DOI:
10.1039/D4LC00826J
(Paper)
Lab Chip, 2025,
25, 16-27
Repeated pulses of ultrasound maintain sperm motility†
Received
1st October 2024
, Accepted 20th November 2024
First published on 26th November 2024
Abstract
Sperm motility is a primary criterion for selecting viable and functional sperm in assisted reproduction, where the most motile sperm are used to increase the likelihood of successful conception. Traditional chemical agents to enhance motility pose embryo-toxicity risks, necessitating safer alternatives. This study investigates the use of low-intensity pulsed ultrasound exposure as a non-invasive treatment within an acoustofluidic device to maintain sperm motility. We utilized a droplet-based platform to examine the effects of repeated ultrasound pulses on single human sperm cells. Our findings demonstrate that repeated pulsed ultrasound maintains sperm motility over an hour, with significant improvements in motility parameters by at least 25% as compared to non-exposed sperm. Moreover, we show that the motility enhancements by repeated pulsed ultrasound are more significant in initially non-progressive sperm. Importantly, this method did not compromise sperm viability or DNA integrity. These results suggest a viable, sperm safe approach to enhance and maintain sperm motility, potentially improving assisted reproduction outcomes.
1. Introduction
Sperm motility is a critical factor in both natural and assisted reproduction, closely associated with semen quality and significantly influencing the success rates of reproductive outcomes.1–4 In natural reproduction, semen samples ideally contain at least 42% motile sperm, including 30% progressively motile cells.5 In assisted reproduction, sperm motility enables the utilization of sperm selection methods that feature in vivo characteristics of the natural selection of sperm in the female reproductive tract.6 In intrauterine insemination (IUI), a concentration of 30% progressively motile sperm7 is considered a threshold value, and for in vitro fertilisation (IVF) a semen sample is required to have at least 30% motile sperm including 15% progressively motile cells.8 Moreover, intracytoplasmic sperm injection (ICSI), as the most common and successful treatment method, consists of injecting a selected sperm into the egg. However, even here, sperm motility assists, even in the form of twitching motility, as it is a crucial indicator of a live sperm with intact DNA. Indeed, in each ICSI cycle, the injection of motile sperm significantly increases the fertilization rate by 28%.9
The pivotal role of motility in treatment procedures has led to the utilization of chemical drugs such as pentoxifylline which stimulate motility by inducing flagellum protein phosphorylation.10–12 However, these drugs found limited use in assisted reproduction as they are proven to be toxic to the embryo13,14 which necessitates multistep washing procedures that can cause the generation of reactive oxygen species (ROS) and DNA damage.15,16 Hence, a method to increase sperm motility, without the concerns about chemical toxicity, may prove advantageous in clinical practices.
In understanding the mechanisms behind sperm motility metabolic pathways and environmental stimuli play crucial roles, underscoring the possibilities for enhancing sperm motility through various methods. Sperm swimming is driven by flagellar dyneins through a mechanochemical cycle of adenosine triphosphate (ATP) hydrolysis.17 Oxidative phosphorylation (OXPHOS) in the midpiece and glycolysis in the principal piece are the two main metabolic pathways responsible for the generation of ATP in sperm.18 Sperm engage either of these two pathways as they travel towards the egg, regulating motility and metabolic activity levels.19 Metabolic activity and ATP synthesis can be regulated in response to cellular functions as well as environmental stresses.20,21 Sperm cells also utilize metabolism regulation for different cell processes22,23 and in response to conditions such as thermal,24 electrical25 and magnetic26 stimulations. Ion channels play a vital role in the response of different cell types to environmental stimulations by providing signalling pathways across the cell membrane.27,28 Similarly, sperm cells utilize pathways for ion transport which are necessary to trigger motility and adapt the cells to their surrounding environment.29,30
Sperm motility improvements have been shown as a result of exposure to ultrasound in an acoustofluidic device, resulting in an increase in bull sperm metabolic activity and motility at the population level after a brief acoustic exposure.31 Similar acoustofluidic systems have emerged with widespread biomedical applications for contactless, label-free, and biocompatible single-cell manipulation and analysis,32–35 tissue engineering,36 drug delivery,37–39 point-of-care testing,40 and disease diagnosis.41–43 Studies on the biological effects of low-intensity ultrasound on live cells revealed promising results in therapeutic applications such as fracture healing,44 tissue regeneration,45 neuromodulation,46 cancer treatment,47 and inflammation inhibition.48 In addition, the application of surface acoustic waves (SAWs) in an acoustofluidic system showed an enhancement in the metabolic activity of human keratinocyte and mouse fibroblast upon exposure to ultrasound.49
Acoustofluidics provide the capability of exposing microfluidic samples to ultrasound,50–52 however, that small fluidic sample can be subdivided by using droplet fluidics within such devices.53–56 By reducing volumes further, it is possible to explore the effect of ultrasound at the single-cell level, with each cell being isolated in a droplet. Such single-cell analysis allows pre-exposure motility to be linked to post-exposure for each individual cell, and has demonstrated that the motility of poorly motile sperm can be significantly enhanced and live immotile sperm can start to show twitching motility and flagellar deformation.57 This provides a promising opportunity for the potential use of ultrasound in assisted reproduction of the poorest samples and would allow the detection of live immotile cells in very challenging testicular samples. However, in order to also consider potential treatment applications which are more time-consuming, including sperm preparation and incubation steps, it is useful to explore the temporal variations in sperm motility following ultrasound exposure.
A single short pulse of ultrasound has been shown to considerably increase sperm motility.57,58 In this work, we utilized a droplet-based acoustofluidic platform to study the effect, on single-cells, of repeated pulses of ultrasound, referred to as pulsed ultrasound, with a view to understanding how long the behavioural change lasts, and how often it can be effectively administered. We showed that pulsed exposure proves effective in maintaining human sperm motility for over an hour, with significantly higher motility when compared to a non-exposed sperm control. Our single-cell analysis demonstrates that sperm cells exposed to the 5-minute repetition time pulsed ultrasound experience improvement in motility parameters at the end of the 1-hour monitoring duration compared to the non-exposed sperm by at least 25% on average. Moreover, our results reveal that pulsed ultrasound is particularly effective in enhancing the motility of initially nonprogressive sperm cells by at least 33% improvements at the end of the 1-hour duration compared to the non-exposed sperm. In addition, pulsed ultrasound has no adverse effects on sperm viability and DNA integrity. These findings highlight the potential of pulsed ultrasound as a biocompatible approach to maintain the motility of sperm cells during time-consuming sperm preparation and incubation procedures of assisted reproduction methods, to improve selection and fertilisation outcomes.
2. Results
Individual sperm cells were encapsulated in droplets and assembled in a low flow expansion chamber (Fig. 1a) for imaging and motility tracking (Fig. S1a†) at the single-cell level. To generate SAWs, two opposing interdigital transducers (IDTs) were excited by an RF sinusoidal signal at the power of 800 mW and the frequency of 40 MHz for 10 seconds. The SAWs generated by the IDTs propagate along the piezoelectric substrate and couple into the aqueous droplets upon contact, effectively generating ultrasound waves within the droplet medium. This coupling is facilitated by the direct contact between the droplets and the substrate, which allows the surface waves to transfer as longitudinal ultrasound waves into the aqueous medium of each droplet. These ultrasound waves provide localized acoustic fields that directly interact with the sperm cells (Fig. 1b). The efficiency of this coupling is influenced by the acoustic impedance mismatch between the piezoelectric substrate, the oil phase, and the droplets.
