InTech, 2018 DOI:10.5772/intechopen.75104.
- M. Batool, M. F. Nazar, A. Awan, M. B. Tahir, A. Rahdar, A. E. Shalan, S. Lanceros-Méndez and M. N. Zafar, Nano-Struct. Nano-Objects., 2021, 27, 100762 CrossRef CAS.
- J. Ni, X. Bi, Y. Jiang, L. Li and J. Lu, Nano Energy, 2017, 34, 356–366 CrossRef CAS.
- T. O. Ajiboye, O. A. Oyewo and D. C. Onwudiwe, Surf. Interfaces, 2021, 23, 100927 CrossRef CAS.
- J. Huang, Q. Huang, M. Liu, Q. Chen and K. Ai, Front. Pharmacol., 2022, 13, 844037 CrossRef CAS PubMed.
- J. Jiang, X. Che, Y. Qian, L. Wang, Y. Zhang and Z. Wang, Front. Mater., 2020, 7, 234 Search PubMed.
- M. Dastgir, Y. Ayyami, A. Pourfarshid, M. Ghorbani and T. Mortezazadeh, J. Drug Delivery Sci. Technol., 2024, 92, 105279 CrossRef CAS.
- M.-A. Shahbazi, L. Faghfouri, M. P. A. Ferreira, P. Figueiredo, H. Maleki, F. Sefat, J. Hirvonen and H. A. Santos, Chem. Soc. Rev., 2020, 49, 1253–1321 RSC.
- Y. He, H. Chen, W. Li, L. Xu, H. Yao, Y. Cao, Z. Wang, L. Zhang, D. Wang and D. Zhou, J. Nanobiotechnol., 2023, 21, 209 Search PubMed.
- K. Poudel, A. Banstola, M. Gautam, Z. C. Soe, L. M. Pham, J.-H. Jeong, H.-G. Choi, S. K. Ku, C. S. Yong, T. H. Tran and J. O. Kim, Nanoscale, 2021, 13, 1231–1247 Search PubMed.
- L. Li, Y. Lu, C. Jiang, Y. Zhu, X. Yang, X. Hu, Z. Lin, Y. Zhang, M. Peng, H. Xia and C. Mao, Adv. Funct. Mater., 2018, 28, 1704623 CrossRef PubMed.
- N. N. Talik Sisin, K. Abdul Razak, S. Zainal Abidin, N. F. Che Mat, R. Abdullah, R. Ab Rashid, M. A. Khairil Anuar and W. N. Rahman, Int. J. Nanomed., 2020, 7805–7823 CrossRef PubMed.
- Z. Song, T. Liu, H. Lai, X. Meng, L. Yang, J. Su and T. Chen, ACS Nano, 2022, 16, 4379–4396 CrossRef CAS PubMed.
- C. Yang, Y. Chen, W. Guo, Y. Gao, C. Song, Q. Zhang, N. Zheng, X. Han and C. Guo, Adv. Funct. Mater., 2018, 28, 1706827 CrossRef.
- J. Du, Z. Gu, L. Yan, Y. Yong, X. Yi, X. Zhang, J. Liu, R. Wu, C. Ge, C. Chen and Y. Zhao, Adv. Mater., 2017, 29, 1701268 CrossRef PubMed.
- J. Liu, X. Zheng, L. Yan, L. Zhou, G. Tian, W. Yin, L. Wang, Y. Liu, Z. Hu and Z. Gu, ACS Nano, 2015, 9, 696–707 CrossRef CAS PubMed.
- X. Zheng, J. Shi, Y. Bu, G. Tian, X. Zhang, W. Yin, B. Gao, Z. Yang, Z. Hu, X. Liu, L. Yan, Z. Gu and Y. Zhao, Nanoscale, 2015, 7, 12581–12591 RSC.
- Y. Chen, G. Zhao, S. Wang, Y. He, S. Han, C. Du, S. Li, Z. Fan, C. Wang and J. Wang, Biomater. Sci., 2019, 7, 3450–3459 RSC.
- X. Cheng, Y. Yong, Y. Dai, X. Song, G. Yang, Y. Pan and C. Ge, Theranostics, 2017, 7, 4087–4098 CrossRef CAS PubMed.
- Y. Cheng, Y. Chang, Y. Feng, H. Jian, X. Wu, R. Zheng, K. Xu and H. Zhang, Adv. Mater., 2019, 31, 1806808 CrossRef PubMed.
- H. Zhang, G. Chen, B. Yu, Y. Shen and H. Cong, ACS Appl. Bio Mater., 2019, 2, 3870–3876 CrossRef CAS PubMed.
- F. Akbarzadeh, K. Khoshgard, E. Arkan, L. Hosseinzadeh and A. Hemati Azandaryani, Artif. Cells, Nanomed., Biotechnol., 2018, 46, S514–S523 CrossRef CAS PubMed.
