DOI:
10.1039/D4NR03819C
(Paper)
Nanoscale, 2025,
17, 314-321
Radiative energy transfer enabling upconverted circularly polarized persistent luminescence for multilevel information encryption†
Received
17th September 2024
, Accepted 15th November 2024
First published on 15th November 2024
Abstract
Optically active persistent luminescent materials are highly promising for anticounterfeiting applications due to their distinct luminescent features and the ability to display unique optical polarization properties. Despite significant progress in the development of circularly polarized persistent luminescence (CPPL) materials, the fabrication of upconverted circularly polarized persistent luminescence (UC-CPPL) materials remains a considerable challenge. In this study, we present an efficient strategy to construct UC-CPPL materials by embedding upconversion nanoparticles (UCNPs) and phosphors into chiral nematic liquid crystals (N*LC). The system operates through a radiative energy transfer mechanism between the UCNPs and phosphors. Upon excitation by low-energy near-infrared light (980 nm), the UCNPs emit high-energy ultraviolet light, which is effectively transferred to the phosphors, resulting in the emission of circularly polarized persistent visible light. By precisely tuning the photonic bandgap of the chiral N*LC, the UC-CPPL luminescence dissymmetry factor (gUC-CPPL) can be amplified to approximately 0.6. The concept of UC-CPPL was realized through the integration of three advanced optical properties: circularly polarized luminescence, long persistent luminescence, and upconversion luminescence. This integration enables more sophisticated and secure information encryption. The incorporation of upconversion materials facilitates the controlled concealment and selective release of encrypted information, while the multileveled encoding scheme further enhances the complexity and security of the encryption process, achieving true information hiding and encryption.
Introduction
The field of traditional optical information encryption has achieved significant maturity, with multilevel encryption emerging as a key frontier in advancement of optical information storage.1–3 Circularly polarized luminescent (CPL) materials offer a promising approach for enhancing the complexity and efficacy of anti-counterfeiting techniques due to their unique photon polarization characteristics.4–14 Organic materials capable of prolonged luminescence—lasting tens of milliseconds or more after the excitation source is removed—introduce a temporal dimension to the luminescence signal, influencing its intensity.15–21 When combined with chirality, such materials exhibit circularly polarized persistent luminescence (CPPL), opening up new possibilities for applications in optical anti-counterfeiting, information encryption, and bio-imaging.1,22–28 However, despite their potential, CPPL materials have been relatively underexplored for their anti-counterfeiting encryption, primarily due to their low luminescence dissymmetry factor (glum). While Zhuang et al. have developed CPL-based long-afterglow materials for security tagging, current dual-layer information systems still lack the complexity and diversity required to significantly elevate anti-counterfeiting security.29
Upconversion materials, capable of converting low-energy photons into high-energy ones, have become widely utilized in two-photon and ultraviolet devices, as well as in information science.9,30–33 Their ability to be excited by near-infrared light offers exciting new opportunities for enhancing the anti-counterfeiting and encryption capabilities of CPL materials.34–38 On one hand, invisible light excitation improves the decryption capability of these systems; on the other, near-infrared excitation upon.39–42 In this work, we integrate two luminescence mechanisms—photon upconversion and radiative energy transfer—to develop a novel class of upconverted circularly polarized persistent luminescence (UC-CPPL) materials for anti-counterfeiting encryption. By incorporating both the temporal and polarization dimensions of the emitted light, this system utilizes the exceptional optical properties of upconversion nanoparticles (UCNPs) and phosphors, which serve as energy donors and acceptors, respectively. The excitation energy of the phosphorescent material is derived from the upconverted photon of the UCNPs. Additionally, by tuning the photonic bandgap of chiral liquid crystal to overlap with the primary emission peaks of the phosphorescent material, we enhance the intensity of circularly polarized emission and improve the glum value. The energy transfer between UCNPs and phosphors serves as a crucial carrier for multilevel information encryption. This design enales the transmission of multi-color, multilevel information. As illustrated in Fig. 1b, by using a left-handed circular polarization filter (L-CPF) and a right-handed circular polarization filter (R-CPF), the UC-CPPL materials reveal a multilevel information pattern through the modulation of upconverted circularly polarized luminescence (UC-CPL) and UC-CPPL. Our research presents innovative materials and methodologies for advancing the application of circularly polarized luminescent materials in optical anti-counterfeiting and encryption technologies.