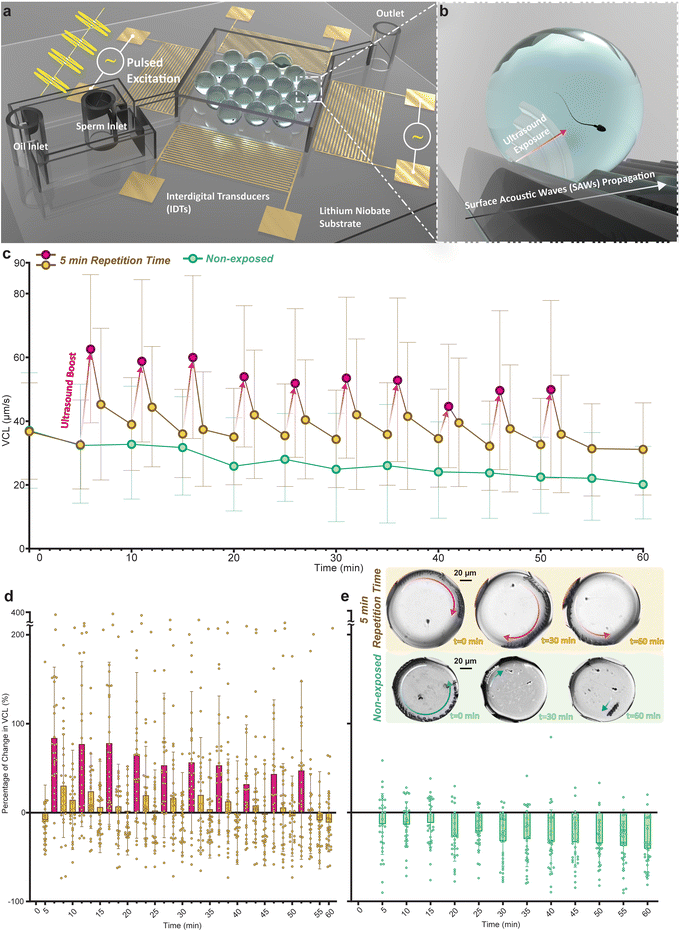 |
| Fig. 1 Exposure of human sperm cells to pulsed ultrasound maintains their motility. (a) Schematic representation of the droplet acoustofluidic device utilized for the encapsulation of individual sperm cells and investigation of ultrasound effects. The device incorporates a flow-focusing junction droplet generation microfluidic system integrated with interdigital transducers (IDTs) on a lithium niobate piezoelectric substrate. (b) Surface acoustic waves (SAWs) propagating on the substrate interact with droplets within the expansion chamber, generating ultrasound waves that impact encapsulated sperm. (c) Comparison of average curvilinear velocities (VCL) over a 60-minute monitoring duration between encapsulated sperm exposed to the 5-minute repetition time pulsed ultrasound (n = 30 at each tracking period, and Power (1-β) = 99.99% for a total sample size of 990 and α = 0.05) and non-exposed encapsulated sperm (n = 30 at each tracking period, and Power (1-β) = 99.78% for a total sample size of 390 and α = 0.05). (d and e) Percentage of change in VCL for individual sperm cells over time relative to VCL at t = 0 for the 5-minute and non-exposed groups, respectively (n = 30, at each tracking period). Overlaid images of a representative exposed and a representative non-exposed sperm cell at t = 0, 30, and 60 minutes and the distance they swam are shown in (e). Sperm exposed to pulsed ultrasound (10 pulses, each lasting 10 seconds, with the repetition time of 5 minutes) almost maintained its motility while the non-exposed sperm showed a considerable decline. | |
First, we assessed the temporal changes in sperm motility following exposure to a single-pulse ultrasound. After this pulse the curvilinear velocity (VCL) increased initially by 73% above the pre-exposure level, however, this increase fell to 12% after five minutes (Fig. S2†). To evaluate the efficacy of pulsed ultrasound for enhancing and maintaining sperm motility, pulsed excitation was achieved by applying the aforementioned signal with 3 different repetition times of 5, 10, and 20 minutes for 1 hour (shown schematically in Fig. S1b†). The sperm cells were monitored throughout the 1-hour duration, with tracking conducted over 2-second periods separated by 5-minute intervals, and motility parameters for encapsulated sperm cells at each tracking period were calculated (Fig. S1c†). To precisely monitor the temporal variations in the motility parameters upon the exposure pulses we utilized two additional tracking periods 5 seconds and 1 minute after each exposure, thus enhancing the post-exposure temporal resolution of the dataset.
Fig. 1c shows the average VCL values of sperm cell responses to the 5-minute repetition time pulsed exposure as compared to the non-exposed sperm (Tables S1 and S2† list corresponding statistical significances). Non-exposed sperm cells showed a gradual decrease in their VCL over time, exhibiting an average decline of 41% at the end of the 1-hour monitoring duration (t = 60 minutes). Sperm cells exposed to the 5-minute repetition time pulsed ultrasound (referred to as the 5-minute group) showed an average between 92% and 29% boost to VCL at each exposure (red circles followed by red arrows, Fig. 1c). Similar to the single exposure results, this group also mostly showed a significant decline in the motility boost 5 minutes after each exposure. However, at the end of the monitoring duration, the 5-minute group showed a higher VCL compared to the non-exposed sperm cells and exhibited only an average of 11% drop at the end of the 1-hour monitoring duration.
The percentage of change in the VCL at each tracking period with respect to the VCL at t = 0 minute for the 5-minute group and the non-exposed group are shown in Fig. 1d and e, respectively. For the 5-minute group, the highest average increases with respect to VCL at t = 0 minute were obtained in periods immediately after the exposure (red bars), with individual sperm cells displaying boosts of up to 378% in VCL. On average, these sperm cells only in 7 out of 32 tracking periods (at t = 5, 30, 45, 50, 52, 55, and 60 minutes) showed a negative change in the VCL with respect to their initial VCL. After 50 minutes no further pulses were administered so that the post exposure delay could be measured over a final ten minute spell. However, at t = 60 minutes there are still 10 out of 30 sperm cells with a VCL higher than their initial VCL. Meanwhile, non-exposed sperm cells on average experienced a steady decline in the VCL during the 1-hour monitoring duration with a negative average change in VCL in all the 12 tracking periods. The trajectories of a representative sperm in the 5-minute group (red arrow on top droplets) and a non-exposed sperm (blue arrows on bottom droplets) at 3 different tracking periods are shown in Fig. 1e. The sperm exposed to the 5-minute repetition time ultrasound conserved its motility, while the non-exposed sperm showed a considerable drop in motility (see ESI† Movie S1).
To assess the effect of the rate of ultrasound pulsing on maintaining sperm motility, responses of individual sperm cells to 10 and 20-minute repetition time pulsed exposure were examined (referred to as 10- and 20-minute groups, respectively). Fig. 2a and b show the VCL graph for the 10- and 20-minute groups alongside the non-exposed group, respectively (with the percentage change in VCL shown in Fig. S3†). The 10-minute group experienced a total of 5 exposures with average boost values between 92% and 70% while the 20-minute group received 2 pulses resulting in the average boosts of 72% and 76%, respectively (Tables S3 and S4†). The 10-minute group experienced a lower relative decline in average VCL compared to the 20-minute group (downward arrows in Fig. 2a and b). The percentage of change in VCL, VAP (average path velocity), ALH (amplitude of lateral head displacement), and BCF (beat cross-frequency) of encapsulated sperm cells exposed to 5, 10, and 20-minute pulsed ultrasound as well as non-exposed sperm at t = 60 minutes with respect to corresponding initial values at t = 0 is shown in Fig. 2c–f, respectively. The results indicate that the sperm cells that were exposed to a higher repetition rate exhibited less decline in the motility parameters after 1 hour. Specifically, the 5-minute group showed a significantly lower decline in VCL, VAP, and ALH compared to the non-exposed sperm at the end of the monitoring duration. As a result, compared to non-exposed sperm, sperm cells exposed to pulsed ultrasound swim more progressively, with a higher beating amplitude at the end of the monitoring duration.