- F. Akbarzadeh and K. Khoshgard, Photodiagn. Photodyn. Ther., 2024, 46, 104025 CrossRef CAS PubMed.
- J. Bai, X. Jia, Y. Ruan, C. Wang and X. Jiang, Inorg. Chem., 2018, 57, 10180–10188 CrossRef CAS PubMed.
- Y. Ma, D.-Y. Zhang, Z. Peng, S. Guan and J. Zhai, Mol. Pharm., 2020, 17, 3403–3411 CrossRef CAS PubMed.
- F. Du, J. Lou, R. Jiang, Z. Fang, X. Zhao, Y. Niu, S. Zou, M. Zhang, A. Gong and C. Wu, Int. J. Nanomed., 2017, 12, 5973–5992 CrossRef CAS PubMed.
- Y. Lu, L. Li, Z. Lin, M. Li, X. Hu, Y. Zhang, M. Peng, H. Xia and G. Han, Adv. Healthcare Mater., 2018, 7, 1800602 CrossRef PubMed.
- G. Chen, Z. Yang, J. Du, Z. He, Y. Zhang, K. Zheng, S. Cai, M. Chen, Y. Li, L. Zheng, Y. Miao and D. Zhang, Small, 2023, 19, e2304032 CrossRef PubMed.
- Z. Li, Y. Hu, K. A. Howard, T. Jiang, X. Fan, Z. Miao, Y. Sun, F. Besenbacher and M. Yu, ACS Nano, 2016, 10, 984–997 CrossRef CAS PubMed.
- H. Nosrati, J. Charmi, M. Salehiabar, F. Abhari and H. Danafar, ACS Biomater. Sci. Eng., 2019, 5, 4416–4424 CrossRef CAS PubMed.
- S. Javani, M. Barsbay, M. Ghaffarlou, N. Mousazadeh, A. Mohammadi, F. Mozafari, H. Rezaeejam, L. Nasehi, H. Nosrati, T. Kavetskyy and H. Danafar, J. Drug Delivery Sci. Technol., 2022, 71, 103336 CrossRef CAS.
- L. Zhang, Q. Chen, X. Zou, J. Chen, L. Hu, Z. Dong, J. Zhou, Y. Chen, Z. Liu and L. Cheng, J. Mater. Chem. B, 2019, 7, 5170–5181 RSC.
- M. H. Faghfoori, H. Nosrati, H. Rezaeejam, J. Charmi, S. Kaboli, B. Johari and H. Danafar, Int. J. Pharm., 2020, 582, 119320 CrossRef CAS PubMed.
- S. Azizi, H. Nosrati, A. Sharafi and H. Danafar, Appl. Organomet. Chem., 2020, 34, e5251 CrossRef CAS.
- H. Yu, Y. Yang, T. Jiang, X. Zhang, Y. Zhao, G. Pang, Y. Feng, S. Zhang, F. Wang, Y. Wang, Y. Wang and L. W. Zhang, ACS Appl. Mater. Interfaces, 2019, 11, 27536–27547 CrossRef CAS PubMed.
- Y. Wang, Y. Wu, Y. Liu, J. Shen, L. Lv, L. Li, L. Yang, J. Zeng, Y. Wang, L. W. Zhang, Z. Li, M. Gao and Z. Chai, Adv. Funct. Mater., 2016, 26, 5335–5344 CrossRef CAS.
- H. Nosrati, M. Salehiabar, F. Mozafari, J. Charmi, N. Erdoğan, M. Ghaffarlou, F. Abhari, H. Danafar, A. Ramazani and Y. Nuri Ertas, Appl. Organomet. Chem., 2022, 36, e6861 CrossRef CAS.
- H. Nosrati, F. Abhari, J. Charmi, M. Rahmati, B. Johari, S. Azizi, H. Rezaeejam and H. Danafar, Artif. Cells, Nanomed., Biotechnol., 2019, 47, 3832–3838 CrossRef CAS PubMed.
- B. Colak and Y. N. Ertas, ACS Appl. Mater. Interfaces, 2024, 16, 15718–15729 CrossRef CAS PubMed.
- Y. Choghazardi, H. Azimian, A. M. Abadi, M. M. Khoshisani, F. V. Nezamdoust and H. Gholamhosseinian, J. Nanomater., 2023, 2023, 1–11 CrossRef.
- H. Yu, Y. Yang, T. Jiang, X. Zhang, Y. Zhao, G. Pang, Y. Feng, S. Zhang, F. Wang, Y. Wang, Y. Wang and L. W. Zhang, ACS Appl. Mater. Interfaces, 2019, 11, 27536–27547 CrossRef CAS PubMed.
- D. Wang, Y. Yao, J. He, X. Zhong, B. Li, S. Rao, H. Yu, S. He, X. Feng, T. Xu, B. Yang, T. Yong, L. Gan, J. Hu and X. Yang, Adv. Sci., 2020, 7, 1901293 CrossRef CAS PubMed.
