High stability of robust anti-thermal-quenching lead-free double perovskite crystals for optoelectronic devices and high-performance fibers†
Received
2nd September 2024
, Accepted 28th October 2024
First published on 29th October 2024
Abstract
Lead-free double perovskites are environmentally friendly, and their good photoelectric efficiency has received widespread attention. However, the stability and efficiency of lead-free perovskites need to be further improved to meet the growing application needs. In this study, thermally quenched perovskite (Cs2NaHoCl6) crystals (PCs) were successfully synthesized by co-precipitation. By doping with Sb3+, the excitation wavelength of the original perovskite increased significantly to 250–360 nm, and the emission wavelength of the original perovskite also increased significantly to 660 nm. Cs2NaHoCl6:Sb3+ PCs are made of red and white light-emitting diodes (LEDs) for general lighting applications. In addition, Cs2NaHoCl6:Sb3+ PCs are made into flexible luminescent fibers with aramid/polyphenylene sulfide (ACFs/PPS) composite fibers. Based on the good thermal quenching resistance of Cs2NaHoCl6:Sb3+ PCs, the flexible luminescent fibers showed excellent high-temperature luminescence stability. At 125 °C, flexible luminescent fibers retained 99.8% of the original luminescence intensity; at 250 °C, they retained 75.6% of the original. These flexible luminescent fibers have the potential to be used in fluorescence detection in high-temperature environments. In summary, this study used a simple method to prepare lead-free perovskites with good optical properties and stability, expanding the application of perovskites in the field of fibers.
Introduction
Halide perovskites have attracted widespread attention due to their excellent optoelectronic properties.1–4 This has led to various LED and solar cell applications.5–8 However, toxicity and instability limit their broad commercialization.9–11 The molecular formula of a lead-free double perovskite (DP) is A2B+B3+X6 (A = Cs+, MA+, FA+; B+ = Ag+, K+, Na+, Li+; B3+ = Bi3+, Sb3+, In3+; X = Cl−, Br−, I−). This non-toxic and stable material has the characteristics of a flexible structure.12–14 However, low photoluminescence quantum yields (PLQYs) and differential spectral tunability remain challenges faced by the widespread application of primitive lead-free double perovskite materials, mainly due to their indirect bandgap or dual prohibition transitions in direct bandgap systems.15–17 Metallic element hybridization is a strategy for regulating the optical properties of perovskites, which have wide applications in anti-counterfeiting, biomedicine, and LEDs.18–23 Cs2NaInCl6 has a low PLQY and a faint blue glow due to the conversion restriction imposed by parity prohibition. Through Sb3+ doping, the partial prohibition of Cs2NaInCl6 PCs was broken, and the PLQY increased.15,24,25 Cs2NaHoCl6 is a lead-free double perovskite with resistance to thermal quenching, and emits red light when excited by blue light. By doping with Sb3+, the excitation of Cs2NaHoCl6 under ultraviolet (UV) light is significantly enhanced, and the red-light emission of Cs2NaHoCl6 is improved dramatically. This paper reports that the synthesis method is more energy-efficient and more conducive to large-scale industrial production in the later stage compared with previous reports.25
At the same time, perovskite-based LEDs are widely used in the lighting field because of their high chromaticity, wide color gamut, and low cost.26–29 Cs2NaHo1−xCl6:xSb3+ PCs have extremely high color purity, so we made them red and white LEDs. Perovskite is compounded with fibers to obtain flexible luminescent fibers, which have been widely reported in recent years.30,31 ACF/PPS composite fibers strongly resist high temperatures and corrosion and are used for industrial exhaust gas filtration.32,33 Cs2NaHo1−xCl6:xSb3+ PCs are modified ACFs/PPS composite fibers to obtain flexible luminescent fibers. These flexible luminescent fibers exhibit good luminescence stability in a variety of environments. Based on the good thermal quenching resistance of Cs2NaHo1−xCl6:xSb3+ PCs and the good high-temperature resistance of the ACFs/PPS composite fiber, the flexible luminescence fibers have surprising luminescence stability and structural stability at high temperatures. These fluorescent fiber paper can be used in fluorescent anti-counterfeiting and intelligent wearable devices in different environments. This work expands the application of lead-free perovskites in optoelectronics and flexible and functional fibers.
Results and discussion
In this research, the Cs2NaHoCl6:Sb3+ PCs were successfully synthesized using an energy-saving co-precipitation method (the detailed process is shown in the Experimental section). After the reaction, the perovskite crystal solution is centrifuged and dried to obtain perovskite crystals.
The prepared perovskite crystals are pale yellow powder, showing noticeable red luminescence under a 254 nm ultraviolet lamp. The Cs2NaHoCl6:Sb3+ PCs were combined with UV chips to obtain red and white LEDs. In addition, the samples were modified with the ACFs/PPS composite fibers prepared by the hot-pressing process to obtain flexible, functional luminescent composite fibers (Fig. 1). The Cs2NaHoCl6 PCs have a cubic crystal structure of the Fm
m space group, consisting of [NaCl6]5− and [HoCl6]3−, connected by horns. Cs+ enters the octahedral cavity (Fig. 2a). In doping Sb3+, Sb3+ usually replaces Ho3+ to form a [SbCl6]3−octahedron to maintain the charge balance. To verify the phase purity of Cs2NaHCl6:Sb3+ PCs, X-ray diffraction (XRD) tests were conducted on Cs2NaHCl6:Sb3+ PCs. Fig. 2b shows that the XRD patterns of Cs2NaHoCl6:Sb3+ PCs at different doping contents are consistent with the simulated results of pure Cs2NaHoCl6:Sb3+ PCs. Due to the low doping content of Sb3+, the diffraction peak of Cs2NaHoCl6:Sb3+ PCs is not significantly shifted (Fig. 2b). The above results show that the synthesized Cs2NaHoCl6:Sb3+ PCs have excellent crystallinity and high phase purity, and they also prove the reliability of the efficient co-precipitation method. Fig. 3a illustrates the morphology of Cs2NaHo1−xCl6:xSb3+ PCs by scanning electron microscopy (SEM). The SEM image shows that the crystals of Cs2NaHo1−xCl6:xSb3+ have a diameter of 5–10 μm and exhibit an octahedral morphology (Fig. 3a). Although partially agglomerating, some tiny crystals at 2–5 μm remain in octahedral morphology (Fig. S1, ESI†).