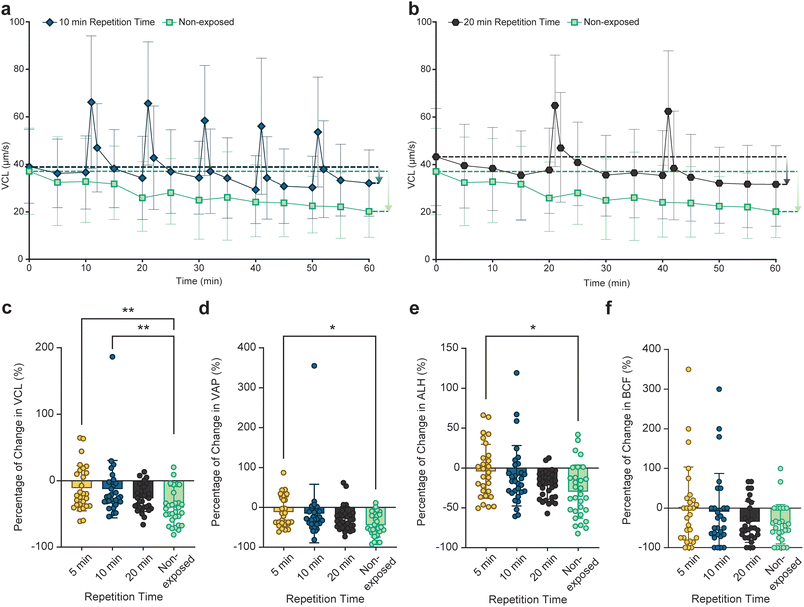 |
| Fig. 2 Comparison of average motility drop between individual non-exposed sperm cells and cells exposed to pulsed ultrasound with 5-, 10-, and 20-minute repetition times. (a) VCL profile for sperm cells exposed to 10-minute repetition time pulsed exposure (5 pulses, each lasting 10 seconds, with the repetition time of 10 minutes, n = 30 at each tracking period, and Power (1-β) = 99.99% for a total sample size of 690 and α = 0.05) versus non-exposed sperm (n = 30 at each tracking period). (b) VCL profile for sperm cells exposed to 20-minute repetition time pulsed exposure (2 pulses, each lasting 10 seconds, with the repetition time of 20 minutes, n = 30 at each tracking period, and Power (1-β) = 99.99% for a total sample size of 510 and α = 0.05) versus non-exposed sperm (n = 30 at each tracking period). (c–f) Comparison between 5, 10, and 20-minute groups in terms of the percentage of change in sperm motility parameters at t = 60 minutes with respect to motility parameters at t = 0 minutes for VCL, VAP, ALH, and BCF, respectively (n = 30 at each group). Statistical significance was determined using ordinary one-way ANOVA with Tukey's multiple-comparison test (* P ≤ 0.05 and ** P ≤ 0.01). | |
To evaluate the effect of pulsed ultrasound on sperm with different initial motilities, we graded the sperm cells in the 5-minute group by their initial straight-line velocities (VSL) into grade B with 5 μm s−1 ≤ VSL < 25 μm s−1 and grade C with VSL < 5 μm s−1 based on the criteria provided by the World Health Organization.59Fig. 3 compares the variations in motility parameters for the grade B and grade C sperm in the 5-minute group (statistical significance of VCL profiles detailed in Tables S5 and S6†). Percentage changes in motility parameters for encapsulated sperm in both grades, as well as non-exposed sperm, at t = 60 minutes relative to initial values at t = 0 minutes, are presented in Fig. 3b (for VCL), Fig. 3d (for VAP), Fig. 3f (for AHL), and Fig. 3h (for BCF). Our findings indicate that pulsed ultrasound more effectively preserves motility parameters in grade C sperm, resulting in a lower decline compared to grade B sperm. Notably, grade C sperm exposed to the 5-minute repetition time pulsed ultrasound exhibited a significantly lower decrease in VCL, VAP, and ALH compared to non-exposed sperm. Remarkably, grade C sperm exposed to the pulsed ultrasound displayed a 3% and 27% increase in average ALH and BCF at the end of the monitoring duration compared to initial values, respectively.
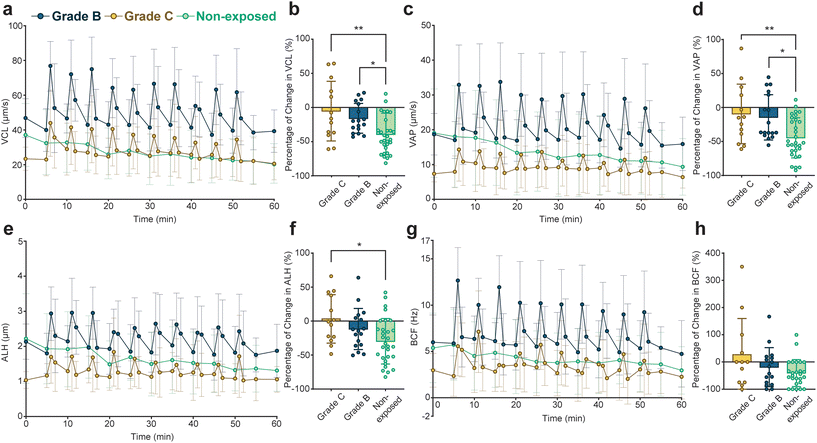 |
| Fig. 3 Temporal variations in motility parameters over 60 minutes for individual grade B and C sperm cells exposed to the 5-minute repetition time pulsed ultrasound. (a) VCL, (c) VAP, (e) ALH, and (g) BCF motility parameters for the grade B (n = 17 for each tracking period and Power (1-β) = 99.99% for all of the motility parameters, with a total sample size of 561 and α = 0.05) and C (n = 13 for each tracking period and Power (1-β) = 99.99% for all of the motility parameters, with a total sample size of 429 and α = 0.05) sperm cells exposed to the 5-minute repetition time pulsed ultrasound over 60 minutes versus non-exposed sperm cells. Comparison between grade B and C sperm cells in terms of the percentage of change in motility parameters at t = 60 minutes with respect to motility parameters at t = 0 minutes for (b) VCL, (d) VAP, (f) ALH, and (h) BCF, respectively. Statistical significance was determined using one-way ANOVA with Tukey's multiple-comparison test (* P ≤ 0.05, ** P ≤ 0.01). | |
In addition to finding the motility at the end of the 1-hour monitoring duration, we examined the motility dynamics of sperm cells throughout the monitoring duration. The time-average VCL, VAP, ALH, and BCF values of each sperm cell in 5, 10, and 20-minute groups as well as non-exposed sperm cells were calculated. As shown as an example in Fig. 4a, the time-average VCL of individual sperm cells before (area shown by green patterns) and after (area shown by red patterns) applying the pulsed ultrasound was calculated by integrating the VCL curve for each sperm. Corresponding time-average values for individual non-exposed sperm were also calculated (depicted by dotted pattern areas in Fig. 4a). Although sperm cells in the 5-minute group exhibited a significant rise in the time-average VCL post-exposure, non-exposed sperm showed a significant decline (Fig. 4b). Furthermore, sperm cells in both the non-exposed and the 5-minute group displayed similar time-average VCL of 35 μm s−1 in 0 ≤ t ≤ 5 minutes (the time before the first pulse for the 5-minute pulsed exposure setup, Fig. 4c). However, in 5 ≤ t ≤ 60 minutes, sperm cells exposed to the 5-minute repetition time pulsed ultrasound showed a higher time-average VCL of 39 μm s−1 compared to non-exposed sperm cells with the time-average VCL of 26 μm s−1. Comparisons between time-average motility parameters in all of the exposure setups and non-exposed sperm are presented in Fig. S4.†
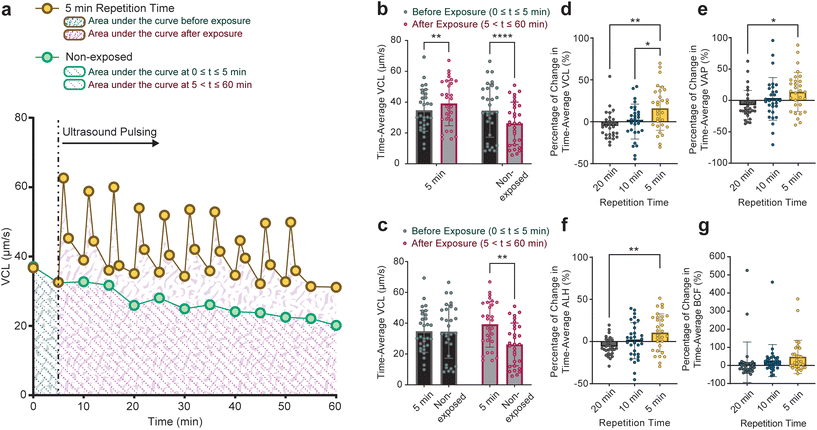 |
| Fig. 4 Comparison of time-average motility parameters between sperm cells exposed to pulsed ultrasound and non-exposed sperm. (a) The area under the curves of the motility graphs (e.g., VCL as depicted in this figure) of sperm exposed to the pulsed ultrasound and non-exposed sperm was used to calculate the time-average motility parameters. Before-exposure time-average VCL was derived by the integral of the graph at 0 ≤ t ≤ 5 minutes and the corresponding after-exposure value was obtained by the integral of VCL at 5 ≤ t ≤ 60 minutes (before exposure: , and after exposure: ). (b) Comparison of time-average VCL between 0 ≤ t ≤ 5 minutes (before exposure) and 5 ≤ t ≤ 60 minutes (after exposure) for sperm cells exposed to the 5-minute pulsed ultrasound and non-exposed sperm (n = 30 for the 5-minute and non-exposed groups, before and after exposure). (c) Comparison of time-average VCL between sperm cells in the 5-minute group and non-exposed sperm, before and after exposure (n = 30 for the 5-minute and non-exposed groups, before and after exposure). (d–g) Comparison of the percentage change in time-average motility parameters for the 5-, 10-, and 20-minute groups for VCL, VAP, ALH, and BCF, respectively (n = 30 for each group). Values are reported as mean ± s.d. Statistical significance for the comparison of time-average VCL (b and c) was determined using two-way ANOVA with Šídák's multiple comparisons test, while for the comparison of percentage change in motility parameters (d–g), one-way ANOVA with Tukey's multiple comparisons test was employed (* P ≤ 0.05, ** P ≤ 0.01, and **** P ≤ 0.0001). | |