- S. Badrigilan, B. Shaabani, N. Gharehaghaji and A. Mesbahi, Photodiagn. Photodyn. Ther., 2019, 25, 504–514 CrossRef CAS PubMed.
- Y. Xuan, X.-L. Song, X.-Q. Yang, R.-Y. Zhang, Z.-Y. Song, D.-H. Zhao, X.-L. Hou, J. An, X.-S. Zhang and Y.-D. Zhao, Chem. Eng. J., 2019, 375, 122000 CrossRef CAS.
- J. Du, H. Ding, S. Fu, D. Li and B. Yu, Front. Bioeng. Biotechnol., 2023, 10, 1098923 CrossRef PubMed.
- S. Goel, D. Ni and W. Cai, ACS Nano, 2017, 11, 5233–5237 CrossRef CAS PubMed.
- L. R. H. Gerken, M. E. Gerdes, M. Pruschy and I. K. Herrmann, Mater. Horiz., 2023, 10, 4059–4082 RSC.
- J. Li and H. Wang, Nanoscale Horiz., 2023, 8, 1155–1173 RSC.
- B. Chen, C. Zhang, W. Wang, Z. Chu, Z. Zha, X. He, W. Zhou, T. Liu, H. Wang and H. Qian, ACS Nano, 2020, 14, 14919–14928 CrossRef CAS PubMed.
- P. Jia, H. Ji, S. Liu, R. Zhang, F. He, L. Zhong and P. Yang, J. Mater. Chem. B, 2021, 9, 101–111 RSC.
- X. Meng, J. Liu, Q. Zheng, S. Li, H. Xiao, J. Huang, L. Ma, Y. Liu and J. Tang, ACS Appl. Mater. Interfaces, 2023, 15, 58041–58053 CrossRef CAS PubMed.
- F. Abhari, J. Charmi, H. Rezaeejam, Z. Karimimoghaddam, H. Nosrati, H. Danafar and A. Farajollahi, ACS Sustainable Chem. Eng., 2020, 8, 5260–5269 CrossRef CAS.
- F. Wu, H. Chen, R. Liu, Y. Suo, Q. Li, Y. Zhang, H. Liu, Z. Cheng and Y. Chang, Adv. Healthcare Mater., 2022, 11, 2200809 CrossRef CAS PubMed.
- Z. Li, X. Fan, J. Liu, Y. Hu, Y. Yang, Z. Li, Y. Sun, C. Chen and M. Yu, Nanomedicine, 2018, 13, 2283–2300 CrossRef CAS PubMed.
- B. Li, Y. Cheng, R. Zheng, X. Wu, F. Qi, Y. Wu, Y. Hu and X. Li, J. Mater. Chem. B, 2020, 8, 8803–8808 RSC.
- L. Dong, P. Zhang, X. Liu, R. Deng, K. Du, J. Feng and H. Zhang, ACS Appl. Mater. Interfaces, 2019, 11, 7774–7781 CrossRef CAS PubMed.
- K. Song, J. Du, X. Wang, L. Zheng, R. Ouyang, Y. Li, Y. Miao and D. Zhang, Adv. Healthcare Mater., 2022, 11, 2102503 CrossRef CAS PubMed.
- S. Wen, W. Zhang, J. Yang, Z. Zhou, Q. Xiang and H. Dong, ACS Nano, 2024, 18, 23672–23683 CrossRef CAS PubMed.
- Y. Dong, S. Dong, B. Liu, C. Yu, J. Liu, D. Yang, P. Yang and J. Lin, Adv. Mater., 2021, 33, 2106838 CrossRef CAS PubMed.
- A. Bijelic, M. Aureliano and A. Rompel, Angew. Chem., Int. Ed., 2019, 58, 2980–2999 CrossRef CAS PubMed.
- F. Carvalho and M. Aureliano, Int. J. Mol. Sci., 2023, 24, 5043 CrossRef CAS PubMed.
- R. Zhou, H. Wang, Y. Yang, C. Zhang, X. Dong, J. Du, L. Yan, G. Zhang, Z. Gu and Y. Zhao, Biomaterials, 2019, 189, 11–22 Search PubMed.
- C. Orellana-Tavra, M. Köppen, A. Li, N. Stock and D. Fairen-Jimenez, ACS Appl. Mater. Interfaces, 2020, 12, 5633–5641 CrossRef CAS PubMed.
- R. Zhou, X. Liu, Y. Wu, H. Xiang, J. Cao, Y. Li, W. Yin, Y. Zu, J. Li, R. Liu, F. Zhao, Z. Liu, C. Chen, Z. Gu, L. Yan and Y. Zhao, ACS Nano, 2020, 14, 13016–13029 CrossRef CAS PubMed.