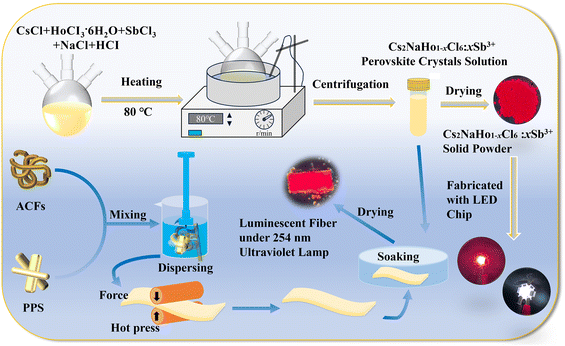 |
| Fig. 1 The schematic diagrams of the preparation process of Cs2NaHo1−xCl6:xSb3+ PCs, ACFs/PPS flexible luminescent fiber paper, and LEDs. | |
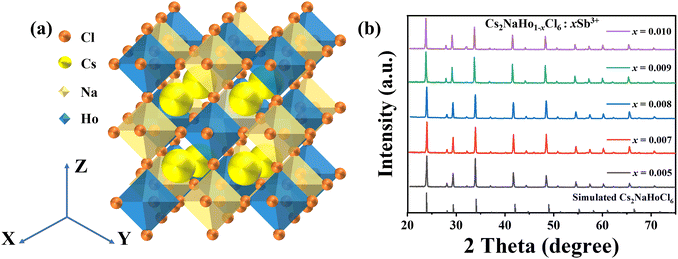 |
| Fig. 2 (a) Crystal structure of Cs2NaHoCl6. (b) XRD patterns of Cs2NaHo1−xCl6:xSb3+ (x = 0.005, 0.007, 0.008, 0.009, and 0.010). | |
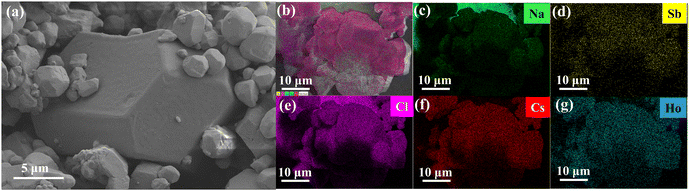 |
| Fig. 3 (a) SEM image of Cs2NaHo0.992Cl6:0.008Sb3+ PCs; (b) SEM image of Cs2NaHo0.992Cl6:0.008Sb3+ PCs. Elemental mapping images of Na (c), Sb (d), Cl (e), Cs (f), and Ho (g) in Cs2NaHo0.992Cl6:0.008Sb3+ PCs. | |
Cs, Sb and other elements are uniformly distributed in Cs2NaHo0.992Cl6:0.008Sb3+ PCs (Fig. 3b-g). EDS analysis supports the successful doping of Sb3+ into PCs. Moreover, various elements are evenly distributed inside the crystal. The Cs, Na, Ho, and Cl atomic ratios in Cs2NaHoCl6 were close to 2
:
1
:
1
:
6 (shown in Table S1, ESI†). These Cs2NaHo0.992Cl6:0.008Sb3+ PCs with good morphology further demonstrate the reliability of the synthesis method. The chemical composition and electronic properties of Cs2NaHo1−xCl6:xSb3+ are investigated using X-ray photoelectron spectroscopy (XPS) (Fig. 4a). Comparison of XPS images of Cs2NaHoCl6 and Cs2NaHo0.992Cl6:0.008Sb3+ shows that Cs 3d, Na 1s, Cl 2p, and Ho 4d orbital signals are present in both crystals (Fig. 4c–f). Furthermore, the signal of the Sb orbital was detected in Cs2NaHo1−xCl6:xSb3+ PCs, while the Sb signal was not observed in the unmixed Cs2NaHoCl6 PCs (Fig. 4b). This provides evidence for the successful doping of Sb with Cs2NaHoCl6. In addition, the Sb and Ho were shown to be with +3 valence, with peaks at 539.7 eV and 530.4 eV attributed to 3d3/2 and 3d5/2 orbital Sb3+, while peaks at 161.4 eV were assigned to the 4d5/2 orbital Ho3+ (Fig. 4b and f).34,35
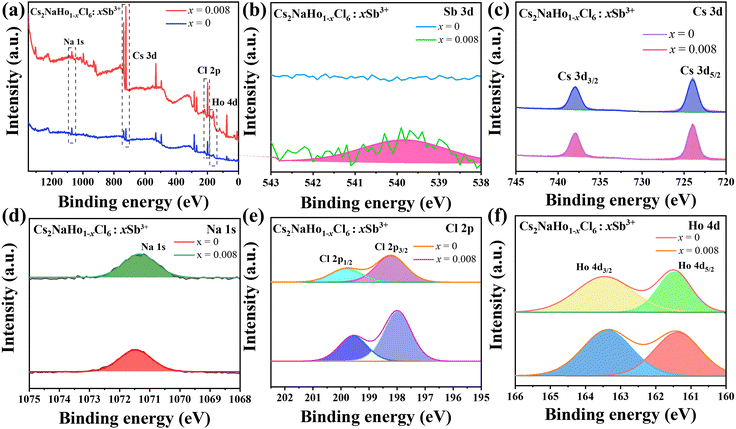 |
| Fig. 4 (a) XPS spectra of Cs2NaHo1−xCl6:xSb3+ PCs (x = 0 and 0.008). High resolution XPS spectra of Sb 3d (b), Cs 3d (c), Na 1s (d), Cl 2p (e) and Ho 4d (f) in Cs2NaHo1−xCl6:xSb3+ PCs (x = 0 and 0.008). | |
Subsequently, the optical properties of Cs2NaHo1−xCl6:xSb3+ PCs at different Sb-doped concentrations were tested. The PLE spectra of Cs2NaHoCl6 PCs at 660 nm show excitation peaks at 254 nm, 365 nm, and 450 nm (Fig. S2a, ESI†). Under 450 nm blue light excitation, there is a broad emission peak at 635 nm to 680 nm, and its maximum emission wavelength is 660 nm, which is attributed to the 5F5 → 5I8 transition of Ho3+ (Fig. S2b, ESI†). The strongest of these excitation wavelengths is 450 nm. With the mixing of Sb3+, the PL spectrum of Cs2NaHoCl6:Sb3+ PCs did not show new emission peaks compared with the PL spectrum of Cs2NaHoCl6 PCs. This suggests that the Sb3+ ions did not become the center of emission. With the mixing of Sb3+, a new excitation peak at 334 nm appeared in the PLE spectrum of Cs2NaHoCl6:Sb3+ PCs at 250–360 nm compared with the PLE spectrum of Cs2NaHoCl6 PCs, which was due to the 1S0 → 3P1 electron transition of Sb3+ in the band edges. With the increase of the concentration of Sb3+, the excitation peaks of Cs2NaHoCl6:Sb3+ PCs at 265 nm and 334 nm were significantly enhanced and even exceeded the excitation peak of Cs2NaHoCl6 PCs at 445 nm (Fig. 5b). This is attributed reason which in turn significantly improves the luminescence performance of Cs2NaHoCl6:Sb3+ PCs. The luminous intensity increased gradually with the increase of the concentration of Sb3+. When