The percentage change in time-average motility parameters following exposure (5 ≤ t ≤ 60 for the 5-minute, 10 ≤ t ≤ 60 for the 10-minute, and 20 ≤ t ≤ 60 for the 20-minute groups) with respect to pre-exposure time-average values is shown in Fig. 4d–g. These figures show that the percentage of change in time-average motility parameters is tendentially linked to the repetition rate of the ultrasound pulses, with the highest increase for the 5-minute. Notably, sperm cells in the 5-minute group experienced a rise in time-average motility parameters relative to corresponding pre-exposure values. The enhancement of sperm motility parameters upon exposure to pulsed ultrasound contributes to an improvement in sperm motility grading. The motility grading of sperm cells in the 5, 10, and 20-minute groups shown in Fig. S5† shows that a higher ultrasound exposure rate correlates with a higher quality of sperm grading.
To evaluate the applicability of the pulsed ultrasound for use in assisted reproduction, we conducted a biocompatibility assay by exploring the viability, and DNA integrity of sperm cells after 1 hour of being exposed to the 5-minute repetition time pulsed ultrasound. Sperm viability and DNA integrity are two crucial factors contributing to the transmission of genetic information.60 Results shown in Fig. 5 reveal that pulsed ultrasound exposure has no detrimental effects on viability or DNA integrity.
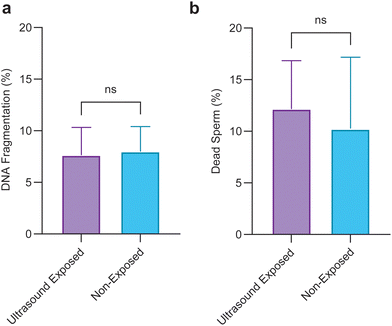 |
| Fig. 5 Assessment of the non-invasiveness of pulsed ultrasound. Sperm cells exposed to a 5-minute repetition time pulsed ultrasound were compared with non-exposed sperm. (a) Percentage of DNA fragmentation for the ultrasound-exposed and non-exposed sperm (n = 3708 sperm for the non-exposed group and n = 1984 sperm for the ultrasound-exposed group). (b) Percentage of viability for the ultrasound-exposed and non-exposed sperm (n = 477 sperm for the non-exposed group and n = 787 sperm for the ultrasound-exposed group). Values are reported as mean ± s.d. Statistical significance was determined using paired t-test (ns denotes non-significant). | |
3. Discussion
Through performing a single cell analysis facilitated by our droplet acoustofluidic platform, we showed that sperm motility boost caused by a 10-second single pulse ultrasound operating at 800 mW and 40 MHz shows a significant decline after 5 minutes post exposure (Fig. S2†). Given that assisted reproduction methods mainly require relatively longer preparation and incubation times, we explored the efficacy of utilizing multi-pulse exposure in providing a long-lasting enhancement to sperm motility. Regular tracking of individual sperm cells in each exposure setup for 1 hour revealed that a higher rate of the pulsed exposure leads to less of a decline in sperm motility after the 1-hour monitoring duration. Compared to non-exposed sperm cells, sperm cells exposed to pulsed ultrasound with 5, 10, and 20-minute repetition times showed 30%, 28%, and 14% improvement in average VCL at the end of the 1-hour monitoring duration respectively. A comprehensive understanding of the mechanisms underlying the regulation of sperm motility by pulsed ultrasound necessitates a detailed investigation into cellular processes, including respiration, substrate consumption, and ion channel modulation in sperm cells following ultrasound exposure.
Sperm utilize processes for metabolism regulation in response to cell functions and environmental stresses.22 Ultrasound may regulate sperm metabolic activity by enhancing ATP consumption or supply. Our results show that a higher rate of ultrasound exposure in a 1-hour duration leads to less of a decline in sperm motility at the end of the duration (Fig. 2). In addition, we showed that a higher rate of ultrasound exposure results in a higher time-average motility during the 1-hour duration (Fig. 4). To discern information about the intercellular mechanisms underlying sperm response to pulsed ultrasound, it is important to note two key points. Firstly, the heightened motility induced by ultrasound might be attributed to a heightened rate of ATP consumption. Secondly, given that sperm can sustain a higher time-average motility over 1 hour of pulsed exposure proportional to the exposure rate, if this effect is attributed to an increase in flagellar ATP hydrolysis, then a higher rate of ATP supply is also required. This increase in the rate of ATP supply could be triggered by the activation of sperm membrane mechanosensitive ion channels that result in a higher mitochondrial Ca2+ uptake as a key regulator of sperm metabolic activity61 which activates matrix dehydrogenases to adapt oxidative phosphorylation substrate supply for ATP needs.62–64
To comprehensively characterize the temporal effects of ultrasound on sperm motility and discern potential sperm bioenergetic responses to pulsed ultrasound, we employed nonlinear regression analysis on individual sperm cell curvilinear velocity (VCL) profiles to evaluate boost, peak VCL, plateaued VCL, and half-life across different exposure rates (Fig. 6, S6 and S7†). The average peak and plateau VCL values decreased over time across all exposure setups (Fig. 6b and c), and sperm cells subjected to 10 and 20-minute exposure repetition exhibited higher average boost and peak VCL values compared to those exposed to 5-minute repetition (Fig. 6d and e). These findings suggest that while a higher rate of pulsed ultrasound exposure may sustain sperm motility more effectively, it compromises the ability of sperm cells to exhibit spontaneous motility enhancement in response to pulsed exposure. Furthermore, we determined the half-life values of average VCL profiles at boost incidents for all exposure setups, as outlined in Fig. S7.† Half-life values, obtained via nonlinear regression analysis, show the rate of decline in VCL over time after exposure. As shown in Fig. S8,† sperm cells in the 5-minute group exhibited half-life values of an average of 0.72 minutes. Conversely, sperm cells in the 10 and 20-minute groups displayed average half-life values of 0.50, and 0.59 minutes, respectively. Notably, the average half-life values showed an inverse relationship with average boost values, indicating that a higher boost is associated with a more rapid decline in peak VCL. These insights underscore the intricate interplay between ultrasound exposure rate, sperm motility dynamics, and cellular energetics, warranting further investigation into the underlying mechanisms driving these observations.