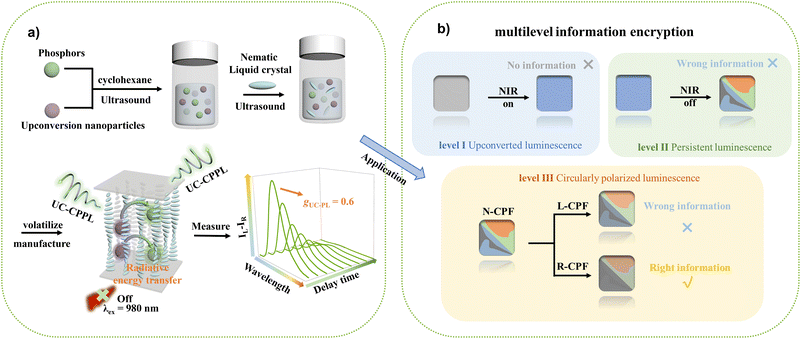 |
| Fig. 1 (a) Schematic illustration of upconverted circularly polarized persistent luminescence (UC-CPPL) achieved by integrating UCNPs and phosphors into N*LC. UCNPs serve as energy donors and phosphors as energy acceptors. Time-resolved UC-CPPL spectra in N*LC under 980 nm laser excitation, with a maximum gUC-PL value of 0.6. (b) Schematic representation of multilevel information encryption utilizing NIR excitation light and circularly polarization information. Note that the changes of pattern upon the excitation of NIR and in the afterglow state. In the persistent luminescence state, the pattern changes due to the change of brightness of different systems under different polarizers. Blue light under 980 nm laser excitation and multicolor afterglow emission after laser shutdown. Red, green, and blue light intensities vary with R-CPF and L-CPF. N-CPF, no polarized filter; R-CPF, right-handed circularly polarized filter; L-CPF, left-handed circularly polarized filter. | |
Results and discussion
Photoluminescence behavior in liquid crystal
The upconverted persistent emission is realized by combining one kind of UCNPs capable of emitting strong ultraviolet light under 980 nm laser excitation with a series of phosphors. To enhance the presentation of UC-CPPL performance, three commercial inorganic rare-earth phosphors were selected as the afterglow sources due to their stable and bright persistent luminescence. These phosphors emit red, green, and blue light at 630 nm, 520 nm, and 470 nm, respectively, and their long-persistent emission profiles are denoted as RP (red phosphors), GP (green phosphors), and BP (blue phosphors).29 The nematic liquid crystal (N*LC) SLC1717 was chosen as the chiral host because its photonic bandgap can be flexibly tuned by the chiral dopants R/S-811.37,43–45 First, we adjusted the photonic bandgaps of the SLC1717 liquid crystals to align with the emission wavelengths of the phosphors. We then doped the phosphors into the N*LC to assess their compatibility. Notably, excessive dopping of phosphors can disrupt the alignment of the liquid crystal textures (Fig. S1†). To prevent this, we maintained a fixed mass ratio of phosphors to N*LC at 3
:
10, ensuring the preservation of both the liquid crystal texture and the bright afterglow of phosphors. As shown in Fig. 2a, the photonic bandgaps of the N*LCs effectively overlap with the emission peaks of the corresponding doped phosphors, indicating that both the optical properties of the phosphors and the chiral N*LC are retained in the composite materials.
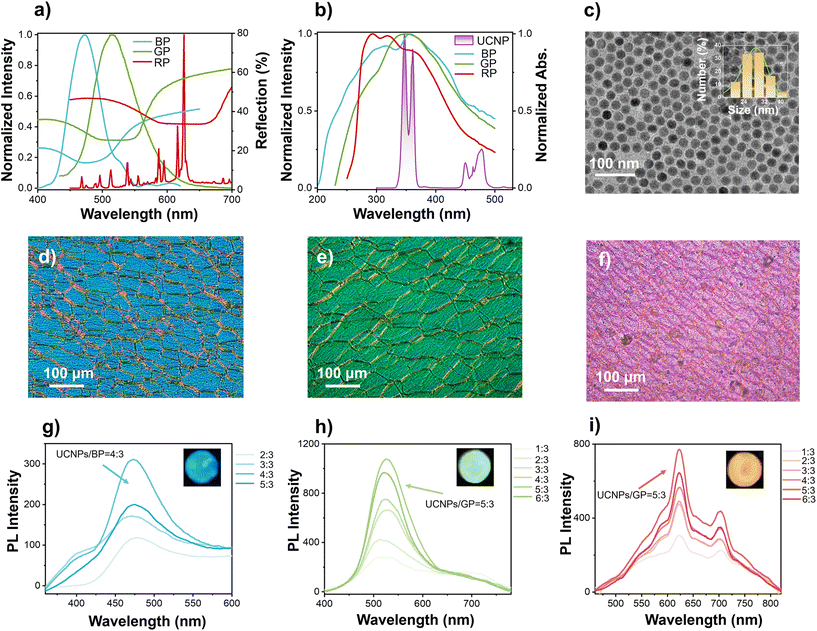 |
| Fig. 2 (a) Long afterglow emission spectra of phosphors in N*LC (left vertical axis) and the corresponding reflection spectra at the excitation of 360 nm. Central wavelengths of reflection bands for blue, green, and red phosphors/N*LC: 468, 522, and 617 nm, respectively (right vertical axis). (b) Normalized upconversion emission spectra of UCNPs in N*LC upon excitation of 980 nm laser ([UCNP] = 2 mg mL−1, power density: 200 W cm−2) (left vertical axis) and normalized UV-vis absorption spectra of BP, GP and RP, respectively (right vertical axis). (c) TEM image of NaYF4:Yb,Tm UCNPs and size distribution histogram of UCNPs obtained by DLS measurement. (d–f) Polarized optical microscopy (POM) images of blue, green, and red phosphors/UCNPs in N*LC under the crossed polarizers. (g) Photoluminescence intensity of UCNPs/BP in SLC1717 delayed by 0.1 s at different UCNPs/BP weight ratios (excited by 10 W laser, λex = 980 nm, power density: 200 W cm−2). (h) Photoluminescence intensity of UCNPs/GP in SLC1717 delayed by 0.1 s at different UCNPs/GP weight ratios (excited by 10 W laser, λex = 980 nm, power density: 200 W cm−2). (i) Photoluminescence intensity of UCNPs/RP in SLC1717 delayed by 0.1 s at different UCNPs/RP weight ratios (excited by 10 W laser, λex = 980 nm, power density: 200 W cm−2). | |