the doping concentration exceeds 0.008, the intensity of the emission peak of Cs2NaHo1−xCl6:xSb3+ PCs decreases due to the quenching effect of fluorescence concentration (Fig. 5b).25 Fig. S3 (ESI†) shows the fluorescence of Cs2NaHo1−xCl6:xSb3+ PCs at 254 nm and 365 nm UV lamps, consistent with that shown in the PL spectrum. In addition, the PL spectra of Cs2NaHo1−xCl6:xSb3+ PCs under 320 nm and 450 nm UV excitation were also tested (Fig. S4, ESI†). They exhibit emission peaks consistent with those of 250 nm UV excitation. Fig. 5c and d show the PL and PLE spectra of Cs2NaHo0.992Cl6:0.008Sb3+ PCs at different excitation and detection wavelengths. It can be observed that the PL and PLE spectra of Cs2NaHo1−xCl6:xSb3+ PCs do not change regardless of the change in excitation and emission wavelengths, thus proving that the luminescence properties of Cs2NaHo1−xCl6:xSb3+ PCs are not due to lattice defects or surface defects.22 The two-dimensional (2D) and three-dimensional (3D) wavelength scans of Cs2NaHo1−xCl6:xSb3+ PCs also confirm the luminescence properties of Cs2NaHo1−xCl6:xSb3+ PCs (Fig. 5e and f).
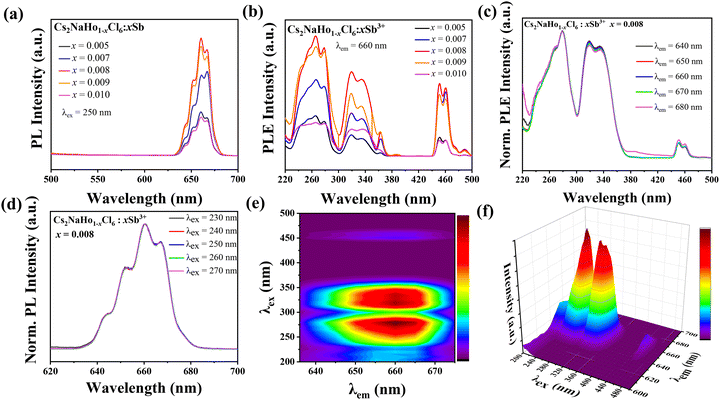 |
| Fig. 5 (a) PL and (b) PLE spectra of Cs2NaHo1−xCl6:xSb3+ (x = 0.005, 0.007, 0.008, 0.009, and 0.010). (c) Normalized PLE spectra of Cs2NaHo0.992Cl6:0.008Sb3+ PCs at different monitoring wavelengths. (d) Normalized PL spectra of Cs2NaHo0.992Cl6:0.008Sb3+ PCs at different excitation wavelengths. (e) The 2D wavelength scanning spectra. (f) 3D wavelength scanning spectra. | |
To further explore the luminescence properties of Cs2NaHo1−xCl6:xSb3+ PCs, the time-resolved PL (TRPL) spectra and temperature-dependent PL were measured. The TRPL decay curve of Cs2NaHo1−xCl6:xSb3+ PCs can be well-fitted to a triple exponential equation:10
| I(t) = A1 exp(−t/τ1) + A2 exp(−t/τ2) + A3 exp(−t/τ3) | (1) |
The average life expectancy of Cs2NaHo1−xCl6:xSb3+PCs can be calculated using the following equation:36
| τavg = (A1τ12 + A2τ22 + A3τ32)/(A1τ1 + A2τ2 + A3τ3) | (2) |
where
A1,
A2 and
A3 are the amplitudes and
τ1,
τ2 and
τ3 represent the fast and slow decay lifetimes, respectively. It can be calculated that the average fluorescence lifetimes of Cs
2NaHo
1−xCl
6:
xSb
3+ PCs are 1.67 ms (
x = 0.005), 2.44 ms (
x = 0.007), 2.49 ms (
x = 0.008), 1.82 ms (
x = 0.009) and 1.76 ms (
x = 0.01) (
Fig. 6a). The variation in the average fluorescence lifetime was the same as in PL intensity, and the average fluorescence lifetime was the longest when the Sb
3+ content was 0.008 (
Fig. 6a). The average fluorescence lifetime of Cs
2NaHo
0.992Cl
6:0.008Sb
3+ PCs was 2.49 ms. These proved that the hybridization of Sb
3+ would improve the fluorescence performance of Cs
2NaHoCl
6 PCs. The PLQY is 5.1%. According to previous literature, the specific luminescence mechanism of Cs
2NaHo
1−xCl
6:
xSb
3+ PCs has been reported. The valence band of Cs
2NaHo
1−xCl
6:
xSb
3+ PCs is dominated by the Cl 3p orbital, and the Ho 5d orbital forms the bottom conduction band. Sb
3+ was doped at the Ho
3+ site, the Sb 5s state was introduced above the host valence band maximum (VBM), and the Sb 5p state was introduced below the host conduction band minimum (CBM), indicating that the 5s–5p optical transition of Sb
3+ occurred in the Cs
2NaHoCl
6 main crystal. The incorporation of Sb
3+ reduces the band gap of the host and increases the mechanism of the absorption channel. The f–f electron transition probability of Ho
3+ increases accordingly.