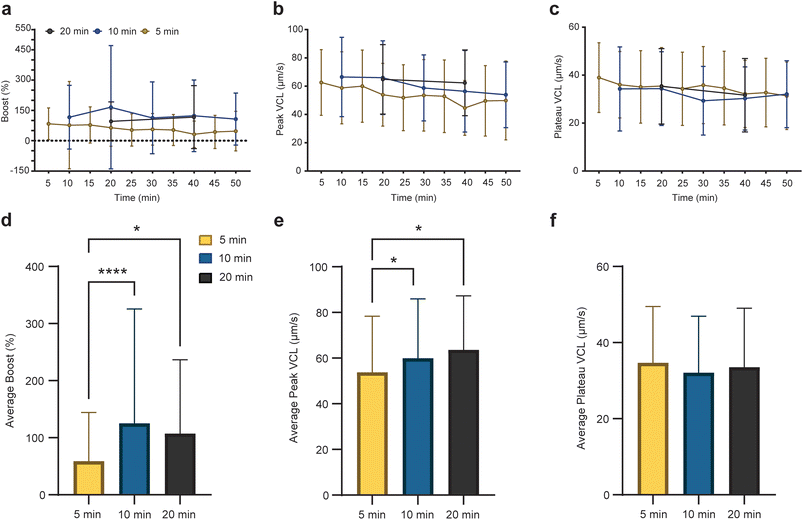 |
| Fig. 6 Characterisation of boost parameters for the sperm cells exposed to pulsed ultrasound by applying a nonlinear regression. (a) Boost in VCL, (b) peak VCL, and (c) plateau VCL profiles for sperm cells subjected to 5-,10-, and 20-minute exposure repetition times (n = 30 at each tracking period). Comparison of average (d) boost in VCL, (e) peak VCL, and (f) plateau VCL at all tracking periods between 5-,10-, and 20-minute exposure repetition times (n = 300 for 5-min, n = 150 for 10-min, and n = 60 for 20-min). Values are reported as mean ± s.d. Statistical significance was determined using one-way ANOVA with Tukey's multiple comparisons test (* P ≤ 0.05, ** P ≤ 0.01). | |
Maintaining sperm motility by utilizing pulsed ultrasound offers extensive applications for use in assisted reproduction. In standard assisted reproduction procedures, semen samples undergo liquefaction, washing, and preparation steps.65,66 Sperm pre-incubation is then conducted to facilitate sperm capacitation and acrosome reaction, processes essential for egg binding and fusion. While the duration of pre-incubation can vary, studies suggest that it typically ranges from 1 to 4 hours, depending on individual clinic protocols and the specific needs of the patient.67,68 Our pulsed exposure platform is promising for maintaining the motility of sperm cells during sperm preparation and incubation steps. Enhanced sperm motility is anticipated to lead to improved fertilization rates in assisted reproduction.69 More specifically, our results reveal that pulsed ultrasound proves more effective in maintaining the motility of non-progressive (grade C) sperm cells (Fig. 3). This highlights the potential efficacy of using pulsed ultrasound for the treatment of semen samples from patients suffering from asthenozoospermia or testicular samples of non-obstructive azoospermia patients. Moreover, the results show that pulsed ultrasound has the capability to induce motility in immotile sperm multiple times although these immotile sperm cells were unable to maintain their motility post-exposure (see ESI Movie S2†). This emphasises the capacity of pulsed ultrasound for detecting live immotile sperm for use in ICSI. However, pulsed ultrasound exposure exceeding one hour requires further assessment to confirm its biocompatibility.
4. Conclusions
Collectively, our single-cell analysis demonstrates that pulsed ultrasound application mitigates the decline in sperm motility observed over a 1-hour monitoring duration. Notably, the most substantial improvement, with a 30% increase in VCL compared to non-exposed sperm cells, is associated with the highest exposure rate of 5-minute repetition time. Moreover, our approach enhances the motility of poorly motile grade C sperm by 36% in VCL compared to non-exposed sperm. These findings hold promise for employing pulsed ultrasound during the time-consuming stages of sperm preparation and application in assisted reproduction, particularly for semen samples with suboptimal motility. Enhanced sperm motility facilitates clinicians in selecting less invasive assisted reproduction techniques, informed by natural sperm selection, thereby improving fertilization outcomes. Furthermore, pulsed ultrasound's ability to stimulate motility in immotile sperm multiple times suggests its potential utility in clinics for identifying viable sperm for use in ICSI when it comes to more challenging and severe infertility cases. Integrating this pulsed ultrasound technique into existing clinical workflows for IVF could involve incorporating it within microfluidic sperm selection devices. In addition, the repeated ultrasound system could be integrated into clinical workflows during stages where sperm samples are incubated or undergo procedures such as swim-up, both of which require a stationary period for sperm selection and processing. This setup would allow ART labs to enhance sperm motility before IVF procedures, with minimal adjustments to existing protocols and workflows. Future studies will aim to investigate the underlying mechanisms of motility enhancement by examining ATP production and mechanosensitive ion channel activity more directly. Employing metabolic inhibitors and ion channel blockers can help to isolate and evaluate the specific impact of different pathways on sperm motility. Also, it may aim to translate this technology for clinical applications that include exploring egg and embryo safety and evaluating the impact of pulsed ultrasound on testicular samples. Beyond assisted reproduction, this pulsed ultrasound technique could potentially be adapted to enhance motility or mechanosensitive activation in other cell types, such as immune cells for cancer therapies or stem cells in regenerative medicine. Further research in these contexts may reveal additional therapeutic applications.
5. Materials and methods
Device fabrication
LayoutEditor™ software was used to draw lithography mask schematics for both the droplet microfluidic mold and the interdigital transducers (IDTs). The 60 μm height droplet microfluidic mold comprised a flow-focusing junction for droplet generation and sperm encapsulation followed by a lozenge expansion chamber of 2800 × 2800 μm for encapsulated sperm assembly fabricated in SU-8 negative photoresist with which the Polydimethylsiloxane (PDMS) replicas were fabricated. To fabricate PDMS devices, a 10
:
1 ratio of the silicone elastomer base (SYLGARD™ 184, Dow Corning, MI, USA) and the curing agent (SYLGARD™ 184 silicone elastomer curing agent, Dow Corning, MI, USA) were mixed and cast onto the master mold. Two pairs of opposing IDTs were patterned on a 128° Y-cut lithium niobite (LN) piezoelectric substrate by deposition of 10 nm Cr, 200 nm Au, and 1 nm Cr and utilizing the lift-off procedure. IDTs were aligned at 45° to the x-axis of the LN wafer to ensure equal electromechanical couplings. To enhance bonding to PDMS, 300 nm of SiO2 was deposited on the LN substrate and patterned IDTs. Finally, plasma-treated LN and PDMS parts were aligned and bonded together.