Subsequently, oleic acid (OA)-modified NaYF4:Yb,Tm UCNPs (UCNPs-Tm) were synthesized as a near-infrared (NIR) light collector to transfer energy to the phosphors and achieve upconversion persistent luminescence (Fig. S2†). Transmission electron microscopy (TEM) analyses confirmed the UCNPs-Tm morphology, while dynamic light scattering (DLS) measured their size at approximately 30 nm (Fig. 2c). These UCNPs exhibit two emission peaks at around 360 nm and 460 nm, which can be absorbed by the selected phosphors, enabling radiative energy transfer (Fig. 2b). To minimize any impact on the phosphorescent N*LC, we first prepared a homogeneous and stable mixture of UCNPs-Tm and phosphors via ultrasonic treatment. This mixture was then added to the liquid crystal solution, followed by solvent removal to fabricate the liquid crystal material. Polarized light microscopy (POM) images (Fig. 2d–f) confirmed a uniform texture in the liquid crystal complex, indicating excellent compatibility between the N*LC and the luminescent additives. In addition to the material compatibility, efficient radiative energy transfer between the donor (UCNPs) and acceptor (phosphors) in the liquid crystal matrix is crucial for optimizing the upconversion long-afterglow performance. Thus, we optimized the mass ratios of UCNPs-Tm to phosphors to achieve the strongest upconverted phosphorescence. As shown in Fig. 2g–i, upon 980 nm laser excitation, the optimal mass ratios of UCNPs-Tm to BP, GP, and RP for blue, green, and red afterglow were determined to be 4
:
3, 5
:
3, and 5
:
3, respectively.
CPPL in N*LC
To investigate the chiral properties of these materials, we conducted optical activity measurements using a circular dichroism (CD) spectrometer. Significant mirror-image signals were observed in the CD spectra, corresponding to selective reflections of various photonic bandgaps, confirming the helical arrangement of the LC molecules (Fig. S3†). These selective reflection and transmission characteristics promote circularly polarized emission from the luminophores embedded in the chiral N*LC. CPL measurements of the phosphor-doped chiral N*LC using a CPL spectrometer revealed opposite peaks at 470 nm, 520 nm, and 580 nm under 365 nm UV excitation. These peaks correspond to the blue, green, and red phosphors in R- or S-chiral N*LC, with calculated glum values of approximately ±0.1 (Fig. S4†). Additionally, the UCNPs exhibited symmetrical UC-CPL spectra in the chiral liquid crystal, with an emission peak at 480 nm, corresponding to the 1G4 → 3H6 transitions of Tm3+ ions. Notably, the UCNPs demonstrate a larger UC-CPL luminescence dissymmetry factor (∼±0.6) compared to the phosphors, attributed to the UCNPs’ regular morphology and the excellent compatibility between the liquid crystals and oleic acid ligands (Fig. S5†). Interestingly, the incorporation of UCNPs enhanced the CPL performance of the phosphorescent N*LC under 365 nm excitation. The CPL spectra of the CPPL materials (Fig. 3a–c) revealed corresponding glum values of ±0.3, ±0.6, and ±0.6 (Fig. S5†), which are much higher than ±0.1. These large values should be attributed to the ultrasonic pretreatment which can improve the compatibility between the nanomaterials and liquid crystals.