25 The mechanism is shown in
Fig. 6b.
Fig. 6c shows the temperature-dependent PL spectra of Cs
2NaHo
0.992Cl
6:0.008Sb
3+ PCs. Cs
2NaHo
0.992Cl
6:0.008Sb
3+ PCs prepared by the coprecipitation method also showed excellent thermal quenching properties, consistent with previous research results.
25
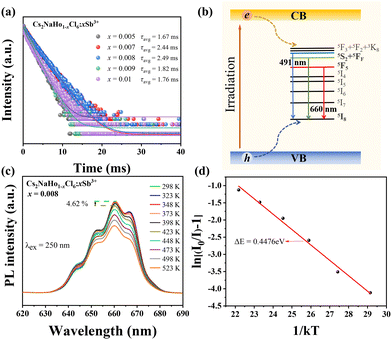 |
| Fig. 6 (a) TRPL attenuation curve of Cs2NaHo1−xCl6:xSb3+. (b) The schematic diagram of PL enhancement. (c) Temperature-dependent PL of Cs2NaHo0.992Cl6:0.008Sb3+ PCs. (d) Cs2NaHo0.992Cl6:0.008Sb3+ variable temperature activation energy during the high-temperature test. | |
The ambient temperature increased from 298.5 K to 423.5 K, and the PL strength of Cs2NaHo0.992Cl6:0.008Sb3+ PCs decreased by 4.62% (Fig. 6c). This phenomenon occurs because the luminescence of Ho3+ is attributed to f–f transitions, where 4f electrons exist in the inner shell. Therefore, these inner shell electrons are relatively less susceptible to external environmental factors.25 In addition, as the temperature increases, the matrix′s lattice inevitably expands, leading to a decrease in local concentration around Ho3+. Therefore, the concentration quenching effect weakens. The full width at half maximum (FWHM) increases due to the interaction between excitons and phonons.22 To prove their fluorescence thermal stability, the variable temperature activation energy of Cs2NaHo1−xCl6:xSb3+ PCs was calculated using the following equation:37
| 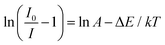 | (3) |
In eqn (3), I is the fluorescence intensity of the optimal emission wavelength, I0 is the fluorescence intensity at the initial temperature, A is the constant, ΔE is the variable temperature activation energy, k is the Boltzmann constant (8.629 × 10−5 eV·K−1), and T is the thermodynamic temperature. After linear fitting, the ΔE was 0.4476 eV, indicating that the variable temperature activation energy that led to the fluorescence quenching of Cs2NaHo0.992Cl6:0.008Sb3+ PCs was 0.4476 eV (Fig. 6d). The above results show that Cs2NaHo0.992Cl6:0.008Sb3+ PCs have outstanding thermal stability. Based on the PL spectra of Cs2NaHo1−xCl6:xSb3+ PCs, the Commission Internationale de L'Eclairage (CIE) coordinates are calculated. The color purity formula for Cs2NaHo1−xCl6:xSb3+ PCs can be calculated from the following equation:31
| 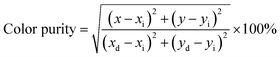 | (4) |
where (
x, y), (
xi, yi), and (
xd,
yd) correspond to the CIE color coordinates of the PCs, white illumination, and dominant wavelength, respectively. Cs
2NaHo
0.992Cl
6:0.008Sb
3+ has the highest color purity value of 99.5% (Fig. S5a, ESI
†). Such high color purity indicates that it can be used in LED lighting applications. To further study the application ability of Cs
2NaHo
1−xCl
6:
xSb
3+ PCs in the field of LEDs, Cs
2NaHo
0.992Cl
6:0.008Sb
3+ PCs with the UV chips are made into a red LED and subjected to the electroluminescence (EL) test. The driving current is 150 mA, and the corresponding output power is 1 W. The EL spectrum of red-LED shows an emission peak at 660 nm, the same as the peak position of the PL spectrum of the original perovskite. The red LED produces CIE color coordinates of (0.72, 0.27) with a correlated color temperature (CCT) of 704 K (
Fig. 7a and b). The red LEDs also have a very high color purity; the value is 99.5%. Cs
2NaHo
0.992Cl
6:0.008Sb
3+ PCs are mixed with red and green commercial phosphors to make white LEDs to further expand their application in the lighting sector. The driving current is 150 mA, and the corresponding output power is 1 W. The CIE color coordinates of this white LED are (0.29, 0.35) with a correlated color temperature (CCT) of 7506 K (
Fig. 7c and Fig. S5b, ESI
†). These results demonstrate their potential in the field of lighting.