Experimental procedure
Our droplet microfluidic device comprised a middle inlet for the sperm sample stream and two side inlets for the biocompatible oil (Engineered oil (3M™ Novec™) supplemented with surfactant (Pico-Surf™ 1, Sphere Fluidics, UK)) stream to facilitate droplet generation and single-sperm encapsulation at the flow-focusing junction. Droplets containing individual sperm cells were suspended in an oil phase within the low flow expansion chamber to maintain droplet integrity, minimize evaporation, and enable stable imaging conditions. The streams were driven by the MFCS™-EZ Fluigent pressure pump to generate sufficient number of droplets containing sperm within the expansion chamber. By applying atmospheric pressure to the inlets and the outlet, the droplets could be maintained still within the chamber. Encapsulated sperm were imaged for 1 hour, with regular imaging conducted in 2-second periods of 35 frames per second separated by 5-minute intervals, and motility parameters for encapsulated sperm cells at each imaging period were calculated. For the exposed groups (20, 10, and 5-minute ultrasound exposure repetition times), two additional tracking periods 5 seconds (ensuring that streaming inside droplets had ceased) and 1 minute post exposure were also included to enhance after-exposure time resolution. Microscope imaging was conducted using a 10× magnification objective by an inverted fluorescent microscope (Olympus IX83, Japan) equipped with an ORCA-Flash4.0 Digital CMOS camera (Hamamatsu Photonics, Japan). F20 powerSAW (Belektronig, Germany) was used to actuate IDTs with a sinusoid RF signal operating at 40 MHz and 800 mW for 10 seconds, leading to the generation of surface acoustic waves (SAWs). Actuation of opposing IDTs generates a standing wave pattern with periodic high-energy zones, which are relatively smaller than droplet size, along the central axis of the chamber due to constructive interference. This configuration enhances the acoustic field uniformity near the chamber center. However, due to natural attenuation over the width of the chamber, the acoustic energy density decreases toward the edges, leading to a non-uniformity in energy distribution across the chamber width.
Sperm motility was analyzed in droplets positioned at various locations across the chamber to provide a representative sampling of motility across the acoustic field. The influence of specific droplet distances from the IDTs on motility was not assessed, as our focus was on evaluating overall motility preservation across the chamber. In addition, sperm motility analysis was conducted only after confirming that acoustic streaming and droplet movement had ceased, ensuring that measurements reflected the direct effects of acoustic waves on sperm motility. ESI† Movie S3 demonstrates a representative streaming effect during acoustic exposure and its cessation prior to motility tracking. To control for potential thermal effects on sperm cells, we continuously monitored the temperature using a forward-looking infrared (FLIR) thermal camera within the chamber during ultrasound exposure, confirming that it remained within physiological limits.
Sperm preparation
Healthy human participants contributed to this study by donating fresh semen samples after 2 days of sexual abstinence, with prior informed consent from donors and approval from the Monash University Human Research Ethics Committee (MUHREC). The approval was granted under a license issued by the Australian National Health and Medical Research Council (NHMRC) Embryo Research Licensing Committee (ERLC) which administers the regulatory framework for human embryo research established by the Research Involving Human Embryos Act 2002 (RIHE Act) and the Prohibition of Human Cloning for Reproduction Act 2002 (PHCR Act). In this study, a total of six biological replicates with relatively stable motility and concentration parameters from three human donors were used. After the collection of the semen samples, they were incubated at 37 °C for 30 min to liquefy. To reach the optimum sperm concentration of 1.4 million cells per ml required to facilitate single sperm encapsulation, semen samples were diluted with HEPES-buffered salt solution (117 mM NaCl, 5.3 mM KCl, 2.3 mM CaCl2, 0.8 mM MgSO4, 1 mM NaH2PO4, 5.5 mM D-glucose, 0.03 mM phenol red, 4 mM NaHCO3, 21 mM HEPES, 0.33 mM Na pyruvate, 21.4 mM Na lactate) supplemented with 1 mg ml−1 polyvinyl alcohol.
Live/dead assay
To explore the effect of pulsed ultrasound on sperm viability the LIVE/DEAD™ Sperm Viability Kit (Invitrogen, USA) was used. This kit was employed to differentiate live sperm, which fluoresce green due to staining with DNA binding SYBR-14, from dead sperm, which exhibit red fluorescence upon staining with propidium iodide due to compromised membrane permeability. To stain sperm cells, prepared sperm samples were supplemented with 5 μL of 5 mM SYBR-14 and 5 μL of 2.4 mM propidium iodide. After incubation for 10 minutes at 37 °C in the dark, fluorescent microscopy (Olympus IX83, Japan) with FITC filter was used to identify live sperm emitting green fluorescence (∼516 nm), and dead sperm emitting red fluorescence (∼617 nm) were identified using CY3 filter.
DNA integrity assay
To evaluate the effect of pulsed ultrasound on the DNA integrity of sperm cells, the SpermFunc® DNAf test kit (BRED Life Science Technology Inc., Shenzhen, P.R.C) including tubes with low melting point gel, solution A, solution B, Wright's stain, and Wright's buffer was used to explore the DNA fragmentation of the cells based on the Sperm Chromatin Dispersion (SCD) method. Initially, 60 μL of the sperm sample was dispensed into a tube containing 140 μL of low-melting-point agarose gel and thoroughly mixed. Subsequently, 30 μL of the prepared sperm-gel mixture was dispensed onto a glass slide coated with agarose, which was pre-chilled at 2–8 °C. The slides with exposed and non-exposed sperm were then refrigerated at 2–8 °C for 5 minutes before being transferred to containers containing solution A, where they were incubated for 7 minutes at room temperature. Following this step, the slides were immersed in solution B and incubated for 25 minutes at room temperature. Afterwards, they were horizontally immersed in deionized water for 5 minutes. The slides were sequentially immersed in 70%, 90%, and 100% v/v ethanol solutions blended with deionized water, with each concentration being applied for 2 minutes. After air-drying the slides at room temperature, 15–20 drops of Wright's stain were applied, followed by dispensing 30–40 drops of Wright's buffer onto the slides. The mixture of stain and buffer was then gently blown across the slides for 15 minutes. Subsequently, the slides were gently rinsed with deionized water. The slides containing sperm samples were then examined using an inverted fluorescent microscope (Olympus IX83, Japan). Sperm cells displaying large halos (with a diameter greater than 1/3 of the sperm head's thinnest diameter) were indicative of DNA integrity, whereas those with no halo or a small halo (with a diameter less than 1/3 of the sperm head's thinnest diameter) indicated DNA fragmentation. The DNA fragmentation index was calculated by determining the ratio of sperm cells with fragmented DNA to the total number of sperm cells.
Motility analysis
Sperm tracking was performed using the manual tracking plugin in ImageJ to monitor the position of the sperm head in each recorded frame. Subsequently, a custom MATLAB code70 was employed to quantify various motility parameters of individual sperm based on their 2D head positions and the frame rate. These parameters, as defined by the World Health Organization59 are illustrated in Fig. S1a† and include average curvilinear velocity (VCL), average path velocity (VAP), average amplitude of head displacement (ALH), average head beating frequency (BCF), and average straight-line velocity (VSL).
Statistical analysis
A power analysis based on preliminary data from temporal changes of sperm motility parameters was conducted to determine the sample size required to detect significant differences in sperm motility with 80% power at a 0.05 significance level. This analysis informed the sample size used in the study, allowing for robust statistical power to support the validity of our findings. To evaluate the statistical significance of the results, Prism software (version 9) was used. One-way ANOVA matched values with Tukey's multiple-comparison test was used to determine the statistical significance of VCL, VAP, ALH, and BCF profiles as shown in Fig. 1–3, S2 and S7.† Statistical significance for comparison between time-averaged VCL (Fig. 4b and c and S4†) was determined using two-way ANOVA matched values with Šídák's multiple comparisons test. Unpaired T-test was used to determine the statistical significance of DNA fragmentation and viability results shown in Fig. 5. One-way ANOVA matched values with Tukey's multiple-comparison test was used to evaluate the statistical significance of the boost, peak, and plateau profiles shown in Fig. 6. Statistical significance of comparison between exposure setups in terms of cumulative boost, peak, and plateau was obtained using one-way ANOVA with Tukey's multiple-comparison test. P less than 0.05 was considered significant (*P ≤ 0.05, **P ≤ 0.01, ***P ≤ 0.001, ****P ≤ 0.0001). Sample size and power analyses were conducted using G*Power software (version 3.1).