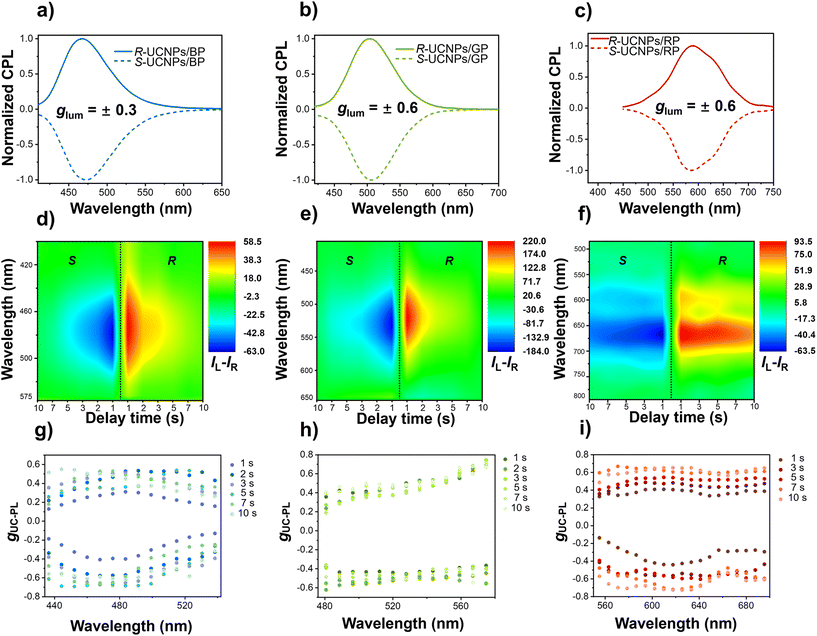 |
| Fig. 3 (a–c) Normalized CPL emission spectra of UCNPs/phosphors in N*LC under λex = 365 nm excitation: UCNPs/BP = 4 : 3; UCNPs/GP = 5 : 3; UCNPs/RP = 5 : 3 (weight ratio). (d–f) two-dimensional time-resolved UC-CPPL emission (IL–IR) spectra of UCNPs/BP, UCNPs/GP, UCNPs/RP in N*LC within 10 s (excited by 10 W laser, λex = 980 nm, power density: 200 W cm−2). (g–i) gUC-PL of UCNPs/BP, UCNPs/GP and UCNPs/RP in N*LC within 10 s (excited by 10 W laser, λex = 980 nm, power density: 200 W cm−2). | |
In CPPL-based information encryption, the time-dependent behavior of CPPL plays a critical role in accurately revealing hidden information. To explore this, we investigated the time-dependent CPPL performance of chiral luminescent materials using a custom-built circularly polarized luminescence detection system. For real-time CPPL detection, we employed a charge-coupled device (CCD) spectrometer paired with a programmable time delay (PTD) generator, allowing rapid acquisition of emission spectra and synchronized control of both the excitation source and CCD. The experimental procedure was as follows: after laser excitation ceased, the PTD generator triggered the CCD to monitor emission decay over a defined integration time. By rotating the optical axes of a quarter-wave plate and a linear polarizer to +45° and −45°, respectively, we obtained the decay spectra of left circularly polarized (IL) and right circularly polarized (IR) persistent luminescence. With this setup, we observed that the CPPL signals from the three different afterglow materials decayed within one minute after the 360 nm laser excitation source was turned off, as shown in Fig. S7.†46,47 To evaluate the effect of this decay on CPPL performance, we calculated the g-factor (gPL) at various stages of luminescence attenuation, using the formula gPL = 2 × (IL − IR)/(IL + IR). Notably, the gPL values fluctuated up and down within a certain range with time decay, but basically remained consistent. The average gPL values are around ±0.4, ±0.5, and ±0.6, aligning closely with the glum values from steady-state CPL measurements (Fig. S8–S10†). These results indicate that CPPL-based encryption retains its ability to differentiate information across the luminescence decay process.