- S. A. Anushya, S. Prabhu, V. Ravikumar and A. Philominal, J. Inorg. Organomet. Polym. Mater., 2023, 33, 1369–1380 CrossRef CAS.
- R. Dou, Z. Du, T. Bao, X. Dong, X. Zheng, M. Yu, W. Yin, B. Dong, L. Yan and Z. Gu, Nanoscale, 2016, 8, 11531–11542 RSC.
- Y. Zhang, H. Zhang, Y. Wang, H. Wu, B. Zeng, Y. Zhang, Q. Tian and S. Yang, J. Mater. Chem. B, 2017, 5, 1846–1855 RSC.
- Y. Yao, P. Li, J. He, D. Wang, J. Hu and X. Yang, ACS Appl. Mater. Interfaces, 2021, 13, 28650–28661 CrossRef CAS PubMed.
- H. Zhao, J. Wang, X. Li, Y. Li, C. Li, X. Wang, J. Wang, S. Guan, Y. Xu, G. Deng, Y. Chen, J. Lu and X. Liu, J. Colloid Interface Sci., 2021, 604, 80–90 CrossRef CAS PubMed.
- Y. Yao, P. Li, J. He, D. Wang, J. Hu and X. Yang, ACS Appl. Mater. Interfaces, 2021, 13, 28650–28661 CrossRef CAS PubMed.
- J. Liu, J. Zhang, K. Song, J. Du, X. Wang, J. Liu, B. Li, R. Ouyang, Y. Miao, Y. Sun and Y. Li, Small, 2021, 17, e2101015 CrossRef PubMed.
- H. Nosrati, M. Ghaffarlou, M. Salehiabar, N. Mousazadeh, F. Abhari, M. Barsbay, Y. N. Ertas, H. Rashidzadeh, A. Mohammadi, L. Nasehi, H. Rezaeejam, S. Davaran, A. Ramazani, J. Conde and H. Danafar, Biomater. Adv., 2022, 140, 213090 CrossRef CAS PubMed.
- A. Rajaee, S. Wang, L. Zhao, D. Wang, Y. Liu, J. Wang and K. Ying, Phys. Med. Biol., 2019, 64, 195007 CrossRef CAS PubMed.
- A. Detappe, E. Thomas, M. W. Tibbitt, S. Kunjachan, O. Zavidij, N. Parnandi, E. Reznichenko, F. Lux, O. Tillement and R. Berbeco, Nano Lett., 2017, 17, 1733–1740 Search PubMed.
- R. Zhang, X. Wang, Y. Zhang, Q. Liu, H. Shen, B. Chen, C. Tan, W. Liu and X. Jin, ACS Appl. Nano Mater., 2024, 7, 11871–11881 Search PubMed.
- L. Zeng, H. Zhao, Y. Zhu, S. Chen, Y. Zhang, D. Wei, J. Sun and H. Fan, J. Mater. Chem. B, 2020, 8, 4093–4105 RSC.
- A. Ingham, L. Wharton, T. El Sayed, L. Southcott, B. L. McNeil, M. B. Ezhova, B. O. Patrick, M. D. G. Jaraquemada-Peláez and C. Orvig, Inorg. Chem., 2022, 61, 9119–9137 CrossRef CAS PubMed.
- K. A. Morgan, S. E. Rudd, A. Noor and P. S. Donnelly, Chem. Rev., 2023, 123, 12004–12035 CrossRef CAS PubMed.
- R. Eychenne, M. Chérel, F. Haddad, F. Guérard and J.-F. Gestin, Pharmaceutics, 2021, 13, 906 CrossRef CAS PubMed.
- M. G. Ferrier, V. Radchenko and D. S. Wilbur, Radiochim. Acta, 2019, 107, 1065–1085 CrossRef CAS.
- D. Szücs, J. P. Szabó, V. Arató, B. Gyuricza, D. Szikra, I. Tóth, Z. Képes, G. Trencsényi and A. Fekete, Pharmaceuticals, 2023, 16, 1280 CrossRef PubMed.
- S. Hassfjell, K. Ingebrigtsen and Ø. S. Bruland, Nucl. Med. Biol., 2001, 28, 425–433 CrossRef CAS PubMed.
- I. n. d. services, https://www-nds.iaea.org/, (accessed 21st of August, 2024).
- G. Henriksen and P. Hoff, Appl. Radiat. Isot., 1998, 49, 357–359 CrossRef CAS.
- S. Ahenkorah, I. Cassells, C. M. Deroose, T. Cardinaels, A. R. Burgoyne, G. Bormans, M. Ooms and F. Cleeren, Pharmaceutics, 2021, 13, 599 CrossRef CAS PubMed.
- S. Franchi, V. Di Marco and M. Tosato, Nucl. Med. Biol., 2022, 114–115, 168–188 CrossRef CAS PubMed.