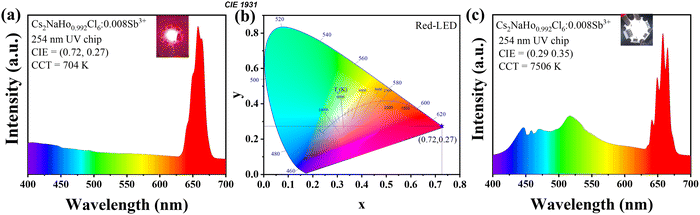 |
| Fig. 7 (a) The EL spectrum of the LED fabricated by combining the 254 nm d-UV chip and Cs2NaHo1−xCl6:xSb3+ PCs (x = 0.008). (b) CIE color coordinates of the LED fabricated by combining the 254 nm UV chip and Cs2NaHo1−xCl6:xSb3+ PCs (x = 0.008). (c) The EL spectrum of the white LED. | |
Meanwhile, we also modified ACFs/PPS composite fibers with Cs2NaHo1−xCl6:xSb3+ PCs to make flexible luminescent fibers. The fibers were prepared using the wet synthesis method we reported previously. The original ACFs/PPS composite fibers were not fluorescent under the UV lamp. The ACFs/PPS fibers modified by Cs2NaHo1−xCl6:xSb3+ PCs emit red light under the 254 nm ultraviolet lamp (Fig. S6, ESI†). The PL spectra of modified ACFs/PPS composite fibers and unmodified also show that the modified ACFs/PPS composite fibers have an emission peak at 660 nm (Fig. S6, ESI†). The luminous intensity of the original perovskite differs by 9.8% compared with the modified luminescent fibers, and no significant intensity decrease occurred. (Fig. 8a). The fibers will block the excitation and emission of light to a small extent, reducing luminous intensity. Fig. 8b exhibits that the fluorescence intensity of the flexible luminescent fibers remains at 97.5% of its pristine luminescence intensity after being folded 100 times. This indicates that the perovskite is very tightly bound to the fiber. The luminous intensity of the modified luminescent fiber in a 98% humidity environment for 20 minutes is 17.9% less than that of the original perovskite powder (Fig. S7, ESI†). Additionally, the luminescent fibers can still maintain uniform luminescence in humid environments, which proves that perovskite fiber composites can effectively improve the stability of perovskites in damp environments. To explore the luminescence stability of the ACFs/PPS composite luminescent fibers in different environments, the luminescence intensity of the ACFs/PPS composite luminescent fibers was tested in high and low temperature and acidic liquid environments. Based on the excellent heat quenching resistance of Cs2NaHo1−xCl6:xSb3+ PCs, the ACFs/PPS composite luminescent fibers can maintain good luminescence stability at high temperatures. When the ambient temperature increases from 25 °C to 125 °C, the luminous intensity of ACFs/PPS composite luminescent fibers decreases only by 0.2%. When the ambient temperature increases from 25 °C to 250 °C, the luminous intensity of ACFs/PPS composite luminescent fibers decreases by 24.4% (Fig. 8c and Fig. S8a, ESI†). Meanwhile, the ACFs/PPS composite luminescent fiber was also placed at a high temperature of 220 °C for 4 h, and the luminous intensity only decreased by 5% (Fig. 8b and Fig. S8b). ACF/PPS composite luminescent fibers exhibit beneficial luminescence properties in low-temperature environments.
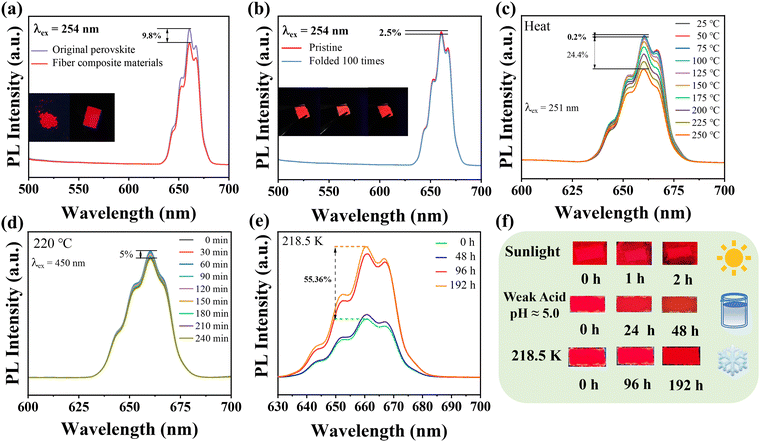 |
| Fig. 8 (a) PL spectra of primitive perovskites and modified composite fiber. (b) PL spectra before and after 100 folds of ACFs/PPS flexible luminescent fibers. (c) Temperature-dependent PL spectra of ACFs/PPS flexible luminescent fibers modified with Cs2NaHo0.992Cl6:0.008Sb3+ PCs. (d) PL spectra of ACFs/PPS composite luminescent fiber in the 220 °C environment. (e) The low temperature (−55 °C) PL spectra of the ACFs/PPS composite luminescent fibers. (f) Luminescence of ACFs/PPS composite luminescent fibers in sunny, weakly acidic, and low-temperature environments. | |
The luminous intensity of ACFs/PPS composite luminescent fibers was observed in an environment of −55 °C for 144 h, and it gradually increased to 55.36% higher than when it was not frozen (Fig. 8e). This is due to the low-temperature inhibition of non-radiative recombination, which substantially increases the fluorescence intensity of composite fluorescent fiber paper at low temperatures.22Fig. 8f shows that the modified ACFs/PPS composite luminescent fiber paper still maintains fluorescence after 2 h of sunlight. However, in weakly acidic (pH ≈ 5) environments, the luminescence of the modified ACFs/PPS compound fibers paper gradually weakens. This is due to the presence of a large amount of water in a weak acid environment, and the Ho3+ in Cs2NaHo1−xCl6:xSb3+ PCs are easy to combine with water molecules to form crystalline hydrates, resulting in the rupture of the crystal lattice, which causes the decrease of luminous intensity. These results prove that ACFs/PPS composite luminous fibers can maintain good luminescence performance in different use environments. ACFs/PPS composite luminous fibers show strong luminescence stability in high-temperature environments and can be used in the field of flexible functional luminescent fibers.
Experimental
Materials
Cesium chloride (CsCl, 99.99%), holmium chloride hexahydrate (HoCl3·6H2O, 99.5%), antimony chloride hexahydrate (SbCl3·6H2O, 99.99%), sodium chloride (NaCl, 99.99%), and absolute ethanol (AR) were purchased from Aladdin. Hydrochloric acid (HCl, Sinopharm 37 wt%), polyphenylene sulfide (PPS, Deyang Chemical Co., Ltd), aramid chopped fibers (ACFs, China BlueStar Chengrand Research Institute of Chemical Industry), polyethylene oxide (PEO Sumitomo Keiretsu Co., Ltd), anionic polyacrylamide (APAM China Pharmaceutical Group Chemical Reagent Co., Ltd), and sodium dodecylbenzene sulfonate (SDS China Pharmaceutical Group Chemical Reagent Co., Ltd) were also employed. No additional decontamination of materials and chemicals is carried out prior to use.