Code availability
MATLAB version 9.7.0.1190202 (R2019b) was used to generate custom scripts to analyze the motility parameters for the tracked sperm and reconstruct the swimming trajectory. Please contact the corresponding authors for access.
Data availability
All microscopy images and relevant data supporting the findings of this study are available within the article and its ESI† files or upon request from the corresponding authors.
Conflicts of interest
The authors declare the following competing interests: A. N. and R. N. have a patent in the use of ultrasound to increase sperm motility.
Acknowledgements
This work was supported by the Australian Research Council (ARC) Discovery Project Grant (DP210103361 to A. N. and R. N), Australian National Health and Medical Research Council (NHMRC) fellowship (Investigator Grant 2017370 to R. N.), Monash Data Futures Institute Seed Grant (to R. N.). This work was performed in part at the Melbourne Centre for Nanofabrication (MCN) in the Victorian Node of the Australian National Fabrication Facility (ANFF).
References
- E. Wallach, R. D. Amelar, L. Dubin and C. Schoenfeld, Fertil. Steril., 1980, 34, 197–215 CrossRef PubMed.
- R. E. Anderson, H. A. Hanson, D. Thai, C. Zhang, A. P. Presson, K. I. Aston, D. T. Carrell, K. R. Smith and J. M. Hotaling, J. Assist. Reprod. Genet., 2018, 35, 793–799 CrossRef PubMed.
- A. Shulman, R. Hauser, S. Lipitz, Y. Frenkel, J. Dor, D. Bider, S. Mashiach, L. Yogev and H. Yavetz, J. Assist. Reprod. Genet., 1998, 15, 381–385 CrossRef CAS PubMed.
- M. T. Villani, D. Morini, G. Spaggiari, A. I. Falbo, B. Melli, G. B. La Sala, M. Romeo, M. Simoni, L. Aguzzoli and D. Santi, Andrology, 2022, 10, 310–321 CrossRef.
- F. Boitrelle, R. Shah, R. Saleh, R. Henkel, H. Kandil, E. Chung, P. Vogiatzi, A. Zini, M. Arafa and A. Agarwal, Life, 2021, 11, 1–13 CrossRef PubMed.
- D. Sakkas, M. Ramalingam, N. Garrido and C. L. R. Barratt, Hum. Reprod. Update, 2015, 21, 711–726 CrossRef CAS PubMed.
- R. P. Dickey, R. Pyrzak, P. Y. Lu, S. N. Taylor and P. H. Rye, Fertil. Steril., 1999, 71, 684–689 CrossRef CAS PubMed.
- H. W. Michelmann, Int. J. Androl., 1995, 18, 81–87 Search PubMed.
- A. Aboukhshaba, N. Punjani, S. Doukakis, N. Zaninovic, G. Palermo and P. N. Schlegel, Fertil. Steril., 2022, 117, 522–527 CrossRef PubMed.
- C. Ortega, G. Verheyen, D. Raick, M. Camus, P. Devroey and H. Tournaye, Hum. Reprod. Update, 2011, 17, 684–692 CrossRef CAS PubMed.
- P. Rubino, P. Viganò, A. Luddi and P. Piomboni, Hum. Reprod. Update, 2015, dmv050 CrossRef PubMed.
- D. Mortimer, C. L. R. Barratt, L. Bjorndahl, C. de Jager, A. M. Jequier and C. H. Muller, Hum. Reprod. Update, 2013, 19, i1–i45 CrossRef PubMed.
- H. Tournaye, M. Van Der Linden, E. Van Den Abbeel, P. Devroey and A. Van Steirteghem, Hum. Reprod., 1993, 8, 1475–1480 CrossRef CAS.
- L. Scott and S. Smith, Fertil. Steril., 1995, 63, 166–175 CrossRef CAS.
- R. E. Jackson, C. L. Bormann, P. A. Hassun, A. M. Rocha, E. L. A. Motta, P. C. Serafini and G. D. Smith, Fertil. Steril., 2010, 94, 2626–2630 CrossRef CAS.
- A. Agarwal, I. Ikemoto and K. R. Loughlin, Arch. Androl., 1994, 33, 157–162 CrossRef CAS PubMed.
- K. Inaba, Mol. Hum. Reprod., 2011, 17, 524–538 CrossRef CAS PubMed.
- A. Amaral, WIREs Mechanisms of Disease, 2022, 14, 1–20 CrossRef PubMed.
- E. Ruiz-Pesini, C. Díez-Sánchez, M. J. López-Pérez and J. A. Enríquez, Curr Top Dev Biol, 2007, 77, 3–19 CrossRef CAS PubMed.
-
C. M. O'Connor, J. U. Adams and J. Fairman, Essentials of cell biology, NPG Education, Cambridge, MA, 2010, vol. 1, pp. 19–20 Search PubMed.
- M. Bonora, S. Patergnani, A. Rimessi, E. de Marchi, J. M. Suski, A. Bononi, C. Giorgi, S. Marchi, S. Missiroli, F. Poletti, M. R. Wieckowski and P. Pinton, Purinergic Signal, 2012, 8, 343–357 CrossRef CAS PubMed.
- F. J. Peña, J. M. Ortiz-Rodríguez, G. L. Gaitskell-Phillips, M. C. Gil, C. Ortega-Ferrusola and F. E. Martín-Cano, Anim Reprod Sci, 2022, 246, 106805 CrossRef PubMed.
- M. Zaferani, S. S. Suarez and A. Abbaspourrad, Proc. Natl. Acad. Sci. U. S. A., 2021, 118(44), e2107500118 CrossRef CAS PubMed.
- J. K. Voglmayr, B. P. Setchell and I. G. White, J. Reprod. Fertil., 1971, 24, 71–80 CrossRef CAS PubMed.
- K. Saito, Y. Kinoshita and M. Hosaka, International Journal of Urology, 1999, 6, 196–199 CrossRef CAS PubMed.
- N. Bernabò, E. Tettamanti, M. G. Pistilli, D. Nardinocchi, P. Berardinelli, M. Mattioli and B. Barboni, Theriogenology, 2007, 67, 801–815 CrossRef PubMed.
- Y. Liu, Z. Yi, Y. Yao, B. Guo and X. Liu, Acc Mater Res, 2022, 3, 247–258 CrossRef CAS.
- Q. Huang, W. Zhu, X. Gao, X. Liu, Z. Zhang and B. Xing, Adv. Drug Delivery Rev., 2023, 195, 114763 CrossRef CAS PubMed.
- E. Pinart, Int. J. Mol. Sci., 2022, 23(11), 5880 CrossRef PubMed.
- K. Nowicka-Bauer and M. Szymczak-Cendlak, Int. J. Mol. Sci., 2021, 22(6), 3259 CrossRef CAS PubMed.
- J. Gai, E. Dervisevic, C. Devendran, V. J. Cadarso, M. K. O'Bryan, R. Nosrati and A. Neild, Adv. Sci., 2022, 9, 2104362 CrossRef PubMed.
- B. Ang, R. Habibi, C. Kett, W. H. Chin, J. J. Barr, K. L. Tuck, A. Neild and V. J. Cadarso, Sens Actuators B Chem, 2023, 374, 132769 CrossRef CAS.
- C. Richard, E. J. Vargas-Ordaz, Y. Zhang, J. Li, V. J. Cadarso and A. Neild, Lab Chip, 2024, 24(3), 480 RSC.