- L. Wharton, C. Zhang, H. Yang, J. Zeisler, V. Radchenko, C. Rodríguez-Rodríguez, M. Osooly, B. O. Patrick, K.-S. Lin, F. Bénard, P. Schaffer and C. Orvig, Bioconjugate Chem., 2022, 33, 505–522 CrossRef CAS PubMed.
- J. Šimeček, P. Hermann, C. Seidl, F. Bruchertseifer, A. Morgenstern, H.-J. Wester and J. Notni, EJNMMI Res., 2018, 8, 78 CrossRef PubMed.
- A. Hu, V. Brown, S. N. Macmillan, V. Radchenko, H. Yang, L. Wharton, C. F. Ramogida and J. J. Wilson, Inorg. Chem., 2022, 61, 801–806 CrossRef CAS PubMed.
- P. Randhawa, K. J. Kadassery, B. L. McNeil, S. N. MacMillan, L. Wharton, H. Yang, J. J. Wilson and C. F. Ramogida, Inorg. Chem., 2024, 63, 21177–21193 CrossRef CAS PubMed.
- F. Bruchertseifer, P. Comba, B. Martin, A. Morgenstern, J. Notni, M. Starke and H. Wadepohl, ChemMedChem, 2020, 15, 1591–1600 CrossRef CAS PubMed.
- I. Kopp, P. Cieslik, K. Anger, T. Josephy, L. Neupert, G. Velmurugan, M. Gast, H. Wadepohl, S. A. Brühlmann, M. Walther, K. Kopka, M. Bachmann, H. Stephan, M. Kubeil and P. Comba, Inorg. Chem., 2023, 62, 20754–20768 CrossRef CAS PubMed.
- H. Song, M. Hedayati, R. F. Hobbs, C. Shao, F. Bruchertseifer, A. Morgenstern, T. L. Deweese and G. Sgouros, Mol. Cancer Ther., 2013, 12, 2043–2054 CrossRef CAS PubMed.
- M. Lingappa, H. Song, S. Thompson, F. Bruchertseifer, A. Morgenstern and G. Sgouros, Cancer Res., 2010, 70, 6815–6823 CrossRef CAS PubMed.
- E. Revskaya, Z. Jiang, A. Morgenstern, F. Bruchertseifer, M. Sesay, S. Walker, S. Fuller, M. S. Lebowitz, C. Gravekamp, H. A. Ghanbari and E. Dadachova, Cancer Biother. Radiopharm., 2017, 32, 57–65 CrossRef CAS PubMed.
- M. E. Autenrieth, C. Seidl, F. Bruchertseifer, T. Horn, F. Kurtz, B. Feuerecker, C. D'Alessandria, C. Pfob, S. Nekolla, C. Apostolidis, S. Mirzadeh, J. E. Gschwend, M. Schwaiger, K. Scheidhauer and A. Morgenstern, Eur. J. Nucl. Med. Mol. Imaging, 2018, 45, 1364–1371 CrossRef CAS PubMed.
- S. Heskamp, R. Hernandez, J. D. M. Molkenboer-Kuenen, M. Essler, F. Bruchertseifer, A. Morgenstern, E. J. Steenbergen, W. Cai, C. Seidl, W. J. McBride, D. M. Goldenberg and O. C. Boerman, J. Nucl. Med., 2017, 58, 926–933 CrossRef CAS PubMed.
- D. E. Milenic, E. D. Brady, K. Garmestani, P. S. Albert, A. Abdulla and M. W. Brechbiel, Cancer, 2010, 116, 1059–1066 CrossRef CAS PubMed.
- L. Królicki, F. Bruchertseifer, J. Kunikowska, H. Koziara, B. Królicki, M. Jakuciński, D. Pawlak, C. Apostolidis, S. Mirzadeh, R. Rola, A. Merlo and A. Morgenstern, Eur. J. Nucl. Med. Mol. Imaging, 2019, 46, 614–622 CrossRef PubMed.
- E. Drecoll, F. C. Gaertner, M. Miederer, B. Blechert, M. Vallon, J. M. Müller, A. Alke, C. Seidl, F. Bruchertseifer, A. Morgenstern, R. Senekowitsch-Schmidtke and M. Essler, PLoS One, 2009, 4, e5715 CrossRef PubMed.
- J. D. Nosanchuk, A. Jeyakumar, A. Ray, E. Revskaya, Z. Jiang, R. A. Bryan, K. J. H. Allen, R. Jiao, M. E. Malo, B. L. Gómez, A. Morgenstern, F. Bruchertseifer, D. Rickles, G. B. Thornton, A. Bowen, A. Casadevall and E. Dadachova, Sci. Rep., 2018, 8, 5466 CrossRef CAS PubMed.