Synthesis of Cs2NaHo1−xCl6:xSb3+ perovskite crystals
The Cs2NaHo1−xCl6:xSb3+ perovskite crystals were synthesized by a co-precipitation method following the established procedure. Briefly, a mixture containing 2 mmol CsCl, 1 mmol NaCl, 0.008 mmol SbCl3·6H2O, and 1 mmol HoCl3·6H2O was prepared in 10 mL HCl in a 50 mL three necked flask. The mixture was heated at 80 °C for 1 h in the oil bath and then naturally cooled to room temperature. The original solution was purified and separated by centrifugation (5 min at 6000 rpm). After that, the supernatant was discarded, and the particles were dried in a vacuum furnace at 60 °C for 4 h.
Synthesis of ACFs/PPS compound paper
First, ACFs were washed with acetone, and PPS nonwoven microfiber was employed to remove extra contaminants. PPS microfiber pulp, produced by a valley pulping machine to create PPS nonwoven fabric, was then beaten. To prepare an even dispersion solution (APAMS/SDS/PEO), deionized water was mixed with APAM, SDS, and PEO in a mass ratio of 1
:
3
:
6. The combination was agitated at room temperature for a thorough 4 h. Subsequently, the PPS microfiber slurry and ACFs were dispersed using a fiber dissociator at a mass ratio of 3
:
7, generating a uniformly dispersed mixed fiber suspension. The final step involved wet papermaking, directly producing ACFs/PPS mixed paper on a paper machine.
Fabrication of LEDs from Cs2NaHo1−xCl6:xSb3+ PCs
Gallium nitride light-emitting diode chips without phosphor coating were prepared using Sb3+-doped Cs2NaHoCl6 crystal powder, which was ground, dissolved in ethanol, and epoxy resin gum. The fluorescent layer was repeatedly applied and dried to form a colloidal solution before being assembled into LEDs.
Test of the electroluminescence spectrum
The electroluminescence (EL) testing of the LED was carried out using a fluorescence spectrophotometer (HITACHI, F-4700). The specific operation steps of the LED performance test were as follows: as an external power supply with a voltage of 5.5 V and a current of 150 mA, the adjustable direct current (DC) power supply (MAISHENG, MS-155D) was connected to the two ends of the LED. Then the LED was securely affixed to the fluorescence indexer receiver port. Finally, after the power was turned on, the electroluminescence properties of the LED could be determined.
Measurements and characterization
An X-ray diffractometer (XRD, PANalytical B.V., Empyrean) was used to acquire X-ray diffraction patterns for determining the crystal structure of samples. The elemental composition and chemical state of samples were identified by X-ray photoelectron spectroscopy (XPS, Thermo Scientific, K-Alpha). The scanning electron microscopy (SEM) observations with energy-dispersive X-ray spectroscopy (EDS) were performed with a Gemini SEM 300, ZEISS, Germany. The photoluminescence (PL), photoluminescence excitation (PLE) spectra, electroluminescence (EL), and temperature-dependent PL spectra were measured with a fluorescence spectrophotometer (HITACHI, F-4700). The photoluminescence quantum yield (PLQY) and PL decay were measured with fluorescence spectrometers (Edinburgh, FLS1000).
Conclusions
In this study, Cs2NaHo1−xCl6:xSb3+ PCs were prepared by energy-efficient co-precipitation. The original Cs2NaHoCl6 PC had an emission peak at 660 nm and was red when excited by an ultraviolet lamp. After doping Sb3+, a new emission peak was added at 320 nm, and the emission peak intensity at 254 nm was significantly increased. This results in a significant increase in the red luminescence of Cs2NaHo1−xCl6:xSb3+ PCs at 660 nm. The down-conversion luminescence presents anti-thermal quenching PL properties through the temperature-dependent spectra analysis because of the reduced concentration quenching effect. Cs2NaHo1−xCl6:xSb3+ PCs were prepared using UV 254 nm LED chips to prepare red and white LEDs. At the same time, flexible luminescent fibers were prepared from Cs2NaHo1−xCl6:xSb3+ PCs and ACFs/PPS composite fibers. These fibers exhibit excellent luminescence stability at high temperatures. They also exhibit good luminescence stability in some other environments. In the future, fluorescent fiber paper can be used in fluorescent anti-counterfeiting and intelligent wearable devices. Functional fabrics made of luminescent crystals and fibers can be used in applications such as high-performance textile testing. When an area of a textile is damaged, and a specific laser is used to irradiate the textile, the detector cannot detect the fluorescence. The modification methods and applications provide ideas for expanding the research and application scope of perovskite and ACFs/PPS fibers.
Author contributions
The idea and concept of the current paper were created by Xiaoman Zhang, Yehua Zheng, Kun Nie, and Xiaoxue Ma. They also worked on the study design strategy and chose the discussion subjects. The primary experiments and paper writing were completed by Xiaoman Zhang. Yehua Zheng, Kun Nie, and Xiaoxue Ma provided advice and supervision while editing and revising the final manuscript. After conducting literature searches, Ziyao Hu, Ming Yang and Xiuqiang Duan extracted the data from the full-text papers that met the eligibility criteria. Tests and advice for experiments were given by Xiaodong Zhang and Mingquan Li. Lefu Mei, Luoxin Wang, and Hua Wang oversaw the investigation. All the authors discussed the results and commented on the manuscript.
Data availability
The data supporting this article have been included as part of the main text and ESI.†
Conflicts of interest
There are no conflicts to declare.
Acknowledgements
This work was financially supported by the National Natural Science Foundation of China (52274273), the Hubei Province Integrative Technology and Innovation Center for Advanced Fiberous Materials (XC202415), and the Open Project Program of High-Tech Organic Fibers Key Laboratory of Sichuan Province (PLN2023-05). We sincerely thank Liu Ying from the Analytical and Testing Center of Wuhan Textile University for providing technical support. The authors would like to thank Liu Nian and Liu Tianying from Shiyanjia Lab (www.shiyanjia.com) for the XPS and SEM characterization.
References
- L. Protesescu, S. Yakunin, M. I. Bodnarchuk, F. Krieg, R. Caputo, C. Hendon, R. Yang, A. Walsh and M. Kovalenko, Nanocrystals of cesium lead halide perovskites (CsPbX3, X = Cl, Br, and I): novel optoelectronic materials showing bright emission with wide color gamut, Nano Lett., 2015, 15, 3692–3696 CrossRef CAS
.