Upconverted circularly polarized persistent luminescence of the chiral composite materials
Our goal is to develop multilevel CPPL information encryption materials by integrating upconversion luminescence technology. Therefore, we examined the time-resolved UC-CPPL spectra at three distinct afterglow wavelengths, adjusting the optical path settings of the detection system (Fig. S11†). Under optimized conditions, we observed distinct time-dependent UC-CPPL signals, attributed to the radiative energy transfer between UCNPs-Tm and BP, GP, and RP, respectively (Fig. S12†). The two-dimensional time-resolved UC-CPPL spectra revealed that when these afterglow materials were doped into R- or S-chiral liquid crystals, they produced opposite UC-CPPL signals at corresponding emission wavelengths, confirming the successful achievement of UC-CPPL in both left- and right-handed directions. Furthmore, after the 980 nm laser excitation was turned off, the UC-CPPL spectra of BP- and GP-doped materials decayed rapidly within 10 seconds, while RP-doped liquid crystal exhibited a more prolonged afterglow (Fig. 3d–f). The average gUC-PL values at different time decay showed that the gUC-PL factors for the three materials remained around ±0.4, ±0.5, and ±0.6 (Fig. 3g–i and Fig. S13–S15†).
Multilevel information encryption
To date, we have successfully fabricated UC-CPPL materials that display blue-violet UC-CPL under 980 nm NIR laser excitation and exhibit blue, green, or red UC-CPPL after the excitation is stopped, enabling multilevel information encryption. Based on this phenomenon, we designed a multilevel encryption system that combines different afterglow colors and circular polarization directions, as illustrated in Fig. 4a. For example, simple patterns such as “10” and “UC-CPPL” demonstrate two-level encryption by selectively adding BP to the UCNPs-doped liquid crystal. In the presence or absence of BP, the material emits blue-violet light under NIR excitation, forming the first level of information. After the excitation ceases, RP-containing samples display an orange afterglow, revealing patterns such as “0” and “CPPL”, constituting the second layer of information. Moreover, the UC-CPPL intensity exhibited siginificant variation when filtered through a left-handed (L-CPF) or right-handed circular polarization filter (R-CPF). This suggests that incorporating three distinct color N*LC materials further enriches and complicates the encrypted information. For instance, in a blue dot array illuminated by a 980 nm laser, turning off the light reveals a colorful house pattern with a red roof, green walls, and blue windows. When viewed through the R-CPF, the blue windows disappear, adding another encryption level. Hidden numbers such as “8” or “0” also demonstrate the system's enhanced encryption capabilities.
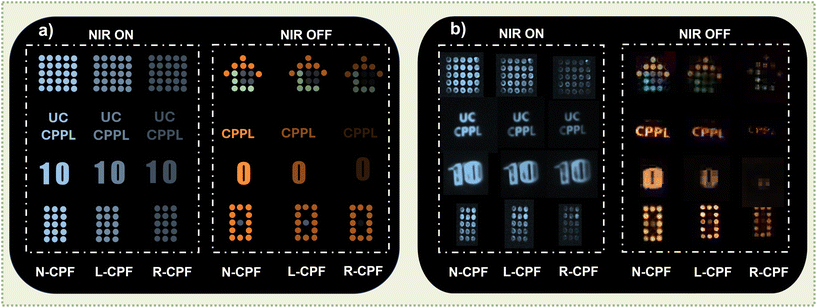 |
| Fig. 4 Encryption patterns of UCNPs/BP, UCNPs/GP and UCNPs/RP in N*LC doped by R811. The photos were taken with the L-CPF, R-CPF, and without the circular polarizer, respectively. N-CPF, no polarized filter; R-CPF, right-handed circularly polarized filter; L-CPF, left-handed circularly polarized filter. (a) Schematic diagram, (b) true graph. | |
We also demonstrated the robust information encryption potential of multicolor UC-CPPL materials by utilizing Morse code symbols as an encryption medium.48,49 As shown in Fig. 5, a custom-designed pattern containing encoded Morse code employed chiral N*LC doped with UCNPs@BP (blue afterglow), UCNPs@GP (green afterglow), and UCNPs@RP (red afterglow) to convey these message. Upon NIR laser excitation, a uniform blue UC-CPL pattern was visible. In the afterglow mode, through radiative energy transfer, the UC-CPPL produced bright multicolored patterns of red, green, and blue. Notably, the blue afterglow was weaker than the green and red emission. Viewed under the R-CPF, only red and green light points remained visible. We further designed a special 5 × 5 array pattern where the green and red afterglow emissions represented Morse code symbols “•” and “–”, respectively, with black spaces indicating interference signals or “blank”. By decoding each row using a Morse code table, the encoded message “NCNST” was accurately retrieved. This integration of multicolor afterglow chiral materials with Morse code significantly enhances the complexity of the encryption system, proposing a novel approach to information security.