- B. J. Allen, C. Raja, S. Rizvi, Y. Li, W. Tsui, P. Graham, J. Thompson, R. Reisfeld, J. Kearsley, A. Morgenstern and C. Apostolidis, Cancer Biol. Ther., 2005, 4, 1318–1324 CrossRef CAS PubMed.
- C. Raja, P. Graham, S. Rizvi, E. Song, H. Goldsmith, J. Thompson, A. Bosserhoff, A. Morgenstern, C. Apostolidis, J. Kearsley, R. Reisfeld and B. J. Allen, Cancer Biol. Ther., 2007, 6, 846–852 CrossRef CAS PubMed.
- B. J. Allen, A. A. Singla, S. M. A. Rizvi, P. Graham, F. Bruchertseifer, C. Apostolidis and A. Morgenstern, Immunotherapy, 2011, 3, 1041–1050 CrossRef CAS PubMed.
- J. Ménager, J.-B. Gorin, C. Maurel, L. Drujont, S. Gouard, C. Louvet, M. Chérel, A. Faivre-Chauvet, A. Morgenstern, F. Bruchertseifer, F. Davodeau, J. Gaschet and Y. Guilloux, PLoS One, 2015, 10, e0130249 CrossRef PubMed.
- N. Fichou, S. Gouard, C. Maurel, J. Barbet, L. Ferrer, A. Morgenstern, F. Bruchertseifer, A. Faivre-Chauvet, E. Bigot-Corbel, F. Davodeau, J. Gaschet and M. Chérel, Front. Med., 2015, 2, 76 CrossRef PubMed.
- K. Teiluf, C. Seidl, B. Blechert, F. C. Gaertner, K.-P. Gilbertz, V. Fernandez, F. Bassermann, J. Endell, R. Boxhammer, S. Leclair, M. Vallon, M. Aichler, A. Feuchtinger, F. Bruchertseifer, A. Morgenstern and M. Essler, Oncotarget, 2015, 6, 4692–4703 CrossRef PubMed.
- S. I. Park, J. Shenoi, J. M. Pagel, D. K. Hamlin, D. S. Wilbur, N. Orgun, A. L. Kenoyer, S. Frayo, A. Axtman, T. Bäck, Y. Lin, D. R. Fisher, A. K. Gopal, D. J. Green and O. W. Press, Blood, 2010, 116, 4231–4239 CrossRef CAS PubMed.
- M. Roscher, I. Hormann, O. Leib, S. Marx, J. Moreno, E. Miltner and C. Friesen, Oncotarget, 2013, 4, 218–230 CrossRef PubMed.
- A. Derrien, S. Gouard, C. Maurel, M.-H. Gaugler, F. Bruchertseifer, A. Morgenstern, A. Faivre-Chauvet, J.-M. Classe and M. Chérel, Front. Med., 2015, 2(88) Search PubMed.
- A. Gustafsson-Lutz, T. Bäck, E. Aneheim, R. Hultborn, S. Palm, L. Jacobsson, A. Morgenstern, F. Bruchertseifer, P. Albertsson and S. Lindegren, EJNMMI
Res., 2017, 7, 38 CrossRef PubMed.
- A. M. E. Gustafsson, T. Bäck, J. Elgqvist, L. Jacobsson, R. Hultborn, P. Albertsson, A. Morgenstern, F. Bruchertseifer, H. Jensen and S. Lindegren, Nucl. Med. Biol., 2012, 39, 15–22 Search PubMed.
- D. Horváth, A. Vágner, D. Szikra, G. Trencsényi, N. Demitri, N. Guidolin, A. Maiocchi, S. Ghiani, F. Travagin, G. B. Giovenzana and Z. Baranyai, Angew. Chem., Int. Ed., 2022, 61, e202207120 Search PubMed.
- H. S. Chan, M. W. Konijnenberg, T. Daniels, M. Nysus, M. Makvandi, E. De Blois, W. A. Breeman, R. W. Atcher, M. De Jong and J. P. Norenberg, EJNMMI Res., 2016, 6, 83 Search PubMed.
- C. Kratochwil, F. L. Giesel, F. Bruchertseifer, W. Mier, C. Apostolidis, R. Boll, K. Murphy, U. Haberkorn and A. Morgenstern, Eur. J. Nucl. Med. Mol. Imaging, 2014, 41, 2106–2119 Search PubMed.
- S. H. Kurtzman, A. Russo, J. B. Mitchell, W. Degraff, W. F. Sindelar, M. W. Brechbiel, O. A. Gansow, A. M. Friedman, J. J. Hines, J. Gamson and R. W. Atcher, JNCI, J. Natl. Cancer Inst., 1988, 80, 449–452 Search PubMed.
- R. Jiao, K. J. H. Allen, M. E. Malo, M. Helal, Z. Jiang, K. Smart, S. V. Buhl, D. Rickles, R. A. Bryan and E. Dadachova, Cancer Med., 2019, 8, 5289–5300 Search PubMed.