- G. Nedelcu, L. Protesescu, S. Yakunin, M. I. Bodnarchuk, M. J. Grotevent and M. Kovalenko, Fast anion-exchange in highly luminescent nanocrystals of cesium lead halide perovskites (CsPbX3, X = Cl, Br, I), Nano Lett., 2015, 15, 5635–5640 CrossRef CAS
.
- J. Song, J. Li, X. Li, L. Xu, Y. Dong and H. Zeng, Quantum dot light-emitting diodes based on inorganic perovskite cesium lead halides (CsPbX3), Adv. Mater., 2015, 27, 7162–7167 CrossRef CAS
.
- Y. Han, S. Yue and B. Cui, Low-dimensional metal halide perovskite crystal materials: structure strategies and luminescence applications, Adv. Sci., 2021, 8, 2004805 CrossRef CAS
.
- J. S. Kim, J. M. Heo, G. S. Park, S. J. Woo, C. Cho, H. J. Yun, D. H. Kim, J. Park, S. C. Lee, S. Park, E. Yoon, N. C. Greenham and T. W. Lee, Ultra-bright, efficient and stable perovskite light-emitting diodes, Nature, 2022, 611, 688–694 CrossRef CAS PubMed
.
- Y. H. Song, J. S. Yoo, E. K. Ji, C. W. Lee, G. S. Han, H. S. Jung and D. Yoon, Design of water stable green-emitting CH3NH3PbBr3 perovskite luminescence materials with encapsulation for applications in optoelectronic device, Chem. Eng. J., 2016, 306, 791–795 CrossRef CAS
.
- Z. L. Yan, J. S. Benas, C. C. Chueh, W. C. Chen, F. C. Liang, Z. Zhang, B. Lin, C. Su, T. Chiba, J. Kido and C. Kuo, Stable blue perovskite light-emitting diodes achieved by optimization of crystal dimension through zinc bromide addition, Chem. Eng. J., 2021, 414, 128774 CrossRef CAS
.
- R. Ollearo, A. Caiazzo, J. Y. Li, M. Fattori, A. J. Breemen, M. M. Wienk, G. H. Gelinck and R. A. Janssen, Multidimensional perovskites for high detectivity photodiodes, Adv. Mater., 2022, 34, 2205261 CrossRef CAS
.
- A. Babayigit, A. Ethirajan, M. Muller and B. Conings, Toxicity of organometal halide perovskite solar cells, Nat. Mater., 2016, 15, 247–251 CrossRef CAS
.
- Z. Hu, X. Wang, K. Nie, R. Zhou, W. Dai, X. Duan, X. Zhang, H. Wang, L. Wang, L. Mei, Y. Liu and X. Ma, High stability and spectral tunability of versatile manganese/europium/tellurium-doped double perovskite crystals toward flexible functional fabric and semiconductor devices, Chem. Eng. J., 2024, 482, 148829 CrossRef CAS
.
- V. H. Vuong, S. V. N. Pammi, S. Ippili, V. Jella, T. N. Thi, K. S. Pasupuleti, M. D. Kim, M. J. Jeong, J. R. Jeong, H. S. Chang and S. G. Yoon, Flexible, stable, and self-powered photodetectors embedded with chemicalvapor deposited lead-free bismuth mixed halide perovskite films, Chem. Eng. J., 2023, 458, 141473 CrossRef CAS
.
- Y. Liu, A. Nag, L. Manna and Z. Xia, Lead-free double perovskite Cs2AgInCl6, Angew. Chem., Int. Ed., 2021, 60, 11592 CrossRef CAS PubMed
.
- H. Yang, X. Chen, H. Lu, Y. Li, W. Sun, Y. Zhang, X. Liu, G. Long, L. Zhang and X. Li, Self-trapped excitons-based
warm-white afterglow by room-temperature engineering toward intelligent multi-channel information system, Adv. Funct. Mater., 2023, 34, 2311437 CrossRef
.
- J. Luo, X. Wang, S. Li, Y. Guo, G. Niu, L. Yao, Y. Fu, L. Gao, Q. Dong, C. Zhao, M. Leng, F. Ma, W. Liang, L. Wang, S. Jin, J. Han, L. Zhang, J. Etheridge, J. Wang, Y. Yan, E. H. Sargent and J. Tang, Efficient and stable emission of warm-white light from lead-free halide double perovskites, Nature, 2018, 563, 541–545 CrossRef CAS PubMed
.
- X. Liu, X. Xu, B. Li, L. Yang, Q. Li, H. Jiang and D. Xu, Tunable dual-emission in monodispersed Sb3+/Mn2+ codoped Cs2NaInCl6 perovskite nanocrystals through an energy transfer process, Small, 2020, 16, 2002547 CrossRef CAS
.
- Z. Liu, Y. Sun, T. Cai, H. Yang, J. Zhao, T. Yin, C. Hao, M. Chen, W. Shi, X. Li, L. Guan, X. Li, X. Wang, A. Tang and O. Chen, Two-dimensional Cs2AgInxBi1−xCl6 alloyed double perovskite nanoplatelets for solution-processed light-emitting diodes, Adv. Mater., 2023, 35, 2211235 CrossRef CAS PubMed
.
- Z. Li, Y. Zhang, Z. Feng, Z. Wang and Z. Zheng, Temperature sensing of novel Tb3+/Ho3+/Bi3+ co-doped lead-free double perovskite Cs2AgInCl6 nanocrystals, J. Lumin., 2024, 268, 120430 CrossRef
.
- Y. Wu, L. Lin, Y. Liu, X. Rong, M. G. Li, M. S. Molokeev, J. Zhao and Z. Xia, Angew. Chem., Int. Ed., 2020, 59, 11634–11640 CrossRef
.
- H. Liu, D. Sun, L. Zhang, X. Lv, W. Cai and Y. Zhang, Incorporating rare-earth terbium(III) ions into Cs2AgInCl6:Bi nanocrystals toward tunable photoluminescence, J. Lumin., 2024, 270, 120567 CrossRef CAS
.
- Y. Zhu, G. Sun, Y. Wang, Y. Sun, X. Xing and M. Shang, Multi-mode excited upconversion and downshifting of Ho3+/Yb3+ doped lead-free double perovskite Cs2NaBiCl6 for anti-counterfeiting and thermometry, Inorg. Chem., 2024, 63, 4438–4446 CrossRef CAS
.