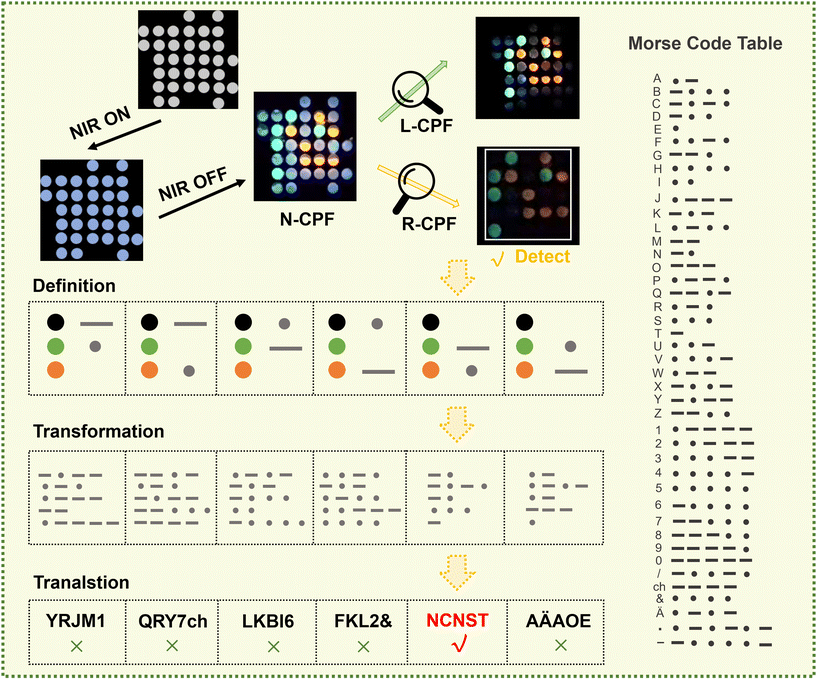 |
| Fig. 5 Information encryption using afterglow colors and circularly polarized information of UCNPs/BP, UCNPs/GP, and UCNPs/RP in N*LC doped with R811 excited by 980 nm laser. Green and red afterglow colors represent Morse code symbols “•” and “–”, respectively. Absence of light (black) denotes a “blank”. N-CPF, no polarized filter; R-CPF, right-handed circularly polarized filter; L-CPF, left-handed circularly polarized filter. | |
Conclusions
In conclusion, by incorporating an optimal amount of phosphors and UCNPs into N*LC, we demonstrated the first example of UC-CPPL based on the radiative energy transfer process in N*LC. Through precise tuning the dopant ratio, stable multicolor UC-CPPL was achieved, with a gUC-PL value of approximately ±0.6. The concept of UC-CPPL introduces multilevel information, enabling more advanced and secure encryption. The materials exhibiting UC-CPPL further enhance both the complexity and security of the encryption process, achieving true information concealment. This work not only broadens the application of UC-CPPL materials but also provides a new perspective for the advancement of multilevel optical information encryption technologies.
Author contributions
H. Mao completed the experiments and wrote the manuscript and ESI.† J. Zhou improves experimental details. X. Jin and P. Duan supervised the work and revised the manuscript. All authors discussed the results and have approved the final version of the manuscript.
Data availability
The data supporting this article have been included as part of the ESI.†
Conflicts of interest
There are no conflicts to declare.
Acknowledgements
This work was supported by the National Natural Science Foundation of China (22172041, 22405215, 22302046, 22205045, 52173159, 92256304); the National Key Basic R&D Re-search Program of Ministry of Science and Technology of the People's Republic of China (2021YFA1200303); the Beijing Municipal Science and Technology Commission (JQ21003); the Strategic Priority Research Program of Chinese Academy of Sciences (XDB36000000).
References
- J. Huang, X. Jin, X. Yang, T. Zhao, H. Xie and P. Duan, ACS Nano, 2024, 18, 15888–15897 CAS.
- S. Lai, Y. Jin, L. Shi, R. Zhou and Y. Li, ACS Appl. Mater. Interfaces, 2022, 14, 47113–47125 CAS.
- C. Li, X. Yang, J. Han, W. Sun and P. Duan, Mater. Adv., 2021, 2, 3851–3855 Search PubMed.
- S. Li, Y. Tang, Q. Fan, Z. Li, X. Zhang, J. Wang, J. Guo and Q. Li, Light: Sci. Appl., 2024, 13, 140 Search PubMed.