- B. J. Allen, S. M. A. Rizvi, C. F. Qu and R. C. Smith, Cancers, 2011, 3, 1821–1843 Search PubMed.
- Y. Li, S. M. A. Rizvi, M. Ranson and B. J. Allen, Br. J. Cancer, 2002, 86, 1197–1203 CrossRef CAS PubMed.
- D. Cordier, F. Forrer, F. Bruchertseifer, A. Morgenstern, C. Apostolidis, S. Good, J. Müller-Brand, H. Mäcke, J. C. Reubi and A. Merlo, Eur. J. Nucl. Med. Mol. Imaging, 2010, 37, 1335–1344 CrossRef CAS PubMed.
- L. Krolicki, F. Bruchertseifer, J. Kunikowska, H. Koziara, B. Królicki, M. Jakuciński, D. Pawlak, C. Apostolidis, S. Mirzadeh, R. Rola, A. Merlo and A. Morgenstern, Eur. J. Nucl. Med. Mol. Imaging, 2018, 45, 1636–1644 CrossRef CAS PubMed.
- J. G. Jurcic, S. M. Larson, G. Sgouros, M. R. McDevitt, R. D. Finn, C. R. Divgi, Å. M. Ballangrud, K. A. Hamacher, D. Ma, J. L. Humm, M. W. Brechbiel, R. Molinet and D. A. Scheinberg, Blood, 2002, 100, 1233–1239 Search PubMed.
- T. L. Rosenblat, M. R. McDevitt, D. A. Mulford, N. Pandit-Taskar, C. R. Divgi, K. S. Panageas, M. L. Heaney, S. Chanel, A. Morgenstern, G. Sgouros, S. M. Larson, D. A. Scheinberg and J. G. Jurcic, Clin. Cancer Res., 2010, 16, 5303–5311 CrossRef CAS PubMed.
- Y. Dekempeneer, V. Caveliers, M. Ooms, D. Maertens, M. Gysemans, T. Lahoutte, C. Xavier, Q. Lecocq, K. Maes, P. Covens, B. W. Miller, F. Bruchertseifer, A. Morgenstern, T. Cardinaels and M. D'Huyvetter, Mol. Pharm., 2020, 17, 3553–3566 CrossRef CAS PubMed.
- C. Kratochwil, K. Schmidt, A. Afshar-Oromieh, F. Bruchertseifer, H. Rathke, A. Morgenstern, U. Haberkorn and F. L. Giesel, Eur. J. Nucl. Med. Mol. Imaging, 2018, 45, 31–37 CrossRef CAS PubMed.
- M. Sathekge, O. Knoesen, M. Meckel, M. Modiselle, M. Vorster and S. Marx, Eur. J. Nucl. Med. Mol. Imaging, 2017, 44, 1099–1100 Search PubMed.
- M. D. Diener, J. M. Alford, S. J. Kennel and S. Mirzadeh, J. Am. Chem. Soc., 2007, 129, 5131–5138 Search PubMed.
- R. Wang, H. T. Wolterbeek and A. G. Denkova, J. Label. Compd. Radiopharm., 2024, 67, 375–383 CrossRef CAS PubMed.
- N. Kauffman, S. K. Singh, J. Morrison and K. R. Zinn, Front. Chem., 2023, 11, 1204872 CrossRef CAS PubMed.
- Z. Cao, K. Deng, J. Jiang, K. Tian and B. Wang, Biomed. Pharmacother., 2025, 182, 117727 CrossRef CAS PubMed.
- Y. Sun, L. Liu, H. He, G. Cui, Y. Zheng, C. Ye, L. Qu, Y. Sun, J. Ji, T. Lammers, Y. Zhang and Z. Zhong, J. Controlled Release, 2025, 379, 327–343 CrossRef CAS PubMed.
- J. Li, C. Tan, J. Yang, Z. Xiang, Y. Wang, M. Shen, S. Zhu, T. He, X. Liang, B. Shao, H. Li, Z. Li, L. Liu and C. Gong, Biomaterials, 2025, 316, 123005 CrossRef CAS PubMed.
- C. Arndt, R. Bergmann, F. Striese, K. Merkel, D. Máthé, L. R. Loureiro, N. Mitwasi, A. Kegler, F. Fasslrinner, K. E. González Soto, C. Neuber, N. Berndt, N. Kovács, D. Szöllősi, N. Hegedűs, G. Tóth, J.-P. Emmermann, K. B. Harikumar, T. Kovacs, M. Bachmann and A. Feldmann, Cancers, 2022, 14, 1996 Search PubMed.
- A. S. Köseer, L. R. Loureiro, J. Jureczek, N. Mitwasi, K. E. González Soto, J. Aepler, T. Bartsch, A. Feldmann, L. A. Kunz-Schughart, A. Linge, M. Krause, M. Bachmann, C. Arndt and A. Dubrovska, Cancers, 2022, 14, 1677 CrossRef PubMed.