- Y. Wu, L. Lin, P. Lu, W. Ma, Z. Li, M. Zhang, Y. Yang, N. Ju, Y. Zhang, H. Liao, Z. Feng, Z. Wang and Z. Zheng, Potential applications of Tb3+/Ho3+-codoped Cs2AgInCl6 nanocrystals in color codes and encryption, ACS Appl. Opt. Mater., 2023, 1, 1697–1705 CrossRef CAS
.
- X. Duan, K. Nie, Z. Hu, X. Zhang, R. Zhou, W. Dai, L. Mei, L. Wang, H. Wang and X. Ma, Enhancement of orange emission in terbium-doped lead-free halide perovskite for flexible functional fibers and light-emitting diodes, Chem. Eng. J., 2024, 480, 147957 CrossRef CAS
.
- Y. Zhang, Z. Zhang, W. Yu, Y. He, Z. Chen, L. Xiao, J. Shi, X. Guo, S. Wang and B. Qu, Lead-free double perovskite Cs2AgIn0.9Bi0.1Cl6 quantum dots for white light-emitting diodes, Adv. Sci., 2022, 9, 2102895 CrossRef CAS PubMed
.
- H. Xu, J. Liu, Q. Hu, J. Yu, Q. Han and W. Wu, Sb/X (X = Mn, Er, Ho) co-doped lead-free double perovskite Cs2NaInCl6 for single-component white light emission, Adv. Opt. Mater., 2024, 12, 2302288 CrossRef CAS
.
- Y. Wang, P. Dang, L. Qiu, G. Zhang, D. Liu, Y. Wei, H. Lian, G. Li, Z. Cheng and J. Lin, Multimode luminescence tailoring and improvement of Cs2NaHoCl6 cryolite crystals via Sb3+/Yb3+ alloying for versatile photoelectric applications, Angew. Chem., Int. Ed., 2023, 62, e202311699 CrossRef CAS PubMed
.
- S. D. Stranks, R. L. Z. Hoye, D. Di, R. H. Friend and F. Deschler, The Physics of light emission in halide perovskite devices, Adv. Mater., 2019, 31, 1803336 CrossRef CAS
.
- X. Li, D. Wang, Y. Zhong, F. Jiang, D. Zhao, S. Sun, P. Lu, M. Lu, Z. Wang, Z. Wu, Y. Gao, Y. Zhang, W. Yu and X. Bai, Halide double perovskite nanocrystals doped with rare-earth ions for multifunctional applications, Adv. Sci., 2023, 10, 2207571 CrossRef CAS
.
- Z. Jiang, L. He, Z. Yang, H. Qiu, X. Chen, X. Yu and W. Li, Ultra-wideband-responsive photon conversion through co-sensitization in lanthanide nanocrystals, Nat. Commun., 2023, 14, 827 CrossRef CAS PubMed
.
- F. Zhang, X. Chen, X. Qi, W. Liang, M. Wang, Z. Ma, X. Ji, D. Yan, M. Jia, D. Wu, X. Li, Y. Zhang, Z. Shi and C. Shan, Regulating the singlet and triplet emission of Sb3+ Ions to achieve single-component white-light emitter with record high color-rendering index and stability, Nano Lett., 2022, 22, 5046–5054 CrossRef CAS
.
- T. Tian, M. Yang, Y. Fang, S. Zhang, Y. Chen, L. Wang and W. Wu, Large-area waterproof and durable perovskite luminescent textiles, Nat. Commun., 2023, 14, 234 CrossRef CAS PubMed
.
- R. Zhou, C. A. Cheng, X. Wang, K. Nie, J. Wu, M. Wu, X. Duan, Z. Hu, I. Huq, H. Wang, L. Wang, L. Mei, H. Liu and X. Ma, Metal halide perovskite nanocrystals with enhanced photoluminescence and stability toward anti-counterfeiting high-performance flexible fibers, Nano Res., 2023, 16, 3542–3551 CrossRef CAS
.
- H. Wang, Z. Zhu, J. Yuan, H. Wang, Z. Wang, F. Yang, J. Zhan and L. Wang, A new recycling strategy for preparing flame retardants from polyphenylene sulfide waste textiles, Comput. Commun., 2021, 27, 100852 Search PubMed
.
- Y. Peng, J. Ding, X. Guo, Q. Qiu, S. Lu, Y. Wang and B. Ma, Low-temperature catalytic oxidation of PCDD/Fs over MnCeCoOx/PPS catalytic filter, Sci. Pollut. Res., 2023, 30, 120355 CrossRef CAS
.
- E. V. Benvenutti, Y. Gushikem, A. Vasquez, S. C. Castro and G. A. P. Zaldivar, X-Ray photoelectron spectroscopy and mössbauer spectroscopy study of iron(III) and antimony(V) oxides grafted onto a silica gel surface, J. Chem. Soc., Chem. Commun., 1991, 19, 1325–1327 RSC
.
- W. E. Morgan, W. J. Stec and J. R. Wazer, Inner-orbital binding-energy shifts of antimony and bismuth compounds, Inorg. Chem., 1973, 12, 953–955 CrossRef CAS
.
- J. E. Thomaz, K. P. Lindquist, H. I. Karunadasa and M. D. Fayer, Single ensemble nonexponential photoluminescent population decays from a broadband white-light-emitting perovskite, J. Am. Chem. Soc., 2020, 142, 16622–16631 CrossRef CAS
.
- X. Zhang, K. Nie, Z. Hu, R. Zhou, X. Duan, W. Dai, S. Nie, S. Yao, L. Wang, L. Mei, H. Wang, Y. Yao and X. Ma, Facile and effective synthesis strategy for terbium-doped hydroxyapatite toward photoelectric devices and flexible functional fibers, Rare Met., 2024, 43, 1713–1723 CrossRef CAS
.
Footnotes |
† Electronic supplementary information (ESI) available. See DOI: https://doi.org/10.1039/d4tc03773a |
‡ Xiaoman Zhang and Yehua Zheng contributed equally to this work. |
|
This journal is © The Royal Society of Chemistry 2025 |
Click here to see how this site uses Cookies. View our privacy policy here.