- Y. Sang, J. Han, T. Zhao, P. Duan and M. Liu, Adv. Mater., 2020, 32, 1900110 Search PubMed.
- X. Yang, X. Gao, Y.-X. Zheng, H. Kuang, C.-F. Chen, M. Liu, P. Duan and Z. Tang, CCS Chem., 2023, 5, 2760–2789 CAS.
- X. Yang, X. Jin, A. Zheng and P. Duan, ACS Nano, 2023, 17, 2661–2668 CAS.
- D.-W. Zhang, M. Li and C.-F. Chen, Chem. Soc. Rev., 2020, 49, 1331–1343 CAS.
- T. Zhao and P. Duan, Angew. Chem., Int. Ed., 2024, 63, e202406524 CrossRef CAS.
- Z.-L. Gong, X. Zhu, Z. Zhou, S.-W. Zhang, D. Yang, B. Zhao, Y.-P. Zhang, J. Deng, Y. Cheng, Y.-X. Zheng, S.-Q. Zang, H. Kuang, P. Duan, M. Yuan, C.-F. Chen, Y. S. Zhao, Y.-W. Zhong, B. Z. Tang and M. Liu, Sci. China: Chem., 2021, 64, 2060–2104 CrossRef CAS.
- C. Jiang, P. Pan, X. Jin and P. Duan, Chin. Sci. Bull., 2023, 68, 4302–4317 Search PubMed.
- X. Yang, X. Jin, T. Zhao and P. Duan, Mater. Chem. Front., 2021, 5, 4821–4832 RSC.
- Q. Yang, X. Liu, Z. Xu, X. Wu, G. Liang, M. Lin, Z. Shen and K. Sui, Adv. Compos. Hybrid Mater., 2024, 7, 121 CrossRef CAS.
- F. Zhang, Z. Shen, K. Sui and M. Liu, J. Colloid Interface Sci., 2024, 657, 853–857 CrossRef CAS.
- Z. An, C. Zheng, Y. Tao, R. Chen, H. Shi, T. Chen, Z. Wang, H. Li, R. Deng, X. Liu and W. Huang, Nat. Mater., 2015, 14, 685–690 CrossRef CAS PubMed.
- K. Gu, Z. Meng, X. W. Liu, Y. Wu, X. Qi, Y. Ren, Z.-Q. Yu and B. Z. Tang, Aggregate, 2023, 4, e337 CrossRef CAS.
- W. Jia, Q. Wang, H. Shi, Z. An and W. Huang, Chem. – Eur. J., 2020, 26, 4437–4448 CAS.
- K. Jinnai, R. Kabe, Z. Lin and C. Adachi, Nat. Mater., 2022, 21, 338–344 CrossRef CAS PubMed.
- R. Kabe and C. Adachi, Nature, 2017, 550, 384–387 Search PubMed.
- T. Maldiney, A. Bessière, J. Seguin, E. Teston, S. K. Sharma, B. Viana, A. J. J. Bos, P. Dorenbos, M. Bessodes, D. Gourier, D. Scherman and C. Richard, Nat. Mater., 2014, 13, 418–426 Search PubMed.
- Y. Zhu, Y. Guan, Y. Niu, P. Wang, R. Chen, Y. Wang, P. Wang and H.-L. Xie, Adv. Opt. Mater., 2021, 9, 2100782 CAS.
- M. Cao, Y. Ren, Y. Wu, J. Shen, S. Li, Z.-Q. Yu, S. Liu, J. Li, O. J. Rojas and Z. Chen, Nat. Commun., 2024, 15, 2375 CAS.
- R. Liu, B. Ding, D. Liu and X. Ma, Chem. Eng. J., 2021, 421, 129732 CrossRef CAS.
- F. Nie and D. Yan, Angew. Chem., Int. Ed., 2023, 62, e202302751 CrossRef CAS PubMed.
- D. Xu, C. Liu, H. Li, Y. Cheng and W.-H. Zheng, Adv. Opt. Mater., 2024, 12, 2303019 CrossRef CAS.
- J. You, C. Yin, S. Wang, X. Wang, K. Jin, Y. Wang, J. Wang, L. Liu, J. Zhang and J. Zhang, Nat. Commun., 2024, 15, 7149 CrossRef CAS PubMed.
- M.-Y. Zheng, Z.-B. Jin, Z.-Z. Ma, Z.-G. Gu and J. Zhang, Adv. Mater., 2024, 36, 2313749 CrossRef CAS.
- X. Wang, S. Ma, B. Zhao and J. Deng, Adv. Funct. Mater., 2023, 33, 2214364 CrossRef CAS.