- C. Arndt, L. R. Loureiro, A. Feldmann, J. Jureczek, R. Bergmann, D. Máthé, N. Hegedüs, N. Berndt, S. Koristka, N. Mitwasi, F. Fasslrinner, C. Lamprecht, A. Kegler, A. Hoffmann, T. Bartsch, A. S. Köseer, G. Egan, M. Schmitz, V. Hořejší, M. Krause, A. Dubrovska and M. Bachmann, OncoImmunology, 2020, 9, 1743036 CrossRef PubMed.
- L. R. Loureiro, L. Hoffmann, C. Neuber, L. Rupp, C. Arndt, A. Kegler, M. Kubeil, C. E. Hagemeyer, H. Stephan, M. Schmitz, A. Feldmann and M. Bachmann, J. Exp. Clin. Cancer Res., 2023, 42, 341 CrossRef CAS PubMed.
- M. Bachmann, Immunol. Lett., 2019, 211, 13–22 CrossRef CAS PubMed.
- P. Valent, B. Groner, U. Schumacher, G. Superti-Furga, M. Busslinger, R. Kralovics, C. Zielinski, J. M. Penninger, D. Kerjaschki, G. Stingl, J. S. Smolen, R. Valenta, H. Lassmann, H. Kovar, U. Jäger, G. Kornek, M. Müller and F. Sörgel, J. Innate Immun., 2016, 8, 111–120 CrossRef CAS PubMed.
- M. J. Smyth and M. W. Teng, Clin. Transl. Immunol., 2018, 7, e1041 Search PubMed.
- A. Patterson and N. Auslander, Nat. Commun., 2022, 13, 5151 CrossRef CAS PubMed.
- C. Arndt, F. Fasslrinner, L. R. Loureiro, S. Koristka, A. Feldmann and M. Bachmann, Cancers, 2020, 12, 1302 Search PubMed.
- G. Gross, T. Waks and Z. Eshhar, Proc. Natl. Acad. Sci. U. S. A., 1989, 86, 10024–10028 Search PubMed.
- C. Arndt, A. Feldmann, S. Koristka, M. Cartellieri, M. Dimmel, A. Ehninger, G. Ehninger and M. Bachmann, Prostate, 2014, 74, 1335–1346 CrossRef CAS PubMed.
-
M. Bachmann, US patent10246516B2, 2016 Search PubMed.
-
M. Bachmann, US patent10766943, 2020 Search PubMed.
-
M. Bachmann, US patent17/251447, 2021 Search PubMed.
- M. Cartellieri, A. Feldmann, S. Koristka, C. Arndt, S. Loff, A. Ehninger, M. Von Bonin, E. P. Bejestani, G. Ehninger and M. P. Bachmann, Blood Cancer J., 2016, 6, e458–e458 CrossRef CAS PubMed.
- A. Feldmann, A. Hoffmann, R. Bergmann, S. Koristka, N. Berndt, C. Arndt, L. Rodrigues Loureiro, E. Kittel-Boselli, N. Mitwasi, A. Kegler, C. Lamprecht, K. E. González Soto and M. Bachmann, OncoImmunology, 2020, 9, 1785608 CrossRef PubMed.
- L. E. Pelepenko, A. C. P. Janini, B. P. F. A. Gomes, A. De-Jesus-Soares and M. A. Marciano, Antibiotics, 2022, 11, 1741 CrossRef CAS PubMed.
- M. Epple, V. M. Rotello and K. Dawson, Acc. Chem. Res., 2023, 56, 3369–3378 CrossRef CAS PubMed.
- S. Li, C. Cortez-Jugo, Y. Ju and F. Caruso, ACS Nano, 2024, 18, 33257–33263 CrossRef CAS PubMed.
- C. Domingues, A. Santos, C. Alvarez-Lorenzo, A. Concheiro, I. Jarak, F. Veiga, I. Barbosa, M. Dourado and A. Figueiras, ACS Nano, 2022, 16, 9994–10041 CrossRef CAS PubMed.
- R. Prasad, A. Ghosh, V. Patel, B. Peng, B. B. Mendes, E. H. A. Win, L. G. Delogu, J. Y. Wong, K. J. Pischel, J. R. Bellare, A. Bar-Shir, A. S. Thakor, W. J. Parak, Z. M. Bhujwalla, Y. S. Zhang, N. Kommineni, V. M. Rotello, W. Cai, T. Lammers, T. W. Odom, G. Padmanaban, D. Peer, J. F. Lovell, R. Srivastava, R. Langer and J. Conde, ACS Nano, 2025, 19, 2979–2991 CrossRef CAS PubMed.
|
This journal is © The Royal Society of Chemistry 2025 |
Click here to see how this site uses Cookies. View our privacy policy here.