- L. Shen, Z. Tian, J. Zhang, H. Zhu, K. Yang, T. Li, J. Rich, N. Upreti, N. Hao, Z. Pei, G. Jin, S. Yang, Y. Liang, W. Chaohui and T. J. Huang, Biosens. Bioelectron., 2023, 224, 115061 CrossRef CAS PubMed.
- F. Guo, W. Zhou, P. Li, Z. Mao, N. H. Yennawar, J. B. French and T. J. Huang, Small, 2015, 11, 2733–2737 CrossRef CAS PubMed.
- B. Kang, J. Shin, H.-J. Park, C. Rhyou, D. Kang, S.-J. Lee, Y.-S. Yoon, S.-W. Cho and H. Lee, Nat. Commun., 2018, 9, 5402, DOI:10.1038/s41467-018-07823-5.
- M. Sesen, C. Devendran, S. Malikides, T. Alan and A. Neild, Lab Chip, 2017, 17, 438–447 RSC.
- N. Bose, X. Zhang, T. K. Maiti and S. Chakraborty, Biomicrofluidics, 2015, 9, 052609, DOI:10.1063/1.4928947.
- J. J. Kwan, R. Myers, C. M. Coviello, S. M. Graham, A. R. Shah, E. Stride, R. C. Carlisle and C. C. Coussios, Small, 2015, 11, 5305–5314 CrossRef CAS PubMed.
- L. Zhang, Z. Tian, H. Bachman, P. Zhang and T. J. Huang, ACS Nano, 2020, 14, 3159–3169 CrossRef CAS PubMed.
- M. Mohammadi, H. Zargartalebi, R. Salahandish, R. Aburashed, K. Wey Yong and A. Sanati-Nezhad, Biosens. Bioelectron., 2021, 183, 113176 CrossRef CAS PubMed.
- N. Hao, Z. Wang, P. Liu, R. Becker, S. Yang, K. Yang, Z. Pei, P. Zhang, J. Xia, L. Shen, L. Wang, K. A. Welsh-Bohmer, L. Sanders, L. P. Lee and T. J. Huang, Biosens. Bioelectron., 2022, 196, 113730 CrossRef CAS PubMed.
- A. Del Campo Fonseca and D. Ahmed, Adv. Drug Delivery Rev., 2024, 205, 115164 CrossRef CAS PubMed.
- C. Rubin, M. Bolander, J. P. Ryaby and M. Hadjiargyrou, J. Bone Joint Surg. Am., 2001, 83, 259–270 CrossRef CAS PubMed.
- M. S. Brugger, K. Baumgartner, S. C. F. Mauritz, S. C. Gerlach, F. Röder, C. Schlosser, R. Fluhrer, A. Wixforth and C. Westerhausen, Proc. Natl. Acad. Sci. U. S. A., 2020, 117, 31603–31613 CrossRef CAS PubMed.
- A. Fomenko, C. Neudorfer, R. F. Dallapiazza, S. K. Kalia and A. M. Lozano, Brain Stimul., 2018, 11, 1209–1217 CrossRef PubMed.
- A. K. W. Wood and C. M. Sehgal, Ultrasound Med. Biol., 2015, 41, 905–928 CrossRef PubMed.
- X. Jiang, O. Savchenko, Y. Li, S. Qi, T. Yang, W. Zhang and J. Chen, IEEE Trans. Biomed. Eng., 2019, 66, 2704–2718 Search PubMed.
- C. Devendran, J. Carthew, J. E. Frith and A. Neild, Adv. Sci., 2019, 6, 1902326, DOI:10.1002/advs.201902326.
-
C. Devendran and A. Neild, in Field-Driven Micro and Nanorobots for Biology and Medicine, Springer, 2022, pp. 61–90 Search PubMed.
-
J. Berthier, Acoustic methods for manipulating droplets, Micro-Drops and Digital Microfluidics, Micro & Nano Technologies Series, Elsevier, 2nd edn, 2013, ch. 13, pp. 457–492 Search PubMed.
- D. J. Collins, B. Morahan, J. Garcia-Bustos, C. Doerig, M. Plebanski and A. Neild, Nat. Commun., 2015, 6, 1–11 Search PubMed.
- M. R. Raveshi, M. S. Abdul Halim, S. N. Agnihotri, M. K. O'Bryan, A. Neild and R. Nosrati, Nat. Commun., 2021, 12, 3446, DOI:10.1038/s41467-021-23773-x.
- E. S. Richter, A. Link, J. S. McGrath, R. W. Sparrow, M. Gantz, E. J. Medcalf, F. Hollfelder and T. Franke, Lab Chip, 2022, 23, 195–202 RSC.
- S. N. Agnihotri, M. R. Raveshi, R. Bhardwaj and A. Neild, Microfluid. Nanofluid., 2021, 25, 88 CrossRef CAS.
- S. Wang, X. Lv, Y. Su, Z. Fan, W. Fang, J. Duan, S. Zhang, B. Ma, F. Liu, H. Chen, Z. Geng and H. Liu, Small, 2019, 15, 1804593, DOI:10.1002/smll.201804593.
- A. Vafaie, M. R. Raveshi, C. Devendran, R. Nosrati and A. Neild, Sci. Adv., 2024, 10, eadk2864 CrossRef CAS PubMed.
- J. Gai, R. Nosrati and A. Neild, Lab Chip, 2020, 20, 4262–4272 RSC.
- World Health Organization, WHO laboratory manual for the examination and processing of human semen Sixth Edition, 2021, vol. Edition, V.
- A. Agarwal and T. M. Said, Hum. Reprod. Update, 2003, 9, 331–345 CrossRef CAS PubMed.
- S. Meyers, E. Bulkeley and A. Foutouhi, Reprod. Domest. Anim., 2019, 54, 22–28 CrossRef PubMed.
- D. Poburko, J. Santo-Domingo and N. Demaurex, J. Biol. Chem., 2011, 286, 11672–11684 CrossRef CAS PubMed.
- R. M. Denton, Biochim. Biophys. Acta, Bioenerg., 2009, 1787, 1309–1316 CrossRef CAS PubMed.
- M. Szibor, Z. Gizatullina, T. Gainutdinov, T. Endres, G. Debska-Vielhaber, M. Kunz, N. Karavasili, K. Hallmann, F. Schreiber, A. Bamberger, M. Schwarzer, T. Doenst, H. J. Heinze, V. Lessmann, S. Vielhaber, W. S. Kunz and F. N. Gellerich, J. Biol. Chem., 2020, 295, 4383–4397 CrossRef CAS PubMed.
-
D. Mortimer, L. Björndahl, C. L. R. Barratt, J. A. Castilla, R. Menkveld, U. Kvist, J. G. Alvarez and T. B. Haugen, Sperm Preparation, A Practical Guide to Basic Laboratory Andrology, Cambridge University Press, 2022, ch. 9, pp. 194–217 Search PubMed.
- S. M. Knowlton, M. Sadasivam and S. Tasoglu, Trends Biotechnol., 2015, 33, 221–229 CrossRef CAS PubMed.
- S. Liu, G. Wu, L. Wang, Y. Zhao, Y. Lv, N. Dang and Y. Yao, Ginekol. Pol., 2023, 94, 107–112 CrossRef PubMed.
- R. T. Mansour, M. G. Serour, A. M. Abbas, A. Kamal, N. A. Tawab, M. A. Aboulghar and G. I. Serour, Fertil. Steril., 2008, 90, 584–591 CrossRef PubMed.
- E. T. Donnelly, S. E. M. Lewis, J. A. McNally and W. Thompson, Fertil. Steril., 1998, 70, 305–314 CrossRef CAS PubMed.
- R. Nosrati, A. Driouchi, C. M. Yip and D. Sinton, Nat. Commun., 2015, 6, 8703, DOI:10.1038/ncomms9703.
|
This journal is © The Royal Society of Chemistry 2025 |
Click here to see how this site uses Cookies. View our privacy policy here.