- S. Zhao, G. Li, Q. Guo, Y. Wang, M. Zhang, Y. Zhou, S. Jin, M. Zhu and T. Zhuang, Adv. Opt. Mater., 2023, 11, 2202933 CrossRef CAS.
- H.-J. Feng, L. Zeng, J.-Y. Li, W.-Y. Lin, F. Qi, L.-H. Jiang, M.-Y. Zhang, Y. Zhao, L. Huang and D.-W. Pang, J. Am. Chem. Soc., 2024, 146, 21791–21805 CrossRef PubMed.
- H. Ji, B. Wu, E. Tian, C. Ren, X. Jin, L. Zhu and P. Duan, Sci. China: Chem., 2024, 67, 2571–2577 CrossRef CAS.
- G. Wang, X. Chen, Y. Zeng, X. Li, X. Wang and K. Zhang, J. Am. Chem. Soc., 2024, 146, 24871–24883 CrossRef CAS PubMed.
- L. Yan, J. Huang, Z. An, Q. Zhang and B. Zhou, Nat. Commun., 2024, 15, 1923 CAS.
- H. He, M. Cen, J. Wang, Y. Xu, J. Liu, W. Cai, D. Kong, K. Li, D. Luo, T. Cao and Y. J. Liu, ACS Appl. Mater. Interfaces, 2022, 14, 53981–53989 CrossRef CAS PubMed.
- C. Jiang, Y. Shi, X. Yang, T. Zhao, J. Zhou, X. Jin, Y. Zhang and P. Duan, Adv. Opt. Mater., 2023, 11, 2300001 CAS.
- X. Jin, Y. Sang, Y. Shi, Y. Li, X. Zhu, P. Duan and M. Liu, ACS Nano, 2019, 13, 2804–2811 CrossRef CAS PubMed.
- X. Yang, M. Zhou, Y. Wang and P. Duan, Adv. Mater., 2020, 32, 2000820 CAS.
- T. Zhao, J. Han, Y. Shi, J. Zhou and P. Duan, Adv. Mater., 2021, 33, 2101797 CrossRef CAS PubMed.
- L. Hu, Y. Fan, L. Liu, X. Li, B. Zhao, R. Wang, P. Wang, A. M. El-Toni and F. Zhang, Adv. Opt. Mater., 2017, 5, 1700680 CrossRef.
- J. Lin, C. Yang, P. Huang, S. Wang, M. Liu, N. Jiang and D. Chen, Chem. Eng. J., 2020, 395, 125214 CrossRef CAS.
- Q. Ma, J. Wang, Z. Li, D. Wang, X. Hu, Y. Xu and Q. Yuan, Inorg. Chem. Front., 2017, 4, 1166–1172 Search PubMed.
- X. Zhang, J. Zhang and Q. Zhu, Opt. Mater., 2022, 125, 112100 CrossRef CAS.
- Y. Chen, Y. Zhang, H. Li, Y. Li, W. Zheng, Y. Quan and Y. Cheng, Adv. Mater., 2022, 34, 2202309 CrossRef CAS.
- J. Liu, Z.-P. Song, J. Wei, J.-J. Wu, M.-Z. Wang, J.-G. Li, Y. Ma, B.-X. Li, Y.-Q. Lu and Q. Zhao, Adv. Mater., 2024, 36, 2306834 CrossRef CAS PubMed.
- X. Wang, B. Zhao and J. Deng, Adv. Mater., 2023, 35, 2304405 CrossRef CAS.
- T. Harada, H. Hayakawa, M. Watanabe and M. Takamoto, Rev. Sci. Instrum., 2016, 87, 075102 CrossRef PubMed.
- C. Zhang, D. Sun, R. McLaughlin, D. Semenov, S. McGill, Z.-G. Yu, E. Ehrenfreund and Z. V. Vardeny, Phys. Rev. B, 2018, 98, 155205 CrossRef CAS.
- X.-J. Liao, D. Pu, L. Yuan, J. Tong, S. Xing, Z.-L. Tu, J.-L. Zuo, W.-H. Zheng and Y.-X. Zheng, Angew. Chem., Int. Ed., 2023, 62, e202217045 CrossRef CAS PubMed.
- Y. Ling, J. Liu, Y. Dong, Y. Chen, J. Chen, X. Yu, B. Liang, X. Zhang, W. An, D. Wang, S. Feng and W. Huang, Adv. Mater., 2023, 35, 2303641 CrossRef CAS.
|
This journal is © The Royal Society of Chemistry 2025 |
Click here to see how this site uses Cookies. View our privacy policy here.